- 1Department of Physical Geography, Stockholm University, Stockholm, Sweden
- 2Bolin Centre for Climate Research, Stockholm, Sweden
- 3Free University of Bozen-Bolzano, Bolzano, Italy
- 4Department of Ecology, Environment and Plant Sciences, Stockholm University, Stockholm, Sweden
- 5Department of Geological Sciences, Stockholm University, Stockholm, Sweden
- 6The Nature Conservancy, Delmont, NJ, United States
The stable isotope composition of water (δ18O and δ2H) is an increasingly utilized tool to distinguish between different pools of water along the soil-plant-atmosphere continuum (SPAC) and thus provides information on how plants use water. Clear bottlenecks for the ubiquitous application of isotopic analysis across the SPAC are the relatively high-energy and specialized materials required to extract water from plant materials. Could simple and cost-effective do-it-yourself “MacGyver” methods be sufficient for extracting plant water for isotopic analysis? This study develops a suite of novel techniques for plant water extraction and compares them to a standard research-grade water extraction method. Our results show that low-tech methods using locally-sourced materials can indeed extract plant water consistently and comparably to what is done with other state-of-the-art methods. Further, our findings show that other factors play a larger role than water extraction methods in achieving the desired accuracy and precision of stable isotope composition: (1) appropriate transport, (2) fast sample processing and (3) efficient workflows. These results are methodologically promising for the rapid expansion of isotopic investigations, especially for citizen science and/or school projects or in remote areas, where improved SPAC understanding could help manage water resources to fulfill agricultural and other competing water needs.
Introduction
Stable isotope ratios of water (δ18O and δ2H), have been successfully used to study atmospheric and hydrological processes around the world for decades (Dansgaard, 1953; Craig, 1961; Sklash et al., 1976). When quantifying catchment water storage and release, water samples of rainfall, soil moisture, groundwater and stream flow are collected (Klaus and McDonnell, 2013), and subsequently analyzed for their isotopic composition and related to various catchment compartments in space and time. Technological innovations such as laser spectroscopy (Kerstel et al., 1999) have drastically reduced the cost of isotope analysis (Lis et al., 2008; Lyon et al., 2009). This development encouraged hydrologists to collect an ever-increasing number of water samples across space (Fischer et al., 2015, 2017) and time (Berman et al., 2009; von Freyberg et al., 2016). This development also stimulated the use of stable isotopes to explore how vegetation interacts with the atmosphere and the surrounding catchment (Brooks et al., 2010; McDonnell, 2014). To determine which pools of water are used by vegetation and returned to the atmosphere as transpiration, a common approach is to analyze the isotopic composition of plant water, e.g., water found in the root, xylem and/or leaf tissues (Dawson and Ehleringer, 1991; Brooks et al., 2010; Beyer et al., 2016; Goldsmith et al., 2018). Collecting rain or stream water samples for stable isotope analysis is relatively easy with a laser spectroscope where precisions of <0.1‰ for δ18O and <1‰ for δ2H are achieved. However, collecting plant water is more challenging because the desired water is part of the living plant tissues and must first be extracted.
Water extraction through squeezing or cooking plant tissue to obtain chemical components and essential oils has been conducted for thousands of years (Kockmann, 2014). More recently, water extraction approaches for stable isotope analysis based on high-tech versions of squeezing or cooking plant material were developed, such as cryogenic vacuum extraction (Dalton, 1989; West et al., 2006; Koeniger et al., 2011), distillation (Vendramini et al., 2007), cryogenic freezing and crushing (Peters and Yakir, 2008), microwave (Munksgaard et al., 2014), or monitored in situ using the direct vapor equilibration of water (Wassenaar et al., 2008; Sprenger et al., 2015; Volkmann et al., 2016). The different high-tech methods require a controlled environment to achieve desired accuracy and precision. In addition, each of the aforementioned plant water extraction method is associated with challenges concerning accuracy, precision and repeatability (Orlowski et al., 2016a, 2018; Millar et al., 2018). Extraction time during cryogenic vacuum distillation, for example, affects the apparent stable isotope composition (West et al., 2006). In addition, different “common” methods have the tendency to co-extract various chemical compounds, which can affect the accuracy of laser spectroscopes (West et al., 2010; Millar et al., 2018). As such, there is no general agreement upon optimal or best practice for plant water extraction methods. However, the choice of extraction method may affect study results and represents a subjective and potentially influential factor.
All current plant water extraction methods tend to be resource-intensive, costly, and demand specialized materials and supporting infrastructure. These requirements limit leveraging of citizen science projects which have been beneficial for other isotopic-centered hydrological efforts, such as spatial rainfall sampling during storm events (Good et al., 2014). The relatively high resource demands of plant water extraction is especially problematic when working in remote areas that lack infrastructure where plant water isotopic information could be most useful e.g., in central Tanzania (Koutsouris and Lyon, 2018) or in northern Sweden (Dahlke et al., 2014). Therefore, methodological innovations are necessary for fast, easy, reliable and cost-efficient plant water extraction.
With this perspective in mind, this study develops do-it-yourself “MacGyver” plant water extraction methods using materials found in common kitchens or laboratories around the world and techniques that can be implemented without specialized training. As a proof of concept, we used herbaceous plants species, such as grasses and melon plants, to evaluate the effectiveness of the various techniques and compared the isotopic composition of the extracted water with a “standard” extraction technique, i.e., the cryogenic vacuum distillation. In addition, we simulated the effect of plant sample transport and storage on the plant water isotopic composition. Since all plant water extraction methods have sources of error and uncertainty, which we can control through adequate methodological characterization and clearly defined protocols, our study proposes and tests the hypothesis that simple plant water extraction methods can be used to generate isotopic data with precisions that are comparable to that of more demanding methods.
Materials and Methods
Plant Material Growth and Initial Processing
Plant water extraction methods were tested on four plant material groups: (A) grass grown indoors (ryegrass; Lolium perenne); (B) melon plants grown indoor (water melon; Citrullus lanatus); (C) grass grown outdoors on a mown lawn and (D) grass grown outdoors on a grazed pasture (both the pasture and the lawn C and D are a combination of mainly Poa annua and Festuca rubra) (Supplementary Figure S1).
Indoor plant groups grew in trays on an office windowsill. Each tray (22 × 36 × 6 cm) contained 40, free-draining seedling pots (4 × 4 × 5 cm). Each pot contained one turf briquette, which was soaked for 30 min in water to reach field capacity before sowing five grass seeds or two melon seeds. To control isotopic composition, we used two 25-L closed-top barrels filled with tap water at the beginning of the experiment giving a constant and known isotope composition (δ18O = 7.97 ± 0.3‰ and δ2H = −62.02 ± 0.5‰) for the initial soaking and subsequent irrigation. One of the trays rested on a kitchen balance connected to an ArduinoTM UNO micro-controller with SD-shield (AMC) to measure changes in weight due to evaporation and transpiration at 5 min intervals. In addition, AMC-connected, low-budget soil moisture sensors (HL-69) were installed into one seedling pot to monitor volumetric soil moisture content (%). The AMC information was used to monitor water content and adjust the irrigation scheme, which consisted of irrigation every 2–3 days with 10 ml of water to maintain a moisture content of approximately 60–80% across both trays. Two growing lamps (Plantagen, 6 W, 180 lumen, 265 μmol at 200 mm) were used to supplement light since the experiment was ran in the winter in Sweden (low natural radiation and short days). The lamps were positioned 40 cm above each tray and provided 20 h of light per 24 h cycle. To maintain homogeneous growing conditions, the growing pots in each tray were randomly turned around daily. After 40 days, when grass leaves reached a length of >20 cm and 2–5 mm width and to melon plants had three leaves >15 cm long, the plants were harvested.
The plant groups grown outdoors consisted of grasses collected from a lawn and a pasture at Stockholm University’s Frescati campus. Plant material samples were collected after a rain event during 1 day in October 2018 (autumn). At the moment of sampling, the qualitative soil moisture content was assessed as class 5, i.e., where squelchy noise can be heard when stepping on the ground but no water is visible (Rinderer et al., 2012). At the time of sample collection, both grasses had an average height between 10 and 20 cm, a leaf width larger than 5 mm, and were fibrous. To have a consistent sample size for the various extraction techniques (next section) and isolate potential variability in isotopic composition in the outdoor grass, the grass collected at each site was taken from three 20 × 30 cm plots located within 1 m of each other. The lawn grass and pasture grass samples were composited separately and then cut into 2 cm pieces for water extraction.
Directly after harvest of both the indoor and outdoor plant material, three replicates were prepared for each of the extraction techniques by weighing plant samples (Precisa XT4200C, ± 0.01 g). Due to the low plant weight and to be able to extract sufficient water for stable isotope analysis, a sample consisted of a leaf and stem.
Plant Water Extraction Methods
Reference Method (REF) – Cryogenic Vacuum Extraction
The cryogenic vacuum extraction technique described by Koeniger et al. (2011) was used as the reference method (REF method) for the evaluation of the MacGyver methods. This method was chosen because it is considered relatively inexpensive, fast, and reliable when working in well-controlled environments without material procurement limitations. The REF method (Figure 1a) uses a heated vial (EXE-I, Exetainer® vial with standard cap and rubber septum, Labco Ltd, Lampeter, United Kingdom) and a cold trap vial (EXE-II, Exetainer® vial with standard cap and rubber septum, Labco Ltd, Lampeter, United Kingdom). We transferred 3 g of plant material into EXE-I immediately after harvest and stored for 1 h at −20°C to avoid decomposition and fractionation. Before extraction, EXE-I and EXE-II were connected through steel capillary tubing (bended syringe 150 × 2 mm, washed and oven dried at 200°C before use) and the entire system evacuated with a hand vacuum pump (Mityvac) to a threshold of 85 kPa. EXE-I was heated for 1 h in a 100°C water bath while EXE-II rested in a Dewar flask containing liquid nitrogen (∼−196°C). Every 15 min the Dewar flask was refilled with liquid nitrogen. After 1 h the extraction was stopped and EXE-II was sealed with Parafilm. After thawing, the extracted liquid water was pipetted into 2 ml vial for stable isotope analysis.
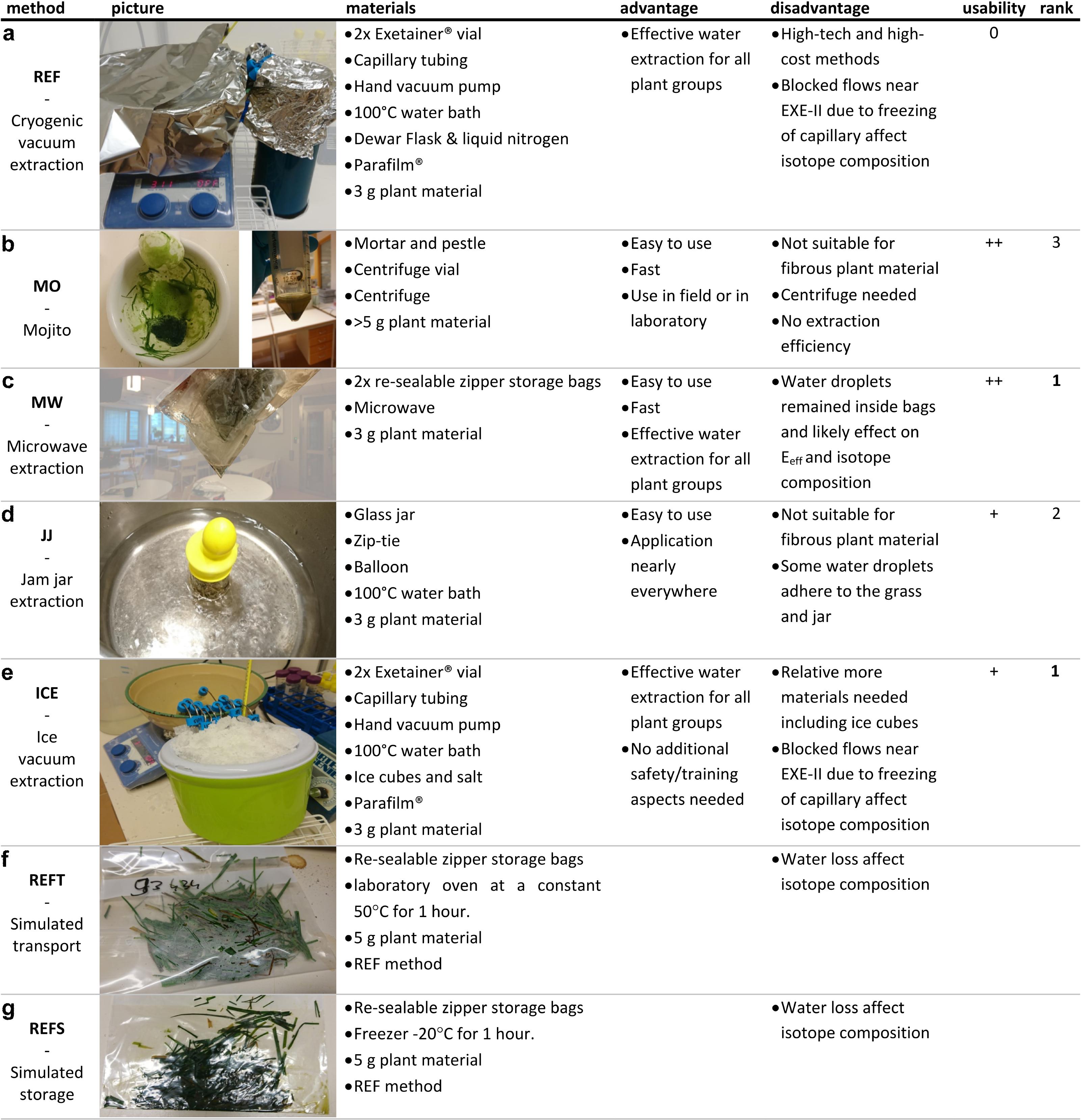
Figure 1. The different plant water extraction techniques used: (a) REF (cryogenic vacuum), (b) MO (mojito, left) and mojito after centrifuge (right), (c) MW (microwave), (d) JJ (jam jar), (e) ICE (ice cube), (f) REFT (REF with simulated transport, grass samples after 1 h in the oven to simulate transport of the material) and (g) REFS (REF with simulated storage, the grass samples thawing after 1 h in the freezer with exfiltration, i.e., loss of plant water). For each method the different materials needed, advantages, disadvantages, usability (easy, neutral, challenging indicated as ++, +, or 0) and overall rank (best to reasonable indicated as 1–3; based on Z-scores) are listed in the respective columns.
Method 1 (MO) - Pestle and Mortar Extraction (Mojito Method)
A mojito is a cocktail where mint leaves are gently mashed with a muddler to extract essential oils. With this in mind, the idea of the mojito methods was born. Interested in water instead of essential oils, we transferred 5 g of plant material to a mortar immediately after harvest and slightly crushed it with a pestle until a mushy, watery puree developed (Figure 1b). The puree was squeezed with the pestle to separate fibrous material from the green liquid. The green liquid was transferred into a centrifuge vial and laboratory centrifuged for 30 min at 5000 rpm to separate the water from the grounded plant particles. As an alternative, in remote areas a hand-made centrifuge can be used [e.g., Bhamla et al. (2017)]. After centrifuging, the liquid water was pipetted into 2 ml vial for stable isotope analysis.
Method 2 (MW) – Household Microwave and Re-sealable Zipper Storage Bags
This method used a standard kitchen microwave and re-sealable zipper storage bags (Figure 1c). We transferred 3 g of plant material into a double re-sealable zipper storage bags immediately after harvest and then microwaved at 300 W for 1 min (longer times were not used to prevent the plant material from burning). The extracted water pooled in the bottom of each plastic bag. The extracted water was transferred to a 2 ml vial for stable isotope analysis.
Method 3 (JJ) – Jam Jar Extraction
An expandable container was constructed by affixing a latex balloon secured with a zip-tie to the top of a clean 200-ml glass jar (Figure 1d). We transferred 3 g of plant material immediately after harvest into the jar before sealing the container. The jar was then placed in a 100°C water bath for 1 h (same extraction time as in REF method). During the cooking process, the balloon expands and water condensates against the inner surface. After cooking, the jar was removed from the water bath and allowed to return to room temperature. Once at room temperature, the jar was unsealed and the water in the balloon was pipetted into 2 ml vials for isotopic analysis.
Method 4 (ICE) – Ice Vacuum Extraction Using Ice Cubes and Cooking Salt
A mix of ice cubes and table salt [weight ratio 3:1 (Arbouw, 2018)] was used for cooling (−20°C) in place of the liquid nitrogen used in the aforementioned REF method (Figure 1e). Using the same setup outlined for the REF method, we transferred 3 g of plant material into EXE-I and stored frozen (−20°C) until extraction. As in REF method was conducted by placing EXE-I into a 100°C water bath and EXE-II into the ice-salt mixture. The ice-salt mixture was mixed every 15 min and the temperature was continuously monitored with a laboratory thermometer. After 1 h, EXE-II was removed and sealed with Parafilm®. After thawing, the extracted liquid water was pipetted into 2 ml vial for stable isotope analysis.
Simulated Transport (REFT) and Storage Impacts (REFS)
We explored the potential impact of transport (i.e., changes introduced after sampling and moving the samples from field to the laboratory) and storage (i.e., changes introduced by delayed analysis) on the stable isotope compositions. Since our goal was to assess the magnitude of errors introduced by transport and storage, we only consider REF method for this experiment.
To simulate the transport error, 5 g of plant material were transferred immediately after harvest into a re-sealable zipper storage bag and excess air was removed by hand. This bag was placed in a second re-sealable zipper storage bag. After being sealed, the bags were stored in a laboratory oven at a constant 50°C to simulate warm transport conditions (e.g., transport in a car without refrigeration). After 1 h, 3 g of plant material were transferred into EXE-I for plant water extraction REFT (simulated transport using the REF method to extract the plant water).
To simulate the impact of storage on the stable isotope composition, 5 g of plant material were transferred into double-bagged re-sealable zipper storage bags immediately after harvest and stored in a standard freezer at −20°C for 1 h. This test allows assessing how freezing and subsequent thawing affects isotopic composition, which would be a typical concern around storage of plant samples waiting to be processed. After removal form the freezer, 3 g of the plant material were transferred into EXE-I for plant water extraction REFS (storage impact using the REF method to extract the plant water).
The extraction efficiencies (Eeff) were assessed as the ratio of the weight of the extracted plant water over the weight of the total plant water expressed as:
where the weight of the pre-extraction plant sample is WI, weight of the post-extraction plant sample (WE) and the weight of the plant sample after oven dried at 105°C (WD, weighed repeatedly until there was no change in weight).
Isotopic Measurement and Extraction Method Comparison
Each extracted plant water sample was pipetted into a 2 mL vial (32 × 11.6 mm screw neck vials with cap and PTFE/silicone/PTFE septa). All water samples were analyzed using a Thermo Scientific isotope-ratio mass spectrometer (IRMS, Delta V Advantage Conflo IV) coupled with a Thermo Scientific Gas Bench II to determine δ18O. Water samples (0.2 ml) were placed in Exetainer® vials and the headspace flushed by a 0.3% CO2-He gas mixture of known isotopic composition. After an equilibration phase of 24 h, the headspace vapor phase was injected 8 times which allowed for a precision of 0.08‰ for δ18O. Deuterium composition was determined by direct injection on the same IRMS, coupled with a Thermo Scientific High Temperature Conversion Elemental Analyzer (TC/EA), equipped with an autosampler (Thermo Scientific AI/AS 3000). Each sample was injected and analyzed 5 times. This allowed for a final precision of 0.7‰ for δ2H. Vienna Standard Mean Ocean Water (VSMOW) and Standard Light Antarctic Precipitation (SLAP) were used as internal lab standards for both water isotopes. Isotopic composition is reported normalized to the composition of VSMOW, which is defined as 0‰ δ18O and 0‰ δ2H. Further, deuterium excess (D-excess) is defined as D-excess = δ2H-8⋅δ18O (Craig, 1961). All extracted plant water samples were analyzed with an IRMS (high-tech, high-cost) contradicting the low-cost character of this study. However, using an IRMS we could avoid issues with solutes released during the extraction that impact the accuracy of laser spectroscopes (West et al., 2010; Millar et al., 2018). In this way, our analysis could focus on the water extraction method, assuming the isotope analysis was reliable.
The isotopic compositions of water from the different extraction methods were compared in dual isotope space (i.e., plotting δ18O against δ2H). For each extraction method the average, standard deviation (SD), range (max-min) and the difference of the average composition of a method to the average of the reference plant water extraction were determined. The plant water extraction methods were also compared by Z-scores (Wassenaar et al., 2012):
where Mn is the isotopic composition of water extracted with the trial method (namely MO, MW, JJ, ICE, REFT, or REFS), MREF is the isotopic composition of the reference method, and SD is the analysis standard deviation. Instead of using the machine precision as SD (Wassenaar et al., 2012; Orlowski et al., 2016b), 1.44‰ for δ18O and 2.2‰ for δ2H was used as SD. These SD are based on the by Millar et al. (2018) reported average SD [leaf and stem obtained using the cryogenic vacuum extraction method Koeniger et al. (2011)] and were used in this study to better represent the natural variability of stable isotope composition in plant material. An adapted comparison criterion as proposed by Orlowski et al. (2016b) was used to reclassify Z-scores such that a Z-score <2 were comparable, scores from | 2–5| were considered acceptable and a score >5 was considered unacceptable.
Results
Plant Water Extraction
All four MacGyver methods (Figures 1b–e) were able to extract 0.5–2 ml water from most plant groups with extraction efficiencies ranging from 0.5 to 1.0 (Supplementary Figure S2). In addition, the extraction efficiency varied across methods and there were qualitative differences among methods that are noted as part of the assessment of these MacGyver methods.
The MO method (Figure 1b) could extract water from the indoor plant groups. Despite centrifugation, it was not possible to separate all water from the puree and therefore no extraction efficiency was determined (Figure 1b). In contrast, outdoor grass plant material were largely fibrous given the autumn such that it was not possible to obtain enough water for stable isotope analysis.
The MW method (Figure 1c) was effective for all plant groups but some water droplets remained inside the re-sealable zipper storage bags due to adhesion to the inner side of the bag.
The JJ method (Figure 1d) was able to extract water for the indoor grown plant samples but was not able to extract sufficient water (<0.5 ml) from lawn grass samples. Some water droplets could not be piped due to adhesion to the grass and jar.
The ICE method (Figure 1e) and the REF method (Figure 1a) extracted plant water from all plant groups. As such, there was not a marked difference in the extraction efficiency comparing the MW, JJ, ICE methods and “standard” research-grade extraction technique REF.
Simulating a 1 h car ride at 50°C, the plant weight after transport decreased by 0.1–0.15 g, with the different grass samples (indoor and outdoor) losing 3% and the melon samples losing 10% of total water content, respectively (Figure 1f). Simulating the effect of storage (freezing and thawing), the plant weight after thawing decreased by 0.1–0.15 g with the different grass samples (indoor and outdoor) losing 1–10% of the total water content while melon plants decreased by 0.5 g, which is 20% of total water content (Figure 1g). From the plant materials used in REFT and REFS, we could extract 1–3 ml of water (extraction efficiency 0.3–0.98, Supplementary Figure S2) using the REF method.
Isotopic Composition of Plant Water Extracted
Considering the REF method, the outdoor grass samples were more depleted in δ18O and δ2H relative to the indoor plant samples (Table 1). Moreover, the indoor plant samples showed evaporative enrichment, falling below the global meteoric water line (GMWL, Figure 2). In contrast, grass grown outdoors on the lawn or pasture clustered along the GMWL (Figure 2). The water used for irrigation of the indoor plants had a constant isotope composition (δ18O = −7.97 ± 0.3‰ and δ2H = −62.02 ± 0.5‰) throughout the experiment and was on the GMWL (Figure 2).
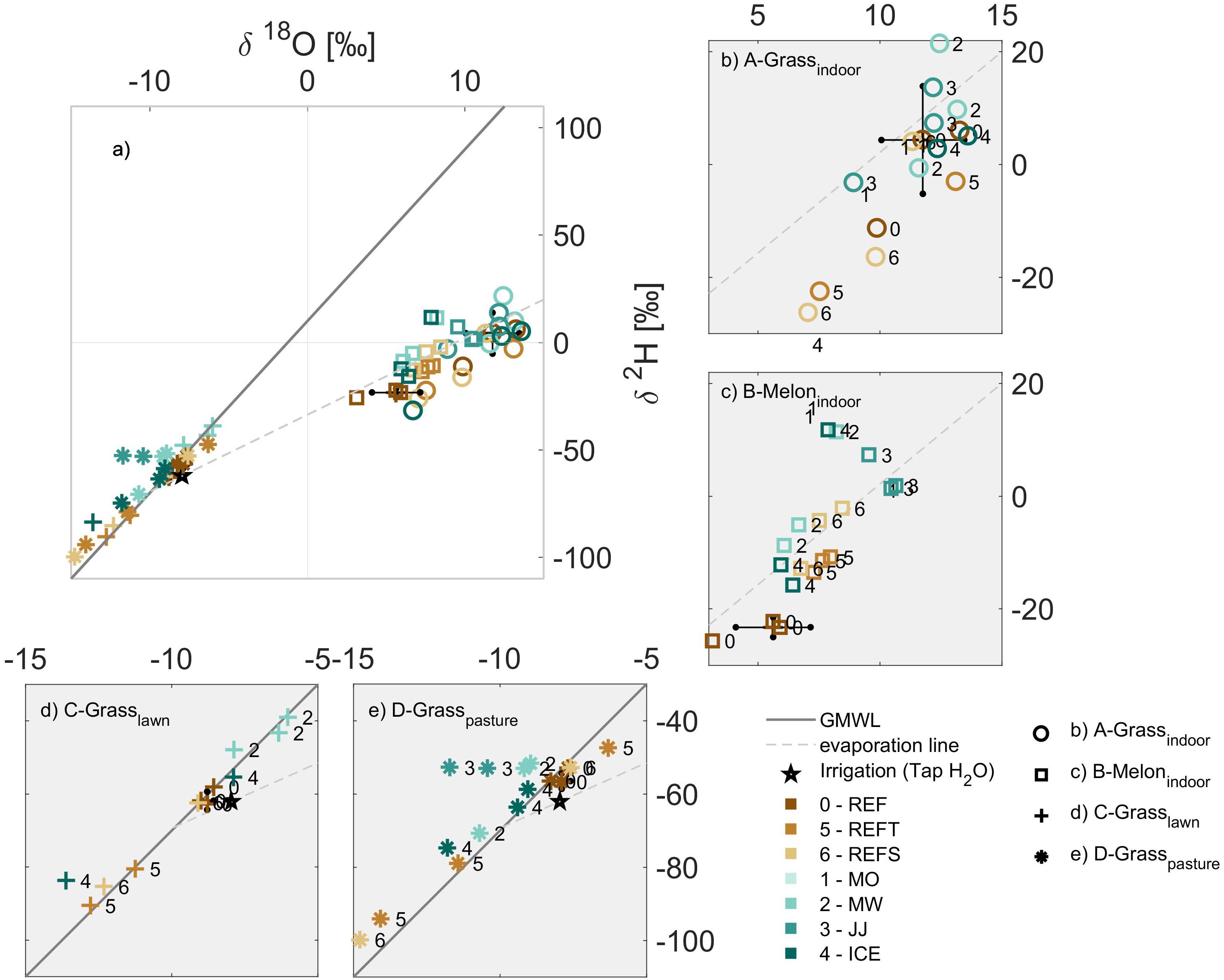
Figure 2. Dual isotope space (δ18O and δ2H) including all extracted plant water samples, the global meteoric water line (solid gray line), the evaporation line calculated as in Benettin et al. (2018) (dashed line) using the median irrigation water (star). The median and error of the REF are indicated as cross hairs with black closed circles. (a) Symbols indicate the indoor grass (circles), melon (square), campus lawn grass (cross), grass from the pasture (asterisk) and irrigation water (star). Different colors indicate cryogenic vacuum extraction (REF), simulated transport using REF (REFT), simulated storage using REF (REFS) and the different extraction methods MO, MW, JJ, and ICE (mojito, microwave, jam jar and ice cube, respectively). The side panels show data for each plant group (A-D) separately (b–e).
The isotopic range and SD obtained from a given method were 3‰ and 1.5‰ for δ18O, and 17.7‰ and 9‰ for δ2H respectively (Figure 2 and Table 1). The average isotope composition of water extracted with any single MacGyver method differed from the average of the REF extracted plant water (Table 1).
For the different grass samples (indoor, lawn and pasture), most extraction methods provided δ18O that were comparable (28 out of 32 samples, 88%) to those obtained from the REF method, such that Z-scores were less than 2 (Figure 3). The remaining extraction methods (4 out of 32 samples, 12%) provided δ18O values regarded as acceptable (Z-scores between 2 and 5, Figure 3). Many extraction methods yielded δ2H values comparable (11 out of 32 samples, 34%) to those from the REF method, such that Z-scores were less than 2 (Figure 3). Eight out of 32 samples (25%) provided acceptable δ2H values (Z-scores between 2 and 5; Figure 3), while the remaining (13 out of 32 samples, 45%) were different from those obtained with the reference method.
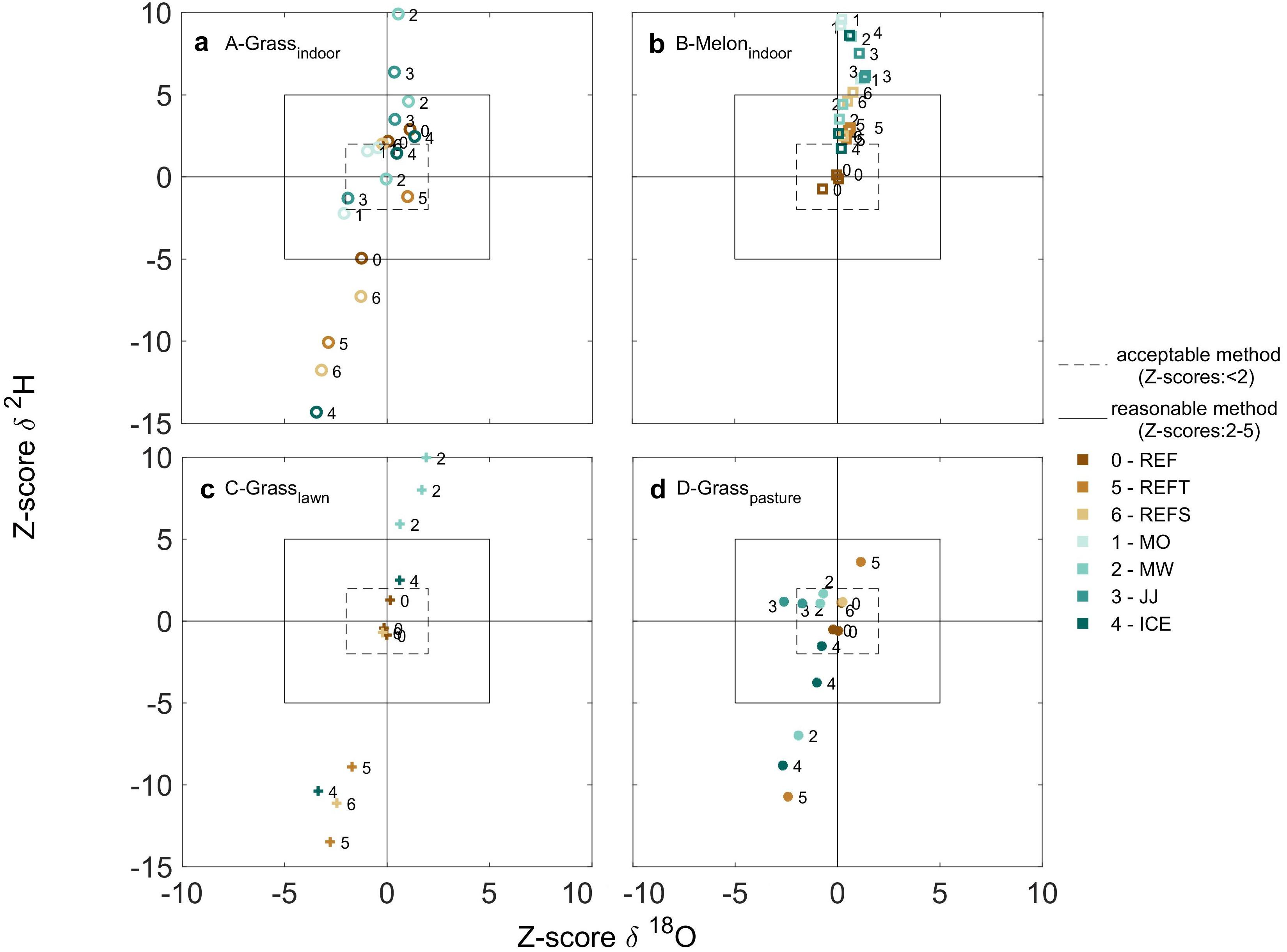
Figure 3. For each plant group A-D (a–d), performance of the different plant water extraction methods using the Z-scores. Symbols indicate the indoor grass (circles), melon (square), campus grass (cross), grass from the pasture (asterisk), and irrigation water (star). Different colors indicate cryogenic vacuum extraction (REF), simulated transport using REF (REFT), simulated storage using REF (REFS) and the different extraction methods MO, MW, JJ, and ICE (mojito, microwave, jam jar and ice cube, respectively).
The melon plants had fewer isotope values since not all methods were able to extract water (Figure 3b). For the methods that were able to extract water, most were comparable to the REF method for δ18O (4 out of 5 samples) such that Z-scores were less than 2 (Figure 3). The remaining extraction method (1 out of 5 samples) was acceptable for δ18O such that the Z-scores was between 2 and 5 (Figure 3). In contrast, most methods were different from the REF method for δ2H (4 out of 5 samples), such that Z-scores were larger than 5 (Figure 3). This lower reliability for δ2H with melon plants could not be explained by lower extraction efficiencies (Supplementary Figure S3).
Most of the samples affected by simulated transport or storage (REFT and REFS) were comparable (7 out of 14, 50%) or acceptable (7 out of 14 samples, 50%) for δ18O when compared to values obtained from the REF method, such that Z-scores were less than 5 (Figure 3). In contrast, most of the samples affected by transport or storage (REFT and REFS) were unacceptable for δ2H (9 out of 14, 65%), such that Z-scores were larger than 5. This result indicates a significant influence of transport and storage on the isotopic composition of plant water.
Discussion
Usability of the Different Extraction Methods
Each of the investigated MacGyver methods has advantages and disadvantages concerning usability and efficiency to extract water (Figure 1). The MO method was easy to use in the field or in laboratory but could not extract water from fibrous plants. The MW extraction was quick and able to extract water from all different plant materials considered, but some water droplets remained in the bag, which likely had an effect on the calculated extraction efficiency and isotope composition of the extracted water. The JJ method can be applied nearly everywhere, including in remote areas with only access to an outdoor stove or fire, but had difficulty to extract water in fibrous plants and water droplets adhering to the leaves and jar, possibly effecting the calculated extraction efficiency and isotope composition of the extracted water. The disadvantage of the ICE method, which is a low-technology variant of the REF method, was that more materials including ice cubes were needed compared to other MacGyver methods. Still, a benefit of the ICE method was that it could extract water from all different plants considered and no additional safety or training aspects were needed (e.g., handling liquid nitrogen −196 °C). Hence, the ICE method could be used safely in citizen science and/or school projects.
Even though the low-technology and low-cost plant water extraction methods were able to effectively and economically extract water from plants, different aspects need to be examined in more detail. In the methods based on heating plant material to release plant water (MW, JJ, and ICE), a fixed extraction time of 1 h was selected from literature values (West et al., 2006; Koeniger et al., 2011). However, as observed by West et al. (2006), the extraction time of cryogenic vacuum extraction affects the stable isotope composition. Therefore, as a next step as we seek to develop and differentiate these MacGyver methods, the extraction time should be optimized for each method and investigate the effect of co-extracted chemical compounds on laser spectroscopes. In addition, it is necessary to test all methods (MO, MW, JJ, and ICE) on other types of plant (e.g., trees).
Method Precision and Plant Water Isotopic Composition
Besides the effectiveness and applicability of each method considered in this study, it is important to assess how the isotopic signatures of the extracted water compare across the different methods.
The different MacGyver plant water extraction methods were able to extract water across a range of plant species and growing conditions (Figure 2). The methods seemed to correctly capture the observed evaporative enrichment in the indoor-grown plants and that outdoor grass had an isotopic composition similar to that of the GMWL (Figure 2). Single outliers in isotopic composition can be explained by the freezing of the outer and inner part of the syringe (for both ICE and REF) in proximity to the ice or liquid nitrogen, which blocked flows near EXE-II and impacted extraction and eventually the isotopic composition.
Comparing the isotope composition of the different plant water extraction methods across the different plant groups, the ICE method provided results that were closest to the REF method (Figures 1, 3 and Supplementary Figure S4). However, also a range of plant water stable isotope compositions larger than the precision of the stable isotope analyzer could be noticed (Figure 2). The average range of 3‰ for δ18O for the different MacGyver methods is large but also similar to that reported by Millar et al. (2018) and West et al. (2006) using standard research-grade extraction methods. As such, the MacGyver methods can be used with some confidence knowing that the relative performance regarding final stable isotope composition is equivalent to high-tech and high-cost methods.
In general, the performance of the different methods for δ18O were acceptable for all plants and for δ2H for the grasses (Figure 3 and Supplementary Figure S4). The higher deviation of Z-scores for δ2H of melon plant water suggests either that fractionation occurred during sample processing or that the melon plants used in this study experience greater transpiration from the leaves. Unfortunately, due to the limited amount of plant material considered, we could not further investigate this effect through separating the different plant components (e.g., roots, stems and leaves) as was done by e.g., Millar et al. (2018). In addition, it is also uncertain whether each method extracts the same water pool from each plant type or if different pools of water are extracted according to method (e.g., water from stems vs. leaves, or from xylem vs. intercellular water). This issue begs the question of how representative any bulk extraction method (i.e., cryogenic vacuum distillation) would be when it removes all water from plant tissues.
Potential Effect of Transport and Storage on the Stable Isotope Composition
Water samples for stable isotope analysis are typically collected in the field using bottles (preferably glass or HDPE-bottles) and hermetically sealed with a cap. Under such conditions, samples collected today could be analyzed years later (Spangenberg, 2012). Our study, however, highlights that during transport from the field to the laboratory the plant water stable isotope compositions can change considerable (Figures 1, 3, 4) and to a greater extent than the accuracy of extraction and stable isotope analysis. When plants are collected and not immediately cooled, plant material continues to transpire or lose water via evaporation from the cut surfaces, resulting in water loss of up to 10% compared to the initial sample weight. Hence, it is advisable to cool the plant material directly in the field. In addition, a common practice is to store the collected plant material in a freezer until processing to prevent decomposition and fractionation until the plant water is extracted. When freezing plant material, the cell walls burst. Upon thawing, there can be 10–20% loss of the total plant water impacting the remaining plant water isotopic composition.
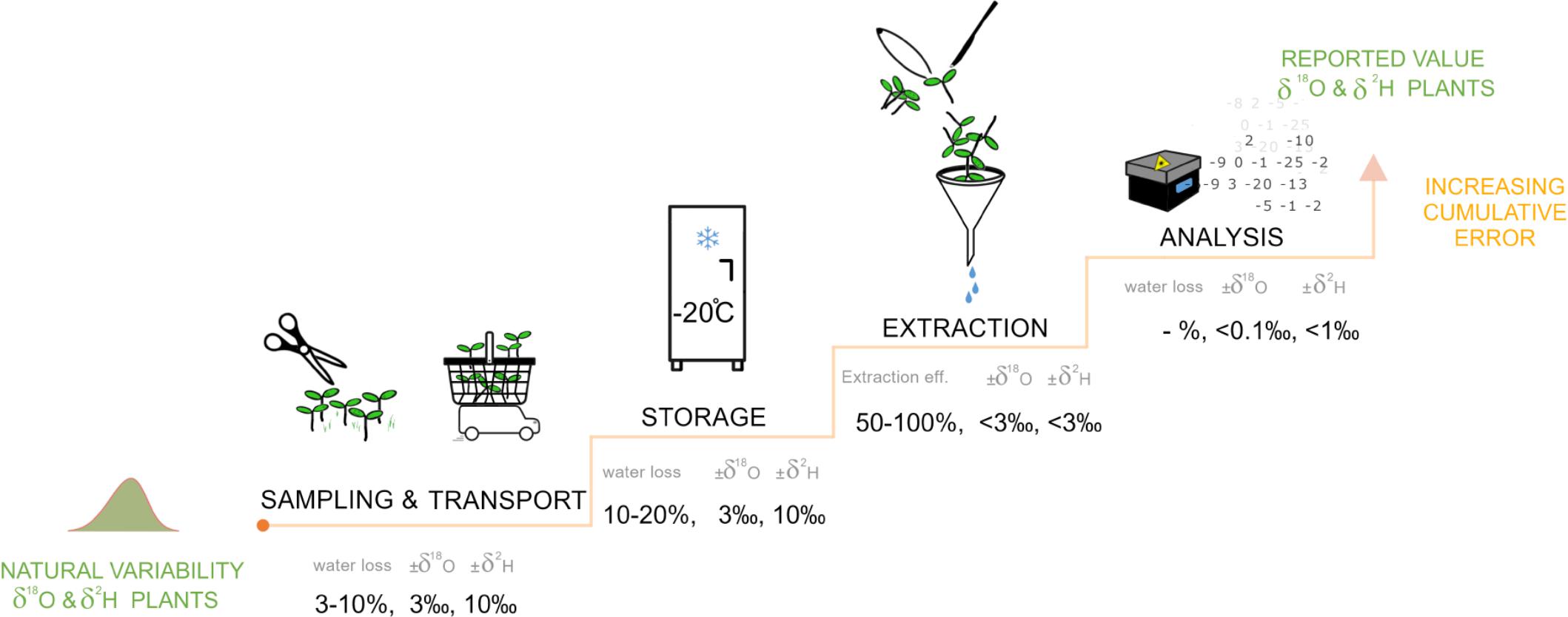
Figure 4. The process chain from plant sample collection and transport, storage, plant water extraction and isotope analysis to the final isotope composition. At each step, the potential water loss and associated error in isotopic composition for δ18O and δ2H are indicated as % and ‰, respectively. With each step the cumulative error in the isotope composition increases, highlighting the importance to focus not only on the extraction technique but on the full process chain.
Clearly, focusing only on equipment and laboratory techniques while neglecting how consistency in transport and storage can impact isotopic composition can bring about significant misinterpretations. This is where MacGyver methods could provide a remedy or supplement to standard methods such as the cryogenic vacuum extraction (Koeniger et al., 2011) or direct vapor equilibration method as proposed by Millar et al. (2018), by virtue of their speed and ease of use to help bring about consistency.
Concluding Remarks
Our results show that simple MacGyver methods can generate isotopic data with a precision that is generally comparable to that of higher-demand research grade methods. In addition, we demonstrated that it is necessary to consider the full process chain from plant sample collection to isotope analysis, as there are several possible sources of errors along this chain (Figure 4). All plant water extraction methods have sources of error and uncertainty, which can be controlled through adequate methodological characterization and clearly defined protocols. Therefore, the MacGyver plant water extraction methods presented here are methodologically promising for the rapid expansion of isotopic investigation especially in remote areas with technological limitations or in citizen science and/or school projects that require high safety standards.
Data Availability
All datasets generated for this study are included in the manuscript and/or the Supplementary Files.
Author Contributions
BF designed and performed all the lab work and wrote the first draft of the manuscript. JF contributed in analyzing the isotopic composition of the water samples. All authors contributed to the revision of the manuscript, read, and approved the submitted version.
Funding
This research was supported by the Bolin RA7 seed funding 2018 and by the Agricultural Water Innovations in the Tropics (AgWIT) project funded by the joint call of the Water Joint Programming Initiative (Water JPI) and the Joint Programming Initiative on Agriculture, Food Security and Climate Change (FACCE-JPI) of the European Union and partner countries. SM and SL acknowledge partial support from the Swedish Research Agencies Vetenskapsrådet, Formas, and Sida through the joint call on Sustainability and Resilience – Tackling Climate and Environmental Changes (grant VR 2016-06313). SM also acknowledges Formas (grant 2016-00998).
Conflict of Interest Statement
The authors declare that the research was conducted in the absence of any commercial or financial relationships that could be construed as a potential conflict of interest.
Acknowledgments
We thank all the people who helped and contributed to this study. Martina Hättestrand supporting the laboratory extraction, Anna Scaini for sample logistics, and Dr. Christian Ceccon for the isotope analysis.
Supplementary Material
The Supplementary Material for this article can be found online at: https://www.frontiersin.org/articles/10.3389/feart.2019.00150/full#supplementary-material
FIGURE S1 | Growing tray with indoor grass-A in windowsill (a), growing tray with melon-B in windowsill (b), outdoor grass-C lawn with sampling locations indicated by re-sealable zipper storage bags (c), outdoor grass-D pasture (source: Google Street view) (d), pod with indoor grass-A just before harvest (e) and pods with melon just before harvest (f).
FIGURE S2 | For each plant group (column A–D), the boxplots show δ2H, δ18O, deuterium excesses (D-exe), and water extraction efficiency. The letters indicate the cryogenic vacuum (REF), simulated transport using REF (REFT), simulated storage using REF (REFS), mojito (MO), microwave (MW), jam jar (JJ) and ice cube (ICE) extraction methods. The red line indicates the median of the REF, and the gray lines indicate the analytical standard deviation.
FIGURE S3 | The extraction efficiency as a function of δ18O Z-scores (top row) and deuterium excess Z-scores (bottom row) for the different extraction methods: cryogenic vacuum (REF), simulated transport using REF (REFT), simulated storage using REF (REFS), microwave (MW), jam jar (JJ) and ice cube (ICE) extraction methods. Symbols indicate the indoor grass (circles), melon (square), grass from the lawn (cross), grass from the pasture (asterisk) and irrigation water (star). Different colors indicate cryogenic vacuum extraction (REF), simulated transport using REF (REFT) and simulated storage using REF (REFS).
FIGURE S4 | Data of Figure 3 represented as boxplot to compare the different plant water extraction methods (mojito (MO), jam jar (JJ) and ice cube (ICE) extraction methods) for plant groups A, C, and D (plant group B excluded due to few data points) using the Z-score. Individual data points are indicated with circles and boxes indicate the 25 and 75th percentiles.
TABLE S1 | The complete stable isotope dataset that was generated and analyzed for this study.
References
Arbouw, E. (2018). Cold Wine or Delicious Nachos on the Beach (Koud wijntje of heerlijke nacho’s op het strand). Amsterdam: Volkskrant.
Benettin, P., Volkmann, T. H. M., von Freyberg, J., Frentress, J., Penna, D., Dawson, T. E., et al. (2018). Effects of climatic seasonality on the isotopic composition of evaporating soil waters. Hydrol. Earth Syst. Sci. 22, 2881–2890. doi: 10.5194/hess-22-2881-2018
Berman, E. S. F., Gupta, M., Gabrielli, C., Garland, T., and McDonnell, J. J. (2009). High-frequency field-deployable isotope analyzer for hydrological applications. Water Resour. Res. 45:W10201. doi: 10.1029/2009WR008265
Beyer, M., Koeniger, P., Gaj, M., Hamutoko, J. T., Wanke, H., and Himmelsbach, T. (2016). A deuterium-based labeling technique for the investigation of rooting depths, water uptake dynamics and unsaturated zone water transport in semiarid environments. J. Hydrol. 533, 627–643. doi: 10.1016/j.jhydrol.2015.12.037
Bhamla, M. S., Benson, B., Chai, C., Katsikis, G., Johri, A., and Prakash, M. (2017). Hand-powered ultralow-cost paper centrifuge. Nat. Biomed. Eng. 1:0009. doi: 10.1038/s41551-016-0009
Brooks, R. J., Barnard, H. R., Coulombe, R., and McDonnell, J. J. (2010). Ecohydrologic separation of water between trees and streams in a Mediterranean climate. Nat. Geosci. 3, 100–104. doi: 10.1038/ngeo722
Craig, H. (1961). Isotopic variations in meteoric waters. Science 133, 1702–1703. doi: 10.1126/science.133.3465.1702
Dahlke, H. E., Lyon, S. W., Jansson, P., Karlin, T., and Rosqvist, G. (2014). Isotopic investigation of runoff generation in a glacierized catchment in northern Sweden. Hydrol. Process. 28, 1383–1398. doi: 10.1002/hyp.9668
Dalton, F. N. (1989). “Plant root water extraction studies using stable isotopes,” in Structural and Functional Aspects of Transport in Roots Developments in Plant and Soil Sciences, eds B. C. Loughamn, O. Gašparíková, and J. Kolek (Dordrecht: Springer), 151–155. doi: 10.1007/978-94-009-0891-8_29
Dansgaard, W. (1953). The abundance of O18 in atmospheric water and water vapour. Tellus 5, 461–469. doi: 10.1111/j.2153-3490.1953.tb01076.x
Dawson, T. E., and Ehleringer, J. R. (1991). Streamside trees that do not use stream water. Nature 350, 335–337. doi: 10.1038/350335a0
Fischer, B. M. C., Rinderer, M., Schneider, P., Ewen, T., and Seibert, J. (2015). Contributing sources to baseflow in pre-alpine headwaters using spatial snapshot sampling. Hydrol. Process. 29, 5321–5336. doi: 10.1002/hyp.10529
Fischer, B. M. C., Stähli, M., and Seibert, J. (2017). Pre-event water contributions to runoff events of different magnitude in pre-alpine headwaters. Hydrol. Res. 48, 28–47. doi: 10.2166/nh.2016.176
Goldsmith, G. R., Allen, S. T., Braun, S., Engbersen, N., González-Quijano, C. R., Kirchner, J. W., et al. (2018). Spatial variation in throughfall, soil, and plant water isotopes in a temperate forest. Ecohydrology 12:e2059. doi: 10.1002/eco.2059
Good, S. P., Mallia, D. V., Lin, J. C., and Bowen, G. J. (2014). Stable isotope analysis of precipitation samples obtained via crowdsourcing reveals the spatiotemporal evolution of superstorm Sandy. PLoS One 9:e91117. doi: 10.1371/journal.pone.0091117
Kerstel, E. R. T., van Trigt, R., Reuss, J., and Meijer, H. A. J. (1999). Simultaneous determination of the 2H/1H, 17O/16O, and 18O/16O isotope abundance ratios in water by means of laser spectrometry. Anal. Chem. 71, 5297–5303. doi: 10.1021/ac990621e
Klaus, J., and McDonnell, J. J. (2013). Hydrograph separation using stable isotopes: review and evaluation. J. Hydrol. 505, 47–64. doi: 10.1016/j.jhydrol.2013.09.006
Kockmann, N. (2014). “Chapter 1 - History of distillation,” in Distillation, eds A. Górak and E. Sorensen (Boston, MA: Academic Press), 1–43. doi: 10.1016/B978-0-12-386547-2.00001-6
Koeniger, P., Marshall, J. D., Link, T., and Mulch, A. (2011). An inexpensive, fast, and reliable method for vacuum extraction of soil and plant water for stable isotope analyses by mass spectrometry: vacuum extraction of soil and plant water for stable isotope analyses. Rapid Commun. Mass Spectrom. 25, 3041–3048. doi: 10.1002/rcm.5198
Koutsouris, A. J., and Lyon, S. W. (2018). Advancing understanding in data-limited conditions: estimating contributions to streamflow across Tanzania’s rapidly developing Kilombero Valley. Hydrol. Sci. J. 63, 197–209. doi: 10.1080/02626667.2018.1426857
Lis, G., Wassenaar, L. I., and Hendry, M. J. (2008). High-precision laser spectroscopy D/H and 18O/16O Measurements of microliter natural water samples. Anal. Chem. 80, 287–293. doi: 10.1021/ac701716q
Lyon, S. W., Desilets, S. L. E., and Troch, P. A. (2009). A tale of two isotopes: differences in hydrograph separation for a runoff event when using δD versus δ18O. Hydrol. Process. 23, 2095–2101. doi: 10.1002/hyp.7326
McDonnell, J. J. (2014). The two water worlds hypothesis: ecohydrological separation of water between streams and trees?: the two water worlds hypothesis. Wiley Interdiscip. Rev. 1, 323–329. doi: 10.1002/wat2.1027
Millar, C., Pratt, D., Schneider, D. J., and McDonnell, J. J. (2018). A comparison of extraction systems for plant water stable isotope analysis. Rapid Commun. Mass Spectrom. 32, 1031–1044. doi: 10.1002/rcm.8136
Munksgaard, N. C., Cheesman, A. W., Wurster, C. M., Cernusak, L. A., and Bird, M. I. (2014). Microwave extraction-isotope ratio infrared spectroscopy (ME-IRIS): a novel technique for rapid extraction and in-line analysis of δ18O and δ2H values of water in plants, soils and insects. Rapid Commun. Mass Spectrom. 28, 2151–2161. doi: 10.1002/rcm.7005
Orlowski, N., Breuer, L., Angeli, N., Boeckx, P., Brumbt, C., Cook, C. S., et al. (2018). Inter-laboratory comparison of cryogenic water extraction systems for stable isotope analysis of soil water. Hydrol. Earth Syst. Sci. 22, 3619–3637. doi: 10.5194/hess-22-3619-2018
Orlowski, N., Breuer, L., and McDonnell, J. J. (2016a). Critical issues with cryogenic extraction of soil water for stable isotope analysis: issues with cryogenic soil water extraction. Ecohydrology 9, 1–5. doi: 10.1002/eco.1722
Orlowski, N., Pratt, D. L., and McDonnell, J. J. (2016b). Intercomparison of soil pore water extraction methods for stable isotope analysis: intercomparison of soil pore water extraction methods. Hydrol. Process. 30, 3434–3449. doi: 10.1002/hyp.10870
Peters, L. I., and Yakir, D. (2008). A direct and rapid leaf water extraction method for isotopic analysis. Rapid Commun. Mass Spectrom. 22, 2929–2936. doi: 10.1002/rcm.3692
Rinderer, M., Kollegger, A., Fischer, B. M. C., Stähli, M., and Seibert, J. (2012). Sensing with boots and trousers — qualitative field observations of shallow soil moisture patterns. Hydrol. Process. 26, 4112–4120. doi: 10.1002/hyp.9531
Sklash, M. G., Farvolden, R. N., and Fritz, P. (1976). A conceptual model of watershed response to rainfall, developed through the use of oxygen-18 as a natural tracer. Can. J. Earth Sci. 13, 271–283. doi: 10.1139/e76-029
Spangenberg, J. E. (2012). Caution on the storage of waters and aqueous solutions in plastic containers for hydrogen and oxygen stable isotope analysis: stable isotope variation of water stored in plastic bottles. Rapid Commun. Mass Spectrom. 26, 2627–2636. doi: 10.1002/rcm.6386
Sprenger, M., Herbstritt, B., and Weiler, M. (2015). Established methods and new opportunities for pore water stable isotope analysis: pore water stable isotope analysis. Hydrol. Process. 29, 5174–5192. doi: 10.1002/hyp.10643
Vendramini, P. F., Sternberg, L., and da, S. L. (2007). A faster plant stem-water extraction method. Rapid Commun. Mass Spectrom. 21, 164–168. doi: 10.1002/rcm.2826
Volkmann, T. H. M., Kühnhammer, K., Herbstritt, B., Gessler, A., and Weiler, M. (2016). A method for in situ monitoring of the isotope composition of tree xylem water using laser spectroscopy. Plant Cell Environ. 39, 2055–2063. doi: 10.1111/pce.12725
von Freyberg, J., Studer, B., and Kirchner, J. W. (2016). A lab in the field: high-frequency analysis of water quality and stable isotopes in streamwater and precipitation. Hydrol. Earth Syst. Sci. Discuss. 2016, 1–32. doi: 10.5194/hess-2016-585
Wassenaar, L. I., Ahmad, M., Aggarwal, P., van Duren, M., Pöltenstein, L., Araguas, L., et al. (2012). Worldwide proficiency test for routine analysis of δ2H and δ18O in water by isotope-ratio mass spectrometry and laser absorption spectroscopy. Rapid Commun. Mass Spectrom. 26, 1641–1648. doi: 10.1002/rcm.6270
Wassenaar, L. I., Hendry, M. J., Chostner, V. L., and Lis, G. P. (2008). High resolution pore water δ 2H and δ18O measurements by H2O (liquid) -H2O (vapor) equilibration laser spectroscopy. Environ. Sci. Technol. 42, 9262–9267. doi: 10.1021/es802065s
West, A. G., Goldsmith, G. R., Brooks, P. D., and Dawson, T. E. (2010). Discrepancies between isotope ratio infrared spectroscopy and isotope ratio mass spectrometry for the stable isotope analysis of plant and soil waters. Rapid Commun. Mass Spectrom. 24, 1948–1954. doi: 10.1002/rcm.4597
Keywords: plant water extraction, cryogenic vacuum extraction, stable water isotopes, method comparison, plant sample transport, plant sample storage, low-tech and low-cost
Citation: Fischer BMC, Frentress J, Manzoni S, Cousins SAO, Hugelius G, Greger M, Smittenberg RH and Lyon SW (2019) Mojito, Anyone? An Exploration of Low-Tech Plant Water Extraction Methods for Isotopic Analysis Using Locally-Sourced Materials. Front. Earth Sci. 7:150. doi: 10.3389/feart.2019.00150
Received: 27 March 2019; Accepted: 27 May 2019;
Published: 20 June 2019.
Edited by:
Rolf Hut, Delft University of Technology, NetherlandsReviewed by:
Tim van Emmerik, Delft University of Technology, NetherlandsNatalie Ceperley, École Polytechnique Fédérale de Lausanne, Switzerland
Copyright © 2019 Fischer, Frentress, Manzoni, Cousins, Hugelius, Greger, Smittenberg and Lyon. This is an open-access article distributed under the terms of the Creative Commons Attribution License (CC BY). The use, distribution or reproduction in other forums is permitted, provided the original author(s) and the copyright owner(s) are credited and that the original publication in this journal is cited, in accordance with accepted academic practice. No use, distribution or reproduction is permitted which does not comply with these terms.
*Correspondence: Benjamin M. C. Fischer, YmVuamFtaW4uZmlzY2hlckBuYXRnZW8uc3Uuc2U=