- 1Key Laboratory of Space Weather, National Satellite Meteorological Center, China Meteorological Administration, Beijing, China
- 2Innovation Center for FengYun Meteorological Satellite (FYSIC), National Satellite Meteorological Center, China Meteorological Administration, Beijing, China
- 3State Key Laboratory of Numerical Modeling for Atmosphere Sciences and Geophysical Fluid Dynamics (LASG), Institute of Atmospheric Physics, Chinese Academy of Sciences, Beijing, China
- 4Key Laboratory of Radiometric Calibration and Validation for Environmental Satellites, National Satellite Meteorological Center, China Meteorological Administration, Beijing, China
- 5Key Laboratory of Earth and Planetary Physics, Institute of Geology and Geophysics, Chinese Academy of Sciences, Beijing, China
- 6Beijing National Observatory of Space Environment, Institute of Geology and Geophysics, Chinese Academy of Sciences, Beijing, China
- 7College of Earth and Planetary Sciences, University of the Chinese Academy of Sciences, Beijing, China
- 8National Climate Center, China Meteorological Administration, Beijing, China
- 9CMA Earth System Modeling and Prediction Centre, China Meteorological Administration, Beijing, China
The Sun has an obvious quasi-11-year cycle and numerous short-term eruptive activities. There are four processes of energy transmission in the effectuation chain of solar forcing to the climate system: solar energy input into the atmosphere, atmospheric absorption of the input energy, transformation of the absorbed energy into dynamic and thermodynamic responses in the atmosphere, and coupling among all the layers affected by solar forcings. However, the four processes have not been discussed in their entirety. This present paper reviews studies over the last decade on how solar radiation varies during the solar cycle and solar eruptions, and, correspondingly, how the terrestrial atmosphere absorbs the input solar energy.
Introduction
The Sun is a typical middle-aged dwarf star on the main sequence (Tayler, 1997). It emits an electromagnetic spectrum approximating to radiation from a black body at a temperature of 5770K and provides the principal source of energy driving the Earth's atmospheric circulation (Kopp, 2018; Ding, 2019). The Sun has an obvious quasi-11-year cycle and numerous short-term eruptive activities. Whether and how these solar activities affect the Earth’s climate has been studied and debated for hundreds of years. The most recent and realistic problem is whether there is a connection between the stagnation period of global warming in the Northern Hemisphere since the beginning of the 21st century and a decrease in solar activity (Lockwood et al., 2010a; Sirocko et al., 2012), recalling the possible recurrence of scenarios like the Maunder minimum. Although there has been much research on this, it has lacked significance and consistency. Over the past 10 years, due to improvements in solar/terrestrial observation technology and the reconstruction and improvement in quality of long series data, our understanding of the impact of the Sun on climate has made some important progress.
Four processes of energy transmission in the effectuation chain of solar forcing to the climate system are relevant to this discussion: solar energy input into the atmosphere, atmospheric absorption of this input energy, transformation of the absorbed energy into dynamic and thermodynamic responses in the atmosphere, and coupling of all the layers affected by solar forcing. This present paper reviews how solar radiation varies during the solar cycle and eruptions, and, correspondingly, how the terrestrial atmosphere absorbs the input solar energy.
Solar irradiance variations during the solar cycle
The continued observation of total solar irradiance (TSI) from space is recorded by different mission instruments, starting in 1978 (Figure 1). The absolute value of TSI has been newly defined at a low value of approximately 1,361 W/m2, which was first observed by TIM aboard the SORCE mission (Kopp and Lean, 2011) and again by PICARD/PREMOS, NORSAT-1/CLARA, and TSIS-1/TIM. A new laboratory calibration technique with the TSI radiometer facility (TRF) can support end-to-end calibration for irradiance, which explained the difference between VIRGO, ACRIM3, and TIM and corrected the dataset to the same reference level (Kopp et al., 2012). The newest TSI product from FY-3E/SIM-II also shows a value of 1,361.82W/m2 (Zhang, et al., 2022). The TSI climate composite data have been built up with different combined methods showing the same 11-year solar cycle patterns, leading to a TSI variation of approximately 0.1% (Dewitte and Nevens, 2016; Dudok de Wit et al., 2017; Montillet et al., 2022). The long-term TSI trend is still under discussion and is partially limited by the accuracy of historical TSI observations. The precision of instrument on-orbit degradation monitoring has become another key point in solving this problem, especially when the instrument has more than a 10-year lifetime.
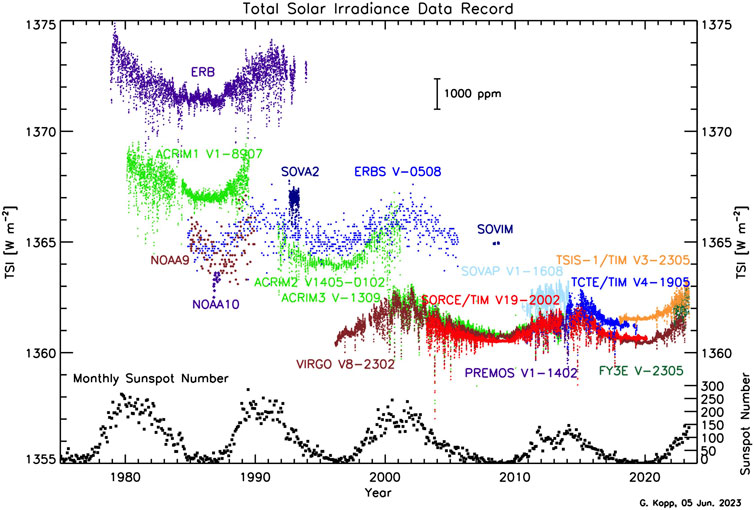
FIGURE 1. Time series of the total solar irradiance dataset and sunspot number (from Greg Kopp’s TSSI Page: https://spot.colorado.edu/∼koppg/TSI/index.html).
The accurate measurement of solar spectral irradiance (SSI) is much more difficult than TSI, especially for absolute calibration and degradation monitoring. The SSI data from different missions usually differ in spectral range and spectral resolution, and also in some gaps in time series. The SORCE mission has given a long time series of approximately 17 years of continued solar spectral irradiance data, which has improved our understanding of the long-term trend of specific wavelength (Woods, et al., 2021). In addition to observations from the upper atmosphere, predictions by theoretical and semi-empirical modeling also give a reference dataset for climate and solar physics application. A time-series comparison between observation and models at a selected wavelength showed that the long-term trend differs much more when it is above 300 nm. Seven spectra which presented the solar minimum state showed differences greater than the instrument pronounced accuracy (Thuillier, et al., 2022). For further improvements, high-accuracy detectors and more robust observation data are needed.
A new solar reference spectrum, TSIS-1 hybrid solar reference spectrum (HSRS), at 0.025 nm resolution from 200 nm to 2,730 nm (Coddington, et al., 2021) was recommended by the committee on Earth observation satellite (CEOS) as the new solar irradiance reference spectrum in March 2022. However, the most violent changes in solar radiation occur at much lower wavelengths and are believed to be major influences on terrestrial climate. The ultra-violet (UV) proportion varies from 6% to 8% over the solar cycle and leads to more ozone and warming during solar maxima in the upper stratosphere (Haigh, 1994; Crooks and Gray, 2005). The magnitudes of UV irradiance variability are wavelength-dependent; emissions differ according to their origins in different layers of the solar atmosphere. Over a solar cycle, chromospheric emissions vary by factors of 1.5–1.7, the transition region and upper chromospheric emissions vary by factors of 1.8–2.4, and coronal emissions vary by a factor of 8 (Woods et al., 2008). Although empirical models have emerged for investigating chromospheric and coronal emissions (Lean et al., 2011), they are contested in the literature (Huang et al., 2016). Due to the data gap and uncertainties surrounding the spectral irradiance observations, UV irradiance variability is still unclear and needs further study.
Solar irradiation variations related to solar activities
The agreement between the variability of TSI and solar activity originated in the evolution of the solar surface magnetic field. Kilogauss-strength magnetic concentrations in the photosphere cyclically emerge in association with dark sunspots and bright faculae on the solar disk (Solanki et al., 2006). Sunspots and pores are dark due to magnetic suppression of the overturning convection around the magnetic concentrations (Rempel and Schlichenmaier, 2011). Lateral heating around the magnetic concentrations overcomes the magnetic suppression to produce increasing brightness (Vögler et al., 2005), and the side walls between the magnetic concentrations and the surrounding heating have a greater view away from the solar disk center (Steiner, 2005). Thus, TSI shows an increasing trend when the active region rotates to the solar limb. Using sunspot and faculae datasets, the observed TSI variability can be reproduced with high accuracy (Lean et al., 2020).
Meanwhile, the effect of flares on TSI is not negligible: large flares make a major contribution to the visible domain (Kretzschmar et al., 2010). During the extraordinary solar storms between 18 October 2003 and 5 November 2003, TSI dropped by an unprecedented 0.34% during this period due to dark sunspots. When an X17 (4B optical) flare erupted on 28 October, TSI increased by 270 ppm in a synthetic result (Woods et al., 2004). However, a possible climate response to the synoptic change of TSI still lacks evidence.
Eruption is another critical form of solar energy export. Burst energy could be carried by radiation, energetic particles, and plasma. Changes during a solar burst at wavelengths shorter than UV are dramatically larger than at longer wavelengths. The initial release of energy during an eruption accelerates charged particles, which precipitate into the denser plasma. This heating of the plasma in the lower solar atmosphere drives an increase of hard X-ray flux via bremsstrahlung. Thereafter, the heated particles confined within the magnetic loops thermally radiate in soft X-rays (SXRs) and in some portions of UV (Neupert, 1968). During large solar flares, the variability of SXR 0.1–14 nm can reach a factor of 150, the EUV region shorter than 115 nm can increase to a factor of 40, the enhancement of FUV 115–200 nm radiation declines toward the longer wavelength, and the total variation is approximately 10% (Woods, 2006). Solar flare irradiance models have been developed to derive the variability of solar irradiance during flares, but much work is required to reconcile the deviation from the observations (Chamberlin et al., 2008; Reep et al., 2022). Radio burst is another violent manifestation of solar eruption. However, it has no direct interaction with our atmosphere.
The physics of the solar atmosphere dominates TSI and SSI variations, as well as the occurrence of solar eruptions. It is the reason why TSI and SSI have the same quasi-11-year variations as solar activity. Although they are highly correlated, TSI and SSI mainly display a decadal variation, while the solar eruptions display as events on timescales of days or shorter. Thus, there is more attention on the solar cycle variation of TSI or SSI when solar forcing on climate is investigated than on eruptive energy changes.
The absorption of the terrestrial atmosphere to solar irradiation at short wavelengths
The absorption of solar visible and infrared radiation is mainly the focus of climatologists. Comparatively, atmospheric absorption of solar radiation at shorter wavelengths is mainly discussed in the domain of space weather. Supplementary Figure S1 shows the altitude of maximum solar radiation absorption by the Earth’s atmosphere for different spectral bands at short wavelengths. These have sometimes been neglected by climate researchers since these absorptions occur in the middle and upper atmosphere. Solar X-ray radiation and extreme UV radiation (wavelengths shorter than 100 nm) are mainly absorbed by the thermosphere; far UV radiation (wavelengths between 100 nm and 200 nm) is absorbed primarily by the thermo- and mesosphere; mid UV radiation (wavelengths between 200 nm and 300 nm) is mostly absorbed by the meso- and stratosphere; near-UV radiation (wavelengths between 300 nm and 400 nm) is primarily absorbed by the strato- and mesosphere; and visible and infrared spectral radiation (wavelengths greater than 400 nm) can reach the troposphere and down to the earth’s surface (including the ocean) (see also Schoeberl and Strobel, 1978; Torr et al., 1980a; Torr et al., 1980b; London, 1980; Brasseur and Solomon, 2005).
The absorption mechanism of solar radiation in the earth’s atmosphere is highly complex. In addition to direct radiation absorption and heating, atmospheric photoionization and photodecomposition directly convert partial radiation energy into atmospheric chemical energy and the kinetic energy of photoelectrons, therefore modulating the absorption efficiency of solar radiation (Roble et al., 1987). The precipitation of high-energy particles (Ree et al., 1983) and Joule heating (Roble and Emery, 1983) related to solar activity can also change the temperature and density in the polar region of the upper atmosphere. Atmospheric chemical, dynamic, thermodynamic, and radiation cooling processes further redistribute absorbed solar radiation energy among different atmospheric layers, thus affecting the temperature structure of the whole atmospheric region (Roble, 1995). To systematically study the solar radiation absorption efficiency of the different Earth atmospheric layers for different spectral bands, a comprehensive study with numerical simulation at its core is necessary (Roble et al., 1987; Roble, 1995; Ren et al., 2009), and three key scientific issues are often the focus. First, the absorption of solar radiation by the earth’s atmosphere usually depends on the atmosphere’s specific chemical composition (Schoeberl and Strobel, 1978; Mertens et al., 1999). Atmospheric chemical composition actually dominates the absorption efficiency of solar radiation, while solar radiation can adjust the atmospheric composition through photochemical processes, affecting the absorption efficiency (Tor and Torr, 1979; Zellner, 1999). Second, atmospheric dynamic processes and heat conduction can directly affect the distribution of atmospheric temperature and can also affect atmospheric chemical processes by transporting the atmosphere’s specific chemical compositions, such as oxygen (Garcia and Solomon, 1994), carbon, hydrogen (Qian et al., 2018), and nitrogen compounds. These processes result in the redistribution of absorbed solar radiation energy between different atmospheric regions and even between different atmospheric layers. Third, the solar radiation energy absorbed by different atmospheric layers of the Earth is often dissipated in the form of long-wave radiation cooling (see López-Puertas and Taylor, 2001 and its references). CO2 (Fomichev et al., 1998), O3 (Fomichev and Shved, 1985), H2O, NO (Kockarts, 1980), and O (Kockarts and Peetermans, 1970) play important roles in long-wave radiation cooling. The coupling between solar radiation absorption, atmospheric radiation cooling, and other mechanisms codetermines the temperature structure of the earth’s atmosphere (Roble, 1995). Changes in the atmospheric radiation cooling process can adjust the effective absorption of solar radiation energy by affecting the temperature and then changing the atmospheric composition (Roble and Dickinson, 1989).
Another factor which may influence the physical processes in the Earth’s atmosphere and change its radiative balance is the flux of cosmic rays. Galactic cosmic rays (GCRs) are influenced by heliospheric magnetic changes, with their intensity peaking during the solar minimum due to quiet solar activity (Fu et al., 2021). SEPs originate in solar eruptions, but their energy is lower than GCR. Large-scale solar wind flow and turbulent interplanetary magnetic fields dominate the transport of cosmic rays and their links to solar activity need more study.
The impact of solar irradiation variation on terrestrial climate
Although the variation range of the total solar radiation is generally considered as one thousandth, the variation ranges of several other influence mechanisms, such as UV radiation and energy particles, are much larger than those of total solar radiation (Gray et al., 2010; Lilensten et al., 2015). Two recent assessment reports from the intergovernmental panel on climate change (IPCC) indicate that, although the total solar radiation mechanism had little impact on modern climate, it could not be ruled out that the Sun might affect the interdecadal change of climate through several indirect radiation amplification mechanisms (IPCC, 2013, 2021). Although there is little consensus on the understanding of the relationship between the Sun and climate on an interdecadal scale (Chiodo, 2019), the observed hiatus in surface warming from 1998 to 2012 may be due to a reduced radiative forcing and a cooling contribution from natural internal variability (medium confidence). The reduced trend in radiative forcing is primarily due to volcanic eruptions and the weakening of solar activity. However, there is low confidence in evaluating the effect of solar forcing on the climate warming hiatus (IPCC, 2013).
In addition, cooling in the tropical upper stratosphere under solar minimum years, which weakens the equator-to-pole temperature gradient, can propagate a solar signal downward to slow tropospheric westerlies, thus causing a negative phase of the arctic oscillation (AO) or NAO and cold winters in Eurasia (Ineson et al., 2011; Kuroda et al., 2022). However, this point needs careful confirmation because, according to reports, there is no significant AO or NAO response to solar irradiance variations on average in the CMIP5 models (Gillett and Fyfe, 2013).
The solar ultraviolet radiation variability recommended by CMIP6 can reach several percent (such as the 200 nm band) in a solar cycle, which is far greater than the TSI variability. Its contribution to the TSI variability has been predicted to increase from 35% of CMIP5 to 50% of CMIP6, and the spectral resolution is high enough. Ozone and atmospheric heating rate changes caused by the solar spectrum are also greatly affected in CMIP6 compared with CMIP5—closer to the latest observations (Matthes et al., 2017). These will help uncover and confirm the response of the middle and lower atmospheres to solar radiation as well as its mechanism. The climate simulation forced by the new SSI input found that the wind field response of the real atmosphere and the resulting climate response are likely to be significantly greater than the model simulation (Ermolli, et al., 2013; Chiodo et al., 2016). In addition, CMIP6 provides a solar-driven energy particle forcing dataset for the first time, which enables it to have a certain ability to simulate high-energy particle forcing (Matthes et al., 2017).
The 11-year cycle is the most stable feature of solar activity variations that can be used to improve interdecadal prediction techniques (Smith et al., 2012; Thieblemont et al., 2015). Previous studies have shown that the 11-year solar cycle change is a source of skills for near-term climate prediction (Dunstone et al., 2016; Kushnir et al., 2019), which has been verified in some regions. The possibility that the stratospheric polar vortex serves as a link between lower atmosphere circulation and solar activity has been noted by Veretenenko and Ogurtsov (2013) and Veretenenko (2022). Dunstone et al. (2016) confirmed that the improvement of the interannual NAO forecasting skills in the North Atlantic region is due to the climate variability of the tropical Pacific and the intensity of the stratospheric polar vortex, which is mainly driven by predictable solar forcing. In the tropical Pacific, verification of the interdecadal modulation of the Sun on Walker circulation has enhanced the confidence of the model in predicting Walker circulation and tropical precipitation (Misios et al., 2019). These results indicate that the prediction method of “Sun+a factor” shows potential to help recent climate prediction, which is consistent with the “drivers of teleconnectivity” framework for interdecadal prediction (Cassou et al., 2018).
One possible way to transform “teleconnectivity” to an understandable causation is to pay more attention to possible climate effects caused by solar eruptions. As discussed previously, the upper and middle atmosphere could vary dramatically and systematically due to solar bursts. A short-term solar eruption can cause dramatic changes in radiation (almost at all bands), CME, flares, and solar wind, which cause strong responses in the Earth’s upper atmosphere (partly bridged by the magnetosphere) (Zhou et al., 2014). The Earth’s upper and middle atmospheric responses include direct radiation absorption and heating, composition change, dynamical change, and electromagnetic change. Such changes can further affect variations in the meridional temperature gradient, vertical propagation of planetary wave, ozone cycle, and upper westerly jets, which can couple with the lower atmosphere (Kodera et al., 2016). However, how this coupling occurs is still poorly understood. For instance, Wang and Zhao (2012) found that the solar cycle modulation on the monsoon rain belt could be attributed to the coupling between the circulations in the upper and lower atmospheres. Nevertheless, how this coupling process occurs and by what mechanism it is amplified and transmitted downward is not very clear (Zhao et al., 2014; Zhao et al., 2017). Furthermore, the fact that most evidence for solar burst impacts on the climate system are around the decadal scale, while the events themselves are of time scales around days or even shorter (or of synoptic scale) means that synoptic-scale factors are often equated to or inundated by irradiation variations since they both vary in synchrony with the solar cycle. Decisive progress could be made in two directions: finding evidence of solar bursts impacts on the climate system at the synoptic scale, and clarifying the coupling processes between/among the dynamical, thermodynamical, chemical, and other processes in the upper, middle, and lower atmospheres.
Conclusion
Both solar irradiation and solar activity have a quasi-11-year period, and these two variations have essential physics connections. Forcing caused by TSI/SSI on the Earth’s climate mainly presents on a decadal scale. Energy surges from the Sun during solar bursts can only slightly change TSI, but these can export energy to the Earth via radiation at bands of EUV and shorter, energetic particles, plasmas, and electro-magnetic fields which dramatically impact the Earth’s upper atmosphere and induce storms there. Whether such storms can couple with the middle and even the lower atmosphere effectively enough to modify the climate system still needs more investigation. However, we can still try to draft a frame diagram for possible routes of solar forcing to climate (Figure 2). As an increment to previous descriptions, this diagram emphasizes more energy transmission through the “space weather” routines from the Sun to the lower terrestrial atmosphere.
At present, solar activity is experiencing a significant decrease after eight strong solar cycles, and some studies suggest that solar activity may even decline to the level of the Maunder minimum by the middle of the 21st century (Lockwood, 2010b; Wang et al., 2010); this may add new uncertainties to future climate change projections. Will this extremely low solar activity period continue to develop after the 24th solar cycle, and will the 11a period change lead to a new cold period (like the Maunder minimum)? These challenging scientific questions are one of the core issues of climate change, and an in-depth study of them will help assess and project future climate risks.
Author contributions
JW: conceptualization, methodology, and writing—reviewing; LZ: drawing, and writing—original draft preparation and editing; ZX, PZ, ZR, WZ, JQ, CH, YX, and YL: Writing—original draft preparation. All authors contributed to the article and approved the submitted version.
Funding
This work is supported by the National Natural Science Foundation of China (42274217 and 42075040).
Conflict of interest
The authors declare that the research was conducted in the absence of any commercial or financial relationships that could be construed as a potential conflict of interest.
Publisher’s note
All claims expressed in this article are solely those of the authors and do not necessarily represent those of their affiliated organizations, or those of the publisher, the editors, and the reviewers. Any product that may be evaluated in this article, or claim that may be made by its manufacturer, is not guaranteed or endorsed by the publisher.
Supplementary material
The Supplementary Material for this article can be found online at: https://www.frontiersin.org/articles/10.3389/feart.2023.1164636/full#supplementary-material
References
Brasseur, G., and Solomon, S. (2005). Aeronomy of the middle atmosphere: Chemistry and physics of the stratosphere and mesosphere, atmospheric and oceanographic sciences library. Berlin, Germany: Springer, 32. doi:10.1007/1-4020-3824-0
Cassou, C., Kushnir, Y., Hawkins, E., Pirani, A., Kucharski, F., Kang, I., et al. (2018). Decadal climate variability and predictability: Challenges and opportunities. Bull. Am. Meteorol. Soc. 99, 479–490. doi:10.1175/BAMS-D-16-0286.1
Chamberlin, P. C., Woods, T. N., and Eparview, F. G. (2008). Flare irradiance spectral model (FISM): Flare component algorithms and results. Space weather. 6 (5), S05001. doi:10.1029/2007SW000372
Chiodo, G., Garcíaherrera, R., Calvo, N., Vaquero, J. M., Añel, J. A., Barriopedro, D., et al. (2016). The impact of a future solar minimum on climate change projections in the Northern Hemisphere. Environ. Res. Lett. 11 (3), 034015. doi:10.1088/1748-9326/11/3/034015
Chiodo, G., Oehrlein, J., Polvani, L. M., Fyfe, J. C., and Smith, A. K. (2019). Insignificant influence of the 11-year solar cycle on the North Atlantic oscillation. Nat. Geosci. 12, 94–99. doi:10.1038/s41561-018-0293-3
Coddington, O. M., Richard, E. C., Harber, D., Pilewskie, P., Woods, T. N., Chance, K., et al. (2021). The TSIS-1 hybrid solar reference spectrum. Geophys. Res. Lett. 48, e2020GL091709. doi:10.1029/2020GL091709
Crooks, S., and Gray, L. (2005). Characterization of the 11-year solar signal using a multiple regression analysis of the ERA-40 dataset. J. Clim. 18, 996–1015. doi:10.1175/JCLI-3308.1
Dewitte, S., and Nevens, S. (2016). The total solar irradiance climate data record. Astrophysical J. 830 (1), 25. doi:10.3847/0004-637x/830/1/25
Ding, Y. H. (2019). Effect of solar activity on Earth’s climate and weather. Meteor. Mon. 45 (3), 297–304. doi:10.7519/j.issn.1000-0526.2019.03.001
Dudok de Wit, T., Kopp, G., Fröhlich, C., and Schöll, M. (2017). Methodology to create a new total solar irradiance record: Making a composite out of multiple data records. Geophys. Res. Lett. 44 (3), 1196–1203. doi:10.1002/2016GL071866
Dunstone, N., Smith, D., Scaife, A., Hermansonet, L., Eade, R., Robinson, N., et al. (2016). Skilful predictions of the winter North Atlantic Oscillation one year ahead. Nat. Geosci. 9, 809–814. doi:10.1038/ngeo2824
Ermolli, I., Matthes, K., de Wit, D., Krivova, N. A., Tourpali, K., Weber, M., et al. (2013). Recent variability of the solar spectral irradiance and its impact on climate modelling. Atmos. Chem. Phys. 13, 3945–3977. doi:10.5194/acp-13-3945-2013
IPCC (2013). “Climate change 2013:the physical science basis,” in Contribution of working group I to the fifth assessment report of the intergovernmental Panel on climate change. T. F. Stocker, D. Qin, and G.-K. Plattner Editors (Cambridge, United Kingdom: Cambridge University Press), 1535.
Fomichev, V. I., Blanchet, J.-P., and Turner, D. S. (1998). Matrix parameterization of the 15 μm CO 2 band cooling in the middle and upper atmosphere for variable CO2 concentration. J. Geophys. Res. 103 (D10), 11505–11528. doi:10.1029/98JD00799
Fomichev, V. I., and Shved, G. M. (1985). Parameterization of the radiative flux divergence in the 9.6-μm O3 band. J. Atmos. Terr. Phys. 47 (11), 1037–1049. doi:10.1016/0021-9169(85)90021-2
Fu, S., Zhang, X., Zhao, L., and Li, Y. (2021). Variations of the galactic cosmic rays in the recent solar cycles. Astrophysical J. Suppl. Ser. 254 (2), 37. doi:10.3847/1538-4365/abf936
Garcia, R. R., and Solomon, S. (1994). A new numerical model of the middle atmosphere: 2, ozone and related species. J. Geophys Res. 99 (12), 12937. doi:10.1029/94JD00725
Gillett, N. A., and Fyfe, J. C. (2013). Annular mode changes in the CMIP5 simulations: Annular mode changes in CMIP5. Geophys. Res. Lett. 40, 1189–1193. doi:10.1002/grl.50249
Gray, L. J., Beer, J., Geller, M., Haigh, J. D., Lockwood, M., Matthes, K., et al. (2010). Solar influences on climate. Rev. Geophys. 48, RG4001. doi:10.1029/2009rg000282
Haigh, J. D. (1994). The role of stratospheric ozone in modulating the solar radiative forcing of climate. Nature 370, 544–546. doi:10.1038/370544a0
Huang, J. P., Hao, Y. Q., Zhang, D. H., and Xiao, Z. (2016). Changes of solar extreme ultraviolet spectrum in solar cycle 24. J. Geophys. Res. Space Phys. 121, 6844–6854. doi:10.1002/2015JA022231
Ineson, S., Scaife, A. A., Knight, J. R., Manners, J. C., Dunstone, N. J., Gray, L. J., et al. (2011). Solar forcing of winter climate variability in the Northern Hemisphere. Nat. Geosic 4 (11), 753–757. doi:10.1038/ngeo1282
Kockarts, G. (1980). Nitric oxide cooling in the terrestrial thermosphere. Geophys. Res. Lett. 7 (2), 137–140. doi:10.1029/GL007i002p00137
Kockarts, G., and Peetermans, W. (1970). Atomic oxygen infrared emission in the earth's upper atmosphere. Space Sci. 18 (2), 271–285. doi:10.1016/0032-0633(70)90163-7
Kodera, K., Thiéblemont, R., Yukimoto, S., and Matthes, K. (2016). How can we understand the global distribution of the solar cycle signal on the earth's surface? Atmos. Chem. Phys. 16 (20), 12925–12944. doi:10.5194/acp-16-12925-2016
Kopp, G. (2018). “Earth’s incoming energy: The total solar irradiance,” in Reference module in earth systems and environmental Sciences. Comprehensive remote sensing (Amsterdam, Netherlands: Elsevier), 32–66. doi:10.1016/B978-0-12-409548-9.10366-5
Kopp, G., Fehlmann, A., Finsterle, W., Harber, D., Heuerman, K., and Willson, R. (2012). Total solar irradiance data record accuracy and consistency improvements. Metrologia 49 (2), S29–S33. doi:10.1088/0026-1394/49/2/S29
Kopp, G., and Lean, J. L. (2011). A new, lower value of total solar irradiance: Evidence and climate significance. Geophys. Res. Lett. 38, L01706. doi:10.1029/2010GL045777
Kuroda, Y., Kodera, K., Yoshida, K., Yukimoto, S., and Gray, L. (2022). Influence of the solar cycle on the North Atlantic oscillation. J. Geophys. Res. Atmos. 127, e2021JD035519. doi:10.1029/2021JD035519
Kushnir, Y., Scaife, A. A., Arritt, R., Balsamo, G., Boer, G., Doblas-Reyes, F., et al. (2019). Towards operational predictions of the near-term climate. Nat. Clim. Change 9, 94–101. doi:10.1038/s41558-018-0359-7
Lean, J. L., Coddington, O., Marchenko, S. V., Machol, J., DeL and, M. T., and Kopp, G. (2020). Solar irradiance variability: Modeling the measurements. Earth Space Sci. 7, 00645. doi:10.1029/2019EA000645
Lean, J. L., Woods, T. N., Eparvier, F. G., Meier, R. R., Strickland, D. J., Correira, J. T., et al. (2011). Solar extreme ultraviolet irradiance: Present, past, and future. J. Geophys. Res. Space Phys. 116, 1102. doi:10.1029/2010JA015901
Lockwood, M., Harrison, R. G., Woollings, T., and Solanki, S. K. (2010a). Are cold winters in Europe associated with low solar activity? Environ. Res. Lett. 5 (2), 024001. doi:10.1088/1748-9326/5/2/024001
Lockwood, M. (2010b). Solar change and climate: An update in the light of the current exceptional solar minimum, Proc. R. Soc. A 466 (2114), 303–329. doi:10.1098/rspa.2009.0519
London, J. L. (1980). “Radiative energy sources and sinks in the stratosphere and mesosphere,” in Atmospheric ozone and its variation and human influences. Proc NATO advanced study (Springfield, Va: NTIS).
López-Puertas, M., and Taylor, F. W. (2001). Non-LTE radiative transfer in the Atmosphere volume 3. Singapore: World Scientific. Available at: https://www.worldscientific.com/doi/abs/10.1142/4650.
Matthes, K., Kruschke, T., Shangguan, M., Barnard, L., Beer, J., Charbonneau, P., et al. (2017). Solar forcing for CMIP6 (v3.2). Geosci. Model. Dev. 10 (6), 2247–2302. doi:10.5194/gmd-10-2247-2017
Matthieu, K., Thierry, D. W., Werner, S., Sabri, M., Jean-François, H., and Dewitte, S. (2010). The effect of flares on total solar irradiance, Nat. Phys. 10, 690–692. doi:10.1038/nphys1741
Mertens, C. J., Mlynczak, M. G., Garcia, R. R., and Portmann, R. W. (1999). A detailed evaluation of the stratospheric heat budget, 1. Radiation transfer. J. Geophys Res. 104, 6021–6038. doi:10.1029/1998JD200100
Misios, S., Gray, L. J., Knudsen, M. F., Haigh, J. D., and Schmidt, H. (2019). Slowdown of the Walker circulation at solar cycle maximum. Proc. Natl. Acad. Sci. 116 (15), 7186–7191. doi:10.1073/pnas.1815060116
Montillet, J.-P., Finsterle, W., Kermarrec, G., Sikonja, R., Haberreiter, M., Schmutz, W., et al. (2022). Data fusion of total solar irradiance composite time series using 41 years of satellite measurements. J. Geophys. Res. Atmos. 127, e2021JD036146. doi:10.1029/2021JD036146
Neupert, W. N. (1968). Comparison of solar X-ray line emission with microwave emission during flares. Astrophys. J. 153, L59. doi:10.1086/180220
Qian, L., Burns, A. G., Solomon, S. S., Smith, A. K., McInerney, J. M., Hunt, L. A., et al. (2018). Temporal variability of atomic hydrogen from the mesopause to the upper thermosphere. J. Geophys. Res. Space Phys. 123, 1006–1017. doi:10.1002/2017JA024998
Reep, J., David, E., and Warren, H. (2022). Solar flare irradiance: observations and physical modeling. The Astrophysi. J. 97, 103. doi:10.3847/1538-4357/ac4784
Rees, M. H., Emery, B. A., Robie, R. G., and Stamnes, K. (1983). Neutral and ion gas heating by auroral electron precipitation. J. Geophys. Res. 88, 6289. doi:10.1029/JA088iA08p06289
Rempel, M., and Schlichenmaier, R. (2011). Sunspot modeling: From simplified models to radiative MHD simulations. Living Rev. Sol. Phys. 8, 3. doi:10.12942/lrsp-2011-3
Ren, Z., Wan, W., and Liu, L. (2009). GCITEM-IGGCAS: A new global coupled ionosphere-thermosphere-electrodynamics model. J. Atmos. Sol. Terr. Phys. 71 (17&18), 2064–2076. doi:10.1016/j.jastp.2009.09.015
Roble, R. G., and Dickinson, R. E. (1989). How will changes in carbon dioxide and methane modify the mean structure of the mesosphere and thermosphere? Geophys. Res. Lett. 16, 1441–1444. doi:10.1029/GL016i012p01441
Roble, R. G., and Emery, B. A. (1983). On the global mean temperature of the thermosphere. Planet Space Sci. 31, 597–614. doi:10.1016/0032-0633(83)90002-8
Roble, R. G. (1995). “Energetics of the mesosphere and thermosphere,” in The upper mesosphere and lower thermosphere: A review of experiment and theory, geophys. Monogr. Ser. Editors R. M. Johnson, and T. L. Killeen (Washington, D.C: Am. Geophys. Union), 1. doi:10.1029/GM087
Roble, R. G., Ridley, E. C., and Dickinson, R. E. (1987). On the global mean structure of the thermosphere. J. Geophys. Res. 92 (A8), 8745–8758. doi:10.1029/JA092iA08p08745
Schoeberl, M. R., and Strobel, D. F. (1978). The zonally averaged circulation of the middle atmosphere. J. Atmos. Sci. 35, 577–591. doi:10.1175/1520-0469(1978)035<0577:tzacot>2.0.co;2
Sirocko, F., Brunck, H., and Pfahl, S. (2012). Solar influence on winter severity in central europe: Rhine freezing. Geophys. Res. Lett. 39 (16), 16704. doi:10.1029/2012GL052412
Smith, D. M., Scaife, A. A., and Kirtman, B. P. (2012). What is the current state of scientific knowledge with regard to seasonal and decadal forecasting. Environ. Res. Lett. 7, 015602. doi:10.1088/1748-9326/7/1/015602
Solanki, S. K., Inhester, B., and Schüssler, M. (2006). The solar magnetic field. Rep. Prog. Phys. 69, 563–668. doi:10.1088/0034-4885/69/3/r02
Steiner, O. (2005). Radiative properties of magnetic elements. II. Center to limb variation of the appearance of photospheric faculae. Astron. Astrophys. 430, 691–700. doi:10.1051/0004-6361:20041286
Thieblemont, R., Matthes, K., Omrani, N. E., Kodera, K., and Hansen, F. (2015). Solar forcing synchronizes decadal North Atlantic climate variability. Nat. Commun. 6, 8268. doi:10.1038/ncomms9268
Thuillier, G., Zhu, P., Snow, M., Zhang, P., and Ye, X. (2022). Characteristics of solar-irradiance spectra from measurements, modeling, and theoretical approach. Light Sci. Appl. 11, 79. doi:10.1038/s41377-022-00750-7
Torr, D. G., and Torr, M. R. (1979). Chemistry of the thermosphere and ionosphere. J. Atmos. Terr. Phys. 41, 797–839. doi:10.1016/0021-9169(79)90126-0
Torr, M. R., Torr, D. G., and Hinteregger, H. E. (1980a). Solar flux variability in the Schumann-Runge continuum as a function of solar cycle 21. J. Geophys. Res. 85, 6063. doi:10.1029/JA085iA11p06063
Torr, M. R., Torr, D. G., and Richards, P. G. (1980b). The solar ultraviolet heating efficiency of the midlatitude thermosphere. Geophys. Res. Lett. 7, 373–376. doi:10.1029/GL007i005p00373
Veretenenko, S., and Ogurtsov, M. (2013). The stratospheric polar vortex as a cause for the temporal variability of solar activity and galactic cosmic ray effects on the lower atmosphere circulation. J. Phys. Conf. Ser. 409, 012238. doi:10.1088/1742-6596/409/1/012238
Veretenenko, S. (2022). Stratospheric polar vortex as an important link between the lower atmosphere circulation and solar activity. Atmosphere 13(7):1132. doi:10.3390/atmos13071132
Vögler, A., Shelyag, S., Schüssler, M., Cattaneo, F., Emonet, T., and Linde, T. (2005). Simulations of magneto-convection in the solar photosphere. Equations, methods, and results of the MURaM code. Astron. Astrophys. 429, 335–351. doi:10.1051/0004-6361:20041507
Wang, J. S., and Zhao, L. (2012). Statistical tests for a correlation between decadal variation in June precipitation in China and sunspot number. J. Geophys. Res. 117 (D23), D23117. doi:10.1029/2012JD018074
Wang, S. W., Wen, X. Y., and Huang, J. B. (2010). Global cooling in the immediate future? Chin. Sci. Bull. 55, 3847–3852. doi:10.1007/s11434-010-4177-1
Woods, Thomas N., Kopp, Greg, and Chamberlin, Phillip C. (2006). Contributions of the solar ultraviolet irradiance to the total solar irradiance during large flares. J. Geophys. Res. 111, A10S14. doi:10.1029/2005JA011507
Woods, T. N., Eparvier, F. G., Fontenla, J., Harder, J., Kopp, G., McClintock, W. E., et al. (2004). Solar irradiance variability during the October 2003 solar storm period. Geophys. Res. Lett. 31, L108021–L108024. doi:10.1029/2004GL019571
Woods, T. N., Harder, J. W., Kopp, G., McCabe, D., Rottman, G., Ryan, S., et al. (2021). Overview of the solar radiation and climate experiment (SORCE) seventeen-year mission. Sol. Phys. 296 (127), 127. doi:10.1007/s11207-021-01869-3
Woods, T. N. (2008). Recent advances in observations and modeling of the solar ultraviolet and X-ray spectral irradiance. Adv. Space Res. 42, 895–902. doi:10.1016/j.asr.2007.09.026
Zellner, R. (1999). “Chemistry of the stratosphere,” in Global aspects of atmospheric chemistry (Berlin, Germany: Springer Verlag).
IPCC (2021). “Summary for policymakers,” in Climate change 2021: The physical science basis. Contribution of working group I to the sixth assessment report of the intergovernmental Panel on climate change Editors P. Zhai, A. Pirani, S. L. Connors, C. Péan, S. Berger, N. Caudet al. (Cambridge, United Kingdom: Cambridge University Press), 3–32. doi:10.1017/9781009157896.001
Zhang, P., Hu, X. Q., Lu, Q. F., Zhu, A. J., Lin, M. Y., Sun, L., et al. (2022). FY-3E: The first operational meteorological satellite mission in an early morning orbit. Adv. Atmos. Sci. 39, 1–8. doi:10.1007/s00376-021-1304-7
Zhao, L., Wang, J., Liu, H., and Xiao, Z. (2017). Amplification of the solar signal in the summer monsoon rainband in China by synergistic actions of different dynamical responses. J. Meteor. Res. 31 (1), 61–72. doi:10.1007/s13351-016-6046-6
Zhao, L., and Wang, J. (2014). Robust response of the East Asian monsoon rainband to solar variability. J. Clim. 27 (8), 3043–3051. doi:10.1175/JCLI-D-13-00482.1
Keywords: solar activity, climate, energy transmission, solar radiation, high-and low-level atmosphere coupling
Citation: Wang J, Zhao L, Xiao Z, Zhang P, Ren Z, Zong W, Qi J, Huang C, Xu Y and Lu Y (2023) Energy transmission processes in the effectuation chain of solar forcing to the terrestrial atmosphere—a review. Front. Earth Sci. 11:1164636. doi: 10.3389/feart.2023.1164636
Received: 13 February 2023; Accepted: 28 July 2023;
Published: 23 August 2023.
Edited by:
Yan Liu, Xi’an University of Architecture and Technology, ChinaReviewed by:
Maxim Ogurtsov, Ioffe Institute (RAS), RussiaCopyright © 2023 Wang, Zhao, Xiao, Zhang, Ren, Zong, Qi, Huang, Xu and Lu. This is an open-access article distributed under the terms of the Creative Commons Attribution License (CC BY). The use, distribution or reproduction in other forums is permitted, provided the original author(s) and the copyright owner(s) are credited and that the original publication in this journal is cited, in accordance with accepted academic practice. No use, distribution or reproduction is permitted which does not comply with these terms.
*Correspondence: Jingsong Wang, d2FuZ2pzQGNtYS5nb3YuY24=