- 1Department of Earth Sciences, University of Minnesota, Minneapolis, MN, United States
- 2Department of Plant and Microbial Biology, University of Minnesota, St. Paul, MN, United States
- 3Biotechnology Institute, University of Minnesota, St. Paul, MN, United States
Mounting geochemical evidence suggests microorganisms capable of oxygenic photosynthesis (e.g., Cyanobacteria) colonized Archean continental surfaces, driving oxidative weathering of detrital pyrites prior to the 2.5 Ga Great Oxidation Event (e.g., Stüeken et al., 2012; Reinhard et al., 2013; Lalonde and Konhauser, 2015; Havig et al., 2017a). Modern terrestrial environments dominated by biofilms comprised of phototrophs include hydrothermal systems (e.g., Yellowstone National Park) and hypolithic communities found in arid to hyper-arid deserts (e.g., McMurdo Dry Valleys of Antarctica, Atacama Desert of Chile). Here, we explore phototrophic communities in both hypolithic and hot spring environments in Yellowstone National Park as potential analogs to Archean continental surfaces. Hypolithic communities in geothermal settings were similar in both composition and carbon uptake rates to proximal hot spring communities. It is our opinion that hydrothermal area hypolithic communities represent modern analogs of communities that colonized Archean continental surfaces, producing oxygen locally, and facilitating microbially-mediated pyrite oxidation prior to the presence of free oxygen in the global atmosphere.
In the distant future, humans explore an Earth-like planet that exhibits all the hallmarks of falling in the “habitable zone” (Seager, 2013) of a young yellow M type star. As predicted by previous terrestrially based spectroscope measurements (Seager and Deming, 2010), the atmosphere of the planet has no detectable O2. While scanning the surface of a continent rising above the global ocean (Taylor and McLennan, 1995; Collerson and Kamber, 1999), the team observes active hydrothermal areas drained by rivers of bright orange waters, similar to those impacted by acid mine drainage on Earth. The occurrence of acid mine drainage (or acid rock drainage or ARD) requires an input of oxygen, and on Earth, this reaction is mediated by microbial communities (e.g., Edwards et al., 2000; Johnson et al., 2002; Havig et al., 2017a). Based on this, the scientists pick that location to search for the source of oxygen feeding this putative ARD site. The timing of the generation of free oxygen via oxygenic photosynthesis on Earth during the Archean has long been debated (e.g., Anbar et al., 2007; Kump and Barley, 2007; Wille et al., 2007; Frei et al., 2009; Holland, 2009; Reinhard et al., 2009; Crowe et al., 2013; Olson et al., 2013; Zahnle et al., 2013; Planavsky et al., 2014; Gumsley et al., 2017), and perhaps this analog planet can provide evidence to settle the debate. Upon landing, measurements show the land surface is bombarded with high levels of ultraviolet radiation due to higher luminosity emitted by the outer atmosphere (Ratner and Walker, 1972; Pavlov et al., 2001), and although the gravity, geology, and atmospheric pressure are similar to Earth, the eerie orange haze (Zerkle et al., 2012) gives this planet a distinctly alien feel. On Earth there is contentious evidence for hydrothermal life potentially as far back as 4.2 Ga (Dodd et al., 2017), for terrestrial hot springs as early as 3.5 Ga (Djokic et al., 2017), and growing support for a possible origin of life in hydrothermal systems (Deamer and Georgiou, 2015; Forsythe et al., 2015; Van Kranendonk et al., 2017; Milshteyn et al., 2018). However, the temperatures of the acidic and alkaline hot springs in the hydrothermal area adjacent to the orange-stained riverbed are out of the range known to support photosynthesis (Brock, 1967; Cox et al., 2011; Boyd et al., 2012), explaining the lack of detectable phototrophs. This would seem to rule out the hydrothermal area as a source for oxygen. Perplexed, the team Geobiologist examines the white siliceous sinter that blankets the ground under her feet. She crouches to examine an area disturbed by her footprints, and a smile quickly spreads across her face. Where her footprints remove the loose silica sinter on the surface, a layer of green pigmented biofilm is revealed underneath (Figure 1).
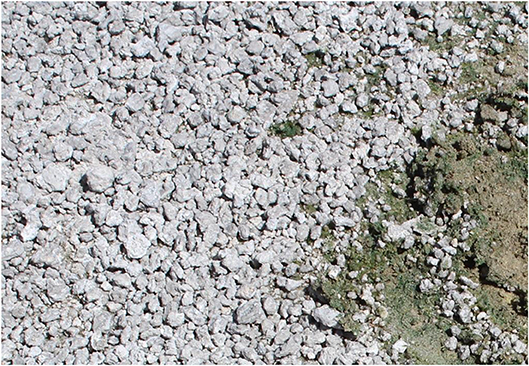
Figure 1. Zoom in highlighting pigmented phototrophic microbial community exposed following disturbance of a thin loose siliceous sinter cover layer due to a footstep, Norris Geyser Basin, Yellowstone National Park, WY, USA. Image is ~15 cm across. Research was conducted under Yellowstone Research Permit YELL-2016-SCI-7020.
Hypolithic (Sub-sinter) Microbial Communities as O2 Producers
Mounting geochemical evidence in rocks of Archean age suggests free oxygen driving oxidative weathering as far back as 3.0 Ga (e.g., Anbar et al., 2007; Kump and Barley, 2007; Wille et al., 2007; Frei et al., 2009; Holland, 2009; Reinhard et al., 2009; Crowe et al., 2013; Zahnle et al., 2013; Planavsky et al., 2014). In contrast, minor sulfur isotope mass independent fractionation (MIF) recorded in sulfide and sulfate minerals suggests there was no free atmospheric oxygen until ~2.45 Ga (e.g., Havig et al., 2017b and references therein), leaving a ~500-million-year mystery. Explanations include oxygenic photosynthesis occurring in the oceans generating transient “whiffs” of atmospheric oxygen (Anbar et al., 2007) or localized “oxygen oases” (Olson et al., 2013). Neither of these explanations of localized ocean oxygen production account for evidence of oxidative weathering on continental surfaces prior to the Great Oxidation Event (Reinhard et al., 2009; Stüeken et al., 2012; Lalonde and Konhauser, 2015; Havig et al., 2017a). Furthermore, transport of transient “whiffs” of O2 from the ocean in quantities large enough to drive oxidative weathering of terrestrial surfaces is problematic in a reducing atmosphere with high UV irradiance (Pavlov et al., 2001), and the presence of detrital oxygen-sensitive pyrite and uraninite in many riverine and delta deposits coupled to MIF values is strong evidence for a reducing atmosphere with a low O2 concentration (Catling and Claire, 2005; Johnson J. E. et al., 2014; Hao et al., 2017). Recent work has suggested a locally generated (i.e., terrestrial) source of O2 could drive oxidative weathering locally without the need for free O2 in the atmosphere (Konhauser et al., 2011; Reinhard et al., 2013; Lalonde and Konhauser, 2015; Havig et al., 2017a; Lenton and Daines, 2017). This scenario would require oxygen-producing microorganisms to overcome high UV radiation (Cnossen et al., 2007), desiccation, and rapid erosion. Arid to hyper-arid environments where phototrophic communities are found under quartz gravels (e.g., Lacap-Bugler et al., 2017 and references therein) likely do not best represent Archean continental surfaces due to low levels of liquid water. Most places where there is abundant water, vascular plants colonize and dominate, out-competing microbial biofilms. A solution to these problems is observed in modern hydrothermal areas, where siliceous sinter gravels and sands act as a translucent mulch layer, water is abundant, and hydrothermal activity limits the presence of vascular plants (Figure 1, Supplementary Figures 3, 4). In Yellowstone National Park, a carpet of siliceous sinter is generated where circum-neutral to alkaline silica-saturated hydrothermal fluids precipitate sinters. In acidic geothermal systems, sulfuric acid acts to dissolve the local rhyolitic bedrock, leaving behind weathering resistant minerals (e.g., quartz) that can become coated with amorphous silica precipitate (Supplementary Figure 1). In YNP, phototrophic microbial communities underlie loose siliceous sinter deposited from both acidic and circum-neutral to alkaline hot springs (Figure 1). It is our opinion that hypolithic phototrophic communities in these hydrothermal systems represent a modern analog for terrestrial oxygen oases in the Archean.
Experimental Findings
Previous work has shown that phototrophic endolithic microbial communities in hydrothermal areas thrive under a protective coating of silica, and may provide clues about life in ancient hydrothermal environments (Walker et al., 2005). Here, we examined extant phototrophic microbial communities in hydrothermal systems to constrain the productivity of these assemblages and interpret their potential role in oxidizing continental surfaces during the Archean. Photosynthetic microbial communities were sampled from three hydrothermal areas, including the Norris Geyser Basin, the Gibbon Geyser Basin, and the Lower Geyser Basin in Yellowstone National Park, WY, USA (Supplementary Figure 2). The sample sites (Figure 2; Supplementary Figure 3) represent separate niches within close proximity: (i) sub-sinter offering UV protection via the overlying amorphous silica sinter (three sites); (ii) subaerial microbial communities relying on biological (pigment, carotenoid) protection from UV radiation damage (Aigner et al., 2013; Holzinger and Pichrtová, 2016) (three sites); and (iii) fully submerged/subaqueous microbial communities (three sites). We characterized microbial community composition and performed in situ carbon uptake mesocosms to assess primary productivity.
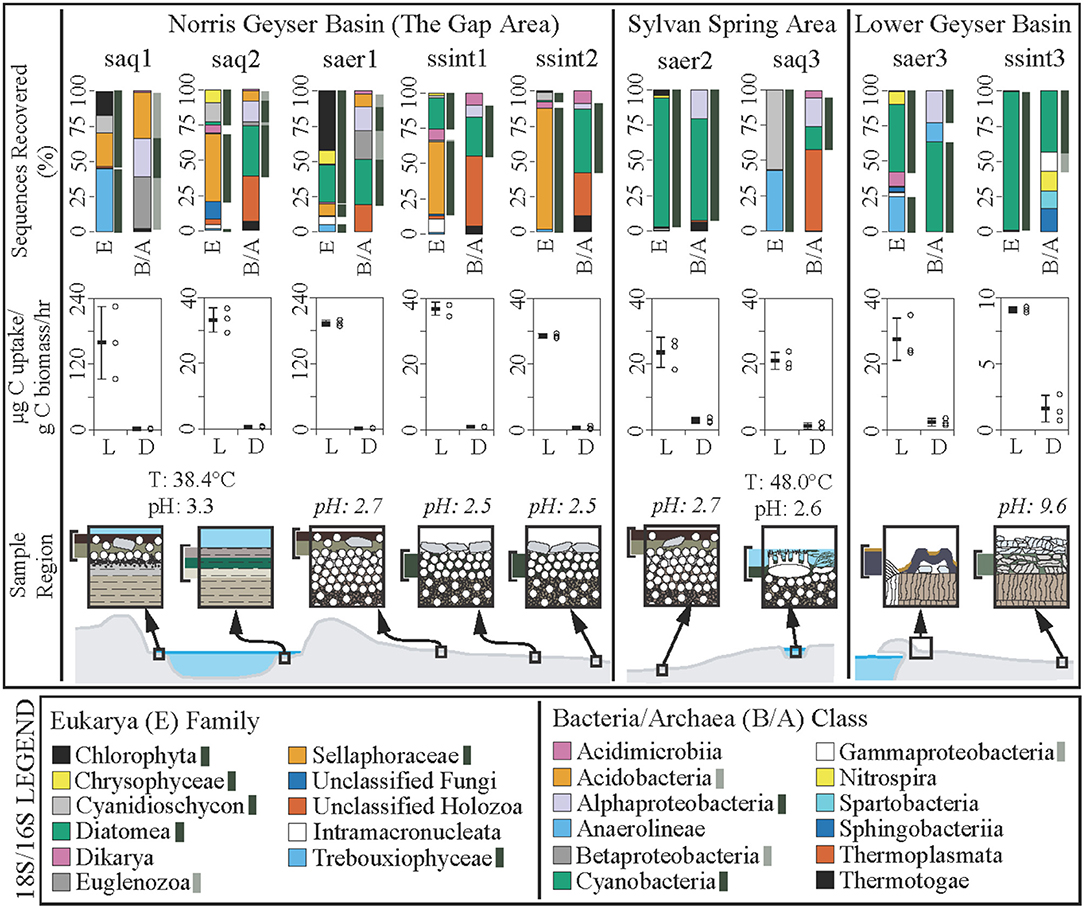
Figure 2. Community composition and carbon uptake rates in hydrothermal areas of the Norris Geyser Basin, Sylvan Spring Area of the Gibbon Geyser Basin, and Sentinel Meadows area of the Lower Geyser Basin, Yellowstone National Park, WY, USA. Sample identifications: saq, subaqueous; saer, subaerial; ssint, sub-sinter. Composition of small subunit rRNA genes recovered at the Family level for Eukarya (E) and at the Class level for Bacteria and Archaea (B/A). Dark green bars indicate OTUs most closely related to characterized phototrophs. Light green bars indicate OTUs affiliated with sequences of organisms that are likely phototrophic. Carbon uptake experiments (middle) are presented as the mean with standard deviation (black bar with error bars) and the raw data (open black circles) for incubations conducted in the light (L) or dark (D), in units of μg C per gram of biomass C per hour. Note differences in y-axis values. Cartoon cross sections of the sample locations (bottom) indicating the regions sampled and their relative spatial/environmental correlation. Temperature (T) and pH of sampled hot springs are given, with estimates of pH values (in italics) for subaerial and sub-sinter sample sites given based on pH determination from ~1:1 addition of 18.2 MΩ/cm water to associated sediments.
Based on16S and 18S rRNA gene sequencing, microbial communities from each niche are comprised of predominantly phototrophic Eukarya and Bacteria including algae, cyanobacteria, and Alphaproteobacteria (Figure 2, top row). The recovery of active cyanobacterial sequences from acidic sites is consistent with previous work demonstrating the occurrence of acid-tolerant cyanobacteria (Steinberg et al., 1998; Fecteau, 2016). Consistent with the recovery of abundant sequences affiliated with photoautotrophs (algae and cyanobacteria), carbon uptake rates for all sites exhibited a strong dependence on light, with average carbon uptake rates for the light treatments ranging from 193.8 to 9.1 μg C/g biomass C/h. Carbon uptake rates in mescocoms performed in the dark ranged from 2.9 to 0.7 μg C/g biomass C/h (Figure 2, middle row, Table 1). Positive carbon uptake rates in dark treatments indicates chemoautotrophic uptake of carbon, consistent with previous work (Schuler et al., 2017). Mean carbon uptake rates measured for sub-sinter, subaerial, and subaqueous photosynthetic communities were indistinguishable (Table 1). Assuming the results from the dark incubations represent chemoautotrophic carbon uptake, we estimated oxygenic photosynthesis uptake rates (light—dark, Table 1) and calculated O2 production rates based on the reaction CO2 + H2O ≥ CH2O + O2. Previous work quantifying oxygenic and anoxygenic photoautotrophic as well as chemoautotrophic carbon uptake in hot spring biofilms has shown that oxygenic photosynthesis is the dominant carbon assimilation mechanism in phototrophic communities, with anoxygenic photoautotrophy contributing a small fraction (≤10.4 %) of total carbon uptake (Schuler et al., 2017). O2 production rates for the sub-sinter samples (3.0–0.6 μmol O2/g biomass C/h) overlapped with values calculated for subaqueous communities and subaerial communities (Table 1). Spectral analyses of loose sinter from alkaline and acidic sites reveal transmitted light that is skewed toward longer wavelengths, providing support for the assumption that sinter provides protection from UV radiation (Supplementary Figure 4). Loose siliceous sinter from alkaline sites allowed deeper light penetration compared to sinter from acidic sites (Supplementary Figure 4). Carbon uptake rates and O2 production values from this study are consistent with other reports from a range of phototrophic microbial ecosystems including hot spring biofilms (Schuler et al., 2017), benthic microbial communities in an acidic lake (Kleeberg et al., 2006), desert crusts (Garcia-Pichel and Belnap, 1996), sandstone hosted endolithic communities (Büdel et al., 2004), microbial mats in Antarctic streams (Vincent and Howarrd-Williams, 1986), and snow algae communities in supraglacial and periglacial terrains (Hamilton and Havig, 2017; Havig and Hamilton, 2019).
Collectively, these results suggest sub-sinter photosynthetic microbial communities can be as productive as subaqueous and subaerial counterparts. However, the amount of surface area in hydrothermal areas covered by siliceous sinter far exceeds that of subaqueous and subaerial surface area—for example, Norris Geyser Basin in YNP includes ~2.4 km2 of hydrothermally altered terrain. As a result, hypolithic (sub-sinter) photosynthetic microbial communities could be overlooked dominant primary producers in hydrothermal areas, both today and throughout Earth's history. Furthermore, sinter deposits provide a niche capable of supporting photosynthetic microorganisms long after the hydrothermal activity has ended (Norris and Castenholz, 2006), providing a potential long-lived source of O2 compared to hydrothermal springs and pools.
A Potential Role for Hypolithic Photosynthetic Communities in Archean Oxidative Weathering
Regions of active or past hydrothermal activity provide ideal locations for oxygenic phototrophs to inhabit, protected from UV radiation by the overlying sinter which also acts as mulch, trapping moisture underneath, and stabilizing the surface from erosion. To illustrate the potential interaction of hypolithic oxygenic photosynthetic communities with pyrite-ladened streams during the Archean, we present a conceptual model based on the following assumptions (Figure 3): (1) Archean rainfall rates were at least as high as modern rates providing a ready influx of runoff charged with locally-sourced dissolved O2 from oxygenic phototrophs; (2) transport of dissolved oxygen into the underlying sediments/rock (a signal of oxidation) could have been preserved in the rock record; (3) Archean streams and rivers were replete with detrital pyrite grains which collected as placer deposits (Krapez, 1985). The delivery of even small amounts of dissolved oxygen to streams and rivers could have fueled microbial Fe oxidation, driving oxidation of detrital pyrite. Today, pyrite-rich acid mine drainage systems are host to Fe oxidizing microorganisms that tolerate a wide range of pH values (from ~2 to 7), gain energy from oxidizing Fe2+ and pyrite, and can fix nitrogen and carbon (e.g., Weber et al., 2006; Johnson D. B. et al., 2014; Havig et al., 2017a); thus a source of dissolved O2 is their only additional requirement. The oxidation of Fe2+ and pyrite during the Archean would have generated sulfuric acid, driving weathering of any rocks present, especially dissolution of carbonate rocks (e.g., calcite, dolomite, siderite) (Reinhard et al., 2009; Stüeken et al., 2012). Oxidation, weathering, and dissolution would enhance delivery of cations, bicarbonate, sulfate, phosphorous, dissolved organic carbon, and fixed nitrogen to the oceans (e.g., Konhauser, 2009; Havig et al., 2017a). The delivery of these nutrients to coastal margins could have driven productivity by sulfate reducers, generating sulfide in turn and potentially stimulating anoxygenic phototrophs. Indeed, evidence exists for productive continental shelfs and coastal margins throughout early Earth history (Lyons et al., 2014).
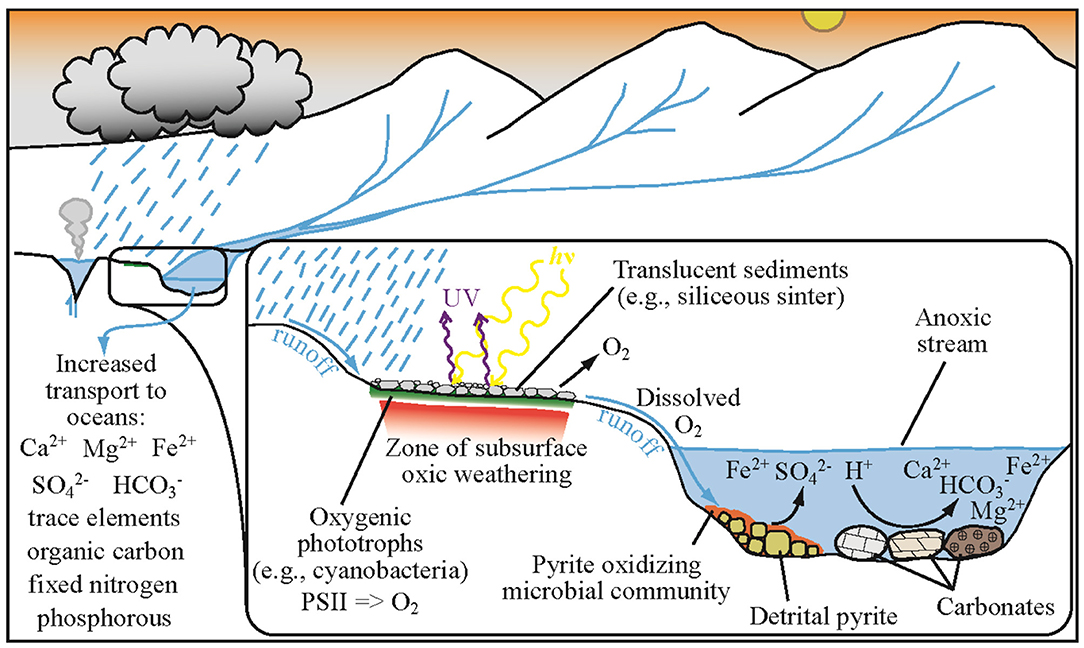
Figure 3. Conceptual cartoon showing the proposed relationship between hydrothermal sub-sinter phototrophic communities and local pyrite oxidation on a Neoarchean terrestrial surface.
The last ~500 million years of the Archean remains a mystery: How to drive oxidative weathering of Archean continental surfaces prior to the Great Oxidation Event? Current dogma has supported an ocean-dominant view for oxygen production, but an increasing body of work suggests terrestrial colonization and oxygen production by microbial communities may have played a large role (e.g., Lalonde and Konhauser, 2015; Sumner et al., 2015; Havig et al., 2017a; Lenton and Daines, 2017). New evidence for silica-depositing terrestrial hot springs at 3.5 Ga highlights a niche for terrestrial productivity (Djokic et al., 2017). In this context, it is our opinion that hypolithic phototrophic microbial communities in hydrothermal areas such as YNP provide a possible example for a source for locally-produced oxygen to drive pyrite oxidation. This study demonstrates that phototrophic sub-sinter microbial communities are predominantly made up of bacterial (and algal) oxygenic phototrophs that demonstrate carbon uptake rates (a proxy for productivity and oxygen production rates) similar to phototrophic communities found in proximal hot springs. We suggest a hypothesis that hypolithic oxygenic phototrophs colonized terrestrial surfaces in locations with protective silica/quartz gravels (best represented by hot spring/proximal hydrothermal areas today, Supplementary Figure 3), with some then developing UV-protective pigments to allow subaerial growth as surface biofilms. These hypolithic communities would, however, be cryptic oases in the rock record given the low likelihood for preservation in the rock record, with their presence primarily inferred from their effects on geochemical proxies, though the reinterpretation of silica deposits in the 3.5 Ga Dresser Fm. as being terrestrial (Djokic et al., 2017) may provide evidence for the presence of these communities early in the rock record.
Methods
Sample Collection and In situ Incubations
Samples collected for molecular analysis as described (Havig et al., 2017a). DNA extraction was performed as described (Havig et al., 2017a). pH, conductivity, and temperature were determined in situ as described (Havig et al., 2017a). Carbon uptake in situ incubations were performed as described (Hamilton and Havig, 2017).
Analyses
16S and 18S rRNA amplicons were sequenced using MiSeq Illumina 2 × 300 bp chemistry with the primers 515Ff and 806rB and E572F and E1009, respectively. Analyses were conducted following the methods as described (Hamilton and Havig, 2017). Biomass from natural abundance and in situ incubations was processed and analyzed via elemental analyzer isotope ratio mass spectrometry as described (Havig et al., 2017a). Spectral analyses were carried out via a BiTec Sensor Luxmeter (Gigahertz-Optik, Türkenfeld, Germany). Acidic sinter was embedded in resin, cut into thin sections, and imaged via scanning electron microscopy coupled to an energy dispersive X-ray spectrometer.
Author Contributions
JH co-designed the project, collected, and processed samples, conducted in situ carbon uptake experiments, analyzed samples for stable C isotopic abundances, interpreted geochemical data, wrote and edited the manuscript, and generated figures. TH co-designed the project, collected and processed molecular samples, collected and processed spectral data, conducted in situ carbon uptake experiments, processed molecular data, edited the manuscript, generated figures, and provided funding for the project.
Conflict of Interest Statement
The authors declare that the research was conducted in the absence of any commercial or financial relationships that could be construed as a potential conflict of interest.
Acknowledgments
We thank E. Oberg, A. Carlson, and the Yellowstone Permit Office for their assistance; E. Herndon for use of scanning electron microscopy-energy dispersive X-ray spectrometer; A. Czaja, A. Gangidine, and A. Gangidine for assistance in the field; A. Diefendorf for assistance with elemental analyzer isotope ratio mass spectrometry analysis; University of Cincinnati Department of Geology for support of JH; A. Gangidine for assistance in making thin sections; and C. Motley for assistance with processing samples.
Supplementary Material
The Supplementary Material for this article can be found online at: https://www.frontiersin.org/articles/10.3389/feart.2019.00015/full#supplementary-material
References
Aigner, S., Remias, D., Karsten, U., and Holzinger, A. (2013). Unusual phenolic compounds contribute to ecophysiological performance in the purple-colored green alga Zygogonium ericetorum (Zygnematophyceae, Streptophyta) from a high-alpine habitat. J. Phycol. 49, 648–660. doi: 10.1111/jpy.12075
Anbar, A. D., Duan, Y., Lyons, T. W., Arnold, G. L., Kendall, B., Creaser, R. A., et al. (2007). A whiff of oxygen before the great oxidation event? Science 317, 1903–1906. doi: 10.1126/science.1140325
Boyd, E. S., Fecteau, K. M., Havig, J. R., Shock, E. L., and Peters, J. W. (2012). Modeling the habitat range of phototrophs in Yellowstone National Park: toward the development of a comprehensive fitness landscape. Front. Microbiol. 3:221. doi: 10.3389/fmicb.2012.00221
Brock, T. D. (1967). Life at high temperatures. Science 158, 1012–1019. doi: 10.1126/science.158.3804.1012
Büdel, B., Weber, B., Kühl, M., Pfanz, H., Sültemeyer, D., and Wessels, D. (2004). Reshaping of sandstone surfaces by cryptoendolithic cyanobacteria: bioalkalization causes chemical weathering in arid landscapes. Gebiology 2, 261–268. doi: 10.1111/j.1472-4677.2004.00040.x
Catling, D. C., and Claire, M. W. (2005). How Earth's atmosphere evolved to an oxic state: a status report. Earth Planet Sci. Let. 237, 1–20. doi: 10.1016/j.epsl.2005.06.013
Cnossen, I., Sanz-Forcada, J., Favata, F., Witasse, O., Zegers, T., and Arnold, N. F. (2007). Habitat of early life: solar X-ray and UV radiation at Earth's surface 4-3.5 billion years ago. J. Geophys. Res. Planets 112:E02008. doi: 10.1029/2006JE002784
Collerson, K. D., and Kamber, B. S. (1999). Evolution of the continents and the atmosphere inferred from Th-U-Nb systematics of the depleted mantle. Science 283, 1519–1522. doi: 10.1126/science.283.5407.1519
Cox, A., Shock, E. L., and Havig, J. R. (2011). The transition to microbial photosynthesis in hot spring ecosystems. Chem. Geol. 280, 344–351. doi: 10.1016/j.chemgeo.2010.11.022
Crowe, S. A., Døssing, L. N., Beukes, N. J., Bau, M., Kruger, S. J., Frei, R., et al. (2013). Atmospheric oxygenation three billion years ago. Nature 501, 535–538. doi: 10.1038/nature12426
Deamer, D. W., and Georgiou, C. D. (2015). Hydrothermal conditions and the origin of cellular life. Astrobiology 15, 1091–1095. doi: 10.1089/ast.2015.1338
Djokic, T., van Kranendonk, M. J., Campbell, K. A., Walter, M. R., and Ward, C. R. (2017). Earliest signs of life on land preserved in ca. 3.5 Ga hot spring deposits. Nat. Commun. 8:15263. doi: 10.1038/ncomms15263
Dodd, M. S., Papineau, D., Grenne, T., Slack, J. F., Rittner, M., Pirajno, F., et al. (2017). Evidence for early life in Earth's oldest hydrothermal vent precipitates. Nature 543, 60–64. doi: 10.1038/nature21377
Edwards, K. J., Bond, P. L., Druschel, G. K., McGuire, M. M., Hamers, R. J., and Banfield, J. F. (2000). Geochemical and biological aspects of sulfide mineral dissolution: lessons from Iron Mountain, California. Chem. Geol. 169, 383–397. doi: 10.1016/S0009-2541(00)00216-3
Fecteau, K. M. (2016). Organic Carbon in Hydrothermal Systems: From Phototrophy to Aldehyde Transformations. Ph.D. Dissertation, Arizona State University, Tempe, AZ.
Forsythe, J. G., Yu, S. S., Mamajanov, I., Grover, M. A., Krishnamurthy, R., Fernández, F. M., et al. (2015). Ester-mediated amide bond formation driven by wet-dry cycles: a possible path to polypeptides on the prebiotic earth. Ang. Chem. Int. Edn. 54, 9871–9875. doi: 10.1002/anie.201503792
Frei, R., Gaucher, C., Poulton, S. W., and Canfield, D. E. (2009). Fluctuations in Precambrian atmospheric oxygenation recorded by chromium isotopes. Nature 461, 250–253. doi: 10.1038/nature08266
Garcia-Pichel, F., and Belnap, J. (1996). Microenvironments and microscale productivity of cyanobacterial desert crusts. J. Phycol. 32, 774–782. doi: 10.1111/j.0022-3646.1996.00774.x
Gumsley, A. P., Chamberlain, K. R., Bleeker, W., Söderlund, U., Olivier de Kock, M., Larsson, E. R., et al. (2017). Timing and tempo of the great oxidation event. Proc. Natl. Acad. Sci. U.S.A. 114, 1811–1816. doi: 10.1073/pnas.1608824114
Hamilton, T. L., and Havig, J. (2017). Primary productivity of snow algae communities on stratovolcanoes of the Pacific Northwest. Geobiology 15, 280–295. doi: 10.1111/gbi.12219
Hao, J., Sverjensky, D. A., and Hazen, R. M. (2017). Mobility of nutrients and trace metals during weathering in the late Archean. Earth Planet. Sci. Lett. 471, 148–159. doi: 10.1016/j.epsl.2017.05.003
Havig, J. R., Grettenberger, C., and Hamilton, T. L. (2017a). Geochemistry and microbial community composition across a range of acid mine drainage impact and implications for the Neoarchean-Paleoproterozoic transition. JGR Biogeosci. 122, 1404–1422. doi: 10.1002/2016JG003594
Havig, J. R., and Hamilton, T. L. (2019). Snow algae drive productivity and weathering at volcanic rock-hosted glaciers. Geochim. Cosmochim. Acta 247, 220–242. doi: 10.1016/j.gca.2018.12.024
Havig, J. R., Hamilton, T. L., Bachan, A., and Kump, L. R. (2017b). Sulfur and carbon isotopic evidence for metabolic pathway evolution and a four-stepped Earth system progression across the Archean and Paleoproterozoic. Earth Sci. Rev. 174, 1–21. doi: 10.1016/j.earscirev.2017.06.014
Holland, H. D. (2009). Why the atmosphere became oxygenated: a proposal. Geochim.Cosmochim. Acta 73, 5241–5255. doi: 10.1016/j.gca.2009.05.070
Holzinger, A., and Pichrtová, M. (2016). Abiotic stress tolerance of charophyte green algae: new challenges for omics techniques. Front. Plant Sci. 7:678. doi: 10.3389/fpls.2016.00678
Johnson, D. B., Dziurla, M. A., Kolmert, A., and Hallberg, K. B. (2002). The microbiology of acid mine drainage: genesis and biotreatment. South Afr. J. Sci. 98, 249–255.
Johnson, D. B., Hallberg, K. B., and Hedrich, S. (2014). Uncovering a microbial enigma: isolation and characterization of the streamer-generating, iron-oxidizing, acidophilic bacterium “Ferrovum myxofaciens”. Appl. Env. Microbiol. 80, 672–680. doi: 10.1128/AEM.03230-13
Johnson, J. E., Gerpheide, A., Lamb, M. P., and Fischer, W. W. (2014). O2 constraints from Paleoproterozoic detrital pyrite and uraninite. GSA Bull. 126, 813–830. doi: 10.1130/B30949.1
Kleeberg, A., Schubert, H., Koschorreck, M., and Nixdorf, B. (2006). Abundance and primary production of filamentous green algae Zygogonium ericetorum in an extremely acid (pH 2.9) mining lake and its impact on alkalinity generation. Freshw. Biol. 51, 925–937. doi: 10.1111/j.1365-2427.2006.01542.x
Konhauser, K. (2009). Biogeochemistry: deepening the early oxygen debate. Nat. Geosci. 2, 241–242. doi: 10.1038/ngeo484
Konhauser, K. O., Lalonde, S. V., Planavsky, N. J., Pecoits, E., Lyons, T. W., Mojzsis, S. J., et al. (2011). Aerobic bacterial pyrite oxidation and acid rock drainage during the Great Oxidation Event. Nature 478, 369–373. doi: 10.1038/nature10511
Krapez, B. (1985). The Ventersdorp contact placer: a gold-pyrite placer of stream and debris-flow origins from the Archaean Witwatersrand Basin of South Africa. Sedimentology 32, 223–234. doi: 10.1111/j.1365-3091.1985.tb00505.x
Kump, L. R., and Barley, M. E. (2007). Increased subaerial volcanism and the rise of atmospheric oxygen 2.5 billion years ago. Nature 448, 1033–1036. doi: 10.1038/nature06058
Lacap-Bugler, D. C., Lee, K. K., Archer, S., Gillman, L. N., Lau, M. C. Y., Leuzinger, S., et al. (2017). Global diversity of desert hypolithic cyanobacteria. Front. Microbiol. 8:867. doi: 10.3389/fmicb.2017.00867
Lalonde, S. V., and Konhauser, K. O. (2015). Benthic perspective on Earth's oldest evidence for oxygenic photosynthesis. Proc. Natl. Acad. Sci. U.S.A. 112, 995–1000. doi: 10.1073/pnas.1415718112
Lenton, T. M., and Daines, S. J. (2017). Matworld–the biogeochemical effects of early life on land. N. Phytol. 215, 531–537. doi: 10.1111/nph.14338
Lyons, T. W., Reinhard, C. T., and Planavsky, N. J. (2014). The rise of oxygen in Earth's early ocean and atmosphere. Nature 506, 307–315. doi: 10.1038/nature13068
Milshteyn, D., Damer, B., Havig, J., and Deamer, D. (2018). Amphiphilic compounds assemble into membranous vesicles in hydrothermal hot spring water but not in seawater. Life 8:E11. doi: 10.3390/life8020011
Norris, T. B., and Castenholz, R. W. (2006). Endolithic photosynthetic communities within ancient and recent travertine deposits in Yellowstone National Park. FEMS Microbiol. Ecol. 57, 470–483. doi: 10.1111/j.1574-6941.2006.00134.x
Olson, S. L., Kump, L. R., and Kasting, J. F. (2013). Quantifying the areal extent and dissolved oxygen concentrations of Archean oxygen oases. Chem. Geol. 362, 35–43. doi: 10.1016/j.chemgeo.2013.08.012
Pavlov, A. A., Brown, L. L., and Kasting, J. F. (2001). UV shielding of NH3 and O2 by organic hazes in the Archean atmosphere. JGR Planets 106, 23267–23287. doi: 10.1029/2000JE001448
Planavsky, N. J., Asael, D., Hofmann, A., Reinhard, C. T., Lalonde, S. V., Knudsen, A., et al. (2014). Evidence for oxygenic photosynthesis half a billion years before the great oxidation event. Nat. Geosci. 7, 283–286. doi: 10.1038/ngeo2122
Ratner, M. I., and Walker, J. C. G. (1972). Atmospheric ozone and the history of life. J. Atmospheric Sci. 29, 803–808. doi: 10.1175/1520-0469(1972)029<0803:AOATHO>2.0.CO;2
Reinhard, C. T., Lalonde, S. V., and Lyons, T. W. (2013). Oxidative sulfide dissolution on the early Earth. Chem. Geol. 362, 44–55. doi: 10.1016/j.chemgeo.2013.10.006
Reinhard, C. T., Raiswell, R., Scott, C., Anbar, A. D., and Lyons, T. W. (2009). A late Archean sulfidic sea stimulated by early oxidative weathering of the continents. Science 326, 713–716. doi: 10.1126/science.1176711
Schuler, C. G., Havig, J. R., and Hamilton, T. L. (2017). Hot spring microbial community composition, morphology, and carbon fixation: implications for interpreting the ancient rock record. Front. Earth Sci. 5:97. doi: 10.3389/feart.2017.00097
Seager, S., and Deming, D. (2010). Exoplanet atmospheres. An. Rev. Astron. Astrophys. 48, 631–672. doi: 10.1146/annurev-astro-081309-130837
Steinberg, C. E. W., Schäfer, H., and Beisker, W. (1998). Do acid-tolerant cyanobacteria exist? Clean Soil Air Water 26, 13–19.
Stüeken, E. E., Catling, D. C., and Buick, R. (2012). Contributions to late Archaean sulphur cycling by life on land. Nat. Geosci. 5, 722–725. doi: 10.1038/ngeo1585
Sumner, D. Y., Hawes, I., Mackey, T. J., Jungblut, A. D., and Doran, P. T. (2015). Antarctic microbial mats: a modern analog for Archean lacustrine oxygen oases. Geology 43, 887–890. doi: 10.1130/G36966.1
Taylor, S. R., and McLennan, S. M. (1995). The geochemical evolution of the continental crust. Rev. Geophys. 33, 241–265. doi: 10.1029/95RG00262
Van Kranendonk, M. J., Deamer, D. W., and Djokic, T. (2017). Life springs. Sci. Amer. 317, 28–35. doi: 10.1038/scientificamerican0817-28
Vincent, W., and Howarrd-Williams, C. (1986). Antarctic stream ecosystems: physiological ecology of a blue-green algal epilithon. Freshw. Biol. 16, 219–233. doi: 10.1111/j.1365-2427.1986.tb00966.x
Walker, J. J., Spear, J. R., and Pace, N. R. (2005). Geobiology of a microbial endolithic community in the Yellowstone geothermal environment. Nature 434, 1011–1014. doi: 10.1038/nature03447
Weber, K. A., Achenbach, L. A., and Coates, J. D. (2006). Microorganisms pumping iron: anaerobic microbial iron oxidation and reduction. Nat. Rev. Microbiol. 4, 752–764. doi: 10.1038/nrmicro1490
Wille, M., Kramers, J. D., Nagler, T. F., Johannes Beukes, N., Schröder, S., Meisel, T. C., et al. (2007). Evidence for a gradual rise of oxygen between 2.6 and 2.5 Ga from Mo isotopes and Re-PGE signatures in shales. Geochim. Cosmochim. Acta 71, 2417–2435. doi: 10.1016/j.gca.2007.02.019
Zahnle, K. J., Catling, D. C., and Claire, M. W. (2013). The rise of oxygen and the hydrogen hourglass. Chem. Geol. 362, 26–34. doi: 10.1016/j.chemgeo.2013.08.004
Keywords: oxygen oases, hydrothermal systems, Archean oxidation, hypolithic autotrophs, oxygenic phototrophs
Citation: Havig JR and Hamilton TL (2019) Hypolithic Photosynthesis in Hydrothermal Areas and Implications for Cryptic Oxygen Oases on Archean Continental Surfaces. Front. Earth Sci. 7:15. doi: 10.3389/feart.2019.00015
Received: 08 September 2017; Accepted: 28 January 2019;
Published: 20 February 2019.
Edited by:
Gilad Antler, Ben-Gurion University of the Negev, IsraelReviewed by:
Stefan Lalonde, Université de Bretagne Occidentale, FranceAlexandra V. Turchyn, University of Cambridge, United Kingdom
Copyright © 2019 Havig and Hamilton. This is an open-access article distributed under the terms of the Creative Commons Attribution License (CC BY). The use, distribution or reproduction in other forums is permitted, provided the original author(s) and the copyright owner(s) are credited and that the original publication in this journal is cited, in accordance with accepted academic practice. No use, distribution or reproduction is permitted which does not comply with these terms.
*Correspondence: Jeff R. Havig, amhhdmlnQHVtbi5lZHU=; amVmZmhhdmlnQGdtYWlsLmNvbQ==