- 1Department of Earth and Planetary Sciences, Stanford University, Stanford, CA, United States
- 2College of Earth, Oceanic, and Atmospheric Sciences, Oregon State University, Corvallis, OR, United States
Named for the Andes, andesites (53%–63% SiO2) are the archetypal magma erupted at magmatic arcs. They have been established as the average composition of continental crust and as such are integral to the growth and evolution of the continental crust. However, andesites are quite variable in trace element and isotopic composition reflecting disparate paths of origin. Herein we return to the original site of their identification, the Central Andes, and use a comprehensive dataset of published and unpublished trace elements and isotopes to show that during the past 6 Myr two distinct types of andesite have erupted in the Central Volcanic Zone (CVZ), which correspond with different geodynamic conditions. Consistent with previous work, we confirm that major composite cones and minor centers of the steady state (low magmatic flux) Quaternary CVZ arc have trace element and isotopic characteristics consistent with magma generation/fractionation in the lower crust. Within the Quaternary arc centers, there are also significant latitudinal variations that correspond with the age, composition, and P-T conditions of the lower crust. However, in contrast to this prevailing model, in the 21–24°S segment 6–1 Ma andesites from ignimbrites and lava domes associated with the peak of the regional Neogene ignimbrite flare-up have compositions that indicate these andesites are hybrids between mantle-derived basalts and upper crustal lithologies. Since ∼1 Ma, andesites in young silicic lava domes associated with the regional flare-up are compositionally indistinguishable from proximal Quaternary arc centers, indicating a return to steady-state magmatism and lower crustal production of andesites. We propose that the transition from upper crustal to lower crustal andesite production results from a decrease in mantle heat input and subsequent relaxation of the regional geotherm during the waning of the flare-up event. The two modes of andesite production have significant implications for the production and evolution of the CVZ arc crust. During the flare-up, prodigious amounts of basalt were emplaced into the mid-crust, resulting in the production of large volumes of hybrid intermediate magmas in the mid and upper crust. In contrast, the lower crustal differentiation recorded in the Quaternary steady state arc andesites would result in the formation of a dense crystalline residue in the lower crust and an overall densification of the lower crust. Over time, gravity instabilities associated with this densification may ultimately aid in the delamination of the dense lower crustal root, triggering flare-ups. These differences in andesite production may help explain the cyclicity (flare-up cycles) observed in mature continental arcs and emphasizes that andesite is not a monotonous composition and can vary with depth-dependent intra-crustal differentiation related to magmatic flux.
Introduction
Andesite was a name first coined in 1836 by German geologist Leopold von Buch to describe “trachytes” from the Central Andes of Chile and Bolivia (Thorpe, 1982). Originally, andesites were defined as an intermediate volcanic rock containing between 53% and 63% SiO2 (Thorpe et al., 1981; Gill, 1981). More recently, this classification was sub-divided (see Le Bas and Streckeisen, 1991), based on major element chemistry, into basaltic andesite (53–57 wt.% SiO2) and andesite (57–63 wt.% SiO2) rock types. Andesites are the most common magma type erupted at continental magmatic arcs and are compositionally analogous to the bulk composition of the continental crust on Earth (Taylor and McLennan, 1985). These compositional similarities have led to the recognition that andesites play a critical role in the evolution and growth of continental crust (e.g., Thorpe et al., 1981; Thorpe, 1982; Taylor and McLennan, 1985; Reubi and Müntener, 2022). However, although andesites have been studied for two centuries, there is still debate surrounding whether continental arc andesites are produced in the subarc mantle (see Straub et al., 2011 and references therein) or within the overlying continental crust (e.g., Eichelberger, 1978; Hildreth and Moorbath, 1988). Importantly, these two scenarios differ significantly with respect to the transfer of mass from the Earth’s mantle to the crust, the rate of crustal growth, and the long-term recycling/fractionation of continental crust.
The Central Andes, the home of andesite, are a unique natural laboratory because the arc has been active and erupting andesitic compositions since the Jurassic (Thorpe et al., 1981; Rogers and Hawkesworth, 1989) and has an episodic history related to major tectonic changes that have impacted the production and storage of magma in the region (Coira et al., 1982; Kay and Coira, 2009). Much of this long history is characterized by andesitic magmatism and the construction of a volcanic arc dominated by composite volcanoes (Coira et al., 1982; Kay and Coira, 2009) classified as normal, low-flux, or steady state (e.g., de Silva, 2008; Burns et al., 2015). However, starting in the middle Miocene, ∼25 Ma, changes in the geometry of the subducting plate triggered a high flux magmatic “flare-up” event during which prodigious volumes of dacite were erupted forming one of the largest silicic volcanic provinces on Earth (de Silva et al., 2006; Kay and Coira, 2009; Freymuth et al., 2015). Since the Quaternary, the arc has returned to steady-state activity represented by the modern active volcanic arc (Burns et al., 2015). These magmatic excursions connote fundamental changes in the role of andesite magmatism with significant implications for the production and potential destruction of continental crust. For instance, it has been proposed that >50% of new continental crust created at convergent boundaries is created during flare-up events (Coleman and Glazner, 1997). This is at odds with previous studies that have hypothesized that flare-up events are associated with the delamination of deep mafic cumulates from the lower crust resulting in a net loss of crust (e.g., Kay and Kay, 1993; Kay and Coira, 2009; de Silva and Kay, 2018).
In this study, we seek to understand the evolution of continental crust in the Central Andes using the composition of erupted andesites. We focus on basaltic andesites and andesites (53–63 wt.% SiO2; herein referred to as andesites; Le Bas and Streckeisen, 1991) erupted in the last 6 Ma as they record two distinct modes of andesite production that correspond to distinct geodynamic states and specific magmatic processes occurring at distinct levels in the crust. We show that andesites are not a monotonous group and have distinct trace element and isotopic compositions depending on their depth of production as a function of the arc magmatic flux. A corollary of this is that the location at which andesites are produced has a significant impact on the production and long-term differentiation of continental crust.
Geological background
The Central Volcanic Zone (CVZ) is one of four active volcanic arc segments that make up the Andean Cordillera in South America (Stern, 2004). Subduction-related arc magmatism has occurred continuously in the CVZ for the past ca. 200 Ma in response to the subduction of the Nazca plate beneath the South American continent (Thorpe et al., 1981; Coira et al., 1982). At ∼25 Ma, magmatism in the CVZ migrated eastward and then back west to its current position in response to changes in the dip of the subducting Nazca plate. The arc migration coincided with crustal shortening, which continued until at least 10 Ma, resulting in crustal thicknesses >70 km beneath parts of the CVZ (Allmendinger et al., 1997). The later history of crustal shortening was broadly synchronous with a dramatic shift in the style and characteristics of magmatism in the CVZ, as an arc-scale magmatic flare-up resulted in the production and eruption of large volumes of silicic “crustal” magmas in the CVZ. The most intense surface expression of this is the Altiplano-Puna Volcanic Complex (APVC) between 21 and 24°S (Figure 1; de Silva, 1989). Between 10 and 1 Ma, estimated magmatic fluxes appear to have increased by more than an order-of-magnitude relative to steady-state CVZ magmatism (de Silva et al., 2006; de Silva and Gosnold, 2007; Burns et al., 2015), resulting in the eruption of >15,000 km3 of mainly crystal-rich dacite magma (de Silva and Gosnold, 2007). After the peak of the flare-up, ∼4 Ma, eruptive activity in the region appears to start waning and the re-emergence of composite volcanoes, small-volume lava domes, and volumetrically dominant andesite (de Silva et al., 1994; Watts et al., 1999; Grunder et al., 2008; Tierney et al., 2016; Godoy et al., 2019) has signaled a return to steady-state conditions (Burns et al., 2015; Burns et al., 2020). In addition to major tectono-magmatic events causing arc-scale changes in crustal architecture and processes, the CVZ arc is built on multiple temporally and compositionally distinct crustal domains that play a significant role in the generation and evolution of the subduction-derived magmas (e.g., Barreiro and Clark, 1984; Aitcheson et al., 1995; Wörner et al., 1994; Mamani et al., 2008).
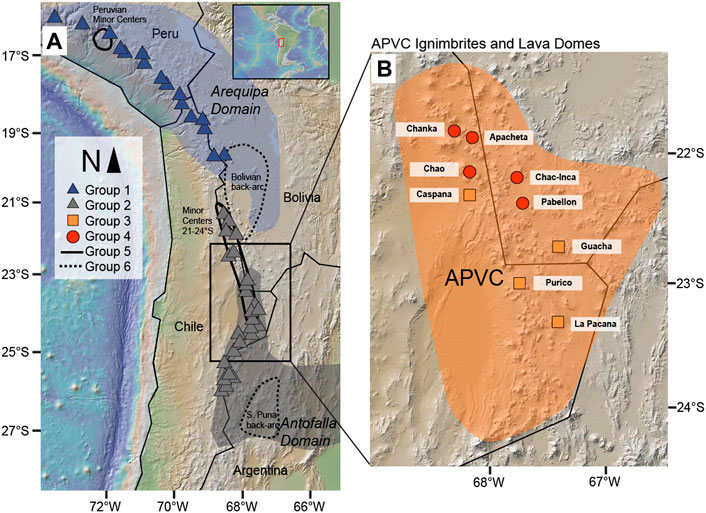
FIGURE 1. Maps showing the various types of andesite-bearing eruptive centers in the CVZ. Diagram includes Symbols for all panels are explained in panel D. These include major eruptive centers (i.e., composite cones) associated with the Quaternary arc (groups 1 and 2), eruptive centers associate the peak of the APVC ignimbrite flare-up (group 3), small volume centers associated with the waning stages of the APVC flare-up (group 4), minor centers associated with the arc (group 5), and minor centers found behind the arc (group 6). The large blue and grey fields in (A) show differences in the proposed basement terrains that underly the two arc segments (Mamani et al., 2010). Map in (B) shows the distribution of APVC flare-up related ignimbrites and domes (orange field) and locations of APVC units discussed in the text.
Methods and data filtration
In this paper we compile all available geochemical data from major and minor centers from Quaternary arc and back-arc centers in the CVZ. We also present 17 new major and trace element analyses, and three new 87Sr/86Sr isotope measurements from the Purico-Chascon volcanic complex in N. Chile. New samples are restricted to the 1 Ma Purico ignimbrite, Cerro Purico lava dome, and Dome D (Hawkesworth et al., 1982; Schmitt et al., 2001; Burns et al., 2015; Burns et al., 2020). These new analyses represent andesites associated with the peak of the regional ignimbrite flare-up and fill a critical gap in understanding of andesites in the CVZ.
The new major element and select trace element concentrations were measured at the Washington State University Geoanalytical Laboratory. Major and select trace element concentrations were collected using a ThermoARL X-ray fluorescence (XRF) spectrometer, and rare-earth element concentrations were measured using an Agilent 7700 inductively coupled plasma mass spectrometer (ICP-MS). A detailed methodology is presented by Johnson et al. (1999). Whole-rock powders from three samples were analyzed for 87Sr/86Sr using thermal ionization mass spectrometry (TIMS) at New Mexico State University following the methodology of Ramos (1992). Although new data was collected as part of this work, it is important to emphasize that the Purico-Chascon samples are not the primary focus of this work, but rather help to fill out a critical group of samples that are paramount to this study (i.e., flare-up andesites).
We have limited our compiled database to samples with SiO2 contents between 50 and 63 wt.% SiO2 (volatile-free). In addition to including large composite volcanoes associated with the arc front, we also include geographically related minor centers and small volcanic fields. These centers and small fields include within-arc minor centers from both major arc segments (16–19°S and 21–26°S), back-arc centers from Bolivia (19–21°S) and back-arc centers from the Southern Puna in Argentina (25–27°S). Although we have compiled a comprehensive chemical database as part of the study, we have omitted REE concentration measured via instrumental neutron activation analysis from figures and discussion when possible. This is due to a lack of reproducibility when compared with replicate analyses made using modern ICP-MS techniques. We have also excluded samples from the northern Puna back-arc (Maro et al., 2017) as they erupt through older, more radiogenic crust. The complete dataset, including references, are provided in the Supplementary Data Table.
Results
Six different groups of andesites can be identified based on their spatiotemporal distributions, surface morphologies, eruptive characteristics (i.e., explosive vs. effusive), and compositions (Figures 1–3): 1) Andesitic lavas erupted from the major Quaternary arc composite cones between 19 and 16 °S; 2) Quaternary arc andesites from major composite cones between 21 and 26°S; 3) Ubiquitous but volumetrically minor lavas and pumices of calderas and ignimbrites erupted during the peak of the APVC flare-up from 6 to 1 Ma (herein referred to as peak flare-up); 4) Andesite enclaves and mingled magma in lava domes and ignimbrites from the waning stage of the flare-up <1 Ma (herein referred to as waning flare-up); 5) Andesites from minor centers spatially associated with the Quaternary arc; 6) Andesites from Quaternary back-arc minor centers.
Quaternary arc andesites of the CVZ (groups 1 and 2)
Quaternary arc andesites from the CVZ define two compositionally distinct groups that correlate with latitude (e.g., Wörner et al., 1992; Wörner et al., 1994; Mamani et al., 2008; Mamani et al., 2010). Andesites from between 16 and 19°S (Group 1) have higher total alkali and lower CaO concentrations at a given SiO2 content when compared to andesites from 21 to 26°S (Group 2; Figure 2). Group 1 andesites also have distinct REE concentrations characterized by much higher LREE abundances and considerably steeper REE patterns (Figure 4; i.e., higher La/Yb, La/Sm, and Sm/Yb ratios). REE abundances also show strong correlations with Sr/Y (Figure 3B), with group 1 andesites displaying considerably higher Sm/Yb and Sr/Y than their counterparts in group 2. Isotopically, there are similarities between andesites from the two segments defining a near-vertical trend in 87Sr/86Sr vs. trace element ratio-space (Figures 3B,C).
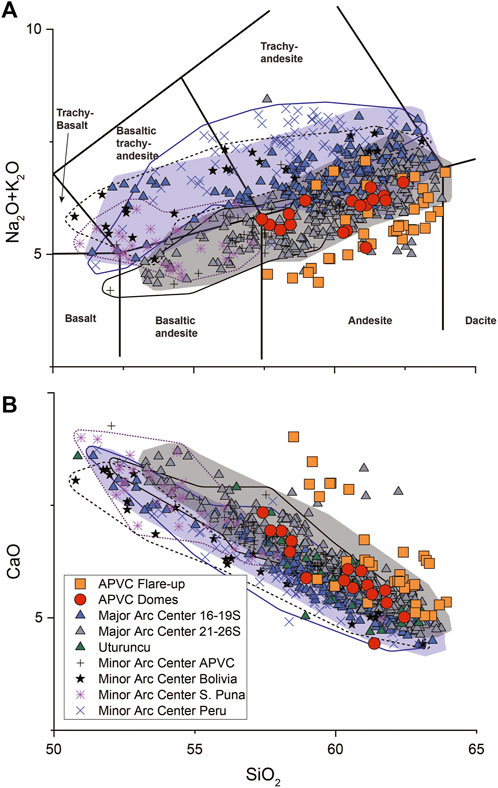
FIGURE 2. (A) Plot of SiO2 vs. total alkali concentrations and (B) SiO2 vs. CaO concentrations. Panel A includes TAS classification grid following Le Maitre (1989). All plotted data has been filtered to only include SiO2 concentrations between 50 and 63 wt.% SiO2. Description of symbols and units for all panels are included in panel (B). Individual groups are outlined to aid in visualization.
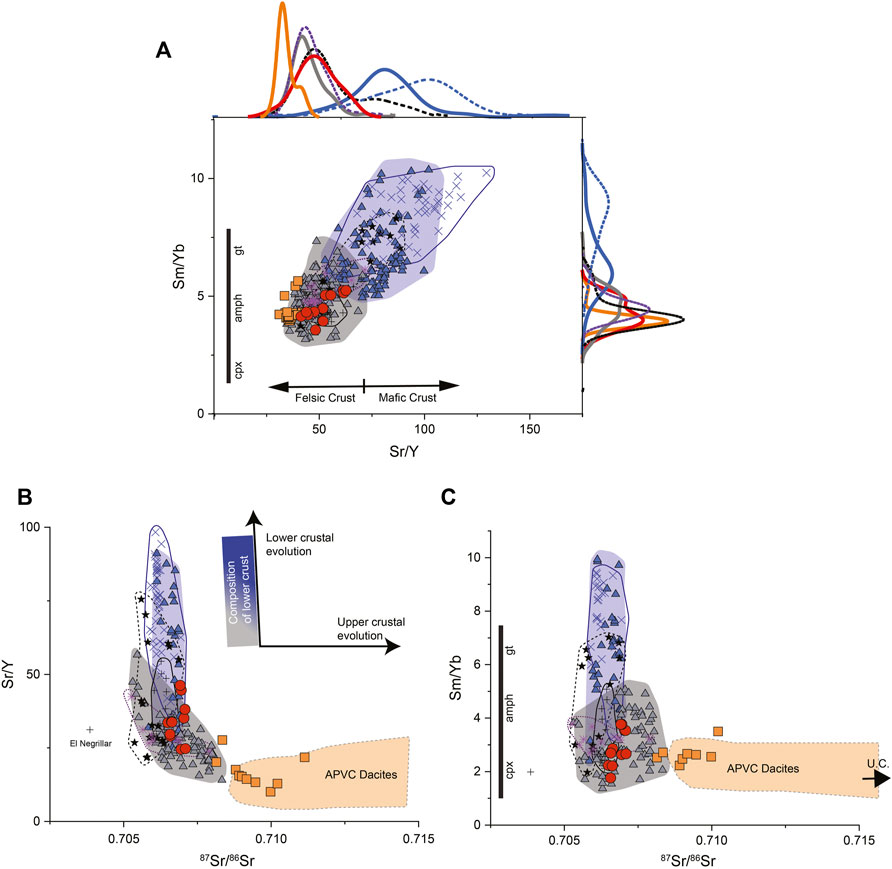
FIGURE 3. (A) Plot showing Sr/Y vs. Sm/Yb. Kernel density estimates (KDEs) for each trace element ratio and group are presented on the top and right side of the plot. KDE bandwidths were calculated using the Silverman method. Arrows show expected Sr/Y ratios in mafic vs. felsic lower crust. The vertical line shows the pressure-dependent relationship between Sm/Yb ratios and pyroxene, amphibole, and garnet after Kay and Mpodozis (2001). (B, C) Diagrams show 87Sr/86Sr vs. the trace element ratios in panel (A). Both panels include an orange field showing the compositions of flare-up related dacite ignimbrites from the APVC. Arrows in Figure 3B show the mineralogically and compositionally dependent evolutionary pathways of lower vs. upper crustal magmas. The vertical bar in Figure 3C is discussed in the text.
Andesites of the peak APVC flare-up 6 Ma to 1 Ma (group 3)
Andesites from the peak of the APVC flare-up (group 3) are compositionally distinct from other andesites in the CVZ. Group 3 andesites define a relatively narrow range of silica contents (58–63 wt.% SiO2) and show no obvious trends when plotted against other major elements (Figure 2). One of the more striking features of the flare-up andesites is that they there show considerably more major element variations at a given SiO2 concentration. This is illustrated in Figure 2A where total alkali concentrations vary by ∼2 wt.% between 59 and 60 wt.% SiO2. Group 3 andesites also have some of the lowest total alkali concentrations in CVZ.
The group 3 andesites also have distinct trace element characteristics relative to the rest of the CVZ. These differences are pronounced on the chondrite normalized trace element diagrams presented in Figure 4. The group 3 andesites have relatively flat REE patterns which are recorded in significantly lower La/Yb and La/Sm and lower Sr/Y than other major and minor centers in the CVZ. The shallow REE patterns are also characterized by relatively high HREE abundances relative to the group 1 and group 2 andesites. One of the most compelling compositional features observed in the group 3 andesites are their elevated Sr isotope ratios. (87Sr/86Sr = .708–.711). They are considerably higher than any of the other andesites in the CVZ, including the waning stage APVC (group 4) andesites (.7055–.7060). These differences result in two distinct trends in Sr isotope-trace element ratio-space (Figures 3B,C). While most of the CVZ andesites show major variations in Sr/Y and Sm/Yb at relatively constant 87Sr/86Sr, group 3 andesites show significant variations in Sr isotope ratios with only minimal changes in Sr/Y and Sm/Yb.
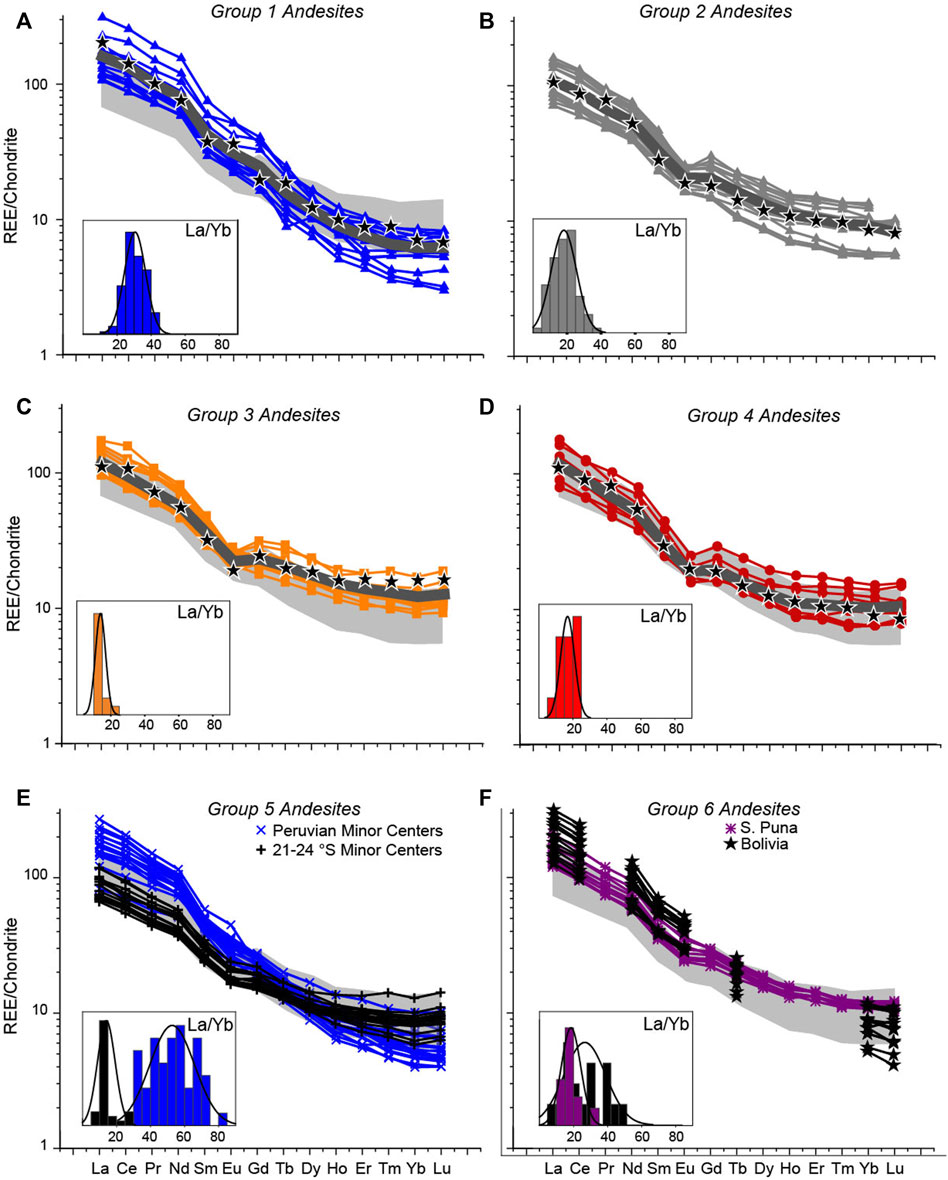
FIGURE 4. Chondrite-normalized (Sun and McDonough, 1989) REE diagrams showing compositional differences between CVZ andesites. The thick black lines represent averages, and the black stars show the crustal melting/mixing models discussed in the text and in Supplementary Appendix SA1. The inset boxes show the distribution(s) of La/Yb ratios in each group of andesite. The La/Yb histograms are overlain with normal distribution curves. Note that the group 1 andesites have considerably steeper REE patterns than other CVZ andesites. The grey field on panels (A, C, D, E, and F) show the range of REE patterns from group 2 (B).
Waning stage APVC andesites <1 Ma (group 4)
The <1 Ma andesites from the waning stage of the APVC are distinct from the flare-up andesites and show considerable compositional overlap with the andesites of group 2 (21–26°S). These andesites have significantly steeper REE patterns (i.e., higher La/Yb, La/Sm, and Sm/Yb ratios) relative to the group 3 andesites with concentrations that are broadly similar to the andesites of group 2 (Figure 4). Andesites from group 4 define tight vertical trends in Figures 3B,C characterized by significant variations in Sr/Y and Sm/Yb with no significant change in Sr isotope ratios.
CVZ minor center andesites (group 5)
Andesites from minor centers in the CVZ show a high degree of compositional diversity that correlates with latitude. Arc minor centers located in Peru are very similar to the major composite cones in the region (group 1). These andesites show elevated total alkali concentrations at a given SiO2 concentration, enriched LREE abundances/steep REE patterns, high Sr/Y concentrations, and Sr isotope ratios indistinguishable from the major composite cones. The only notable difference between the Peruvian minor centers and their major arc counterparts (i.e., group 1) is that the minor centers appear to be slightly more enriched in incompatible elements resulting in slightly higher LREE and incompatible element abundances (Figure 4).
Arc minor centers near the APVC between 21 and 24°S have lower total alkali concentrations, and higher CaO than the Peruvian centers. The minor center andesites also have relatively shallow REE patterns and low Sr/Y ratios, appearing indistinguishable from major arc centers between 21 and 26°S (Figures 3, 4). These andesites are also isotopically indistinguishable from group 2 andesites. One of the minor centers, Cerro Overo, is worth discussing in further detail. Although lavas from Cerro Overo are similar to other minor centers in the region with, bulk compositions ranging from ∼52 to 57 wt.% SiO2, olivine-hosted melt inclusions in the lavas record some of the most primitive mafic compositions erupted in the CVZ. The olivine-hosted melt inclusions are high-Mg basalts and have the lowest 87Sr/86Sr ratios (<.7040) recorded in the CVZ during the past 6 Ma (van Alderwerelt et al., 2021).
CVZ back-arc andesites (group 6)
In this study, we also include back-arc centers from two regions: 1) lavas from the Bolivian back-arc between 19 and 21°S and 2) andesites from the Southern Puna Plateau in Argentina (25–27°S). Andesites from the Southern Puna are the most compositionally restricted group included in this study. There do not appear to be any systematic variations between SiO2 and total alkali concentrations. Andesites from the Southern Puna back-arc define steep REE patterns characterized by enrichments in LREEs (Figure 4). However, they differ from the group 1 and group 2 andesites as they do not show a depletion in HREEs. Isotopically, these andesites are indistinguishable from andesites from the major arc centers. Andesites from the Bolivian back-arc are perhaps the most interesting minor center lavas included in this study. Not only do they occupy an area in space that is transitional between the two major arc segments in the CVZ, but they are also compositionally unique. In major element space (Figure 2), they overlap with group 1 andesites. However, REE abundances and Sr/Y ratios span the compositional gap between the two arc segments (Figures 3, 4).
Discussion
In the following sections, we discuss specific trace elements and trace element ratios that record the presence of distinct crystalline phases during the fractionation/evolution of the arc-related magmas. Central to our discussion of andesite generation in the CVZ are the ratios of Sr/Y and Sm/Yb. Both ratios are influenced by multiple crystalline phases (i.e., garnet, pyroxene, and plagioclase) present in the early evolution of CVZ magmas, and both ratios are highly sensitive to the presence of garnet, providing constraints on the depths and mechanisms responsible for early fractionation events (Chapman et al., 2015; Kay and Mpodozis, 2002; Wörner et al., 2018). For example, Kay and Mpodozis (2002), following Kay and Kay (1991), proposed that Sm/Yb variations in mafic to intermediate magmas in the Central Andes are mostly controlled by pressure-dependent changes from clinopyroxene to amphibole to garnet stability in the mineral residue in equilibrium with evolving magmas. Owing to the sensitivity of these ratios to the composition of the mineral residuum, they serve as critical tools for distinguishing upper versus lower crustal differentiation the CVZ. We also develop isotopically constrained trace element and AFC models to help fingerprint the sources and locations at which various magmatic processes are occurring within the CVZ crust (for a detailed description of the modeling techniques and parameters, see appendix 1). Thus far, we have discussed six distinct groups of andesites based largely on spatiotemporal distributions, surface morphologies, and eruptive characteristics (i.e., explosive vs. effusive). In the previous section, we provided a detailed comparison of the compositional similarities and differences between these groups. Following this comparison, we can now re-group the andesites into two groups based on compositional characteristics. Importantly, the compositional differences between the two groups appear to record disparate evolutionary pathways controlled by arc-scale geodynamic changes.
Upper Crustal andesites: The APVC peak flare-up (group 3)
Group 3 andesites (peak APVC flare-up) are compositionally distinct from all the other andesites in the CVZ and show strong upper crustal affinities characterized by distinctly lower Sr/Y and Sm/Yb and considerably higher 87Sr/86Sr ratios. The physical and compositional characteristics of ignimbrites of the APVC have been studied in detail (e.g., Schmitt et al., 2001; Lindsay et al., 2001; de Silva et al., 2006; Kay et al., 2010; Brandmeier and Wörner, 2016; Grocke et al., 2017; 2018). However, most of this work has been focused on the large, crystal-rich, high-K dacite ignimbrites that dominate the region (de Silva, 1989; de Silva et al., 2006; Salisbury et al., 2011). Dacites associated with the peak of the APVC flare-up lack the HREE depletion characteristic of magmas derived in the garnet-bearing lower crust (e.g., Schmitt et al., 2001; de Silva et al., 2006), and Schmitt et al. (2001) argued that this observation paired with Sr trace element systematics in 1.0 Ma Purico ignimbrite constrain the region of melt production/fractionation for the APVC ignimbrites to between 15 and 35 km. Importantly, isotope mixing models indicate that the large dacite ignimbrites of the APVC require at least 50% mid to upper crust (Lindsay et al., 2001; Schmitt et al., 2001; Soler et al., 2007; Kay et al., 2010; Freymuth et al., 2015). Andesites associated with the APVC (group 3) share some compositional similarities with dacite ignimbrites from the APVC (i.e., low Sr/Y and Sm/Yb). However, elevated 87Sr/86Sr isotope ratios and mineral associations and compositions indicate that the dacites experienced protracted histories in the upper crust (4–8 km depth; de Silva et al., 1994; Lindsay et al., 2001; Schmitt et al., 2001; Soler et al., 2007; Burns et al., 2015). In contrast, the APVC andesites have bulk rock and mineral compositions that appear to record a snapshot into the deeper reaches of the mid to upper crustal magmatic system.
Two-pyroxene thermobarometry from the Purico andesite (group 3) pumice yielded a total range in crystallization/equilibration pressures from 6 to 11 kbars (22–40 km depth) with a higher-pressure subset between 8 and 11 kbars, the former corresponding to crustal depths between 29 and 40 km (see Supplementary Figure S3). Rare-earth element patterns recorded in the group 3 andesites are nearly indistinguishable from the Paleozoic metamorphic rocks that are thought to make up the upper crust in the region (Lucassen et al., 2001). While this could indicate that the andesites are pure crustal melts, this would require nearly 100% melting and the bulk composition would have silica contents exceeding 68% (Lucassen et al., 2001). In addition, the range of 87Sr/86Sr isotope ratios recorded in the andesites are far lower and more restricted than the regional upper crustal basement (.7081–.7111 vs. .7241–1.123). Most of the group 3 andesites do not display significant Eu anomalies (Figure 4), and the few samples that do, we interpret as overprinting due to plagioclase fractionation at higher levels in the upper crust. This is consistent with plagioclase becoming a stable phase at ∼20–17 km depth (Burns et al., 2015). The apparent lack of plagioclase in the host rock is also interesting, as the upper crust in the region is thought to be dominated by plagioclase-bearing gneiss (Lucassen et al., 2001). However, dehydration melting experiments conducted on biotite + plagioclase + quartz gneiss starting compositions (Gardien et al., 2000) show that at 10 kbars plagioclase is readily consumed in hydrous (4 wt.% added H2O), high temperature (900°C) conditions. Beneath the APVC, increased mantle heat input in response to large-scale geodynamic changes (see de Silva et al., 2006; Kay and Coira, 2009; de Silva and Kay, 2018) would have resulted in the ascent and accumulation of large volumes of basalt in the lower to middle crust (∼55–30 km). This basalt would provide the thermal energy required to initiate both the dehydration melting of the biotite and the subsequent break-down of plagioclase. Both the crystallization of basalt and the dehydration of biotite would liberate large volumes of water into the overlying crust, resulting in decreased crustal solidus temperatures, and, overtime, the generation of the prodigious volumes of crustal magma seen during the magmatic flare-up. We propose that the group 3 andesites are mixtures of ascending mafic magmas and the mid to upper crustal melts. The model presented in Figure 4C represents a mixture of 60% high-Al basalt and 40% partial melt of the regional upper crustal basement. For details of the modeling parameters, see Appendix 1.
Lower crustal andesites: Quaternary CVZ arc and minor centers (groups 1, 2, 4, 5, and 6)
Andesites associated with the Quaternary CVZ arc, including both major composite cones (groups 1 and 2) and minor eruptive centers (group 5), do not share the same upper crustal origin recorded in the group 3 andesites. Instead, these andesites record predominantly lower crustal origins with compositional differences that can be correlated with distinct crustal domains (e.g., Wörner et al., 1992; Wörner et al., 1994; Aitcheson et al., 1995; Mamani et al., 2008; Mamani et al., 2010). As illustrated in Figures 3, 4, andesites erupted between 16 and 19°S have distinctly higher Sr/Y, higher Sm/Yb, and steeper REE patterns than andesites erupted between 21 and 26°S. Davidson et al. (1988) attributed the trace element enrichments and Sr systematics in the northern arc segment (based on observations in Nevados de Payachata region of the CVZ at ∼18°S) to fractionation/assimilation in a garnet-bearing plagioclase-free environment. More recently, Blum-Oeste and Wörner (2016); Wörner et al. (2018) proposed that some of the trace element enrichment in the northern arc segment might reflect an enriched mantle wedge beneath the region (either an enriched lithospheric mantle lithosphere or the melting of foundering amphibole-bearing crustal cumulates). While this could help to explain some of the more pronounced enrichments (i.e., Sr and LREEs), the HREEs and elevated Sr isotope ratios are still best reconciled with a lower crustal origin. For this study, in keeping with most other previous works (Davidson et al., 1988; Wörner et al., 1992; Wörner et al., 1994; Aitcheson et al., 1995; Mamani et al., 2008; Mamani et al., 2010), we assume that the major compositional differences between the two arc segments correspond to differences in the composition of the lower crust. We find it probable that there are source heterogeneities along-strike within the CVZ arc, but a quantitative assessment of this is beyond the scope of this work.
Mamani et al. (2008; Mamani et al., 2010), following the work of Wörner et al. (1992, Wörner et al. 1994) and Aitcheson et al. (1995), proposed that various geochemical tracers, including Pb isotopes, delineate multiple distinct crustal domains beneath the CVZ with a transitional terrain boundary at ∼20°S (Arequipa domain above 20°S and Antofalla domain below 20°S. This has been supported by multiple geophysical studies (Schmitz et al., 1997; Yuan et al., 2002), and one of the key features appears to be a significant change in the composition of the lowermost crust between 21 and 22°S. To the north of 20°S, the crust appears to have a significantly larger portion of mafic lower crust. Mamani et al. (2008) proposed that the larger proportion of mafic crust north of 20°S was the cause of the high Sr/Y ratios recorded in young volcanoes in the region. These interpretations are supported by petrophysical models which indicate that garnet stability is inversely correlated with SiO2 contents (Tassara, 2006). In this case, a more mafic lower crust north of 20°S would contain more garnet and impart a stronger garnet signature during lower crustal fractionation processes (Tassara, 2006). South of 20°S, there is additional evidence indicating the lower crust is significantly more felsic. A rare slice of Paleozoic lower crust exposed at ∼22°S in northern Chile (Sierra de Limon Verde) contains a high-grade metamorphic assemblage that includes amphibolite, eclogite, and granulite facies rocks that are largely felsic in composition (∼62–68 wt.% SiO2; Lucassen et al., 2001).
Trace element variations presented in Figure 3 are consistent with the lower crustal differences discussed above. Andesites from 16 to 19°S are consistent with lower crustal fractionation and the mixing between mantle-derived basaltic magmas melt and melts-derived from eclogitic lower crust. In addition, to be devoid of plagioclase, the REE patterns are best reproduced by a lower crustal phase assemblage dominated by garnet and clinopyroxene. In contrast, andesites from 21 to 26°S appear to be derived from a more mineralogically diverse source region. Although depleted HREE concentrations indicate that garnet played a role in the formation of these andesites, depleted MREE concentrations record a strong amphibole signal. There is also evidence for minor amounts of plagioclase. However, this could represent a later stage of crystallization overprinting the deeper signals.
Minor centers from the CVZ arc (group 5) generally share similar compositional characteristics with regional major arc centers. Minor arc centers from Peru define trends similar to those observed in the major arc systems between 16 and 19 °S. The Peruvian minor centers seem to be slightly enriched in incompatible trace elements relative to their major center counterparts (i.e., LREE, Sr, K2O). However, this enrichment could simply be recording a slight decrease in the degree of partial melting in the source region.
Back-arc andesites (group 6)
Some of the best support for major changes in crustal composition controlling the early evolution of CVZ magmas come from minor centers from the Bolivian back-arc (e.g., Davidson and de Silva, 1995). Samples from these centers have REE concentrations and trace element ratios (i.e., Sr/Y and Sm/Yb) that are transitional between the two arc segments. This is significant, as these centers are located in the proposed transition zone between the Arequipa and Antofalla domains (located between 19 and 21°S) and appear to display distinct geochemical signatures characteristic of both domains. This is consistent with the work of Wörner et al. (1994), who conducted a geochemical traverse along the CVZ in this region (17.5–22°S) and showed that compositions erupted on the surface record the boundary. Wörner et al. (1994) also proposed that the boundary is not sharp, but a southward dipping wedge characterized by the southern Antofalla terrain overlying the older Arequipa terrain. In some cases, they observed that volcanic centers could record characteristic of both terrains.
Lavas from the S. Puna have slightly different compositional characteristics than other andesites in the CVZ, including LREE enrichments and high concentrations of incompatible HFSE (Kay et al., 1994; Risse et al., 2013). Some of these differences have been attributed to delamination-driven melting of asthenospheric mantle beneath the Southern Puna (Kay et al., 1994). On a broader scale, the differences between the Quaternary frontal arc lavas and the back-arc may be related to lower degrees of partial melting in the back arc in response to less fluid fluxing from the subduction slab (Burns et al., 2020).
Andesite production and evolution in the CVZ through space and time: The role of mantle flux
Since the Pliocene, two distinct types of andesites have erupted in the CVZ. Between 6 and 1 Ma, during the peak of the APVC flare-up, crustal melting in response to the influx of large volumes of mantle-derived basalt (likely high-Al basalt; Burns et al., 2020) into the middle and upper crust resulted in the production of voluminous hybrid upper crustal andesites (group 3; Figure 5). In contrast, Quaternary andesites associated with the modern CVZ arc (groups 1 and 2) and andesites from the waning stage of the flare-up (group 4) record a distinct lower crustal evolution. Davidson et al. (1991) proposed that the composition(s) of the most primitive lavas erupted in the CVZ represent a compositional “baseline” imparted on mantle-derived magmas in a deep, lower crustal MASH zone (Hildreth and Moorbath, 1988). In this scenario, ascending mantle-derived magmas stall (near the base of the crust), partially melt the lower crust, fractionate, and create hybrid magmas with compositions that reflect a combination of these processes. Recent geophysical studies in other arc settings (e.g., Taupo Volcanic Zone; Harrison and White, 2004), along with numerical simulations (Dufek and Bergantz, 2005; Annen et al., 2006), have shown that this zone likely extends through most of the lower crust in typical continental arc settings.
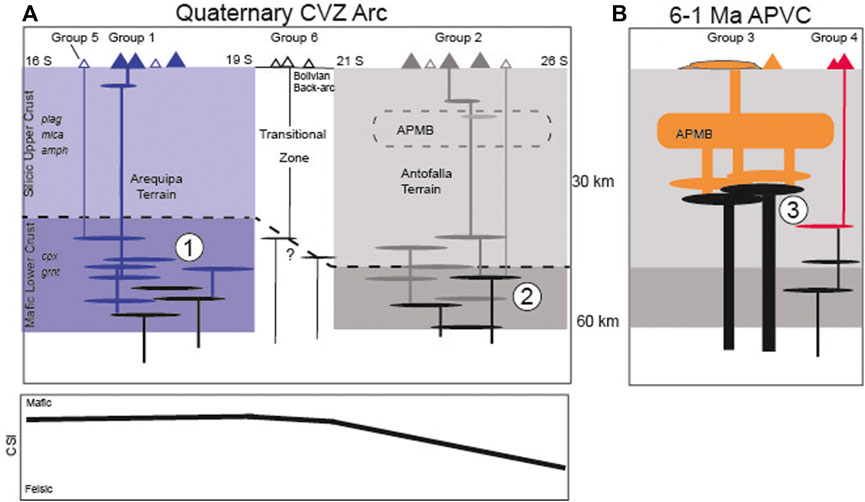
FIGURE 5. Schematic diagrams illustrating the different mechanisms for andesite formation in the CVZ. (A) includes Quaternary andesites from the frontal arc. Diagrams highlight difference in the composition of the crust. Crust between 16 and 19°S contains a significantly higher proportion of mafic crust than the crust between 21 and 26°S. CSI (Crustal Structure Index) is a measure of the proportion of mafic to felsic crust in the basement beneath the CVZ (based on gravity modeling) and is discussed in detail by Mamani et al. (2008). The APMB is the geophysically imaged Altiplano-Puna Magma Body proposed to reside in the upper crust between 21 and 24°S (e.g.; Chmielowski et al., 1999; Ward et al., 2017). (B) includes andesites associated with the peak and waning stages of the regional ignimbrite flare-up. The numbers included in both panels shows location(s) of proposed andesite generation in the crust. See the text for details.
A fundamental question that arises when comparing andesites from the Quaternary arc centers with those from the peak APVC flare-up is whether the “upper crustal” APVC andesites can be derived from the “lower crustal” CVZ andesites. There are multiple lines of evidence that indicate that this cannot be the case. First, there are significant compositional differences between the two groups of andesites at a given SiO2 content (i.e., total alkali and CaO concentrations; Figure 2). In addition, peak APVC flare-up andesites have considerably higher Sr isotope ratios, precluding a scenario wherein the peak APVC flare-up andesites could have differentiated from a basaltic andesite-andesite precursor similar to those erupted from the Quaternary CVZ arc. These differences could indicate distinct processes, different source magmas, or similar processes occurring at different levels of the crust. If these interpretations are correct, and the upper crustal flare-up andesites are not related to the deeper Quaternary arc andesites, two questions arise: 1) What are the mafic magmas fueling the flare-up, and 2) how were they able to escape the lower crust MASH zone without fractionating and evolving to more silicic (basaltic andesite to andesite) compositions? We propose that the answer to this lies in the elevated mantle heat and material flux associated with the regional magmatic flare-up.
Following the work of Shaw (1980), Hildreth (1981) proposed that the injection of basalt into the crust can significantly impact crustal stress regimes resulting in extensional fracturing of the overlying crust, promoting the upward propagation of magma. Once fracturing is initiated, subsequent injections of basalt, and the resulting increase in pore pressure, accelerate crustal failure and result in a focusing of basaltic magma upward with time. With sufficient time and an elevated basalt flux, progressive weakening of the crust can result in the delivery of basalts to higher crustal levels. The crustal depth at which the ascending magmas stall is likely controlled by the density of the crust and perhaps a density-controlled mid-crustal decollement. In the case of Andean crust, 20 and 30 km appears to be a critical depth (Lucassen et al., 2001). Once these basaltic magmas stall, partial melting of the crust and subsequent mixing and fractionation result in the formation of the hybrid intermediate magmas common in large continental magmatic systems (Hildreth, 1981). In the case of the flare-up andesites, asthenospheric upwelling in response to crustal-scale delamination (e.g., Kay and Coira, 2009) and the production of large volumes of basalt are thought to have caused major perturbations of the crustal geothermal gradient (de Silva, 1989; de Silva and Gosnold, 2007). In addition to the changes in the regional stress regimes resulting from the upwelling basalt, the removal of the high density lithospheric “root” would have removed a major mechanical barrier from the lower crust, significantly weaking the crust. These changes would have allowed large volumes of mantle-derived basalt to ascend into the high levels within the crust before stalling, fractionating, and providing the thermal energy necessary to induce crustal melting.
The production of large volumes of andesite in the mid and upper crust beneath the APVC was first proposed by de Silva (1989), who proposed that high basalt flux into the crust resulted in the development of a large mid to upper crustal MASH zone. Once established, this large upper crustal processing center acted as a thermomechanical barrier trapping ascending magmas and creating a positive feedback loop between magma input, crustal melting, and the thermomechanical state of the crust (de Silva and Gosnold, 2007; de Silva and Gregg, 2014). Importantly, these changes were proposed to result in the production of copious amounts of andesite in the upper crust. Recent geophysical studies (Ward et al., 2014; Ward et al., 2017) focused on the crust beneath the APVC have revealed a large low velocity zone extending from ∼25 to 4 km depth that has been interpreted as a large, melt-rich crystal mush, which previous authors coined the Altiplano-Puna Magma Body (APMB; Chmielowski et al., 1999; Zandt et al., 2003). The extent of this low velocity zone is of particular importance here, as the deeper depths correspond well with our estimated andesite generation depths beneath the APVC. We propose that basalts are delivered to a depth of ∼35–25 km where they stall and interact with the continental crust in the lower reaches of the upper crustal MASH zone. During this time intermediate andesite magmas are formed and propagate through the crust, where they accumulate between ∼17 and 20 km depth (see Burns et al., 2015). Our geochemical models here support these previous conceptual models that the deeper reservoir (17–20 km) is andesitic and serves as the magmatic “mothership” feeding the large, predominantly dacitic, upper crustal magma reservoirs feeding the APVC ignimbrite-forming eruptions (de Silva et al., 2006). This scenario is quite distinct from the prevailing model of lower crustal andesite genesis that explains the steady state andesites.
Andesite magmatism and the growth of continental growth
Continental magmatic arcs are one of the primary locations where new continental crust is created on Earth (e.g., Eichelberger, 1978; Thorpe et al., 1981), with the intermediate composition(s) characteristic of subduction zone magmas closely resembling the bulk composition of the Earth’s crust (Taylor and McLennan, 1985). During the past 6 My, andesites from the CVZ record two distinct types of crustal growth. Steady-state andesites, herein represented by the Quaternary arc systems and minor eruptive centers associated with the modern frontal arc, record the fractionation and evolution of mafic, mantle-derived magmas (likely high-Al basalt) in the lower crust. Importantly, this lower crustal fractionation would result in the crystallization and subsequent accumulation of high-density mafic phase assemblages dominated by olivine, clinopyroxene, and possibly garnet at higher pressures (see Burns et al., 2020 for additional discussion of deep crystallizing assemblage), favoring the production of a dense, mafic lower crust. During this deep fractionation, intermediate melts would be produced, extracted, and delivered to the upper crust. However, the physical result of this entire process would be the densification and eventual loss of the lower crust. In contrast, andesites from the APVC flare-up record the delivery of basalts to higher levels in the crust and subsequent generation of upper crustal hybrid magmas. These magmas likely added significant volume to the mid to upper crust but did not result in the same densification of the crust.
It has been well established that continental magmatic arcs experience distinct magmatic episodes throughout their histories that appear to occur in response to major changes in crustal structure/composition (e.g., Kay et al., 1999; Ducea et al., 2005; DeCelles et al., 2009; Kay and Coira, 2009; Chapman et al., 2021). These cyclic episodes are characterized by punctuated short-term periods of intense magmatism (5–20 Myr) wherein mantle power input can increase by more than an order-of-magnitude relative to steady-state arc magmatism (de Silva et al., 2006; Burns et al., 2015). Importantly, it has been proposed that an overwhelming amount of the continental crust formed in continental subduction zones is created during these periods (some estimates are >50% of the total plutonic volume during a single period; Coleman and Glazner, 1997). For the Cretaceous Sierra Nevada arc in California, multiple authors (Ducea et al., 2005; DeCelles et al., 2009; Chapman et al., 2021) have proposed that these high intensity events (flare-ups) occur following periods of crustal shortening where convergence-driven thrusting behind the arc thicken the continental crust. The thickening results in the delivery of melt-fertile lower crust and mantle lithosphere into the melting region beneath the arc, providing fuel for a flare-up. Melting of the lower crustal/mantle lithosphere results in the formation of large volumes of dense residual granulite and eclogite (garnet pyroxenite) facies residuum and the formation of a dense arc “root.” Consequently, the high density of this root results in a density inversion with the underlying asthenosphere and can result in foundering and delamination of the root (Kay and Kay, 1993). In the model for the Sierra Nevada arc, this has been proposed to cause the termination of the flare-up (e.g., Ducea et al., 2005). A critical feature of the model proposed for the Sierra Nevada is that it coincides with the crustal thickening events. This is not applicable to the Neogene Central Andean ignimbrite flare-up that includes the APVC, as there is clear evidence that the crustal thickening and delamination occurred prior to the flare-up (Kay et al., 1999).
Our findings indicate that steady-state magmatism in the Central Andes (on the already thickened crust) results in the deep crystallization of mantle-derived basalts and the dense residuum in the lower crust. During the mid-Miocene, gravity instabilities resulting from the dense root, in concert with changing subducting plate geometry, resulted in the delamination of the deep root and the initiation of the flare-up. An important observation is that although continental crust is being produced during both stages of magmatism in the CVZ (flare-up and steady state) the increase in new crust added during the flare-up may be balanced by loss of lower crust through delamination. In a recent compilation, de Silva and Kay (2018) calculated that in the case of the Central Andes from 21 to 24°S, delamination coupled with forearc subduction erosion has resulted in ∼5.5% loss of continental crust during the flare-up. It is worth noting that to maintain an andesitic continental crust (∼61% SiO2; Taylor and McLennan, 1985), the foundering of mafic to ultramafic materials from the base of the crust is likely a critical process. Within the framework of continental arc cyclicity, it appears that andesitic continental crust is being produced on a nearly continuous basis. However, the long-term survival of the crust created may be heavily dependent on the processes and thermal conditions in which the magmas are generated.
Concluding summary
During the past 6 My, andesites in the Central Andes record two distinct modes of andesite magmatism that correspond with large-scale geodynamic changes in the region. From ca. 6–1 Ma, andesites associated with the peak of the APVC ignimbrite flare-up have geochemical and isotopic signatures consistent with the emplacement of mantle-derived basalts into the upper crust and subsequent melting and mixing (Assimilation-Fractional Crystallization) with upper crustal lithologies. The emplacement of large volumes of basalt into the upper crust result from delamination-driven asthenospheric mantle upwelling following major changes in the geometry of the subducting Nazca plate.
In contrast, major composite cones and minor centers associated with the Quaternary CVZ arc have distinctive trace element and isotopic characteristics showing a clear lower crustal origin. Within the Quaternary centers, there are also distinct latitudinal variations that reflect changes in the age and composition of the lower crust. The transition from upper crustal to lower crustal magmatism during the past 6 My reflects the waning of regional flare-up and a return to steady state arc magmatism. Importantly, this transition from prodigious melt production in the mid to upper crust to lower crustal magma production has significant implications for the density structure and evolution of the crust. During the flare-up, considerable mass is added to the mid to upper crust potentially, resulting in the vertical expansion of the crust. In contrast, during steady state magmatism, lower crustal MASH processes result in the crystallization of large volumes of deep, mafic phases in the lower most crust, creating a dense crystalline residuum and effectively “densifying” the lower crust. This densification may ultimately aid in the delamination of the dense lower crustal root and the triggering of future flare-ups. These differences in andesite production may help to explain the cyclicity observed in mature continental arcs.
Data availability statement
The original contributions presented in the study are included in the article/Supplementary Material, further inquiries can be directed to the corresponding author.
Author contributions
DB and SdS compiled the data for this project. DB organized, plotted, and interpreted the data and wrote the initial draft of the manuscript. SdS provided comments and unpublished data for this work. SdS also helped with the conceptualization of this project.
Funding
The manuscript submission fees will be covered by the Stanford College of Earth, Energy, and Environmental Sciences.
Acknowledgments
Our ideas about andesites and CVZ magmatism have been influenced heavily by many colleagues, too numerous to fully acknowledge, but we wish to call out Peter Francis, Jon Davidson, Anita Grunder, Gerherd Wörner, Sue Kay, and Axel Schmitt, among them. Any shortcomings in our analysis and logic are our own failing. The journal reviews by Ian Smith and Bob Trumbull are gratefully acknowledged for keeping us honest and improving the final product. Editorial handling of Gary Michelfelder and Valerio Acocella is also appreciated. We would also like to thank Cerise Burns for proofreading multiple versions of this manuscript.
Conflict of interest
The authors declare that the research was conducted in the absence of any commercial or financial relationships that could be construed as a potential conflict of interest.
Publisher’s note
All claims expressed in this article are solely those of the authors and do not necessarily represent those of their affiliated organizations, or those of the publisher, the editors and the reviewers. Any product that may be evaluated in this article, or claim that may be made by its manufacturer, is not guaranteed or endorsed by the publisher.
Supplementary material
The Supplementary Material for this article can be found online at: https://www.frontiersin.org/articles/10.3389/feart.2022.961130/full#supplementary-material
References
Aitcheson, S. J., Harmon, R. S., Moorbath, S., Schneider, A., Soler, P., Soria-Escalante, E., et al. (1995). Pb isotopes define basement domains of the Altiplano central Andes. Geology 23, 555–558. doi:10.1130/0091-7613(1995)023<0555:pidbdo>2.3.co;2
Allmendinger, R. W., Jordan, T. E., Kay, S. M., and Isacks, B. L. (1997). The evolution of the Altiplano-Puna plateau of the central Andes. Annu. Rev. Earth Planet Sci. 25, 139–174. doi:10.1146/annurev.earth.25.1.139
Annen, C., Blundy, J. D., and Sparks, S. J. (2006). The Genesis of intermediate and silicic magmas in deep crustal hot zones. J. Petrol. 47, 505–539. doi:10.1093/petrology/egi084
Barreiro, B. A., and Clark, A. H. (1984). Lead isotopic evidence for evolutionary changes in magma-crust interaction, Central Andes, southern Peru. Earth Planet. Sci. Lett. 69, 30–42.
Blum-Oeste, M., and Wörner, G. (2016). Central Andean magmatism can be constrained by three ubiquitous end-members. Terra nova. 28, 434–440. doi:10.1111/ter.12237
Brandmeier, M., and Wörner, G. (2016). Compositional variations of ignimbrite magmas in the central Andes over the past 26 Ma — a multivariate statistical perspective. Lithos 262, 713–728. doi:10.1016/j.lithos.2016.07.011
Burns, D. H., de Silva, S. L., Tepley, F. J., and Schmitt, A. K. (2020). Chasing the mantle: Deciphering cryptic mantle signals through Earth’s thickest continental magmatic arc. Earth Planet Sci. Lett. 531, 115985. doi:10.1016/j.epsl.2019.115985
Burns, D. H., de Silva, S. L., Tepley, F. T., Schmitt, A. K., and Loewen, M. L. (2015). Recording the transition from flare-up to steady-state arc magmatism at the Purico-Chascon volcanic complex, northern Chile. Earth Planet Sci. Lett. 422, 75–86. doi:10.1016/j.epsl.2015.04.002
Chapman, J. B., Ducea, M. N., DeCelles, P. G., and Profeta, L. (2015). Tracking changes in crustal thickness during orogenic evolution with Sr/Y: An example from the North American Cordillera. Geology 43, 919–922.
Chapman, J. B., Shields, J. E., Ducea, M. N., Paterson, S. R., Attia, S., and Ardill, K. E. (2021). The causes of continental arc flare ups and drivers of episodic magmatic activity in Cordilleran orogenic systems. Lithos 398-399, 106307–106399. doi:10.1016/j.lithos.2021.106307
Chmielowski, J., Zandt, G., and Haberland, C. (1999). The central andean altiplano-puna magma body. Geophys Res. Lett. 26, 783–786. doi:10.1029/1999gl900078
Coira, B., Davidson, J., Mpodozis, C., and Ramos, V. (1982). Tectonic and magmatic evolution of the Andes of northern Argentina and Chile. Earth-sci Rev. 18, 303–332. doi:10.1016/0012-8252(82)90042-3
Coleman, D. S., and Glazner, A. F. (1997). The Sierra crest magmatic event: Rapid formation of juvenile crust during the late cretaceous in California. Int. Geol. Rev. 39, 768–787. doi:10.1080/00206819709465302
Davidson, J. P., and de Silva, S. L. (1995). Late cenozoic magmatism of the Bolivian Altiplano. Contrib. Mineral. Petrol 119, 387–408. doi:10.1007/bf00286937
Davidson, J. P., Harmon, R. S., and Wörner, G. (1991). “The source of central Andean magmas; Some considerations,” in Andean magmatism and its tectonic setting. Editors R. S. Harmon, and C. W. Rapela (Boulder, Colorado: Geological Society of America Special). Paper 265.
Davidson, J. P., McMillan, N. J., Moorbath, S., Wörner, G., Harmon, R. S., and Lopez-Escobar, L. (1988). The Nevados de Payachata volcanic region (18 °S/69 °W, N. Chile) II. Evidence for widespread crustal involvement in Andean magmatism. Contrib. Mineral. Petrol 105, 412–432. doi:10.1007/bf00286829
de Silva, S. L. (1989). Altiplano-Puna volcanic complex of the central Andes. Geology 17, 1102–1106. doi:10.1130/0091-7613(1989)017<1102:apvcot>2.3.co;2
de Silva, S. L., and Gosnold, W. D. (2007). Episodic construction of batholiths: Insights from the spatiotemporal development of an ignimbrite flare-up. J. Volcanol. Geoth Res. 167, 320–335. doi:10.1016/j.jvolgeores.2007.07.015
de Silva, S. L., and Gregg, P. M. (2014). Thermomechanical feedbacks in magmatic systems: Implications for growth, longevity, and evolution of large caldera-forming magma reservoirs and their supereruptions. J. Volcanol. Geoth Res. 282, 77–91. doi:10.1016/j.jvolgeores.2014.06.001
de Silva, S. L., and Kay, S. M. (2018). Turning up the heat: High-flux magmatism in the central Andes. Elements 14, 245–250. doi:10.2138/gselements.14.4.245
de Silva, S. L., Self, S., Francis, P. W., Drake, R. E., and Carlos Ramirez, R. (1994). Effusive silicic volcanism in the central Andes: The chao dacite and other young lavas of the altiplano-puna volcanic complex. J. Geophys. Res. 99 (B9), 17805–17825. doi:10.1029/94jb00652
de Silva, S. L., Zandt, G., Trumbull, R., Viramonte, J. G., Salas, G., and Jiméz, N. (2006). “Large ignimbrite eruptions and volcano-tectonic depressions in the central Andes: A thermomechanical perspective,”. Editors C. Troise, G. De Natale, and C. R. J. Kilburn (London: Geological Society), 269, 47–63.Mech. Activity Unrest A. T. Large Calderas.
DeCelles, P. G., Ducea, M. N., Kapp, P., and Zandt, G. (2009). Cyclicity in Cordilleran orogenic systems. Nat. Geosci. 2, 251–257. doi:10.1038/ngeo469
Ducea, M. N., Paterson, S. R., and DeCelles, P. G. (2005). High-volume magmatic events in subduction systems. Elements 11, 99–104. doi:10.2113/gselements.11.2.99
Dufek, J., and Bergantz, G. W. (2005). Lower crustal magma genesis and preservation: A stochastic framework for the evaluation of basalt–crust interaction. J. Petrol 46, 2167–2195. doi:10.1093/petrology/egi049
Eichelberger, J. C. (1978). Andesitic volcanism and crustal evolution. Nature 275, 21–27. doi:10.1038/275021a0
Freymuth, H., Brandmeier, M., and Wörner, G. (2015). The origin and crust/mantle mass balance of Central Andean ignimbrite magmatism constrained by oxygen and strontium isotopes and erupted volumes. Contrib. Mineral. Petr 169, 58. doi:10.1007/s00410-015-1152-5
Gardien, V., Thompson, A. B., and Ulmer, P. (2000). Melting of biotite + plagioclase + quartz gneisses: The role of H2O in the stability of amphibole. J. Petrol 41, 651–666. doi:10.1093/petrology/41.5.651
Gill, J. B. (1981). Orogenic andesites and plate tectonics- Minerals and Rocks No. 16. Berlin Heidelberg: Springer.
Godoy, B., Taussi, M., Gonzalez-Maurel, O., Renzulli, A., Hernandez-Prat, L., le Roux, P., et al. (2019). Linking the mafic volcanism with the magmatic stages during the last 1 Ma in the main volcanic arc of the Altiplano-Puna Volcanic Complex (Central Andes). J. S Am. Earth Sci. 95, 102295. doi:10.1016/j.jsames.2019.102295
Grocke, S. B., de Silva, S. L., Iriate, R., Lindsay, J. M., and Cottrell, E. (2017). Catastrophic Caldera-Forming (CCF) Monotonous Silicic Magma Reservoirs: Geochemical and Petrological Constraints on Heterogeneity, Magma Dynamics, and Eruption Dynamics of the 349 Ma Tara Supereruption, Guacha II Caldera, SW Bolivia. J. Petrol. 58, 227–260.
Grunder, A. L., Klemetti, E. W., Feeley, T. C., and McKee, C. M. (2008 ). Eleven million years of arc volcanism at the aucanquilcha volcanic cluster, northern Chilean Andes: Implications for the life span and emplacement of plutons. Trans. R. Soc. Edinb Earth Sci. 97, 415–436. doi:10.1017/s0263593300001541
Harrison, A. J., and White, R. S. (2004). Crustal structure of the Taupo volcanic zone, New Zealand: Stretching and igneous intrusion. Geophys Res. Lett. 31. doi:10.1029/2004GL019885
Hawkesworth, C. J., Hammill, M., Gledhill, A. R., van Calsteren, P., and Rogers, G. (1982). Isotope and trace element evidence for late-stage intra-crustal melting in the High Andes. Earth Planet S. C. Lett. 58, 240–254. doi:10.1016/0012-821x(82)90197-2
Hildreth, W. (1981). Gradients in silicic magma chambers: Implications for lithospheric magmatism. J. Geophys Res. Solid Earth 86, 10153–10192. doi:10.1029/jb086ib11p10153
Hildreth, W., and Moorbath, S. (1988). Crustal contributions to arc magmatism in the Andes of Central Chile. Contrib. Mineral. Petrol 98, 455–489. doi:10.1007/bf00372365
Johnson, D., Hooper, P., and Conrey, R. (1999). XRF analysis of rocks and minerals for major and trace elements on a single low dilution Li-tetraborate fused bead. Adv. X-ray Analysis 41, 843–867.
Kay, R. W., and Kay, S. M. (1993). Delamination and delamination magmatism. Tectonophysics 219, 177–189. doi:10.1016/0040-1951(93)90295-u
Kay, S. M., Coira, B. L., Caffe, P. J., and Chen, C.-H. (2010). Regional chemical diversity, crustal and mantle sources and evolution of central Andean Puna plateau ignimbrites. J. Volcanol. Geoth Res. 198, 81–111. doi:10.1016/j.jvolgeores.2010.08.013
Kay, S. M., and Coira, B. L. (2009). “Shallowing and steepening subduction zones, continental lithospheric loss, magmatism, and crustal flow under the Central Andean Altiplano-Puna Plateau,” in Backbone of the americas: Shallow subduction, plateau uplift, and ridge and terrane collision. Editors S. M. Kay, V. A. Ramos, and W. R. Dickinson (Boulder, CO: Geological Society of America), 229–259.
Kay, S. M., Coira, B., and Viramonte, J. (1994). Young mafic back arc volcanic rocks as indicators of continental lithospheric delamination beneath the Argentine Puna Plateau, central Andes. J. Geophys Res. Solid Earth 99, 24323–24339. doi:10.1029/94jb00896
Kay, S. M., Mpodozis, C., and Coira, B. (1999). “Neogene magmatism, tectonism, and mineral deposits of the Central Andes (22°-33°S latitude),”. Editor B. J. Skinner (Littleton, CO, USA: Society of Economic Geology Special Publication), 7, 27–59. Geol. Ore Deposits Central Andes.
Kay, S. M., and Mpodozis, C. (2001). Central Andean ore deposits linked to evolving shallow subduction systems and thickening crust. GSA Today 11, 4–9.
Kay, S. M., and Mpodozis, C. (2002). Magmatism as a probe to the Neogene shallowing of the Nazca plate beneath the modern Chilean flat-slab. J. S Am. Earth Sci. 15, 39–57. doi:10.1016/s0895-9811(02)00005-6
Kay, R. W., and Kay, S. M (1991). Creation and destruction of lower continental crust. Geologische Rundschau 80, 259–278.
Le Bas, M. J., and Streckeisen, A. L. (1991). The IUGS systematics of igneous rocks. J. Geol. Soc. Lond. 148, 825–833. doi:10.1144/gsjgs.148.5.0825
Le Maitre, R. W. (1989). A classification of igneous rocks and glossary terms. 2nd Edition. Oxford: Blackwell Scientific Publications.
Lindsay, J. M., Schmitt, A. K., Trumbull, R. B., de Silva, S. L., Siebel, W., and Emmermann, R. (2001). Magmatic evolution of the La pacana caldera system, central Andes, Chile: Compositional variation of two cogenetic, large-volume felsic ignimbrites. J. Petrol 42, 459–486. doi:10.1093/petrology/42.3.459
Lucassen, F., Becchio, R., Harmon, R., Kasemann, S., Franz, G., Trumbull, R., et al. (2001). Composition and density model of the continental crust at an active continental margin—The central Andes between 21° and 27°S. Tectonophysics 341, 195–223. doi:10.1016/s0040-1951(01)00188-3
Mamani, M., Tassara, A., and Wörner, G. (2008). Composition and structural control of crustal domains in the central Andes. Geochem. Geophys. Geosyst 9. doi:10.1029/2007GC001925
Mamani, M., Wörner, G., and Sempere, T. (2010). Geochemical variations in igneous rocks of the Central Andean orocline (13°S to 18°S): Tracing crustal thickening and magma generation through time and space. GSA Bull. 122, 162–182. doi:10.1130/b26538.1
Maro, G., Caffe, P. J., Romer, R. L., and Trumbull, R. B. (2017). Neogene mafic magmatism in the northern Puna Plateau, Argentina: Generation and evolution of a back-arc volcanic suite. J. Petrol 58, 1591–1617. doi:10.1093/petrology/egx066
Ramos, F. C. (1992). Isotope geology of the metamorphic core of the central grouse creek mountains, Box Elder County. University of California Los Angeles.
Reubi, O., and Müntener, O. (2022). Making andesites and the continental crust: Mind the step when wet. J. Petrol. 63 (6). doi:10.1093/petrology/egac044
Risse, A., Trumbull, R. B., Kay, S. M., Coira, B., and Romer, R. L. (2013). Multi-stage evolution of late Neogene mantle-derived magmas from the central Andes back-arc in the southern Puna Plateau of Argentina. J. Petrol 54, 1963–1995. doi:10.1093/petrology/egt038
Rogers, G., and Hawkesworth, C. J. (1989). A geochemical traverse across the north Chilean Andes: Evidence for crust generation from the mantle wedge. Earth Planet Sci. Lett. 91, 271–285. doi:10.1016/0012-821x(89)90003-4
Salisbury, M. J., Jicha, B. R., de Silva, S. L., Singer, B. S., Jiménez, N. C., and Ort, M. H. (2011). 40Ar/39Ar chronostratigraphy of Altiplano-Puna volcanic complex ignimbrites reveals the development of a major magmatic province. Geol. Soc. Am. Bull. 123, 821–840. doi:10.1130/b30280.1
Schmitt, A., Silva, S. D., Trumbull, R., and Emmermann, R. (2001). Magma evolution in the Purico ignimbrite complex, northern Chile: Evidence for zoning of a dacitic magma by injection of rhyolitic melts following mafic recharge. Contrib. Mineral. Petrol 140, 680–700. doi:10.1007/s004100000214
Schmitz, M., Heinsohn, W.-D., and Schilling, F. R. (1997). Seismic, gravity and petrological evidence for partial melt beneath the thickened Central Andean crust (21° – 23°S). Tectonophysics 270, 313–326. doi:10.1016/s0040-1951(96)00217-x
Shaw, H. R. (1980). “The fracture mechanisms of magma transport from the mantle to the surface,” in Physics of magmatic processes. Editor R. B. Hargraves (Princeton, N.J: Princeton University Press), 201–264.
Soler, M. M., Caffe, P. J., Coira, B. L., Onoe, A. T., and Kay, S. M. (2007). Geology of the vilama caldera: A new interpretation of a large-scale explosive event in the central andean plateau during the upper Miocene. J. Volcanol. Geoth Res. 164, 27–53. doi:10.1016/j.jvolgeores.2007.04.002
Stern, C. R. (2004). Active Andean volcanism: Its geologic and tectonic setting. Rev. Geol. Chile 31, 161–206. doi:10.4067/s0716-02082004000200001
Straub, S. M., Gómez, -Tuena, A., Stuart, F. M., Zellmer, G. F., Espinasa-Perena, R., Cai, Y., et al. (2011). Formation of hybrid arc andesites beneath thick continental crust. Earth Planet Sci. Lett. 303, 337–347. doi:10.1016/j.epsl.2011.01.013
Sun, S., and McDonough, W. F. (1989). Chemical and isotopic systematics of oceanic basalts: Implications for mantle composition and processes. Geol. Soc. Lond Spec. Publ. 42, 313–345. doi:10.1144/gsl.sp.1989.042.01.19
Tassara, A. (2006). Factors controlling the crustal density structure underneath active continental margins with implications for their evolution. Geochem. Geophys. Geosyst 7, Q01001. doi:10.1029/2005GC001040
Taylor, S. R., and McLennan, S. M. (1985). The continental crust: Its composition and evolution. Oxford, UK: Blackwell.
Thorpe, R. S. (1982). Andesites: Orogenic andesites and related rocks. Norwich, UK: John Wiley & Sons.
Thorpe, R. S., Francis, P. W., and Harmon, R. S. (1981). Andean andesites and crustal growth. Phil. Trans. R. Soc. Lond. A, 305–320.
Tierney, C. R., Schmitt, A. K., Lovera, O. M., and de Silva, S. L. (2016). Voluminous plutonism during volcanic quiescence revealed by thermochemical modeling of zircon. Geology 44, 683–686. doi:10.1130/g37968.1
van Alderwerelt, B., Ukstins, I. A., and Ramos, F. C. (2021). Sr isotopes and geochemistry of Cerro Overo maar provide a unique window into arc magma Genesis in the Central Volcanic Zone of the Andes. Lithos 386, 105978. doi:10.1016/j.lithos.2021.105978
Ward, K. M., Delph, J. R., Zandt, G., Beck, S. L., and Ducea, M. N. (2017). Magmatic evolution of a Cordilleran flare-up and its role in the creation of silicic crust. Sci. Rep-uk 7, 9047. doi:10.1038/s41598-017-09015-5
Ward, K. M., Zandt, G., Beck, S. L., Christensen, D. H., and McFarlin, H. (2014). Seismic imaging of the magmatic underpinnings beneath the Altiplano-Puna volcanic complex from the joint inversion of surface wave dispersion and receiver functions. Earth Planet S. C. Lett. 404, 43–53. doi:10.1016/j.epsl.2014.07.022
Watts, R. B., de Silva, S. L., de Rios, G. J., and Croudace, I. (1999). Effusive eruption of viscous silicic magma triggered and driven by recharge: A case study of the Cerro chascon-runtu jarita dome complex in southwest Bolivia. B Volcanol. 61, 241–264. doi:10.1007/s004450050274
Wörner, G., Mamani, M., and Blum-Oeste, M. (2018). Magmatism in the central Andes. Elements 14, 237–244. doi:10.2138/gselements.14.4.237
Wörner, G., Moorbath, S., Horn, S., Entenmann, J., Harmon, R. S., Davidson, J. P., et al. (1994). “Large and fine-scale geochemical variations along the Andean arc of northern Chile (17.5° to 22°S),” in Tectonics of the southern central Andes, structure and evolution of an active continental margin. Editors K. J. Ruetter, E. Scheuber, and P. J. Wigger (Berlin: Spinger).
Wörner, G., Moorbath, S., and Harmon, R. S. (1992). Andean Cenozoic volcanic centers reflect basement isotopic domains. Geology 20, 1103–1106. doi:10.1130/0091-7613(1992)020<1103:acvcrb>2.3.co;2
Yuan, X., Sobolev, S., and Kind, R. (2002). Moho topography in the central Andes and its geodynamic implications. Earth Planet Sci. Lett. 199, 389–402. doi:10.1016/s0012-821x(02)00589-7
Keywords: MASH processes, continental crust formation, lower crustal melting and recycling, crustal magmatism, continental arc magmatism, flare-up andesites, andesite petrogenesis, crustal andesites
Citation: Burns DH and de Silva SL (2023) Andesites and evolution of the continental crust: Perspectives from the Central Volcanic Zone of the Andes. Front. Earth Sci. 10:961130. doi: 10.3389/feart.2022.961130
Received: 04 June 2022; Accepted: 22 November 2022;
Published: 13 January 2023.
Edited by:
Gary Michelfelder, Missouri State University, United StatesReviewed by:
Robert Trumbull, GFZ German Research Centre for Geosciences, GermanyIan Ernest Masterman Smith, The University of Auckland, New Zealand
Copyright © 2023 Burns and de Silva. This is an open-access article distributed under the terms of the Creative Commons Attribution License (CC BY). The use, distribution or reproduction in other forums is permitted, provided the original author(s) and the copyright owner(s) are credited and that the original publication in this journal is cited, in accordance with accepted academic practice. No use, distribution or reproduction is permitted which does not comply with these terms.
*Correspondence: Dale H. Burns, ZGhidXJuc0BzdGFuZm9yZC5lZHU=