- 1School of Physics, University College Dublin, Dublin, Ireland
- 2Institute for Discovery, University College Dublin, Dublin, Ireland
- 3ENT-Department, Section of Experimental Oncology und Nanomedicine (SEON), Universitätsklinikum Erlangen, Erlangen, Germany
- 4Thermal Hydraulics and Multiphase Flow Laboratory, Institute of Nuclear & Radiology Sciences and Technology, Energy & Safety, National Centre for Scientific Research “Demokritos”, Athens, Greece
A rational design of drug nanocarriers supported by in silico modelling tools can improve the efficacy of nanosystem-based intravascular drug delivery (IVDD). Computational model development stems from the vision of replacing conventional (pre)clinical trials with advanced simulations and applies to the development of more efficient nanocarriers for intravascular therapies. To establish a standardized framework for in silico preclinical trials, it is necessary to include in silico tools that can model each experimental stage of a preclinical trial for a respective nanocarrier system and give accurate and verifiable results. This review paper highlights the status of intravascular drug delivery supported by nanocarriers and discusses the modelling stages of a physics-based multiscale modelling framework that should be developed, validated and exploited to address the need for an effective preclinical assessment of nanocarriers for IVDD.
1 Introduction to nanoparticle-based drug delivery
Nanoparticles represent an innovative platform, that can be adapted to serve both therapy and the diagnosis of various human diseases. Although multiple delivery routes are possible, the majority of the clinically-relevant nanocarriers, such as anti-cancer and anti-inflammatory nanoparticulate drugs, require intravascular administration. However, the first-generation injectable drug carriers have often been plagued by suboptimal efficacy as a result of rapid clearance by the reticuloendothelial system (RES) (Alexis et al., 2008), or of an inability to reach the target tissue in effective dosage (Chen et al., 2006; Kennel et al., 2008; Moros et al., 2013). Consequently, a rational design of drug carriers supported by modeling tools is expected to improve the efficacy of nanodrugs, by enabling the delivery of a sufficient payload of therapeutics to the diseased regions, limiting their cyto- and genotoxicity, and preventing their unspecific clearance by the RES.
1.1 Nanoparticle types
Nanocapsules or nanoparticles composed of different natural or synthetic materials are gaining attention as potential intravascular drug delivery (IVDD) systems. The commonly reported drug-carrier nanosystems have been the topic of multiple reviews in the previous years (Mitchell et al., 2021; Joudeh and Linke, 2022) and their basic characteristics are briefly outlined below.
1.1.1 Lipid and polymer-based nanoparticles
Liposomes consist of a lipid bilayer containing amphipathic phospholipids (primarily phosphatidylcholine) that enclose an interior aqueous space (Puri et al., 2009). As they are commonly synthesized from natural phospholipids, their toxicity is relatively low (Huwyler et al., 2008; Puri et al., 2009; Balazs and Godbey, 2011; Muller et al., 2011), but there are little data concerning the effects of increased shear forces on their integrity and they are easily cleared from the circulation by RES. To improve the stability and circulation time of liposomes, the head groups of phospholipids can be conjugated with polyethylene glycol (PEG), resulting in so-called stealth liposomes (Almer et al., 2013). Functionalization with maleimide as the PEG end-group allows conjugation of antibodies or other targeting ligands on their surface (van der Meel et al., 2013).
Lipid-core nanoparticles (LCNPs) are composed of a lipid kernel stabilized by a single layer of surfactant shell, which commonly contains a mixture of phospholipids, PEGylated surfactants, or amphiphilic saccharides (Gravier et al., 2011; Devel et al., 2019). Based on the state of their lipidic core components, LCNPs are classified in three categories: liquid lipid NPs (including lipid nanoemulsions and lipid nanocapsules), solid lipid NPs, and nanostructured lipid nanocarriers (Graván et al., 2023). Their size is easily adjustable and lipid composition is similar to those of low-density lipoproteins, enabling prolonged blood circulation and accumulation in atherosclerotic lesions (Devel et al., 2019).
Polymer nanoparticles are composed of synthetic or natural polymers, most commonly poly (lactic-co-glycolic acid) (PLGA), poly (lactic acid), poly (caprolactone), poly (alkylcyanoacrylates), or chitosan (Lai et al., 2014). The polymer core of the nanoparticles can be covalently cross-linked with the coating, forming a biocompatible hydrophilic shell. Dextrans, stable glucose polymers, that contain functional groups for derivatization (Sun and Mao, 2012), are commonly used to improve colloidal stability and biocompatibility of such particles. Their tunable surface properties enable easy grafting of functional groups for conjugation of drugs or targeting ligands (Bachelet et al., 2009; Rouzet et al., 2011).
1.1.2 Inorganic nanoparticle systems
Gold nanoparticles are composed of a dielectric core of silica coated with a metallic layer of gold, or consist of gold core encircled by an organic monolayer, and are tunable to various sizes and forms (Menon et al., 2013). Owing to their optical properties, these particles can be used for e.g., for optical imaging, or photothermal ablation therapy, as well as drug carriers (Lv et al., 2022). However, the important concern related to gold particles is their potential cytotoxicity and a slow elimination resulting in a long-term persistence within many organs (Xia et al., 2016).
Silver nanoparticles usually consist of silver cores stabilized with silica or sodium citrate and their properties range from broad-spectrum antimicrobial efficacy against bacteria, fungi and viruses (Hancharova et al., 2024) to photosensitizing effects (Singh et al., 2024). Consequently, these particles are tested in multiple in vivo applications including wound care, imaging, cancer therapy or tissue engineering, but their long-term safety and biocompatibility remain to be studied.
Superparamagnetic iron oxide nanoparticles (SPIONs) consist of iron oxide core and a shell that is usually composed of organic materials such as fatty acids, polysaccharides, or polymers, aiming to ensure their colloidal stability, biocompatibility and functionalization with drugs or targeting moieties (Tassa et al., 2011; Wahajuddin and Arora, 2012). Owing to their magnetic properties, SPIONs are used as intravascular contrast agents for magnetic resonance imaging (Tang et al., 2009; Sadat et al., 2013; Liu et al., 2014), or for hyperthermia-therapy of cancer (Hayashi et al., 2013).
Quantum dots (QDs) - nanoscale semiconductor crystals with sizes 2–10 nm - are most commonly composed of binary compounds containing elements such as Cd, Pb, Hg, In, Se (Ji et al., 2014). The fluorescence bands of QDs are dependent on their composition, size and shell thickness (Marukhyan and Gasparyan, 2017). Besides serving as imaging tools, QDs have great potential as theranostics platforms for simultaneous imaging and therapy.
Carbon-based nanomaterials characterized by exceptional mechanical, electrical, and thermal properties include fullerenes, graphene quantum dots (GQDs), graphene oxide (GO) and carbon nanotubes (CNT), which were recently reviewed in Malode et al. (2024). Their potential biomedical applications include, among others, biosensing, hyperthermia, drug delivery and tissue engineering (AbouAitah et al., 2023; Sharma et al., 2024), as well as improving the electric conductivity of cardiac patches (Ren et al., 2017).
The above-listed nanomaterials can be combined in order to tailor the characteristics of the final nanosystem, e.g., biocompatibility, colloidal stability, specific charge, size or imaging properties. Given adequate safety and effective drug delivery, such hybrid nanosystems with adjustable characteristics could improve the future therapeutic success of intravascular drug carriers.
1.2 Nanoparticle loading with drugs
The first FDA-approved nanodrug was a PEGylated liposomal formulation of doxorubicin (Doxil®), indicated for the treatment of several types of cancer [reviewed in Barenholz (2012)]. Following this, multiple research groups focused on the development of nanocarriers for drug delivery. Several of the reported carriers and their cargo are briefly highlighted below.
Chemotherapeutics, including doxorubicin, are commonly used in the development of nanosystems aiming at intravascular drug delivery to cancer. Research is ongoing on further improvement of doxorubicin-loaded liposomal nanocapsules alone (Song P. P. et al., 2022; Shim et al., 2022), or in combination with other anti-cancer drugs (Gabizon et al., 2021). Also, liposomal paclitaxel has already entered clinical practice (Zhang W. W. et al., 2022). The development of PEGylated liposomes loaded with oxaliplatin and paclitaxel has been recently reported (Xie et al., 2023) as well as liposomes containing a co-load of hesperetin and cisplatin (Wang X. et al., 2023). Lipid-based nanoparticles can also serve as carriers of biologics, such as nucleic acids (Gilbert et al., 2024). In cancer immunotherapy, ionizable LNPs were developed as carriers of mRNA (Billingsley et al., 2023) or plasmid DNA (Prazeres et al., 2023) to induce chimeric antigen receptor (CAR) expression in T cells.
Polymer nanoparticles as carriers of several anti-cancer agents, including doxorubicin and paclitaxel have been reported (Danhier et al., 2012; Geary and Salem, 2014; Zhang D. et al., 2022; Almoustafa et al., 2023; Miraghaie et al., 2023). Recently, a combination chemo-immunotherapy for cancer using lipid polymer nanocomplexes based on PLGA and 1, 2-distearoyl-sn-glycero-3-phosphoethanolamine (DSPE)-PEG was also proposed. The developed nanosytem served as a carrier of doxorubicin, and as a checkpoint inhibiting peptide against programmed death-ligand 1 (PD-L1) (Zhang N. et al., 2022).
Gold nanoparticles have been utilized for experimental delivery of anti-cancer drugs, such as gemcitabine (Kudgus et al., 2013) doxorubicin (Zhang et al., 2015; Morshed et al., 2016), as well as cisplatin (Quinones et al., 2023), [reviewed in Moreno-Alcantar et al. (2023)]. Cisplatin delivery system based on silver nanoparticles coated with an alginate hydrogel shell was also reported (Maher et al., 2023). Cai et al. reported the development of ultrasmall pH-responsive ZnO QDs functionalized with PEG that were used as carriers of doxorubicin (Cai et al., 2016). QDs were also loaded with small interfering RNA targeting Bcl-2 and different anticancer drugs (carboplatin, paclitaxel, and doxorubicin) for combined therapy of lung cancer (Li et al., 2016).
Similarly, many research groups reported SPION-based nanosysems for drug delivery of chemotherapeutic agents, including doxorubicin (Hernandes et al., 2023; Markhulia et al., 2023), cisplatin (Unterweger et al., 2014; Hernandez et al., 2023), mitoxantrone (Tietze et al., 2013), among others [reviewed in Vangijzegem et al. (2023)]. Dual drug-loading approach was also reported using SPIONs functionalized with anti-angiogenic peptide and loaded with paclitaxel (Ngema et al., 2023).
Further, theranostic nanosystems composed of two or more types of materials have been developed, combining magnetic particles with polymers (Amiryaghoubi et al., 2022) or liposomes (Shetake et al., 2022) for delivery of doxorubicin to cancer. An interesting approach to metastatic breast cancers was recently reported by Hasannia et al., who developed nanovessicles based on the poly (L-glutamic acid) and termed peptosomes, that were loaded with magnetic particles and doxorubicin (Hasannia et al., 2023).
However, compared to the field of oncology, fewer research groups pursue the development of nanosystems for cardiovascular drug delivery. Interestingly, nearly all particle types mentioned above have been employed as experimental drug carriers for various cardiovascular disorders [see a recent review by Luo et al. (2023)]. Some of the main recent examples are highlighted below.
Liposomes have been used as carriers of anti-atherosclerotic drugs including statins (Li et al., 2019; Wu et al., 2021), PCSK9 inhibitor evolocumab (Li et al., 2023), or docosahexaenoic acid (Chong et al., 2023), as well as anti-inflammatory agents such as prednisolone (van der Valk et al., 2015), methotrexate (Di Francesco et al., 2020), or micro RNA (miR-146a) (Ho et al., 2023). Delivery of nucleic acids has furthermore been employed to reduce plasma cholesterol levels using liver-specific ionizable LNPs loaded with small interfering RNA silencing Pcsk9 gene (Huang et al., 2023) and to prevent cardiac fibrosis in heart failure using LNPs carrying modified mRNA encoding the antifibrotic CAR to T lymphocytes (Rurik et al., 2022). In general, lipid-based nanosystems, including liposomes, solid lipid particles as well as reconstituted high-density lipoprotein (HDL) nanoparticles display increased affinity for macrophages, or dendritric cells (Courant et al., 2017; Kornmueller et al., 2019), and accumulate in atherosclerotic plaques, which translates in improved efficacy versus free drugs (Wang et al., 2018).
Polymer particles have been frequently used as nanocarriers for the treatment of cardiovascular diseases (Mura and Couvreur, 2012; Nenna et al., 2021). In particular nanosystems based on PLGA, the most common biodegradable polymer approved for use in humans, have been tested in animal models as drug carriers for tissue plasminogen activator (Korin et al., 2012), streptokinase (Hasanpour et al., 2021), superoxide dismutase (Yun et al., 2013), bevacizumab (De Negri Atanasio et al., 2022), pioglitazone (Nakashiro et al., 2016; Todaro et al., 2022; Groner et al., 2023), senolytic drug ABT263 (Lee et al., 2021), as well as combined gene delivery of VEGF and NGF (Chen Y. et al., 2022) and microRNAs that effectively regulate Nrf2 against myocardial ischemia-reperfusion injury (Wang et al., 2022).
Polymer nanocarriers are also very commonly used for delivery of statins (reviewed in (Montelione et al., 2023), including simvastatin (Alaarg et al., 2017), pitavastatin (Nagaoka et al., 2015; Katsuki et al., 2022), or atorvastatin (Liu et al., 2023). The latter study compared the drug loading and efficacy of polymeric (PLGA and poly-vinyl alcohol) carries with PLGS-lipid hybrid particles. PLGA-based nanosystem was furthermore used to develop reactive oxygen species (ROS)-sensitive complexes for co-delivery of siRNA against vascular cell adhesion molecule-1 (VCAM-1) and dexamethasone in a rat model of myocardial ischemia-reperfusion (Hou et al., 2022).
Compared to polymer or lipid-based nanosystems, metal-based nanoparticles have more commonly been used as imaging agents rather than drug carriers. However, their theranostic potential is increasingly getting acknowledged and the numbers of reports describing new developments of metal-based carriers is growing (Younis et al., 2021). SPIONs have been approved as clinical contrast agents for MRI (Sadat et al., 2013; Florian et al., 2014; Nguyen et al., 2019; Zheng et al., 2019), but are less often used for cardiovascular drug delivery. Of note, SPION-based nanosystems have been developed by different groups as a platform for delivery of thrombolytic drugs (e.g., tPA, streptokinase), either alone (Ma et al., 2009; Tadayon et al., 2015), or in combination with PLGA (Chen et al., 2020) or PLGA and chitosan (Zhou et al., 2014). The delivery of dexamethasone-loaded SPIONs to atherosclerotic plaques (Matuszak et al., 2018) and ischemic brain regions (Lu et al., 2021) was also reported. The initial in vitro evaluation of clinically approved SPION formulation (ferumoxytol) functionalized with vascular endothelial growth factor (VEGF) for potential future use in patients with myocardial infarction showed their positive effect on cell survival and growth (Bietenbeck et al., 2019).
Another type of nanosystem relatively rarely used for cardiovascular drug delivery is based on gold nanoparticles. Clinically approved inotropic agent levosimendan loaded on gold nanoparticles was previously tested in a rat model of heart failure (Spivak et al., 2013). Gold nanoparticles in combination with highly branched poly (amide amine) (PAMAM) dendrimers were also used as carriers of gastrodin, a natural neuroprotective phenolic glycoside, in a model of cerebral ischemia-reperfusion injury (Huang et al., 2022). For thrombotic disorders, mesoporous silica-coated gold nanorods conjugated with near-infrared fluorescent dye have been developed as theranostic carriers of urokinase-type plasminogen activator and algae-derived P-selectin specific anticoagulant fucoidan (Chang et al., 2021).
Similarly, graphene quantum dot-based drug delivery system to atherosclerosis has been developed only recently. Loaded with microRNA223 linked by disulfide bonds, it has been grafted onto monocyte membranes to ensure delivery on the cells transmigrating to the interior of the plaque in ApoE-deficient mice (Liu F. et al., 2019).
1.3 Nanoparticle targeting and interactions with target tissues/cells
The parenterally-administered nanosystems for drug delivery should be able to achieve an increased circulation half-life to avoid scavenging by RES (Haroon et al., 2022), and a high margination rate in bloodstream to allow enhanced interactions with endothelial cells in the target region (Decuzzi et al., 2009).
Passive targeting can be used to deliver nanotherapeutics to the diseased regions, but its efficacy is limited to the microvascular beds where the blood-tissue barrier is compromised, e.g., due to cancer or inflammation (Claesson-Welsh et al., 2021). The hypoxic center of the growing tumor induces angiogenic effect on the surrounding vasculature leading to neovascularization. Due to the conditions in tumor microenvironment, including high interstitial pressure and lack of proper cellular and connective tissue support of the forming vessels, the tumor vasculature is malformed, immature and leaky (Nakamura et al., 2016). The increased permeability of tumor microvessels enables entry of the macromolecules and nanoparticles into the tumor tissue. At the same time, the stromal pressure prevents the formation of functional lymphatic vessels draining the tumor, thus enhancing the retention of molecules and particles in the tumor tissue. The enhanced permeability and retention (EPR) effect can therefore improve the accumulation of chemotherapeutic drugs via compromised endothelial barrier (Wu, 2021). However, despite of it, only about 1% of circulating nanosystems reach the tumor tissue (Wilhelm et al., 2016). EPR effect is also present in advanced atherosclerotic lesions, as the immature vasa vasorum of those plaques resemble the leaky tumor vessels. Another biological mechanism of passive targeting to atherosclerosis utilizes the increased permeability of luminal endothelium for accumulation of lipid-/or lipoprotein-based particles in the plaques. In this context, especially HDL-mimetic particles can be employed as drug carriers targeting atherosclerotic plaques (Sanchez-Gaytan et al., 2015). However, as passive targeting offers limited efficacy, more specific or active targeting approaches are required to enable an increased accumulation of particles in the diseased tissues or vascular beds. These are realized by means of molecular targeting to a specific peptide or receptor expressed on the target cells, or by magnetic targeting method to remotely control the accumulation of drugs in the diseased region.
1.3.1 Active targeting of cancer
Active targeting of tumor cells is commonly realized by functionalization of nanoparticle surface with specific antibodies or ligands, e.g., hyaluronic acid (HA), a ligand of tumor-associated receptor CD44; folic acid, a ligand of folate receptor; transferrin, a ligand of transferrin receptor; RGD peptide, a ligand of integrins, or antibodies inhibiting epidermal growth factor receptor (EGFR), also known as HER1 (human epidermal receptor 1) [reviewed in Hong et al. (2023)]. Furthermore, the active targeting of paclitaxel using SPIONs functionalized with anti-VEGF peptide, HRH, has recently been reported (Ngema et al., 2023).
1.3.2 Active targeting of cardiovascular disorders
Previous experimental studies in vitro models indicated that accumulation of nanocarriers under flow conditions typical for medium and large human arteries may not be sufficient due to the hemodynamic conditions preventing nanoparticle interactions with cells (Yang et al., 2011; Yang et al., 2013). In vivo, untargeted drug delivery into endothelium strongly depends different physiological hemodynamic patterns (capillaries vs. arterioles/arteries) or pathological conditions (e.g., inflammation). Extensive efforts are therefore focused on identifying targeting approaches that could enhance particle accumulation at the disease-specific regions and binding of nanoparticles to vascular endothelium (Figure 1).
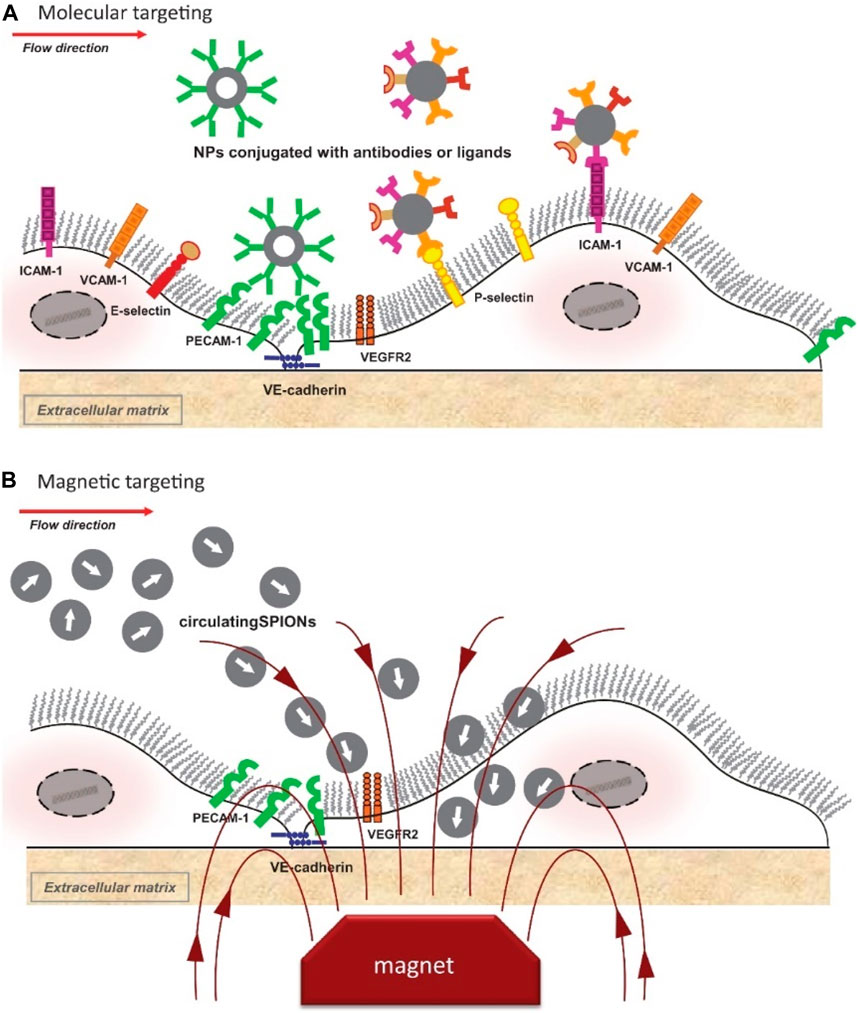
FIGURE 1. Schematic representation of active targeting of endothelial cells with intravascular nanoparticles. (A) Molecular-based-targeting; (B) Magnetic-based targeting. Vascular endothelial cadherin (VE-cadherin), vascular endothelial growth factor receptor 2 (VEGFR2), and other important biomolecular components, including antibodies and ligands, are also illustrated schematically. Reprinted with permission of IOS Press from publication (Cicha, 2016), Copyright 2016, available at IOS Press through: https://doi.org/10.3233/jcb-15020.
1.3.3 Molecular targeting of vascular cells
Endothelial cells: Endothelial activation markers, such as adhesion molecules have been tested as molecular targets in vitro and in vivo already in the 2000s [reviewed in Cicha (2016)]. Ligands or antibodies against VCAM-1; (Yang et al., 2013; Dosta et al., 2021; Reyes-Esteves et al., 2023), intercellular adhesion molecule-1 [ICAM-1; (Bhowmick et al., 2012; Zukerman et al., 2020)], platelet-endothelial cell adhesion molecule-1 [PECAM-1 (Han et al., 2012; de Castro Leao et al., 2021)], as well as E-selectin (Ma et al., 2016; Inyang et al., 2020) were used to functionalize different types of nanoparticles and enhance their internalization by endothelial cells. As an example, functionalization of cationic lipoparticles containing anti-miR-712 with VCAM-1 ligand enabled their specific accumulation in inflamed mouse endothelium and led to effective inhibition atherosclerotic plaque formation (Kheirolomoom et al., 2015). Furthermore, nanoparticles functionalized with Arg-Gly-Asp (RGD) peptide, a ligand of αvβ3 integrin, which is highly expressed on the luminal surface of activated endothelial cells and mediates angiogenesis, have been investigated as carriers for targeted delivery of drugs to activated endothelial cells (Martinez-Jothar et al., 2020; De Negri Atanasio et al., 2022). Another very effective targeting approach involves platelet-mimicry by conjugation of glycoproteins (Kona et al., 2012) or platelet membrane cloaking (Hu et al., 2015), which enables selective binding to endothelial cells under arterial flow conditions. Collectively, these results indicate that molecular targeting can significantly improve endothelial interactions with nanocarriers in vivo and allow enhanced drug delivery even in large vessels under physiologic flow conditions.
Macrophages: Targeting of macrophages in atherosclerotic plaques is commonly realized by functionalization of the nanocarriers’ surface with a ligand of scavenger receptor-AI (Ye M. et al., 2019; Bai et al., 2022; Zhu et al., 2023), or a ligand of class II scavenger receptor, CD36 (Wang Q. et al., 2021), which are highly expressed on in macrophages and foam cells. Furthermore, owing to a high expression of CD44 in inflammatory macrophages, HA is commonly used to target drug-loaded nanocarriers towards atherosclerotic plaque (Song K. et al., 2022; He et al., 2023). Nanoparticles conjugated with antibody against CD11b expressed on monocytes have also been shown to effectively target the ischemic region of the heart and improve outcomes in a mouse model of MI (Li et al., 2022). Further approaches to macrophage targeting in atherosclerosis have been recently reviewed in Chen W. et al. (2022).
Platelets: Markers of activated platelets, such as P-selectin or integrin αIIbβ3, have been commonly used to target drug carriers to thrombotic regions. For this, the surface of nanocarriers has been functionalized with e.g., fucoidan, an algae-derived P-selectin ligand (Chang et al., 2021; Zhang et al., 2021; Wang Y. et al., 2023), or platelet-binding peptide (CGSSSGRGDSPA) that binds to integrin αIIbβ3 (Sun et al., 2020; Sun et al., 2023). Other recently tested approaches enabling targeting of blood clots forming in the vasculature include fibrin targeting with fibrin-binding peptide (cyclo-AC-Y (DGI)C(HPr)YGLCYIQGK-Am) (Sun et al., 2023), or CREKA (Zhao et al., 2020), as well as surface-decoration with vWF-binding peptides (TRYLRIHPQSWVHQI) and collagen-binding peptides (GPO) via conjugation to maleimide-terminated lipid molecules and subsequent incorporation into liposomal nanocarriers (Girish et al., 2022).
1.3.4 Magnetic targeting
In contrast to molecular targeting, which involves functionalization of nanocarriers with specific target-binding molecules, magnetic drug targeting utilizes the intrinsic properties of SPIONs and an external magnetic field to direct and accumulate drug-loaded carriers at the diseased regions (Figure 1). Magnetic targeting has a very high efficacy, but low specificity towards particular cell-types. Due to the specific hemodynamic conditions, this approach is easier to realize in tumor tissues or microvessels, compared to arterial circulation, where magnetic forces must overcome the increased flow rate and pressure.
In cancer therapies, magnetic targeting enables the efficient drug delivery resulting in improved therapeutic outcome [reviewed in Tietze et al. (2015)]. Delivery of doxorubicin in mouse models of hepatoma (Chao et al., 2012), Ehrlich carcinoma (Elbialy et al., 2015), or ovarian cancer (Yu et al., 2015), as well as mitoxantrone in a rabbit tumor model (Tietze et al., 2013), confirmed that active targeting using the magnetic field enhances the specific drug delivery to the tumor vasculature and increases its therapeutic efficacy.
The magnetic properties of SPIONs were also shown to allow magnetic drug targeting in arteries (Chen et al., 2008). Dexamethasone-loaded SPIONs were magnetically targeted to the regions of balloon injury in abdominal aorta and to advanced atherosclerotic plaques in rabbits (Matuszak et al., 2018). In a rat model of myocardial ischemia, magnetic nanobeads carrying VEGF gene were targeted to injured hearts using epicardial magnet (Zhang et al., 2012). Magnetic targeting found a particularly promising application in the treatment of thrombosis, where systemic administration of thrombolytic drugs bears a risk of hemorrhagic complications and local drug delivery is limited by occlusive thrombi. In this context, the delivery of SPIONs loaded with tPA (Ma et al., 2009; Liu C. H. et al., 2019; Wang L. et al., 2021) has a great potential for rapid and localized thrombolysis with minimized side effects.
1.3.5 Unspecific off-target effects and clearance
While the targeted delivery of nanocarrier-bound drugs can enhance their local accumulation in the diseased tissues thus improving therapeutic efficacy and minimizing systemic side effects, the extended circulation time of nanocarriers, as well as multiple degradation products, may result in cytotoxicity (Kumar and Dhawan, 2013), or immunogenicity (Zolnik et al., 2010). Apart from systemic toxicity, the escape of the drug/carrier from the target tissue is expected to reduce its efficacy, as the particle-bound drugs will eventually undergo clearance in liver, kidney, or spleen.
It is therefore important to develop tools supporting the design of drug carriers and their early validation before entering the extensive and costly in vivo experiments. For this, modeling of ligand-receptor, nanocarrier-blood protein, and drug interactions with their target molecules is necessary. Furthermore, advanced tools enabling simulations of nanocarrier behavior in the bloodstream, in dependence of particle composition, size, charge and aspect ratio, as well as differing shear stress magnitudes and durations, are necessary to improve predictability of drug carrier behavior in the circulation.
2 Microscale and mesoscale modelling
Significant developments of computational modeling methods are rapidly bridging the gap between atomistic levels of description of both organic and inorganic molecular systems and multi-supra-molecular structures at the nanoscale, such as those including nanoparticles and other nano-engineered nanomaterials (ENMs) including nanocarriers (Bashiri et al., 2023; Cheng et al., 2023). At atomic levels of description, methods as molecular dynamics (MD) and mixed quantum-classical calculations, such as using quantum mechanical-molecular mechanics (QMMM) methods have seen tremendous developments and recognized with the 2013 Nobel Prize in Chemistry awarded to Karplus, Levitt and Warshel (NobelPrize, 2013). As stated by the associated press release, computational modeling become “.your Virgil in the world of atoms” as “Today the computer is just as important a tool for chemists as the test tube. Simulations are so realistic that they predict the outcome of traditional experiments.” In the same release, it is also notably highlighted the key application of computational modeling to performing “simulations of how a drug couples to its target protein in the body” though the challenge of attaining both accuracy and atomistic detail of physico-chemical description remains (NobelPrize, 2013). Thus, the last decade has seen rapid advances in computational modeling studies aimed at bridging the gap between the more accurate but computationally expensive atomistic and molecular-level simulation methods and the mesoscopic, nano-level coarse-grained modeling methods that are capable of including, within good accuracy limits, both biomolecular and inorganic (i.e., ENMs) systems (Mahmoudi, 2022; Bashiri et al., 2023).
The current developments in the computational modelling for IVDD using nanocarriers (NCs) can be summarized in three major conceptual stages based on their associated interaction processes. For simplicity, here we will focus on NPs as the main NCs, though the same physico-chemical considerations can be applied in the case of other NEM systems (Rizvi and Saleh, 2018; Nasra et al., 2022; Yang et al., 2022). These three conceptual stages, illustrated in Figure 2, mirror the chemical processes occurring during the NP’s exposure to the biological environment:
(i) NP’s functionalization and drug loading,
(ii) formation and dynamic alteration of nanoparticle protein corona (NPPC) structures on the NP in the bloodstream, and
(iii) interactions of NPs with cell membrane and drug release.
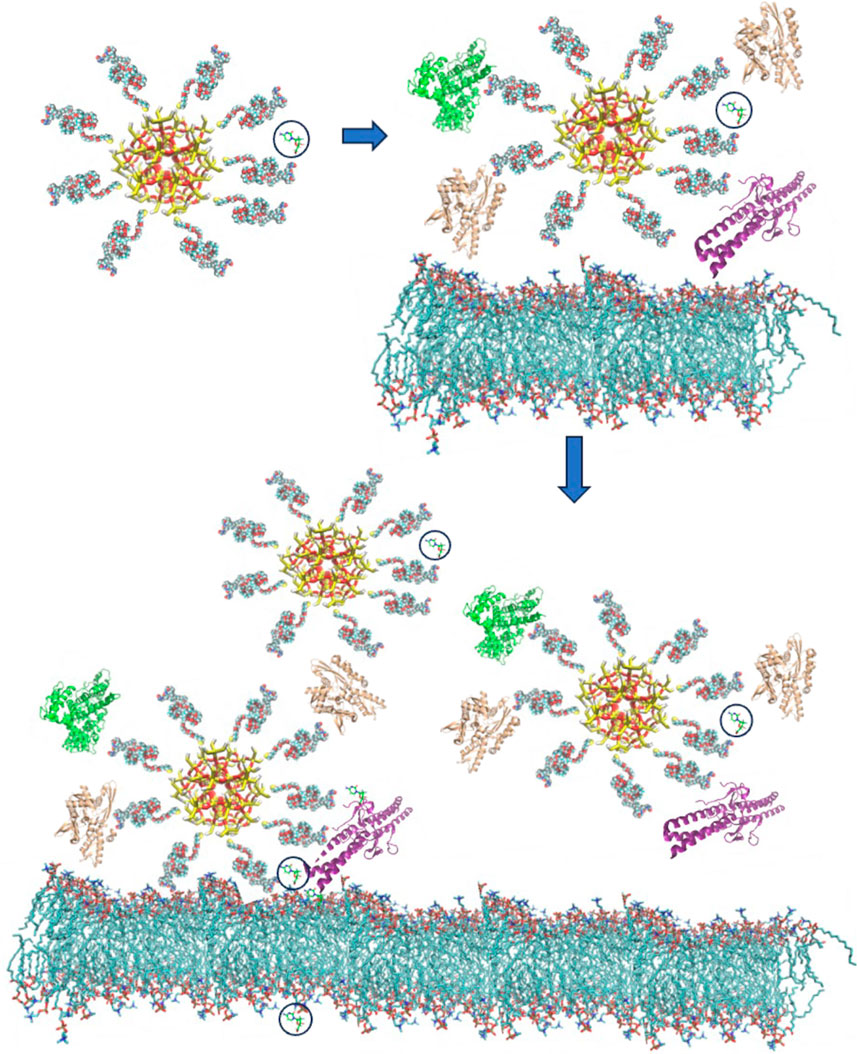
FIGURE 2. Schematic representation of the three main conceptual stages of interaction between a functionalized NC particle carrying a drug molecule (highlighted with a black circle) as it approaches a biological cell. Upon entering the biological environment (e.g., insertion in a blood vessel in the case for IVDD), the NC-drug system recruits additional biomolecules, especially proteins (e.g., shown in a cartoon representation as green, brown, or purple). In the final stage (bottom) the corona-covered NC interacts with the cellular membrane and, possibly with other NCs, and the complex process of drug release is initiated.
2.1 Functionalization of NPs and drug loading
The effects of NP functionalization, grafting density and role of different PEG conformations in modulating the function of the liposome was simulated using coarse grained molecular dynamics (CGMD) techniques (Lemaalem et al., 2020). CGMD can capture the behavior of liposomes made using PEG-coated dipalmitoylphosphatidylcholine (DPPC) membrane bilayers and using MARTINI (Lee et al., 2009) beads for CGMD modeling in an aqueous environment (Lemaalem et al., 2020). The mean squared displacement can be monitored for observables such as the membrane changes during transition of the molecular spacers from mushroom-like into brush-like, more elongated conformations. This particular functionalization can be important in enabling PEG coatings to maintain lubricative property of the liposome and thus can be useful in designing cargo systems for drug delivery (Lemaalem et al., 2020).
Additional examples (Figure 3) which are addressable via all-atom MD/CGMD simulations to probe the choice of carbon nanotubes (CNTs) as possible IVDD vehicle have also been proposed (Mollazadeh et al., 2021). Tunning amino acids type, sequences, and the stoichiometry of CNT-attached self-assembled peptides, are also shown to improve dispersion of CNT; whereas PPI (i.e., polypropylene imine) dendrimers and PAMAM with specific branching densities in vicinity of PEG, are found to form cumbersome architectures and thus block the release of the drug from CNTs (Kavyani et al., 2018). The binding affinity between anti-cancer drug penicillamine and CNT is increased in presence of carboxyl functionalization. Similarly, for oxorubicin drug delivery, N-isopropyl acrylamide (NIP) enhances thermal stability of CNT. Co-loading and release of two anti-cancer drugs in chitosan-modified CNTs is also analyzed using simulations (Karnati and Wang, 2018). Penetration of doxorubicin-CNT into the lipid bilayer is studied and observed using steered MD, and it is shown that parameters such as the suitable length of the CNTs employed and their preferred orientation in membrane are examples (Sahoo et al., 2018) of different important parameters that one can infer through MD simulation in the course of IVDD mechanism (Mollazadeh et al., 2021).
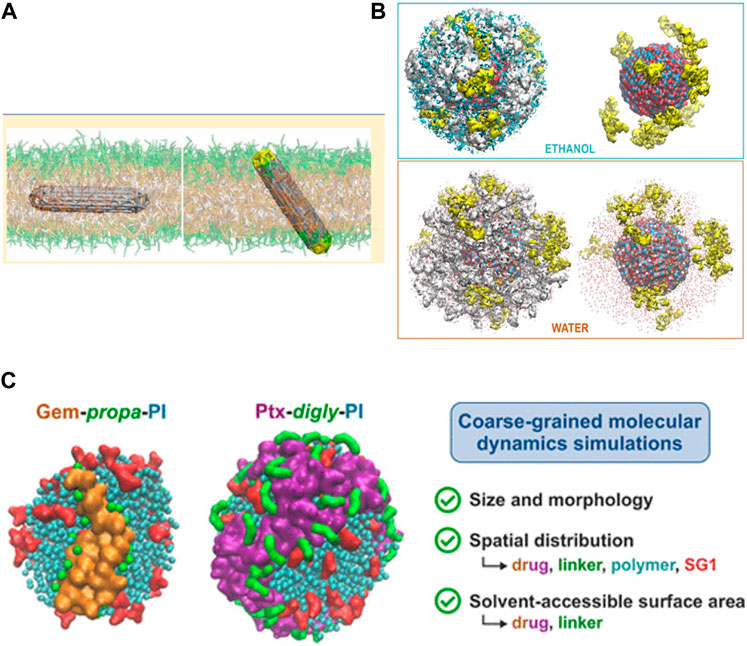
FIGURE 3. Illustration of three different NC systems modelled recently using MD: (A) Penetration of carbon nanotube NCs in bilayers. [Reprinted with permission from Baoukina et al. (2013)]. Copyright 2013 American Chemical Society). (B) Different density distributions of the drug CZX (yellow) in different solvents for a ZnO NP; Oleic acid spacers are shown in while, Zn in black and O atoms in red. [Reproduced from Trouki et al. (2022)], with permission from the Royal Society of Chemistry). (C) Different polymer prodrug NP system combinations: using different drugs (gemcitabine or paclitaxel anticancer drugs), linkers (e.g., propanoate or diglycolate), polymers (e.g., polyisoprene), and a capping agent (SG1). [Reprinted with permission from Gao et al. (2021). Copyright 2021 American Chemical Society).
Umbrella sampling is another technique that can be used to compute quantities such as the potential of mean force (PMF) for releasing certain drug molecules in the cellular medium (Allen and Tildesley, 2017; Frenkel and Smit, 2023). In recent studies (Moradi et al., 2018; Alinejad et al., 2020), this method is applied to study graphene oxide (GO) modified by longer PEG chains or folic acid to enhance the availability of drugs in cell. The penetration of gold NPs (Zolghadr and Moosavi, 2019) and carbon dots (Erimban and Daschakraborty, 2020) from water to DPPC/phosphatidylcholine membrane was estimated quantitatively using free energy calculations. The self-assembly of Janus lipid dendrimers (Elizondo-García et al., 2018) or drug release for a pH sensitive lipid-dendrimer (Otto and de Villiers, 2018; Maji et al., 2019) was studied in terms of binding energy, center of mass, solvent accessible surface area (SASA), radius of gyrations or contact map fluctuations.
2.2 Nanoparticle protein corona structures in the bloodstream
Regarding the second stage of formation and evolution of NPPC structures, kinetic models (Dell’Orco et al., 2012; Darabi Sahneh et al., 2013; Dogra et al., 2019) in cancer nanomedicine were used to interpret association and dissociation of plasma proteins like human serum albumin (HSA), HDL over NP, it highlights the possible role of NPs size in corona formation. CG-MD simulations offer another popular approach to model corona formation processes that was also used to model NP-corona interactions (Tavanti and Menziani, 2015).
To make connection and compare with experiments, Lobaskin et al. used a Kinetic Monte Carlo approach (Rouse and Lobaskin, 2021) to simulate the formation and equilibrium of the NP protein corona composition for silica NPs, both with and without PEG coating (Hasenkopf et al., 2022). Using a coarse-grained representation and rigid modeling of proteins, very good correlations of the calculated and experimental quantities (e.g., adsorption energies for several proteins) are found (Subbotina et al., 2023).
Recently, motivated also by experimental efforts to quantify the important role of the surface hydrophobicity for NP coronas (Mahmoudi, 2022), Buchete et al. developed a multiscale approach based on a stochastic method for protein docking of proteins on small silica and titania nanoparticles (Annapoorani et al., 2023; Mancardi et al., 2023). Due to computational constraints current implementations are limited to modeling only relatively NPs with relatively small diameters (i.e., 2–5 nm) covered by only a few tens of proteins However, the resulting models of NPPC structures have atomistic resolution and allow the efficient calculation of atomistic descriptors such as the hydrophobic or charge fractions of the solvent accessible surface area, with their corresponding statistical errors. These descriptors are important for the following stage of assessing the processes and interactions of protein-covered nanomaterials with different types of cells, tissues, or other biomolecular targets (Annapoorani et al., 2023; Mancardi et al., 2023).
2.3 Interactions of NPs with cell membrane and drug release
Regarding the third stage of interactions between NCs with target tissues and cells, the NPs size, shape and nature of functionalization may also modulate transportation barriers, penetration depth and immune responses faced by NPs within biological tissues (Islam et al., 2017). In this context, a multiscale Brownian dynamics-based model is proposed (Islam et al., 2017) to study dynamics of NPs in an artificial cell-free system as well as in a heterogeneous porous medium. Here, stationary circles of radius 10
In a different study (Tavanti and Menziani, 2015), a lumen structure with evenly implemented stents was used to model the arterial wall coated with PLGA. The length, density of polymers and the curvature of artery walls are modelled in simulation. The loss of PLGA mass over the simulation, affected drug diffusion pattern which implies gradual degradation of PLGA and release of the drug in artery; the drug binding was further estimated via its distribution profile over artery wall. Nonetheless, this model has limitations as it excludes diverse physiological conditions.
With regard to the efficiency of the drug release stage, the cleavage of the drug-polymer linker of a prodrug conjugate, under specific cellular conditions (e.g., lower pH in cancer tumours) is an important parameter that needs to be studied and understood in detail. A CGMD study (Gao et al., 2021) on different prodrugs consisting diverse drug-linker-polymer combinations (either a gemcitabine or a paclitaxel anticancer drug, either a propanoate or a diglycolate linker, and a polymer named polyisoprene, Figure 3) was performed; supramolecular assembly, solvation free energy of such systems is calculated over simulated trajectories. It was observed that gemcitabine becomes more exposed, but paclitaxel remains buried, similarly the bioavailability for all the drug-polymer linkers shows differences as per observations. This justifies usage of MD to model suitable cargo for drug release in targeted area.
The interaction between two anticancer drugs named flutamide (hydrophobic) and glutathione (GSH, hydrophilic) with a model membrane composed of DPPC lipids, in their free as well as in gold NP loaded states, was also studied using MD (Farhadian et al., 2022). Here, gold nanoparticles enhanced permeability of pure GSH within DPPC, whereas for hydrophobic flutamide the diffusivity is decreased. However, the formation of inactive complex by flutamide over bilayer is also an important step, as it blocks androgen receptor. Thus, MD can also reveal different drug release mechanisms and their impacts to achieve desired goal.
The role of pH in a diseased cell was further investigated for two different dendrimers named PPI and PAMAM at three different pH of solutions. The simulations (Gupta and Biswas, 2018) focused on changes of fractal dimensions as well as changes in conformational fluctuations of those dendrimers as function of pH. Thus, one can design the most suitable one after gaining insights from simulated data (Gupta and Biswas, 2018).
Additionally, NPs can also lead to cytotoxicity in vicinity of membrane, as it may alter shape, curvature, thickness or order parameter of hydrophilic tails leading to leakage or pore formation within bilayer (Song et al., 2012; Ding and Ma, 2018; Bunker and Rog, 2020); thus, a balance between increased therapeutic efficiency and decreased cytotoxicity of NCs is demanded. Specifically, MD simulations (Dallavalle et al., 2015; Mhashal and Roy, 2016) reveal that smaller size of gold or graphene NPs has minimalistic effects on lipids fluidity, whereas the larger size show prominent effects. On the other hand, a CGMD study (Lin and Gu, 2014) indicates that surface roughness and spacer densities of NCs can also affect phase transition temperature of bilayer. Shi et al. (2011) demonstrates that NCs with small cap can orient themselves in a vertical manner within bilayer but the elongated nanotube/nanosheets with sharp edges require larger binding energy, which gradually leads to membrane rupture.
Additional crucial parameters are ligand distributions, orientations, or the ligand arrangement pattern over NC’s surface for specific receptor binding within cell, their potential role was mentioned in MD and Monte Carlo simulations (Martinez-Veracoechea and Frenkel, 2011; Liu et al., 2016; Angioletti-Uberti, 2017). Thus, one needs to consider all these aspects while designing NCs for IVDD.
A system of zinc oxide (ZnO), decorated with oleic acid chains and loaded with the drug carfilzomib (Figure 3) was simulated using ReaxFF MD (Trouki et al., 2022). Here, the self-assembly pattern of NC, the binding between drug-NC, the dynamics of the entire cargo, release of the drug within solvent was analyzed theoretically.
To sum up, enabled by the impressive growth of computational atomistic and molecular modeling methods developed in recent years, the field of computational modeling of IVDD using NCs is reaching new frontiers. This growth was accelerated by advances in the availability of (i) faster and affordable high-performance computer (HPC) hardware resources, (ii) multiscale, both atomistic but especially coarse-grained-based modeling approaches, and (iii) revolutionary parameterization methods steaming from machine learning approaches that allow simplified yet accurate modeling and simulation methods to overcome their accuracy and efficiency-related limitations.
3 Macroscale modelling
The convection and diffusion properties of nanoparticles in flow media and particularly in blood affects their ability to interact with the vascular wall. Experiments show that there is a considerable effect of particle size along with hemodynamics, blood rheology, and vessel size on the adhesion efficiency (Charoenphol et al., 2010). However, experimental setups can be costly and difficult to construct. Further to their ability to simulate atherosclerosis formation (Avgerinos and Neofytou, 2019), macroscale modelling provides a cost-effective alternative method for this kind of tests and can assess the effects of parameters, including magnetic field strength (MFS), magnet size, particle size, the initial position of particles, and the relative magnetic permeability of particles, on the efficacy of MDT through the artery walls are characterized (Manshadi et al., 2018; Mahmoodpour et al., 2020). In addition, the optimal particle size range for which the surface density of particles (SDP) adhered on the plaque lumen reaches its maximum can be specified (Shamloo and Forouzandehmehr, 2019). Optimization of physical parameters of such particles and the magnetic configuration, estimating the fraction of particles reaching a given target site in a large patient-specific vascular system can also consider different physiological states (heart rate, cardiac output, etc.) (Patronis et al., 2018). Macroscale modelling in silico methods can also study different classes of magnetic cores and the thickness of biocompatible coating materials (Lunnoo and Puangmali, 2015). Novel computer simulation models have been developed to study nanoparticle transport in a representative artery system, subject to shear-induced diffusion of nanoparticles due to hydrodynamic interactions with red blood cells using computational fluid dynamics (Xu and Kleinstreuer, 2018) or Lattice Boltzmann methods (Liu et al., 2018). Patient specific geometries reconstructed from DICOM images, such of the coeliac trunk, can also be taken into account (Boghi et al., 2017). Particle agglomeration can also be considered to assess the NP bio-related capabilities.
3.1 Nanoparticles in bloodstream
The most widely used methods in simulating nanoparticles in fluid flow are Lagrangian and the Eulerian methods. The Lagrangian methods describe the path of each individual particle whereas the Eulerian methods simulate the particles as a whole, i.e., as a concentration with similar properties at certain different location of the domain. A further distinction concerns the numerical methods for simulation fluid flow namely, conventional Computational Fluid Dynamics (CFD) that solve numerically the Navier-Stokes equations that take into account macroscopically the pressure and the velocities of the medium and the Lattice-Boltzmann Method (LBM) where a state represents the position and the velocity of interacting pseudo-molecular particles of a fluid (Korolija et al., 2017). In addition to this, and in terms of the geometry used in the simulations, modeling approaches use either a simplified geometry which usually represents an axisymmetric model of an artery with a stenosis or an aneurysm (Neofytou and Tsangaris, 2006) or a case-specific geometry of an arterial segment that is derived from CT or MRI scans of a patient (Skiadopoulos et al., 2017). Furthermore, and in terms of the blood properties, blood can be simulated as a Newtonian or Non-Newtonian fluid. For the latter case, Non-Newtonian blood models are to be employed in the computations (Neofytou, 2004).
3.1.1 Lagrangian methods
Roa-Barrantes and Rodriguez-Patarroyo (Roa-Barrantes and Patarroyo, 2022) studied the kinetic behavior of a magnetic nanoparticle subjected to external magnetic fields in an arteriole axisymmetric model with pulsatile flow. They gave the trajectories of the particle with dependence on its starting position and magnetic intensity. A tube model of an artery was also used by Lee et al. (2022) to conduct a parameter study to show the effects of changing the dipole moment, the distance from the magnet to the blood vessel, and the initial release point of the nanoparticles using the Carreau model for modelling the rheology of the blood. A simplified geometry was used by Hussain et al. (2023) depicting an intracranial aneurysm where the effects of Ag nanoparticles are studied. A 2D bifurcation was employed by Larimi et al. (2014) to simulate the flow and magnetic targeting of SPIONs as novel drug delivery vehicles with particle tracking to estimate particle behavior under influence of imposed magnetic field gradients along the bifurcation.
Qiao et al. (2022) used idealized three-dimensional geometric models of coarctation of the aorta for their simulations and the goal was to propose a method to calculate the shear stress thresholds for the diseased aorta. In this respect and although their work does not include nanoparticle dispersion calculations, it preliminary demonstrates the feasibility of shear-activated targeted nanoparticle drug delivery in the treatment of aortic diseases. Tripathi et al. (2021) studied the unsteady hybrid-nanoparticle (silver and copper) hemodynamics in an axisymmetric model of an inclined artery with mild stenosis and aneurysm features. A Finite Difference Scheme together with the Carreau fluid model whereas the NPS were considered to have various shapes (bricks, cylinders, platelets, blades). Results showed that the inclusion of hybrid nanoparticles (Ag–Cu/Blood) within blood increases the axial velocity and temperature magnitudes more significantly as compared to unitary nanoparticles (Ag/blood) at both the stenosis and aneurysm segments.
As opposed to simplified geometries, Shamloo et al. (2019) numerically reproduced the arterial geometry by image processing of CT-scan images of a case specific coronary arterial segment. They implemented the finite element method together with Fluid-Structure Interaction (FSI) capabilities simulation based on physiological boundary conditions and using the non-Newtonian Carreau-Yasuda model for the blood. For the particle dispersion, a Lagrangian description of Magnetite particle dynamics considering momentum equation of motion for each particle under an imposed external magnetic field was assumed. A patient-specific geometry was also used by Boghi et al. (2017) and was reconstructed from a data set of CT scan images of the coeliac trunk of a middle-aged healthy man. Nanoparticles, used for drug targeting for the treatment of hepatic cancer, are supposed to be dragged into the liver by an external magnetic field which was also taken numerically into account by the Maxwell equations. For the simulations, the CFD package OpenFOAM was used that couples the Lagrangian particle dynamics with the Navier–Stokes equations. A widely used CFD package namely, ANSYS Fluent™ was used by Hewlin et al. (2019) for the fluid-particle flow simulation in a patient-specific domain of a diseased left carotid artery bifurcation. The model is used to investigate the transport and targeting efficiency of magnetite micron range particles taking into account an external magnetic field as a way towards effective treatment of both cancer and cardiovascular disease. The latter disease is also the focus of Suo et al. (2012) who simulated the flow in a patient specific part of the left coronary artery to examine the feasibility for treatment with iron nanoparticles and by modeling hypothetical magnet fields. Lindemann et al. (2021) used the finite element method (FEM) of SPIONs in a capillary sized vessel network to study the effect of the magnetic field gradient as well as of magnet distance to the vessel geometry, magnetic flux density of the magnets, SPIONs hydrodynamic diameter and magnetic moment on the Magnetic-drug-targeting effectivity. Aryan et al. (2022) also used FEM aiming at enhancing the SPION delivery to the desired branch of a 3D patient-specific carotid bifurcation using four different magnet configurations implanted adjacent to the artery wall. They employed the non-Newtonian Carreau model for the blood flow and the results show that it is possible to guide more particles to the desired branch (up to 4%) while preventing particles from entering the unwanted branch (up to 13%). A numerical study into nanoparticle transport in a bifurcated carotid artery, and a scaled-down version approximating an arteriole and branch capillaries, has also been conducted by Doig et al. (2012) using an in-house code to establish the influence of size on the trajectory and residence time of particles.
With respect to LBM, Patronis et al. (2018) developed a model for the simulation of magnetic particles in a patient-specific circle of Willis, the central blood distribution system in the brain, in order to determine the fraction of injected particles that reach a defined target site under varying physical parameters (of the nanoparticles) and physiological states (of the patient) using an external stationary magnet. The LBM can be combined with a spectrin-link method for RBCs, and a Langevin dynamics (LD) approach to capturing the motion of the suspended NPs to fully resolve the NP transport in cellular blood flow (Liu et al., 2018). The deformability of particles can also be taken into account by employing a Moving Least Squares (MLS) approach to accurately interpolate the pressure, velocity and force fields between the Eulerian and Lagrangian meshes describing the fluid and the capsule dynamics, respectively (Coclite et al., 2019) thereby addressing particle flows in capillaries. Tan et al. (2016) carried out a numerical study on NP transport and dispersion in red blood cell (RBC) suspensions under shear and channel flow conditions, utilizing an immersed boundary fluid-structure interaction model, an elastic cell membrane model and a particle motion model driven by both hydrodynamic loading and Brownian dynamics. This model accounts also for RBC deformation and results confirm previous findings that the NP dispersion rate is strongly influenced by local disturbances in the flow due to RBC motion and deformation.
3.1.2 Eulerian methods
The treatment of hepatic cancer was also the scope of Xu and Kleinstreuer (2018) that used a geometry representative of the human hepatic artery system to study nanoparticle transport subject to shear-induced diffusion of nanoparticles due to hydrodynamic interactions with red blood cells. The blood flow was described non-Newtonian model capturing the shear-thinning behavior at elevated shear rates whereas the convection-diffusion equation was employed to describe nanoparticle transport, incorporating both Brownian as well as shear-induced diffusion effects. A 2D collapsible tube was employed by Aminfar et al. (2013) to investigate the laminar flow of a ferrofluid consisting of blood and Fe3O4 spherical shape nanoparticles under the influence of different magnetic fields. A 3D Y-branched artery-model that is supposed to supply a tumor region was considered by Gitter and Odenbach (2013) who carried out FEM of the flow simulating the blood as a Carreau -Yasuda fluid to account for its shear-thinning properties. The purpose was to study drug-loaded nanoparticles concentrated within a target region due to the influence of a magnetic field. Poonam et al. (2022) investigated the consequences of nanoparticle addition on blood flow passing through a curved artery model with mild stenosis and aneurysm conditions. They used the Crank-Nicolson method is applied to solve governing equations and showed that with increase in volume fraction of gold (Au) nanoparticles, the velocity profile rises, while, reverse effect is noticed for the volume fraction of aluminium oxide (Al2O3) nanoparticles. The inclusion of inertia in NP dispersion in biological flows was taken into account by Pilou et al. (2011) to study the dispersion and impingement on the wall of particles of various sizes in a bend and subsequently in a physiologically realistic bifurcation (Pilou et al., 2013).
3.2 Nanoparticle agglomeration
NP agglomeration strongly affects their biodistribution, thus determining reactivity, toxicity, fate, transport, and risks. NP agglomeration is induced by the collision frequency and the collision efficiency of the phenomenon. The collision frequency is the mechanism that brings NPs in contact and is governed by Brownian diffusion, fluid motion and differential sedimentation. The collision efficiency is the mechanism that makes NP stick together and is based around the Derjaguin-Landau-Verwey-Overbreak (DLVO) or the extended DLVO (xDLVO) theory (Hotze et al., 2010), according to which the sum of attractive and repulsive forces between NPs such as Van der Waals, Electrostatic and Magnetic forces determines the intensity of agglomeration. Numerical modelling of aggregation/agglomeration is given by the discretised form of the Population Balance Equation (PBE) (von Smoluchowski, 1917) and predicts the evolution of the aggregates size distribution and includes parameters to account for the kinetics and the dynamics of the phenomenon.
Exploiting this, Atmuri et al. (2013) performed integrated experimental and modeling study to explore aggregation in concentrated attractive colloidal suspensions with the use of the PBE model that includes the full calculation of the stability ratio as well as variations in DLVO interactions between particles and aggregates as aggregates grow. The model successfully predicts aggregation regimes of charged colloidal particles over a range of salt concentrations and particle concentrations. Runkana et al. (2004) attempted to integrate the theories of surface forces in presence of polymers with the population balance framework to model polymer-induced flocculation. They solved the geometrically sectioned population balance equation (PBE) according to the scheme by Hounslow et al. 1988) where collision efficiency was estimated as a function of van der Waals attraction, electrical double layer repulsion and bridging attraction or steric repulsion. Biggs and Lant (2002) also applied the method developed by Hounslow et al. (1988) for modelling activated sludge flocculation, providing a good approximation of the change in mean floc size with time.
Liang et al. (2016) used a different kind of sectioning of the PBM for aggregation of particles as, namely, the one proposed by Kumar and Ramkrishna (1996) to predict aggregation behavior of submicron-sized particles of praseodymium-doped zirconium silicate in aqueous suspension. The model was also based on the xDLVO theory and was further modified to predict the stable state of the aggregation by introducing the volume mean size of the aggregate to the stability ratio. The same method of sectioning of the PBM was used by Huang et al. (2020), who studied the dispersion/aggregation behavior of colloidal particles in the suspension under different conditions. The colloidal system consisted of a dispersion of praseodymium-doped zirconium silicate pigment in an aqueous suspension by modified hydroxyl copolymer where the interactions between the models were modelled based on the xDLVO theory and an experimental study was also performed, with the authors reporting that predicted data are similar to the experimental results.
Grabinski et al. (2016) studied the implications of agglomeration and unique transport kinetics of NPs in biological media affecting cellular dose in typical in vitro toxicity experiments involving exposure of a mono- or co-culture of cells to nanoparticles (NPs). For this a transport model was used to approximate the shear rate experienced by the cells in dynamic conditions to evaluate physiological relevance. The transport kinetics show that NP behavior is governed by both gravity and diffusion forces in static conditions and only diffusion in dynamic conditions. Neofytou et al. (2022) performed simulations solving the PBE in conjunction with the xDLVO theory using the Kumar and Ramkrishna (1996) method for assessing the colloidal stability and aggregation tendency of iron oxide nanoflowers (IONfs) in biofluids. Colloidal stability experiments were also conducted on IONfs in aqueous phosphate buffered saline solution. The simulations showed a slight overprediction of the aggregation rate compared to the experiments and this could be attributed either to the patchiness on the NP surface, or to the fractal nature of aggregates that could not be considered in the model.
3.3 Nanoparticle targeting
Simulations of nanoparticle targeting aim at (i) assessing the effect of an externally applied magnetic field on flow of NPs in the circulatory system and ultimate reaching their target-tissue and (ii) looking into the NP’s ability to adhere on the target-tissue depending on the molecular functionalization of NPs intended for binding to a specific receptor in the macroscale by applying proper boundary conditions.
3.3.1 Magnetic targeting
Magnetic targeting simulation (Figure 4) provides insight into the attributes of the magnetic field that is needed to achieve the desired NP-accumulation outcome avoiding thereby the necessity of experimental setups that would have been otherwise costly especially when a parametric study is required regarding magnetic efficiency based on magnet positioning and also both geometric and magnetization attributes of the NPs. Modelling usually facilitates the analytical expressions of the magnetic field induced by a stationary magnet such as bar magnet, circular loop, infinite wire etc (Smythe, 1950).
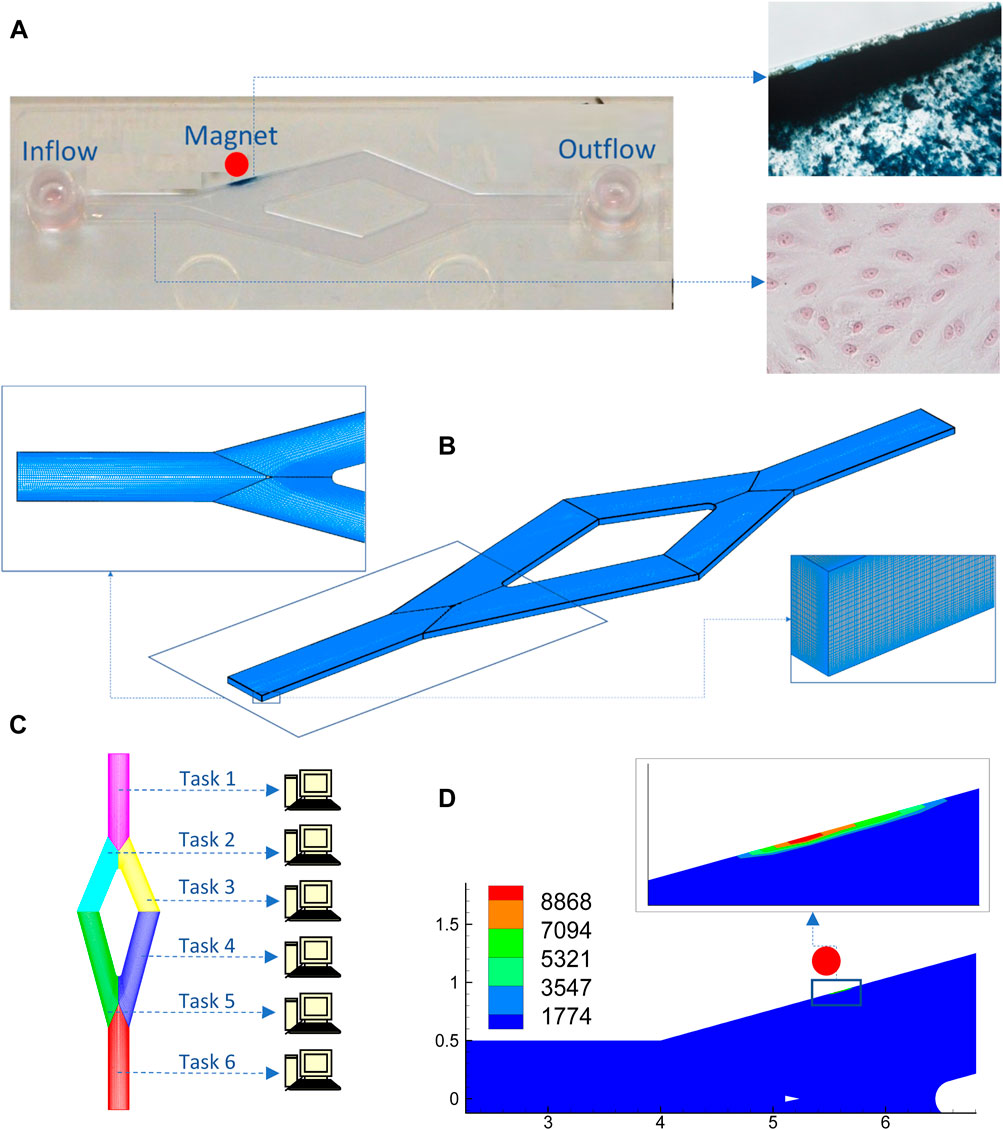
FIGURE 4. Macroscale simulation of an in vitro experiment of a SPIONs solution that is circulating through a channel and is under the influence of an external magnetic field: (A) In vitro slide image (left) with a monolayer of endothelial cells at the bottom (lower-right panel) and accumulated particles (right upper-panel); (B) Grid for flow-particle dynamics simulation with boundary fitting topology (left) and refinement applied near the wall (right) to better capture localized effects; (C) Grid with a multiblock architecture enabling parallel processing; (D) Simulation and results regarding particle internalization using a code encompassing a module for particle dispersion and agglomeration, a module for external magnetic fields and particle-depended wall boundary conditions.
A range of magnetic field strengths were modeled by Lee et al. (2022) for the motion of superparamagnetic nanoparticles in a tube model using equations for both a bar magnet and a point dipole and it was shown that the bar magnet is effective at capturing nanoparticles in limited cases, while the point dipole is highly effective across a range of conditions. The same geometrical setup and the magnetic field by a cylindrical external permanent magnet was used to specify trajectories and capturing of magnetic nanoparticles coated with drug agent injected at the entrance of a vessel and captured by a magnet located at a specified location where tumor exists (Sharma et al., 2015; Shazri and Idres, 2017). A tube model was also used by Roa-Barrantes and Patarroyo (2022) with a magnetic field produced by an infinite cylindrical magnet to study the trajectory of a magnetic NP in pulsatile flow. Manshadi et al. (2018) used a four-layer structural 2D model of the artery tissue and the magnetic field around a rectangular prism to study the delivery of both clusters of drug micro- and nanoparticles and single drug/cargo into the luminal artery for curing inflammation, and their translocation through the artery layers. A 2D channel model of a vessel was also used by Mahmoodpour et al. (2020) together with a cylindrical permanent magnet positioned below with varying-magnetic-field-intensity options to study two cases of initially uniform distribution of drug carrying particles in the blood flow and separate injection of the particles upstream of the target area and showed that capture efficiency increases with increase of the magnetic field intensity and particles diameter and decrease with the blood flow velocity. The magnetic field created by a solenoid situated near a 2D channel model with a stenosis was used to show that it provokes the accumulation of magnetic NPs in stagnant zones with vortices induced in the flow field and appear near the stenosis (Starodumov et al., 2023). A more complex 2D arterial model with multiple bifurcations together with a permanent magnet positioned at its side is used to computationally study the size-dependent capture efficiency of Fe3O4, Fe2O3 and Fe NPs in mimicked arterial flow (Lunnoo and Puangmali, 2015). Carrier sizes of 10 nm-4 μm in radius were considered and results show that particles larger than 2 μm were efficiently captured at the desired location by the external magnetic field, and the capture efficiency was approximately 95%.
A case specific Left Anterior Descending (LAD) coronary artery geometry was used by Shamloo et al. (2019) together with an externally imposed magnetic field with a specific designated direction that would significantly elicit particles to move toward the vessel wall (margination). Under the influence of the magnetic field, the optimal particle size scope for which the surface density of particles adhered on the plaque lumen reaches its maximum was numerically specified. In another case-specific study the magnetic field of a cylindrical electromagnet was used to assess particle capture efficiency in a diseased left carotid bifurcation artery using the magnetic properties of magnetite and equations describing the magnetic forces acting on particles produced by an external cylindrical electromagnetic coil and achieving capture-efficiency up to approximately 98% (Hewlin et al., 2019). A 3D geometry representation of a bifurcating in vitro setup was used by Tsimpoukis et al. (2023) together with a permanent magnet placed at the bifurcation and modelled as an infinite wire to assess the localized accumulation of particles with and without magnetic field.
A combination of stationary magnets namely, twelve small permanent magnets positioned in three consecutive quadrupole arrangements were placed around a tube model of an artery and the flow-particle field was computed so as to predict the amount and position of particle complexes which can be retained by the imposed magnetic field (Heidsieck et al., 2012). The purpose was to distribute complexes consisting of lentivirus and magnetic nanoparticles as homogeneously as possible along a perfused murine aorta as a part of regenerative medicine in the cardiovascular system aiming at the restoration of the healthy physiological conditions to the diseased heart and the vascular system. Lindemann et al. (2021) carried out simulations of Magnetic drug targeting tracing single SPIONs in a 3D geometry of eight multibranched vessels with sizes in the range of capillaries assuming two different configurations of magnet arrays, i.e., 6 bar config-uration and a 5 bar Halbach configuration. The results show that the magnetic targeting effectivity is up to 32% higher than the one calculated without the presence of a magnetic field.
3.3.2 Adhesion on tissues
Adhesion is a physical mechanism that depends on a variety of parameters that range from the local fluid (blood) flow conditions, especially shear rate, to the size, shape and ligand surface decoration of NPs and the availability of the corresponding receptors on the vasculature wall at the site of interest. The simulation of this mechanism is usually defined by a boundary condition that is imposed when the fluid-particle equations are numerically solved.
The effort towards simulation adhesion mainly stems from the need to assess by modelling the deposition of particles suspended in fluids that flow over adhesive walls which are facilitated by the formation and dissociation of biological bonds between receptors and ligands. Adhesion of microparticles such as monocytes and platelets to a vascular surface are usually modelled by a boundary condition for a reactive surface equating mass diffusion at the wall with the adhesive flux (David et al., 2001; Longest and Kleinstreuer, 2003; Weller, 2008; Tokarev et al., 2011). In other words, the adhesive flux given as the product of the particle concentration at the wall times the adhesion rate constant (which depends on parameters such as the binding strength, shape, size, local shear stress, etc.) equals the flux of the particles arriving at the wall by diffusion that is given as the product of the partial derivative of the concentration near the wall to the distance from it times the diffusion coefficient. This equation can be extended to include a term for the dissociation of bound particles (Lyczkowski et al., 2009; Hansen et al., 2012) as a product of the concentration of bound particles times a dissociation constant depending on parameters similar to the adhesion rate constant.
This equation was tailored for predicting delivery carriers across the nano-metric length scale using 43 nm and 1.1 mm diameter polystyrene spheres (Haun et al., 2010) where monoclonal antibody specific for ICAM-1 is utilized as the targeting receptor and adhesion to ICAM-1 coated glass substrates in a parallel-plate flow chamber at physiological shear rates is quantified. This equation was further used to elucidate the effects of particle size on the selective binding efficiency of nanoparticles to vessel wall that experiences temporal and spatial variations in wall shear stress and where non-Newtonian viscosity characteristic of blood was incorporated by using the Carreau model (Kim and Rhee, 2011; Jeong et al., 2013).
The adhesion rate constant for microparticles (MPs) can be given as a function of a probabilistic adhesion model which gives the probability to create a biological bond when ligands on the surface of MPs are close to receptors on the vessel wall (Ye H. L. et al., 2019). Decuzzi and Ferrari (2006) developed a probabilistic model for the specific adhesive interaction between a non-spherical particle and a cell layer under a linear shear flow showing that the strength of adhesion is affected by (i) the geometric features of the particle, (ii) the biophysical parameters, such as the equilibrium separation distance between the substrate and the particle; the maximum distance at which ligand–receptor bonds can be formed; the ratio between the shear stress at the wall and the surface density of receptors; the surface density of the ligands and by (iii) the biochemical parameters, such as the characteristic length of the ligand–receptor bond and the characteristic affinity constant of the ligand–receptor pair. Hossain et al. (2014) exploited this function to predict the vascular deposition of blood-borne nanoparticles (NPs), loaded with therapeutic and imaging agents within an inflamed arterial tree. Results show the difference of adhesion attributes between NPs decorated with antibodies directed toward three endothelial adhesion molecules, namely, ICAM-1, VCAM-1 and E-selectin. Further study exploiting the same function (Hossain et al., 2013) shows that adhesion is dramatically affected by changes in patient specific attributes, such as branching angle and receptor density whereas the adhesion pattern correlates well with the spatial and temporal distribution of the wall shear rates. Pilou et al. (2021) used the same function to study particle adhesion on the diseased vascular wall of a patient-specific infrarenal abdominal aortic aneurysm with for a time-dependent flow field over a cardiac cycle. The effect of particle size for 50 and 500 nm nanoparticles and flow field on adhesion efficiency and location is investigated using CFD and it is found that 50 nm particles adhere diffusely on the vascular wall, whereas adhesion of 500 nm particles is highly localized. The same methodology was used to investigate the adhesion of superparamagnetic iron oxide NPs (SPIONs) under steady flow conditions in a bifurcating in vitro setup lined with primary human endothelial cells cultured at the bottom wall (Tsimpoukis et al., 2023). A similar probabilistic function was used by Ebrahimi et al. (2021) to evaluate the surface density of Nanocarriers adhered via ligand-receptor binding to the inner wall of an Abdominal aortic aneurysm (AAA) using CFD and FSI analysis for both patient-specific and ideal AAA models. Adhesion of particles over an atherosclerotic plaque located in the LAD artery of a patient was also considered by the same function (Shamloo et al., 2019). Coclite et al. (2017) present a modified probability of adhesion function based only on the forward bond rate, and on the number of ligands actually probing the surface, for predicting the adhesive interaction of particles with blood vessel walls under capillary flow using a combined Lattice-Boltzmann–Immersed-Boundary method.
4 Outlook
Nanocarriers developed for IVDD applications have shown clinical efficacy in improving bioavailability of drugs and extending the circulation half-life of encapsulated drugs or imaging agents. Based on the preclinical studies, they also have a great potential for disease targeting and improving the organ-specific accumulation. However, the loading/release rate of the loaded drugs and/or imaging agents, as well as the targeting features of nanocarriers are regulated by several and interconnected mass transfer and biochemical mechanisms occurring over multiple time and space scales (from nano to macro). These features depend on the nature of the nanocarrier, its properties like surface charge, size, hydrophilicity, etc., as well as on the specific interactions between the drug and its carrier, not to mention the role of the solvent and of surface functionalization. Therefore, while a comprehensive understanding of these phenomena is far from being achieved by experiments alone, modern materials modelling techniques may accelerate the optimization of drug-loaded nanocarriers. This complexity can be managed by introducing in silico models to investigate the drug transportation, targeting and release mechanisms of multi-functional nanocarriers, with the final goal to significantly improve preclinical studies in terms of cost, time, and animal welfare especially in view of the growing public pressure against animal experimentation in most developed countries that is leading to the development of alternative methods for preclinical assessment.
In silico model development stems from the vision of replacing conventional (pre)clinical trials within silico methods and applies to the development of more efficient nanocarriers for intravascular therapies. The essence of the concept lies in breaking down the experimental stages of a preclinical trial for a novel nanoparticle and defining in silico tools that can model each stage and give credible results, enabling the establishment of a standardized method for in silico preclinical trials. The models developed, validated and exploited for the single stages of preclinical assessment of the nanocarriers should subsequently be integrated into a multiscale model suite.
Numerical simulations of the nanocarriers distribution in physiological and pathophysiological flow conditions, the effects of blood cells and hemodynamic forces on their margination in the vessels and the attachment to the endothelium before paracellular transport, can provide guidelines for optimized NC design. Similarly, modelling of the delivery of NCs to solid tumors can provide insights for the mechanisms of NC transport from the tumor vasculature into cancer cells. The development of in silico mechanistic models which will aim to accurately represent the multiple stages in preclinical trials such as biodistribution, toxicity and targetability studies addressed by MD, CGMD, Molecular Docking, PBPK and CFD models can lead to nanocarrier optimal-by-design modelling. This approach can combine novel and already available in silico tools that will be tailored on a case-by-case basis and will cover all stages from designing the nanocarrier to testing it in accordance with preclinical requirements. In vivo, in vitro and ex vivo data from literature or from design-by-case experiments should be stored on a sustainable database assisting to the development and exploitation of the in silico tools. This approach is expected to save costs and time required for the evaluation of the NCs. Given that preclinical studies are time-consuming, expensive and involve ethical issues that arise from the use of animals for experimental purposes, the establishment of specific guidelines for NC design is of great importance and priority.
Overall, atomistic MD and CGMD simulations can assist and have already been used in designing NCs for IVDD with suitable functionalization. Biophysical descriptors such as the density of spacer molecules, chain length, charge, or the type, shape, and conformations of NCs can be studied and tuned computationally. The binding affinity for the encapsulated drug can also be estimated using free energy calculations. One can study and predict the drug release dynamics (e.g., using umbrella sampling, steered MD, or Markov State Models). By including models of the cellular environment (e.g., the bilayer membrane), it is also possible to study the effects of the interaction between cells and the developed NC-based cargo to minimize toxicity. For larger systems, coarse grained MD and molecular docking can be used to accelerate studies that require longer simulation times. Computational methods for studying and quantifying the NC behavior at a molecular level are increasingly emerging as viable additional approaches to complement experiments for processes such as probing NC functionalization, drug loading, protein corona structures, drug release, or NC-membrane interactions to minimize toxicity and maximize efficacy in intravascular drug delivery.
Macroscale models such as CFD models can exploit the outcome of microscale model development in order to describe fluid particle interaction more accurately but also to define more accurate parameters for boundary-condition equations in terms of simulating large-scale particle-wall interaction that is triggered by receptor-ligand reaction at vascular areas of interest. In addition, microscale models can also assist towards developing more accurate macroscale particle-aggregation models that will better predict the colloidal stability of a system.
Based on simulation efficiency and high in vitro-in vivo extrapolation potential, a multiscale computational modeling framework for NC-based IVDD can greatly reduce the need for in vivo animal experiments especially in terms of determining plasma and organ tissue concentrations in, e.g., animal models for perfusion limited (or perfusion altered) tissue models such as tumors or necrotic tissue, first-pass metabolism, as well as absorption from the bloodstream. Successively, computational models are expected also to be able to support animal-to-human extrapolation. Furthermore, in silico modelling can in the future provide an automated and flexible protocol for the fast prediction of the drug release rate, response to the environment, and targeting of functionalized and drug-loaded nanocarriers. The integration of computational modeling methods in the regulatory process for nanocarrier development could drastically reduce the cost of innovation and, ultimately, the cost of healthcare. For this, further efforts are necessary to strengthen the reliability, efficacy and robustness of the in silico predictive multiscale models used for development and preclinical assessment of nanocarriers in IVDD considering comprehensively three key elements: physics-based theoretical accuracy, experimental validation, and uncertainty quantification at all stages and levels of representation of the in silico studies.
Author contributions
N-VB: Writing–original draft, Writing–review and editing. IC: Writing–original draft, Writing–review and editing. SD: Writing–original draft, Writing–review and editing. PN: Writing–original draft, Writing–review and editing.
Funding
The author(s) declare financial support was received for the research, authorship, and/or publication of this article. IC and PN acknowledge the support by the German Federal Ministry of Education and Research (BMBF) and the Greek General Secretariat for Research and Innovation (GSRI) within the ERA-Net project “MAGNA” (Grant No. 01DJ21004 and MIS 5161147 respectively). N-VB and SD acknowledge the financial support received from the European Union’s Horizon 2020 research and innovation program “NanoinformaTIX” (H2020-NMBP-14-2018, grant 814426), and wish to thank the DJEI/DES/SFI/HEA Irish Centre for High-End Computing (ICHEC) and the Sonic HPC cluster (UCD Research IT) for the provision of computational facilities.
Conflict of interest
The authors declare that the research was conducted in the absence of any commercial or financial relationships that could be construed as a potential conflict of interest.
The author(s) declared that they were an editorial board member of Frontiers, at the time of submission. This had no impact on the peer review process and the final decision.
Publisher’s note
All claims expressed in this article are solely those of the authors and do not necessarily represent those of their affiliated organizations, or those of the publisher, the editors and the reviewers. Any product that may be evaluated in this article, or claim that may be made by its manufacturer, is not guaranteed or endorsed by the publisher.
References
Abouaitah, K., Sabbagh, F., and Kim, B. S. (2023). Graphene oxide nanostructures as nanoplatforms for delivering natural therapeutic agents: applications in cancer treatment, bacterial infections, and bone regeneration medicine. Nanomater. (Basel) 13, 2666. doi:10.3390/nano13192666
Alaarg, A., Senders, M. L., Varela-Moreira, A., Perez-Medina, C., Zhao, Y., Tang, J., et al. (2017). A systematic comparison of clinically viable nanomedicines targeting HMG-CoA reductase in inflammatory atherosclerosis. J. Control Release 262, 47–57. doi:10.1016/j.jconrel.2017.07.013
Alexis, F., Pridgen, E., Molnar, L. K., and Farokhzad, O. C. (2008). Factors affecting the clearance and biodistribution of polymeric nanoparticles. Mol. Pharm. 5, 505–515. doi:10.1021/mp800051m
Alinejad, A., Raissi, H., and Hashemzadeh, H. (2020). Understanding co-loading of doxorubicin and camptothecin on graphene and folic acid-conjugated graphene for targeting drug delivery: classical MD simulation and DFT calculation. J. Biomol. Struct. Dyn. 38, 2737–2745. doi:10.1080/07391102.2019.1645044
Almer, G., Frascione, D., Pali-Scholl, I., Vonach, C., Lukschal, A., Stremnitzer, C., et al. (2013). Interleukin-10: an anti-inflammatory marker to target atherosclerotic lesions via PEGylated liposomes. Mol. Pharm. 10, 175–186. doi:10.1021/mp300316n
Almoustafa, H. A., Alshawsh, M. A., Al-Suede, F. S. R., Alshehade, S. A., Abdul Majid, A. M. S., and Chik, Z. (2023). The chemotherapeutic efficacy of hyaluronic acid coated polymeric nanoparticles against breast cancer metastasis in female NCr-Nu/Nu nude mice. Polym. (Basel) 15, 284. doi:10.3390/polym15020284
Aminfar, H., Mohammadpourfard, M., and Ghaderi, F. (2013). Two-phase simulation of non-uniform magnetic field effects on biofluid (blood) with magnetic nanoparticles through a collapsible tube. J. Magnetism Magnetic Mater. 332, 172–179. doi:10.1016/j.jmmm.2012.12.003
Amiryaghoubi, N., Abdolahinia, E. D., Nakhlband, A., Aslzad, S., Fathi, M., Barar, J., et al. (2022). Smart chitosan-folate hybrid magnetic nanoparticles for targeted delivery of doxorubicin to osteosarcoma cells. Colloids Surf. B Biointerfaces 220, 112911. doi:10.1016/j.colsurfb.2022.112911
Angioletti-Uberti, S. (2017). Theory, simulations and the design of functionalized nanoparticles for biomedical applications: a Soft Matter Perspective. NPJ Comput. Mater 3, 48. doi:10.1038/s41524-017-0050-y
Annapoorani, V. K., Dutta, S., Ajia, O., Lobaskin, V., and Buchete, N.-V. (2023). Atomistic models of protein-protein interactions in nanoparticle coronas. Dublin: U.C. Dublin. (in preparation).
Aryan, H., Beigzadeh, B., and Siavashi, M. (2022). Euler-Lagrange numerical simulation of improved magnetic drug delivery in a three-dimensional CT-based carotid artery bifurcation. Comput. Methods Programs Biomed. 219, 106778. doi:10.1016/j.cmpb.2022.106778
Atmuri, A. K., Henson, M. A., and Bhatia, S. R. (2013). A population balance equation model to predict regimes of controlled nanoparticle aggregation. Colloids Surfaces a-Physicochemical Eng. Aspects 436, 325–332. doi:10.1016/j.colsurfa.2013.07.002
Avgerinos, N. A., and Neofytou, P. (2019). Mathematical modelling and simulation of atherosclerosis formation and progress: a review. Ann. Biomed. Eng. 47, 1764–1785. doi:10.1007/s10439-019-02268-3
Bachelet, L., Bertholon, I., Lavigne, D., Vassy, R., Jandrot-Perrus, M., Chaubet, F., et al. (2009). Affinity of low molecular weight fucoidan for P-selectin triggers its binding to activated human platelets. Biochim. Biophys. Acta 1790, 141–146. doi:10.1016/j.bbagen.2008.10.008
Bai, Q., Xiao, Y., Hong, H., Cao, X., Zhang, L., Han, R., et al. (2022). Scavenger receptor-targeted plaque delivery of microRNA-coated nanoparticles for alleviating atherosclerosis. Proc. Natl. Acad. Sci. U. S. A. 119, e2201443119. doi:10.1073/pnas.2201443119
Balazs, D. A., and Godbey, W. (2011). Liposomes for use in gene delivery. J. Drug Deliv. 2011, 326497. doi:10.1155/2011/326497
Baoukina, S., Monticelli, L., and Tieleman, D. P. (2013). Interaction of pristine and functionalized carbon nanotubes with lipid membranes. J. Phys. Chem. B 117, 12113–12123. doi:10.1021/jp405732k
Barenholz, Y. (2012). Doxil®--the first FDA-approved nano-drug: lessons learned. J. Control. Release 160, 117–134. doi:10.1016/j.jconrel.2012.03.020
Bashiri, G., Padilla, M. S., Swingle, K. L., Shepherd, S. J., Mitchell, M. J., and Wang, K. (2023). Nanoparticle protein corona: from structure and function to therapeutic targeting. Lab a Chip 23, 1432–1466. doi:10.1039/d2lc00799a
Bhowmick, T., Berk, E., Cui, X., Muzykantov, V. R., and Muro, S. (2012). Effect of flow on endothelial endocytosis of nanocarriers targeted to ICAM-1. J. Control Release 157, 485–492. doi:10.1016/j.jconrel.2011.09.067
Bietenbeck, M., Engel, S., Lamping, S., Hansen, U., Faber, C., Ravoo, B. J., et al. (2019). Functionalization of clinically approved MRI contrast agents for the delivery of VEGF. Bioconjug Chem. 30, 1042–1047. doi:10.1021/acs.bioconjchem.9b00142
Biggs, C. A., and Lant, P. A. (2002). Modelling activated sludge flocculation using population balances. Powder Technol. 124, 201–211. doi:10.1016/s0032-5910(02)00017-7
Billingsley, M. M., Gong, N., Mukalel, A. J., Thatte, A. S., El-Mayta, R., Patel, S. K., et al. (2023). In vivo mRNA CAR T cell engineering via targeted ionizable lipid nanoparticles with extrahepatic tropism. Small, e2304378. doi:10.1002/smll.202304378
Boghi, A., Russo, F., and Gori, F. (2017). Numerical simulation of magnetic nano drug targeting in a patient-specific coeliac trunk. J. Magnetism Magnetic Mater. 437, 86–97. doi:10.1016/j.jmmm.2017.04.055
Bunker, A., and Rog, T. (2020). Mechanistic understanding from molecular dynamics simulation in pharmaceutical research 1: drug delivery. Front. Mol. Biosci. 7, 604770. doi:10.3389/fmolb.2020.604770
Cai, X., Luo, Y., Zhang, W., Du, D., and Lin, Y. (2016). pH-sensitive ZnO quantum dots-doxorubicin nanoparticles for lung cancer targeted drug delivery. ACS Appl. Mater Interfaces 8, 22442–22450. doi:10.1021/acsami.6b04933
Chang, L. H., Chuang, E. Y., Cheng, T. M., Lin, C., Shih, C. M., Wu, A. T., et al. (2021). Thrombus-specific theranostic nanocomposite for codelivery of thrombolytic drug, algae-derived anticoagulant and NIR fluorescent contrast agent. Acta Biomater. 134, 686–701. doi:10.1016/j.actbio.2021.07.072
Chao, X., Zhang, Z. L., Guo, L. L., Zhu, J. J., Peng, M. L., Vermorken, A. J. M., et al. (2012). A novel magnetic nanoparticle drug carrier for enhanced cancer chemotherapy. Plos One 7, e40388. doi:10.1371/journal.pone.0040388
Charoenphol, P., Huang, R. B., and Eniola-Adefeso, O. (2010). Potential role of size and hemodynamics in the efficacy of vascular-targeted spherical drug carriers. Biomaterials 31, 1392–1402. doi:10.1016/j.biomaterials.2009.11.007
Chen, B., Pogue, B. W., Hoopes, P. J., and Hasan, T. (2006). Vascular and cellular targeting for photodynamic therapy. Crit. Rev. Eukaryot. Gene Expr. 16, 279–305. doi:10.1615/critreveukargeneexpr.v16.i4.10
Chen, H. A., Ma, Y. H., Hsu, T. Y., and Chen, J. P. (2020). Preparation of peptide and recombinant tissue plasminogen activator conjugated poly(lactic-Co-glycolic acid) (PLGA) magnetic nanoparticles for dual targeted thrombolytic therapy. Int. J. Mol. Sci. 21, 2690. doi:10.3390/ijms21082690
Chen, H. T., Kaminski, M. D., Pytel, P., Macdonald, L., and Rosengart, A. J. (2008). Capture of magnetic carriers within large arteries using external magnetic fields. J. Drug Target. 16, 262–268. doi:10.1080/10611860801900892
Chen, W., Schilperoort, M., Cao, Y., Shi, J., Tabas, I., and Tao, W. (2022a). Macrophage-targeted nanomedicine for the diagnosis and treatment of atherosclerosis. Nat. Rev. Cardiol. 19, 228–249. doi:10.1038/s41569-021-00629-x
Chen, Y., Chen, Z., Duan, J., Gui, L., Li, H., Liang, X., et al. (2022b). H(2)O(2)-responsive VEGF/NGF gene co-delivery nano-system achieves stable vascularization in ischemic hindlimbs. J. Nanobiotechnology 20, 145. doi:10.1186/s12951-022-01328-6
Cheng, X., Xie, Q., and Sun, Y. (2023). Advances in nanomaterial-based targeted drug delivery systems. Front. Bioeng. Biotechnol. 11. doi:10.3389/fbioe.2023.1177151
Chong, S. Y., Wang, X., Van Bloois, L., Huang, C., Syeda, N. S., Zhang, S., et al. (2023). Injectable liposomal docosahexaenoic acid alleviates atherosclerosis progression and enhances plaque stability. J. Control Release 360, 344–364. doi:10.1016/j.jconrel.2023.06.035
Cicha, I. (2016). Strategies to enhance nanoparticle-endothelial interactions under flow. J. Cell. Biotechnol. 1, 191–208. doi:10.3233/jcb-15020
Claesson-Welsh, L., Dejana, E., and Mcdonald, D. M. (2021). Permeability of the endothelial barrier: identifying and reconciling controversies. Trends Mol. Med. 27, 314–331. doi:10.1016/j.molmed.2020.11.006
Coclite, A., Mollica, H., Ranaldo, S., Pascazio, G., De Tullio, M. D., and Decuzzi, P. (2017). Predicting different adhesive regimens of circulating particles at blood capillary walls. Microfluid. Nanofluidics 21, 168. doi:10.1007/s10404-017-2003-7
Coclite, A., Ranaldo, S., De Tullio, M. D., Decuzzi, P., and Pascazio, G. (2019). Kinematic and dynamic forcing strategies for predicting the transport of inertial capsules via a combined lattice Boltzmann - immersed Boundary method. Comput. Fluids 180, 41–53. doi:10.1016/j.compfluid.2018.12.014
Courant, T., Bayon, E., Reynaud-Dougier, H. L., Villiers, C., Menneteau, M., Marche, P. N., et al. (2017). Tailoring nanostructured lipid carriers for the delivery of protein antigens: physicochemical properties versus immunogenicity studies. Biomaterials 136, 29–42. doi:10.1016/j.biomaterials.2017.05.001
Dallavalle, M., Calvaresi, M., Bottoni, A., Melle-Franco, M., and Zerbetto, F. (2015). Graphene can wreak havoc with cell membranes. ACS Appl. Mater Interfaces 7, 4406–4414. doi:10.1021/am508938u
Danhier, F., Ansorena, E., Silva, J. M., Coco, R., Le Breton, A., and Preat, V. (2012). PLGA-based nanoparticles: an overview of biomedical applications. J. Control Release 161, 505–522. doi:10.1016/j.jconrel.2012.01.043
Darabi Sahneh, F., Scoglio, C., and Riviere, J. (2013). Dynamics of nanoparticle-protein corona complex formation: analytical results from population balance equations. PLoS One 8, e64690. doi:10.1371/journal.pone.0064690
David, T., Thomas, S., and Walker, P. G. (2001). Platelet deposition in stagnation point flow: an analytical and computational simulation. Med. Eng. Phys. 23, 299–312. doi:10.1016/s1350-4533(01)00047-9
De Castro Leao, M., Raffin Pohlmann, A., De Cristo Soares Alves, A., Helena Poliselli Farsky, S., Klimuk Uchiyama, M., Araki, K., et al. (2021). Docosahexaenoic acid nanoencapsulated with anti-PECAM-1 as co-therapy for atherosclerosis regression. Eur. J. Pharm. Biopharm. 159, 99–107. doi:10.1016/j.ejpb.2020.12.016
Decuzzi, P., and Ferrari, M. (2006). The adhesive strength of non-spherical particles mediated by specific interactions. Biomaterials 27, 5307–5314. doi:10.1016/j.biomaterials.2006.05.024
Decuzzi, P., Pasqualini, R., Arap, W., and Ferrari, M. (2009). Intravascular delivery of particulate systems: does geometry really matter? Pharm. Res. 26, 235–243. doi:10.1007/s11095-008-9697-x
Dell’Orco, D., Lundqvist, M., Cedervall, T., and Linse, S. (2012). Delivery success rate of engineered nanoparticles in the presence of the protein corona: a systems-level screening. Nanomedicine 8, 1271–1281. doi:10.1016/j.nano.2012.02.006
De Negri Atanasio, G., Ferrari, P. F., Baiao, A., Perego, P., Sarmento, B., Palombo, D., et al. (2022). Bevacizumab encapsulation into PLGA nanoparticles functionalized with immunouteroglobin-1 as an innovative delivery system for atherosclerosis. Int. J. Biol. Macromol. 221, 1618–1630. doi:10.1016/j.ijbiomac.2022.08.063
Devel, L., Almer, G., Cabella, C., Beau, F., Bernes, M., Oliva, P., et al. (2019). Biodistribution of nanostructured lipid carriers in mice atherosclerotic model. Molecules 24, 3499. doi:10.3390/molecules24193499
Di Francesco, V., Gurgone, D., Palomba, R., Ferreira, M., Catelani, T., Cervadoro, A., et al. (2020). Modulating lipoprotein transcellular transport and atherosclerotic plaque formation in ApoE(-/-) mice via nanoformulated lipid-methotrexate conjugates. ACS Appl. Mater Interfaces 12, 37943–37956. doi:10.1021/acsami.0c12202
Ding, H. M., and Ma, Y. Q. (2018). Computational approaches to cell-nanomaterial interactions: keeping balance between therapeutic efficiency and cytotoxicity. Nanoscale Horiz. 3, 6–27. doi:10.1039/c7nh00138j
Dogra, P., Butner, J. D., Chuang, Y. L., Caserta, S., Goel, S., Brinker, C. J., et al. (2019). Mathematical modeling in cancer nanomedicine: a review. Biomed. Microdevices 21, 40. doi:10.1007/s10544-019-0380-2
Doig, G., Yeoh, G. H., Timochenko, V., Rosengarten, G., Barber, T. J., and Cheung, S. C. P. (2012). Simulation of blood flow and nanoparticle transport in a stenosed carotid bifurcation and pseudo-arteriole. J. Comput. Multiph. Flows 4, 85–102. doi:10.1260/1757-482X.4.1.85
Dosta, P., Tamargo, I., Ramos, V., Kumar, S., Kang, D. W., Borros, S., et al. (2021). Delivery of anti-microRNA-712 to inflamed endothelial cells using poly(β-amino ester) nanoparticles conjugated with VCAM-1 targeting peptide. Adv. Healthc. Mater 10, e2001894. doi:10.1002/adhm.202001894
Ebrahimi, S., Vatani, P., Amani, A., and Shamloo, A. (2021). Drug delivery performance of nanocarriers based on adhesion and interaction for abdominal aortic aneurysm treatment. Int. J. Pharm. 594, 120153. doi:10.1016/j.ijpharm.2020.120153
Elbialy, N. S., Fathy, M. M., and Khalil, W. M. (2015). Doxorubicin loaded magnetic gold nanoparticles for in vivo targeted drug delivery. Int. J. Pharm. 490, 190–199. doi:10.1016/j.ijpharm.2015.05.032
Elizondo-García, M. E., Márquez-Miranda, V., Araya-Durán, I., Valencia-Gallegos, J. A., and González-Nilo, F. D. (2018). Self-assembly behavior of amphiphilic Janus dendrimers in water: a combined experimental and coarse-grained molecular dynamics simulation approach. Molecules 23, 969. doi:10.3390/molecules23040969
Erimban, S., and Daschakraborty, S. (2020). Translocation of a hydroxyl functionalized carbon dot across a lipid bilayer: an all-atom molecular dynamics simulation study. Phys. Chem. Chem. Phys. 22, 6335–6350. doi:10.1039/c9cp05999g
Farhadian, N., Kazemi, M. S., Moosavi Baigi, F., and Khalaj, M. (2022). Molecular dynamics simulation of drug delivery across the cell membrane by applying gold nanoparticle carrier: flutamide as hydrophobic and glutathione as hydrophilic drugs as the case studies. J. Mol. Graph Model. 116, 108271. doi:10.1016/j.jmgm.2022.108271
Florian, A., Ludwig, A., Rosch, S., Yildiz, H., Klumpp, S., Sechtem, U., et al. (2014). Positive effect of intravenous iron-oxide administration on left ventricular remodelling in patients with acute ST-elevation myocardial infarction - a cardiovascular magnetic resonance (CMR) study. Int. J. Cardiol. 173, 184–189. doi:10.1016/j.ijcard.2014.02.016
Frenkel, D., and Smit, B. (2023). Understanding molecular simulation: from algorithms to applications. Third Edition. Elsevier.
Gabizon, A., Ohana, P., Amitay, Y., Gorin, J., Tzemach, D., Mak, L., et al. (2021). Liposome co-encapsulation of anti-cancer agents for pharmacological optimization of nanomedicine-based combination chemotherapy. Cancer Drug Resist 4, 463–484. doi:10.20517/cdr.2020.87
Gao, P., Nicolas, J., and Ha-Duong, T. (2021). Supramolecular organization of polymer prodrug nanoparticles revealed by coarse-grained simulations. J. Am. Chem. Soc. 143, 17412–17423. doi:10.1021/jacs.1c05332
Geary, S. M., and Salem, A. K. (2014). Exploiting the tumor phenotype using biodegradable submicron carriers of chemotherapeutic drugs. Crit. Rev. Oncog. 19, 269–280. doi:10.1615/critrevoncog.2014011518
Gilbert, J., Sebastiani, F., Arteta, M. Y., Terry, A., Fornell, A., Russell, R., et al. (2024). Evolution of the structure of lipid nanoparticles for nucleic acid delivery: from in situ studies of formulation to colloidal stability. J. Colloid Interface Sci. 660, 66–76. doi:10.1016/j.jcis.2023.12.165
Girish, A., Jolly, K., Alsaadi, N., De La Fuente, M., Recchione, A., An, R., et al. (2022). Platelet-inspired intravenous nanomedicine for injury-targeted direct delivery of thrombin to augment hemostasis in coagulopathies. ACS Nano 16, 16292–16313. doi:10.1021/acsnano.2c05306
Gitter, K., and Odenbach, S. (2013). Simulation of magnetic drug targeting for a branched artery-model with non-Newtonian flow behaviour of blood. Magnetohydrodynamics 49, 541–545. doi:10.22364/mhd.49.3-4.54
Grabinski, C., Sharma, M., Maurer, E., Sulentic, C., Sankaran, R. M., and Hussain, S. (2016). The effect of shear flow on nanoparticle agglomeration and deposition in in vitro dynamic flow models. Nanotoxicology 10, 74–83. doi:10.3109/17435390.2015.1018978
Graván, P., Aguilera-Garrido, A., Marchal, J. A., Navarro-Marchal, S. A., and Galisteo-González, F. (2023). Lipid-core nanoparticles: classification, preparation methods, routes of administration and recent advances in cancer treatment. Adv. Colloid Interface Sci. 314, 102871. doi:10.1016/j.cis.2023.102871
Gravier, J., Navarro, F. P., Delmas, T., Mittler, F., Couffin, A. C., Vinet, F., et al. (2011). Lipidots: competitive organic alternative to quantum dots for in vivo fluorescence imaging. J. Biomed. Opt. 16, 096013. doi:10.1117/1.3625405
Groner, J., Tognazzi, M., Walter, M., Fleischmann, D., Mietzner, R., Ziegler, C. E., et al. (2023). Encapsulation of pioglitazone into polymer-nanoparticles for potential treatment of atherosclerotic diseases. ACS Appl. Bio Mater 6, 2111–2121. doi:10.1021/acsabm.2c01001
Gupta, S., and Biswas, P. (2018). Effect of pH on size and internal structure of poly(propylene imine) dendrimers: a molecular dynamics simulation study. J. Phys. Chem. B 122, 9250–9263. doi:10.1021/acs.jpcb.8b04653
Han, J., Zern, B. J., Shuvaev, V. V., Davies, P. F., Muro, S., and Muzykantov, V. (2012). Acute and chronic shear stress differently regulate endothelial internalization of nanocarriers targeted to platelet-endothelial cell adhesion molecule-1. ACS Nano 6, 8824–8836. doi:10.1021/nn302687n
Hancharova, M., Halicka-Stepien, K., Dupla, A., Lesiak, A., Soloducho, J., and Cabaj, J. (2024). Antimicrobial activity of metal-based nanoparticles: a mini-review. Biometals. doi:10.1007/s10534-023-00573-y
Hansen, R., Bruus, H., Callisen, T. H., and Hassager, O. (2012). Transient convection, diffusion, and adsorption in surface-based biosensors. Langmuir 28, 7557–7563. doi:10.1021/la3000763
Haroon, H. B., Hunter, A. C., Farhangrazi, Z. S., and Moghimi, S. M. (2022). A brief history of long circulating nanoparticles. Adv. Drug Deliv. Rev. 188, 114396. doi:10.1016/j.addr.2022.114396
Hasannia, M., Lamei, K., Abnous, K., Taghdisi, S. M., Nekooei, S., Nekooei, N., et al. (2023). Targeted poly(L-glutamic acid)-based hybrid peptosomes co-loaded with doxorubicin and USPIONs as a theranostic platform for metastatic breast cancer. Nanomedicine 48, 102645. doi:10.1016/j.nano.2022.102645
Hasanpour, A., Esmaeili, F., Hosseini, H., and Amani, A. (2021). Use of mPEG-PLGA nanoparticles to improve bioactivity and hemocompatibility of streptokinase: in-vitro and in-vivo studies. Mater Sci. Eng. C Mater Biol. Appl. 118, 111427. doi:10.1016/j.msec.2020.111427
Hasenkopf, I., Mills-Goodlet, R., Johnson, L., Rouse, I., Geppert, M., Duschl, A., et al. (2022). Computational prediction and experimental analysis of the nanoparticle-protein corona: showcasing an in vitro-in silico workflow providing FAIR data. Nano Today 46, 101561. doi:10.1016/j.nantod.2022.101561
Haun, J. B., Robbins, G. P., and Hammer, D. A. (2010). Engineering therapeutic nanocarriers with optimal adhesion for targeting. J. Adhesion 86, 131–159. doi:10.1080/00218460903510414
Hayashi, K., Nakamura, M., Sakamoto, W., Yogo, T., Miki, H., Ozaki, S., et al. (2013). Superparamagnetic nanoparticle clusters for cancer theranostics combining magnetic resonance imaging and hyperthermia treatment. Theranostics 3, 366–376. doi:10.7150/thno.5860
He, J., Zhang, W., Zhou, X., Xu, F., Zou, J., Zhang, Q., et al. (2023). Reactive oxygen species (ROS)-responsive size-reducible nanoassemblies for deeper atherosclerotic plaque penetration and enhanced macrophage-targeted drug delivery. Bioact. Mater 19, 115–126. doi:10.1016/j.bioactmat.2022.03.041
Heidsieck, A., Vosen, S., Zimmermann, K., Wenzel, D., and Gleich, B. (2012). Analysis of trajectories for targeting of magnetic nanoparticles in blood vessels. Mol. Pharm. 9, 2029–2038. doi:10.1021/mp3001155
Hernandes, E. P., Lazarin-Bidoia, D., Bini, R. D., Nakamura, C. V., Cotica, L. F., and De Oliveira Silva Lautenschlager, S. (2023). Doxorubicin-loaded iron oxide nanoparticles induce oxidative stress and cell cycle arrest in breast cancer cells. Antioxidants (Basel) 12, 237. doi:10.3390/antiox12020237
Hernandez, A. P., Iglesias-Anciones, L., Vaquero-Gonzalez, J. J., Pinol, R., Criado, J. J., Rodriguez, E., et al. (2023). Enhancement of tumor cell immunogenicity and antitumor properties derived from platinum-conjugated iron nanoparticles. Cancers (Basel) 15, 3204. doi:10.3390/cancers15123204
Hewlin, R. L., Ciero, A., and Kizito, J. P. (2019). Development of a two-way coupled eulerian-Lagrangian computational magnetic nanoparticle targeting model for pulsatile flow in a patient-specific diseased left carotid bifurcation artery. Cardiovasc. Eng. Technol. 10, 299–313. doi:10.1007/s13239-019-00411-8
Ho, D., Lynd, T. O., Jun, C., Shin, J., Millican, R. C., Estep, B. K., et al. (2023). MiR-146a encapsulated liposomes reduce vascular inflammatory responses through decrease of ICAM-1 expression, macrophage activation, and foam cell formation. Nanoscale 15, 3461–3474. doi:10.1039/d2nr03280e
Hong, L., Li, W., Li, Y., and Yin, S. (2023). Nanoparticle-based drug delivery systems targeting cancer cell surfaces. RSC Adv. 13, 21365–21382. doi:10.1039/d3ra02969g
Hossain, S. S., Hughes, T. J. R., and Decuzzi, P. (2014). Vascular deposition patterns for nanoparticles in an inflamed patient-specific arterial tree. Biomechanics Model. Mechanobiol. 13, 585–597. doi:10.1007/s10237-013-0520-1
Hossain, S. S., Zhang, Y. J., Liang, X. H., Hussain, F., Ferrari, M., Hughes, T. J. R., et al. (2013). In silico vascular modeling for personalized nanoparticle delivery. Nanomedicine 8, 343–357. doi:10.2217/nnm.12.124
Hotze, E. M., Phenrat, T., and Lowry, G. V. (2010). Nanoparticle aggregation: challenges to understanding transport and reactivity in the environment. J. Environ. Qual. 39, 1909–1924. doi:10.2134/jeq2009.0462
Hou, M., Wu, X., Zhao, Z., Deng, Q., Chen, Y., and Yin, L. (2022). Endothelial cell-targeting, ROS-ultrasensitive drug/siRNA co-delivery nanocomplexes mitigate early-stage neutrophil recruitment for the anti-inflammatory treatment of myocardial ischemia reperfusion injury. Acta Biomater. 143, 344–355. doi:10.1016/j.actbio.2022.02.018
Hounslow, M. J., Ryall, R. L., and Marshall, V. R. (1988). A discretized population balance for nucleation, growth, and aggregation. Aiche J. 34, 1821–1832. doi:10.1002/aic.690341108
Hu, C. M., Fang, R. H., Wang, K. C., Luk, B. T., Thamphiwatana, S., Dehaini, D., et al. (2015). Nanoparticle biointerfacing by platelet membrane cloaking. Nature 526, 118–121. doi:10.1038/nature15373
Huang, C., Zhang, Y., Su, J., Guan, X., Chen, S., Xu, X., et al. (2023). Liver-specific ionizable lipid nanoparticles mediated efficient RNA interference to clear "bad cholesterol. Int. J. Nanomedicine 18, 7785–7801. doi:10.2147/IJN.S434908
Huang, G. H., Pan, Z. D., and Wang, Y. M. (2020). Dispersion of praseodymium-doped zirconium silicate pigment in aqueous suspension by modified hydroxyl copolymer. Chem. Eng. Res. Des. 154, 86–100. doi:10.1016/j.cherd.2019.11.037
Huang, W., Wang, L., Zou, Y., Ding, X., Geng, X., Li, J., et al. (2022). Preparation of gastrodin-modified dendrimer-entrapped gold nanoparticles as a drug delivery system for cerebral ischemia-reperfusion injury. Brain Behav. 12, e2810. doi:10.1002/brb3.2810
Hussain, A., Dar, M. N. R., Kanwal, R., and Cheema, W. K. (2023). Assessment of unsteady-state thermal expansion in saccular aneurysms with Newtonian blood circulation including silver nanoparticles. Adv. Mech. Eng. 15. doi:10.1177/16878132231189109
Huwyler, J., Drewe, J., and Krahenbuhl, S. (2008). Tumor targeting using liposomal antineoplastic drugs. Int. J. Nanomedicine 3, 21–29. doi:10.2147/ijn.s1253
Inyang, E., Kuriakose, A. E., Chen, B., Nguyen, K. T., and Cho, M. (2020). Engineering delivery of nonbiologics using poly(lactic-co-glycolic acid) nanoparticles for repair of disrupted brain endothelium. ACS Omega 5, 14730–14740. doi:10.1021/acsomega.0c01517
Islam, M. A., Barua, S., and Barua, D. (2017). A multiscale modeling study of particle size effects on the tissue penetration efficacy of drug-delivery nanoparticles. BMC Syst. Biol. 11, 113. doi:10.1186/s12918-017-0491-4
Jeong, W., Kim, M. J., and Rhee, K. (2013). Computational study of particle size effects on selective binding of nanoparticles in arterial stenosis. Comput. Biol. Med. 43, 417–424. doi:10.1016/j.compbiomed.2013.02.004
Ji, X. Y., Peng, F., Zhong, Y. L., Su, Y. Y., and He, Y. (2014). Fluorescent quantum dots: synthesis, biomedical optical imaging, and biosafety assessment. Colloids Surfaces B-Biointerfaces 124, 132–139. doi:10.1016/j.colsurfb.2014.08.036
Joudeh, N., and Linke, D. (2022). Nanoparticle classification, physicochemical properties, characterization, and applications: a comprehensive review for biologists. J. Nanobiotechnology 20, 262. doi:10.1186/s12951-022-01477-8
Karnati, K. R., and Wang, Y. (2018). Understanding the co-loading and releasing of doxorubicin and paclitaxel using chitosan functionalized single-walled carbon nanotubes by molecular dynamics simulations. Phys. Chem. Chem. Phys. 20, 9389–9400. doi:10.1039/C8CP00124C
Katsuki, S., Koga, J. I., Matoba, T., Umezu, R., Nakashiro, S., Nakano, K., et al. (2022). Nanoparticle-Mediated delivery of pitavastatin to monocytes/macrophages inhibits angiotensin II-induced abdominal aortic aneurysm formation in apoe(-/-) mice. J. Atheroscler. Thromb. 29, 111–125. doi:10.5551/jat.54379
Kavyani, S., Dadvar, M., Modarress, H., and Amjad-Iranagh, S. (2018). A coarse grained molecular dynamics simulation study on the structural properties of carbon nanotube-dendrimer composites. Soft Matter 14, 3151–3163. doi:10.1039/c8sm00253c
Kennel, S. J., Woodward, J. D., Rondinone, A. J., Wall, J., Huang, Y., and Mirzadeh, S. (2008). The fate of MAb-targeted Cd(125m)Te/ZnS nanoparticles in vivo. Nucl. Med. Biol. 35, 501–514. doi:10.1016/j.nucmedbio.2008.02.001
Kheirolomoom, A., Kim, C. W., Seo, J. W., Kumar, S., Son, D. J., Gagnon, M. K., et al. (2015). Multifunctional nanoparticles facilitate molecular targeting and miRNA delivery to inhibit atherosclerosis in ApoE(-/-) mice. ACS Nano 9, 8885–8897. doi:10.1021/acsnano.5b02611
Kim, M. J., and Rhee, K. (2011). Computational analysis of nanoparticle adhesion to endothelium: effects of kinetic rate constants and wall shear rates. Med. Biol. Eng. Comput. 49, 733–741. doi:10.1007/s11517-011-0735-1
Kona, S., Dong, J. F., Liu, Y. L., Tan, J. F., and Nguyen, K. T. (2012). Biodegradable nanoparticles mimicking platelet binding as a targeted and controlled drug delivery system. Int. J. Pharm. 423, 516–524. doi:10.1016/j.ijpharm.2011.11.043
Korin, N., Kanapathipillai, M., Matthews, B. D., Crescente, M., Brill, A., Mammoto, T., et al. (2012). Shear-activated nanotherapeutics for drug targeting to obstructed blood vessels. Science 337, 738–742. doi:10.1126/science.1217815
Kornmueller, K., Vidakovic, I., and Prassl, R. (2019). Artificial high density lipoprotein nanoparticles in cardiovascular research. Molecules 24, 2829. doi:10.3390/molecules24152829
Korolija, N., Popović, J., Cvetanović, M., and Bojović, M. (2017). “Chapter three - dataflow-based parallelization of control-flow algorithms,” in Advances in computers. Editors A. R. Hurson, and V. Milutinović (Elsevier), 73–124.
Kudgus, R. A., Szabolcs, A., Khan, J. A., Walden, C. A., Reid, J. M., Robertson, J. D., et al. (2013). Inhibiting the growth of pancreatic adenocarcinoma in vitro and in vivo through targeted treatment with designer gold nanotherapeutics. PLoS One 8, e57522. doi:10.1371/journal.pone.0057522
Kumar, A., and Dhawan, A. (2013). Genotoxic and carcinogenic potential of engineered nanoparticles: an update. Arch. Toxicol. 87, 1883–1900. doi:10.1007/s00204-013-1128-z
Kumar, S., and Ramkrishna, D. (1996). On the solution of population balance equations by discretization—I. A fixed pivot technique. Chem. Eng. Sci. 51, 1311–1332. doi:10.1016/0009-2509(96)88489-2
Lai, P., Daear, W., Lobenberg, R., and Prenner, E. J. (2014). Overview of the preparation of organic polymeric nanoparticles for drug delivery based on gelatine, chitosan, poly(D,L-lactide-co-glycolic acid) and polyalkylcyanoacrylate. Colloids Surfaces B-Biointerfaces 118, 154–163. doi:10.1016/j.colsurfb.2014.03.017
Larimi, M. M., Ramiar, A., and Ranjbar, A. A. (2014). Numerical simulation of magnetic nanoparticles targeting in a bifurcation vessel. J. Magnetism Magnetic Mater. 362, 58–71. doi:10.1016/j.jmmm.2014.03.002
Lee, H., De Vries, A. H., Marrink, S. J., and Pastor, R. W. (2009). A coarse-grained model for polyethylene oxide and polyethylene glycol: conformation and hydrodynamics. J. Phys. Chem. B 113, 13186–13194. doi:10.1021/jp9058966
Lee, J. R., Park, B. W., Park, J. H., Lim, S., Kwon, S. P., Hwang, J. W., et al. (2021). Local delivery of a senolytic drug in ischemia and reperfusion-injured heart attenuates cardiac remodeling and restores impaired cardiac function. Acta Biomater. 135, 520–533. doi:10.1016/j.actbio.2021.08.028
Lee, M., Shelke, A., Singh, S., Fan, J., Zaleski, P., and Afkhami, S. (2022). Numerical simulation of superparamagnetic nanoparticle motion in blood vessels for magnetic drug delivery. Phys. Rev. E 106, 015104. doi:10.1103/PhysRevE.106.015104
Lemaalem, M., Hadrioui, N., Derouiche, A., and Ridouane, H. (2020). Structure and dynamics of liposomes designed for drug delivery: coarse-grained molecular dynamics simulations to reveal the role of lipopolymer incorporation. RSC Adv. 10, 3745–3755. doi:10.1039/c9ra08632c
Li, H., Zhu, J., Xu, Y. W., Mou, F. F., Shan, X. L., Wang, Q. L., et al. (2022). Notoginsenoside R1-loaded mesoporous silica nanoparticles targeting the site of injury through inflammatory cells improves heart repair after myocardial infarction. Redox Biol. 54, 102384. doi:10.1016/j.redox.2022.102384
Li, J., Wang, Y., Xue, S., Sun, J., Zhang, W., Hu, P., et al. (2016). Effective combination treatment of lung cancer cells by single vehicular delivery of siRNA and different anticancer drugs. Int. J. Nanomedicine 11, 4609–4624. doi:10.2147/IJN.S107345
Li, X., Xiao, H., Lin, C., Sun, W., Wu, T., Wang, J., et al. (2019). Synergistic effects of liposomes encapsulating atorvastatin calcium and curcumin and targeting dysfunctional endothelial cells in reducing atherosclerosis. Int. J. Nanomedicine 14, 649–665. doi:10.2147/IJN.S189819
Li, Z., Zhu, H., Liu, H., Liu, D., Liu, J., Jiang, J., et al. (2023). Evolocumab loaded Bio-Liposomes for efficient atherosclerosis therapy. J. Nanobiotechnology 21, 158. doi:10.1186/s12951-023-01904-4
Liang, L., Wang, Y., and Pan, Z. (2016). Prediction of aggregation behavior of submicron-sized particles of praseodymium-doped zirconium silicate in aqueous suspension by population balance model. Particuology 25, 83–92. doi:10.1016/j.partic.2015.02.009
Lin, X. B., and Gu, N. (2014). Surface properties of encapsulating hydrophobic nanoparticles regulate the main phase transition temperature of lipid bilayers: a simulation study. Nano Res. 7, 1195–1204. doi:10.1007/s12274-014-0482-3
Lindemann, M. C., Luttke, T., Nottrodt, N., Schmitz-Rode, T., and Slabu, I. (2021). FEM based simulation of magnetic drug targeting in a multibranched vessel model. Comput. Methods Programs Biomed. 210, 106354. doi:10.1016/j.cmpb.2021.106354
Liu, C. H., Hsu, H. L., Chen, J. P., Wu, T., and Ma, Y. H. (2019a). Thrombolysis induced by intravenous administration of plasminogen activator in magnetoliposomes: dual targeting by magnetic and thermal manipulation. Nanomedicine 20, 101992. doi:10.1016/j.nano.2019.03.014
Liu, D. F., Qian, C., An, Y. L., Chang, D., Ju, S. H., and Teng, G. J. (2014). Magnetic resonance imaging of post-ischemic blood-brain barrier damage with PEGylated iron oxide nanoparticles. Nanoscale 6, 15161–15167. doi:10.1039/c4nr03942d
Liu, F., Ding, N., Huo, D., Yang, G., Wei, K., Guan, G., et al. (2019b). Surface-engineered monocyte inhibits atherosclerotic plaque destabilization via graphene quantum dot-mediated MicroRNA delivery. Adv. Healthc. Mater 8, e1900386. doi:10.1002/adhm.201900386
Liu, M., Gao, T., Jiang, L., Li, S., Shi, B., and Li, F. (2023). Enhancing the biopharmaceutical attributes of atorvastatin calcium using polymeric and lipid-polymer hybrid nanoparticles: an approach for atherosclerosis treatment. Biomed. Pharmacother. 159, 114261. doi:10.1016/j.biopha.2023.114261
Liu, Y., Peng, B., Sohrabi, S., and Liu, Y. (2016). The configuration of copolymer ligands on nanoparticles affects adhesion and uptake. Langmuir 32, 10136–10143. doi:10.1021/acs.langmuir.6b02371
Liu, Z. X., Zhu, Y. Z., Rao, R. R., Clausen, J. R., and Aidun, C. K. (2018). Nanoparticle transport in cellular blood flow. Comput. Fluids 172, 609–620. doi:10.1016/j.compfluid.2018.03.022
Longest, P. W., and Kleinstreuer, C. (2003). Comparison of blood particle deposition models for non-parallel flow domains. J. Biomechanics 36, 421–430. doi:10.1016/s0021-9290(02)00434-7
Lu, X., Zhang, Y., Wang, L., Li, G., Gao, J., and Wang, Y. (2021). Development of L-carnosine functionalized iron oxide nanoparticles loaded with dexamethasone for simultaneous therapeutic potential of blood brain barrier crossing and ischemic stroke treatment. Drug Deliv. 28, 380–389. doi:10.1080/10717544.2021.1883158
Lunnoo, T., and Puangmali, T. (2015). Capture efficiency of biocompatible magnetic nanoparticles in arterial flow: a computer simulation for magnetic drug targeting. Nanoscale Res. Lett. 10, 426. doi:10.1186/s11671-015-1127-5
Luo, T., Zhang, Z., Xu, J., Liu, H., Cai, L., Huang, G., et al. (2023). Atherosclerosis treatment with nanoagent: potential targets, stimulus signals and drug delivery mechanisms. Front. Bioeng. Biotechnol. 11, 1205751. doi:10.3389/fbioe.2023.1205751
Lv, J., Zhang, L. J., Du, W. Z., Ling, G. X., and Zhang, P. (2022). Functional gold nanoparticles for diagnosis, treatment and prevention of thrombus. J. Control. Release 345, 572–585. doi:10.1016/j.jconrel.2022.03.044
Lyczkowski, R. W., Alevriadou, B. R., Horner, M., Panchal, C. B., and Shroff, S. G. (2009). Application of multiphase computational fluid dynamics to analyze monocyte adhesion. Ann. Biomed. Eng. 37, 1516–1533. doi:10.1007/s10439-009-9729-7
Ma, S., Tian, X. Y., Zhang, Y., Mu, C., Shen, H., Bismuth, J., et al. (2016). E-selectin-targeting delivery of microRNAs by microparticles ameliorates endothelial inflammation and atherosclerosis. Sci. Rep. 6, 22910. doi:10.1038/srep22910
Ma, Y. H., Wu, S. Y., Wu, T., Chang, Y. J., Hua, M. Y., and Chen, J. P. (2009). Magnetically targeted thrombolysis with recombinant tissue plasminogen activator bound to polyacrylic acid-coated nanoparticles. Biomaterials 30, 3343–3351. doi:10.1016/j.biomaterials.2009.02.034
Maher, S., Kalil, H., Liu, G., Sossey-Alaoui, K., and Bayachou, M. (2023). Alginate-based hydrogel platform embedding silver nanoparticles and cisplatin: characterization of the synergistic effect on a breast cancer cell line. Front. Mol. Biosci. 10, 1242838. doi:10.3389/fmolb.2023.1242838
Mahmoodpour, M., Goharkhah, M., and Ashjaee, M. (2020). Investigation on trajectories and capture of magnetic drug carrier nanoparticles after injection into a direct vessel. J. Magnetism Magnetic Mater. 497, 166065. doi:10.1016/j.jmmm.2019.166065
Mahmoudi, M. (2022). The need for improved methodology in protein corona analysis. Nat. Commun. 13, 49. doi:10.1038/s41467-021-27643-4
Maji, R., Omolo, C. A., Agrawal, N., Maduray, K., Hassan, D., Mokhtar, C., et al. (2019). pH-responsive lipid-dendrimer hybrid nanoparticles: an approach to target and eliminate intracellular pathogens. Mol. Pharm. 16, 4594–4609. doi:10.1021/acs.molpharmaceut.9b00713
Malode, S. J., Pandiaraj, S., Alodhayb, A., and Shetti, N. P. (2024). Carbon nanomaterials for biomedical applications: progress and outlook. ACS Appl. Bio Mater. doi:10.1021/acsabm.3c00983
Mancardi, G., Mikolajczyk, A., Annapoorani, V. K., Bahl, A., Blekos, K., Burk, J., et al. (2023). A computational view on nanomaterial intrinsic and extrinsic features for nanosafety and sustainability. Mater. Today 67, 344–370. doi:10.1016/j.mattod.2023.05.029
Manshadi, M. K. D., Saadat, M., Mohammadi, M., Shamsi, M., Dejam, M., Kamali, R., et al. (2018). Delivery of magnetic micro/nanoparticles and magnetic-based drug/cargo into arterial flow for targeted therapy. Drug Deliv. 25, 1963–1973. doi:10.1080/10717544.2018.1497106
Markhulia, J., Kekutia, S., Mikelashvili, V., Saneblidze, L., Tsertsvadze, T., Maisuradze, N., et al. (2023). Synthesis, characterization, and in vitro cytotoxicity evaluation of doxorubicin-loaded magnetite nanoparticles on triple-negative breast cancer cell lines. Pharmaceutics 15, 1758. doi:10.3390/pharmaceutics15061758
Martinez-Jothar, L., Barendrecht, A. D., De Graaff, A. M., Oliveira, S., Van Nostrum, C. F., Schiffelers, R. M., et al. (2020). Endothelial cell targeting by cRGD-functionalized polymeric nanoparticles under static and flow conditions. Nanomater. (Basel) 10, 1353. doi:10.3390/nano10071353
Martinez-Veracoechea, F. J., and Frenkel, D. (2011). Designing super selectivity in multivalent nano-particle binding. Proc. Natl. Acad. Sci. U. S. A. 108, 10963–10968. doi:10.1073/pnas.1105351108
Marukhyan, S. S., and Gasparyan, V. K. (2017). Fluorometric immunoassay for human serum albumin based on its inhibitory effect on the immunoaggregation of quantum dots with silver nanoparticles. Spectrochimica Acta Part a-Molecular Biomol. Spectrosc. 173, 34–38. doi:10.1016/j.saa.2016.08.029
Matuszak, J., Lutz, B., Sekita, A., Zaloga, J., Alexiou, C., Lyer, S., et al. (2018). Drug delivery to atherosclerotic plaques using superparamagnetic iron oxide nanoparticles. Int. J. Nanomedicine 13, 8443–8460. doi:10.2147/IJN.S179273
Menon, J. U., Jadeja, P., Tambe, P., Vu, K., Yuan, B., and Nguyen, K. T. (2013). Nanomaterials for photo-based diagnostic and therapeutic applications. Theranostics 3, 152–166. doi:10.7150/thno.5327
Mhashal, A. R., and Roy, S. (2016). Free energy of bare and capped gold nanoparticles permeating through a lipid bilayer. Chemphyschem 17, 3504–3514. doi:10.1002/cphc.201600690
Miraghaie, S. H., Zandi, A., Davari, Z., Mousavi-Kiasary, M. S., Saghafi, Z., Gilani, A., et al. (2023). Targeted delivery of anticancer drug loaded charged PLGA polymeric nanoparticles using electrostatic field. Macromol. Biosci. 23, e2300181. doi:10.1002/mabi.202300181
Mitchell, M. J., Billingsley, M. M., Haley, R. M., Wechsler, M. E., Peppas, N. A., and Langer, R. (2021). Engineering precision nanoparticles for drug delivery. Nat. Rev. Drug Discov. 20, 101–124. doi:10.1038/s41573-020-0090-8
Mollazadeh, S., Sahebkar, A., Shahlaei, M., and Moradi, S. (2021). Nano drug delivery systems: molecular dynamic simulation. J. Mol. Liq. 332, 115823. doi:10.1016/j.molliq.2021.115823
Montelione, N., Loreni, F., Nenna, A., Catanese, V., Scurto, L., Ferrisi, C., et al. (2023). Tissue engineering and targeted drug delivery in cardiovascular disease: the role of polymer nanocarrier for statin therapy. Biomedicines 11, 798. doi:10.3390/biomedicines11030798
Moradi, S., Taran, M., Mohajeri, P., Sadrjavadi, K., Sarrami, F., Karton, A., et al. (2018). Study of dual encapsulation possibility of hydrophobic and hydrophilic drugs into a nanocarrier based on bio-polymer coated graphene oxide using density functional theory, molecular dynamics simulation and experimental methods. J. Mol. Liq. 262, 204–217. doi:10.1016/j.molliq.2018.04.089
Moreno-Alcantar, G., Picchetti, P., and Casini, A. (2023). Gold complexes in anticancer therapy: from new design principles to particle-based delivery systems. Angew. Chem. Int. Ed. Engl. 62, e202218000. doi:10.1002/anie.202218000
Moros, M., Mitchell, S. G., Grazu, V., and De La Fuente, J. M. (2013). The fate of nanocarriers as nanomedicines in vivo: important considerations and biological barriers to overcome. Curr. Med. Chem. 20, 2759–2778. doi:10.2174/0929867311320220003
Morshed, R. A., Muroski, M. E., Dai, Q., Wegscheid, M. L., Auffinger, B., Yu, D., et al. (2016). Cell-penetrating peptide-modified gold nanoparticles for the delivery of doxorubicin to brain metastatic breast cancer. Mol. Pharm. 13, 1843–1854. doi:10.1021/acs.molpharmaceut.6b00004
Muller, R. H., Shegokar, R., and Keck, C. M. (2011). 20 years of lipid nanoparticles (SLN and NLC): present state of development and industrial applications. Curr. Drug Discov. Technol. 8, 207–227. doi:10.2174/157016311796799062
Mura, S., and Couvreur, P. (2012). Nanotheranostics for personalized medicine. Adv. Drug Deliv. Rev. 64, 1394–1416. doi:10.1016/j.addr.2012.06.006
Nagaoka, K., Matoba, T., Mao, Y., Nakano, Y., Ikeda, G., Egusa, S., et al. (2015). A new therapeutic modality for acute myocardial infarction: nanoparticle-mediated delivery of pitavastatin induces cardioprotection from ischemia-reperfusion injury via activation of PI3K/akt pathway and anti-inflammation in a rat model. PLoS One 10, e0132451. doi:10.1371/journal.pone.0132451
Nakamura, Y., Mochida, A., Choyke, P. L., and Kobayashi, H. (2016). Nanodrug delivery: is the enhanced permeability and retention effect sufficient for curing cancer? Bioconjugate Chem. 27, 2225–2238. doi:10.1021/acs.bioconjchem.6b00437
Nakashiro, S., Matoba, T., Umezu, R., Koga, J., Tokutome, M., Katsuki, S., et al. (2016). Pioglitazone-incorporated nanoparticles prevent plaque destabilization and rupture by regulating monocyte/macrophage differentiation in ApoE-/- mice. Arterioscler. Thromb. Vasc. Biol. 36, 491–500. doi:10.1161/ATVBAHA.115.307057
Nasra, S., Bhatia, D., and Kumar, A. (2022). Recent advances in nanoparticle-based drug delivery systems for rheumatoid arthritis treatment. Nanoscale Adv. 4, 3479–3494. doi:10.1039/d2na00229a
Nenna, A., Nappi, F., Larobina, D., Verghi, E., Chello, M., and Ambrosio, L. (2021). Polymers and nanoparticles for statin delivery: current use and future perspectives in cardiovascular disease. Polym. (Basel) 13, 711. doi:10.3390/polym13050711
Neofytou, P. (2004). Comparison of blood rheological models for physiological flow simulation. Biorheology 41, 693–714.
Neofytou, P., Theodosiou, M., Krokidis, M. G., and Efthimiadou, E. K. (2022). Simulation of colloidal stability and aggregation tendency of magnetic nanoflowers in biofluids. Modelling 3, 14–26. doi:10.3390/modelling3010002
Neofytou, P., and Tsangaris, S. (2006). Flow effects of blood constitutive equations in 3D models of vascular anomalies. Int. J. Numer. Methods Fluids 51, 489–510. doi:10.1002/fld.1124
Ngema, L. M., Adeyemi, S. A., Marimuthu, T., Ubanako, P. N., Ngwa, W., and Choonara, Y. E. (2023). Surface immobilization of anti-VEGF peptide on SPIONs for antiangiogenic and targeted delivery of paclitaxel in non-small-cell lung carcinoma. ACS Appl. Bio Mater 6, 2747–2759. doi:10.1021/acsabm.3c00224
Nguyen, K. L., Yoshida, T., Kathuria-Prakash, N., Zaki, I. H., Varallyay, C. G., Semple, S. I., et al. (2019). Multicenter safety and practice for off-label diagnostic use of ferumoxytol in MRI. Radiology 293, 554–564. doi:10.1148/radiol.2019190477
Nobelprize (2013). Prize announcement. Nobel prize outreach AB. Available at: https://www.nobelprize.org/prizes/chemistry/2013/prize-announcement (Accessed December 11, 2023).
Otto, D. P., and De Villiers, M. M. (2018). All-atomistic molecular dynamics (AA-MD) studies and pharmacokinetic performance of PAMAM-dendrimer-furosemide delivery systems. Int. J. Pharm. 547, 545–555. doi:10.1016/j.ijpharm.2018.06.033
Patronis, A., Richardson, R. A., Schmieschek, S., Wylie, B. J. N., Nash, R. W., and Coveney, P. V. (2018). Modeling patient-specific magnetic drug targeting within the intracranial vasculature. Front. Physiology 9, 331. doi:10.3389/fphys.2018.00331
Pilou, M., Antonopoulos, V., Makris, E., Neofytou, P., Tsangaris, S., and Housiadas, C. (2013). A fully Eulerian approach to particle inertial deposition in a physiologically realistic bifurcation. Appl. Math. Model. 37, 5591–5605. doi:10.1016/j.apm.2012.10.055
Pilou, M., Skiadopoulos, A., and Neofytou, P. (2021). “Particle adhesion on a patient specific abdominal aorta aneurysm,” in 21st IEEE International Conference on BioInformatics and BioEngineering, Kragujevac, Serbia, 25-27 October 2021.
Pilou, M., Tsangaris, S., Neofytou, P., Housiadas, C., and Drossinos, Y. (2011). Inertial particle deposition in a 90° laminar flow bend: an eulerian fluid particle approach. Aerosol Sci. Technol. 45, 1376–1387. doi:10.1080/02786826.2011.596171
Poonam, , Sharma, B. K., Kumawat, C., and Vafai, K. (2022). Computational biomedical simulations of hybrid nanoparticles (Au-Al2O3/blood-mediated) transport in a stenosed and aneurysmal curved artery with heat and mass transfer: hematocrit dependent viscosity approach. Chem. Phys. Lett. 800, 139666. doi:10.1016/j.cplett.2022.139666
Prazeres, P., Ferreira, H., Costa, P. a.C., Da Silva, W., Alves, M. T., Padilla, M., et al. (2023). Delivery of plasmid DNA by ionizable lipid nanoparticles to induce CAR expression in T cells. Int. J. Nanomedicine 18, 5891–5904. doi:10.2147/IJN.S424723
Puri, A., Loomis, K., Smith, B., Lee, J. H., Yavlovich, A., Heldman, E., et al. (2009). Lipid-based nanoparticles as pharmaceutical drug carriers: from concepts to clinic. Crit. Rev. Ther. Drug Carr. Syst. 26, 523–580. doi:10.1615/critrevtherdrugcarriersyst.v26.i6.10
Qiao, Y. H., Wang, Y., Chen, Y. L., Luo, K., and Fan, J. R. (2022). Mathematical modeling of shear-activated targeted nanoparticle drug delivery for the treatment of aortic diseases. Biomechanics Model. Mechanobiol. 21, 221–230. doi:10.1007/s10237-021-01530-9
Quinones, J., Miranda-Castro, F. C., Encinas-Basurto, D., Ibarra, J., Moran-Palacio, E. F., Zamora-Alvarez, L. A., et al. (2023). Gold nanorods with mesoporous silica shell: a promising platform for cisplatin delivery. Micromachines (Basel) 14, 1031. doi:10.3390/mi14051031
Ren, J., Xu, Q., Chen, X., Li, W., Guo, K., Zhao, Y., et al. (2017). Superaligned carbon nanotubes guide oriented cell growth and promote electrophysiological homogeneity for synthetic cardiac tissues. Adv. Mater 29. doi:10.1002/adma.201702713
Reyes-Esteves, S., Nong, J., Glassman, P. M., Omo-Lamai, S., Ohashi, S., Myerson, J. W., et al. (2023). Targeted drug delivery to the brain endothelium dominates over passive delivery via vascular leak in experimental intracerebral hemorrhage. J. Control Release 356, 185–195. doi:10.1016/j.jconrel.2023.02.037
Rizvi, S. a.A., and Saleh, A. M. (2018). Applications of nanoparticle systems in drug delivery technology. Saudi Pharm. J. 26, 64–70. doi:10.1016/j.jsps.2017.10.012
Roa-Barrantes, L. M., and Patarroyo, D. J. R. (2022). Magnetic field effect on the magnetic nanoparticles trajectories in pulsating blood flow: a computational model. Bionanoscience 12, 571–581. doi:10.1007/s12668-022-00949-3
Rouse, I., and Lobaskin, V. (2021). A hard-sphere model of protein corona formation on spherical and cylindrical nanoparticles. Biophysical J. 120, 4457–4471. doi:10.1016/j.bpj.2021.09.002
Rouzet, F., Bachelet-Violette, L., Alsac, J. M., Suzuki, M., Meulemans, A., Louedec, L., et al. (2011). Radiolabeled fucoidan as a p-selectin targeting agent for in vivo imaging of platelet-rich thrombus and endothelial activation. J. Nucl. Med. 52, 1433–1440. doi:10.2967/jnumed.110.085852
Runkana, V., Somasundaran, P., and Kapur, P. C. (2004). Mathematical modeling of polymer-induced flocculation by charge neutralization. J. Colloid Interface Sci. 270, 347–358. doi:10.1016/j.jcis.2003.08.076
Rurik, J. G., Tombacz, I., Yadegari, A., Mendez Fernandez, P. O., Shewale, S. V., Li, L., et al. (2022). CAR T cells produced in vivo to treat cardiac injury. Science 375, 91–96. doi:10.1126/science.abm0594
Sadat, U., Howarth, S. P., Usman, A., Tang, T. Y., Graves, M. J., and Gillard, J. H. (2013). Sequential imaging of asymptomatic carotid atheroma using ultrasmall superparamagnetic iron oxide-enhanced magnetic resonance imaging: a feasibility study. J. Stroke Cerebrovasc. Dis. 22, e271–e276. doi:10.1016/j.jstrokecerebrovasdis.2012.06.015
Sahoo, A. K., Kanchi, S., Mandal, T., Dasgupta, C., and Maiti, P. K. (2018). Translocation of bioactive molecules through carbon nanotubes embedded in the lipid membrane. ACS Appl. Mater. Interfaces 10, 6168–6179. doi:10.1021/acsami.7b18498
Sanchez-Gaytan, B. L., Fay, F., Lobatto, M. E., Tang, J., Ouimet, M., Kim, Y., et al. (2015). HDL-mimetic PLGA nanoparticle to target atherosclerosis plaque macrophages. Bioconjug Chem. 26, 443–451. doi:10.1021/bc500517k
Shamloo, A., Amani, A., Forouzandehmehr, M., and Ghoytasi, I. (2019). In silico study of patient-specific magnetic drug targeting for a coronary LAD atherosclerotic plaque. Int. J. Pharm. 559, 113–129. doi:10.1016/j.ijpharm.2018.12.088
Shamloo, A., and Forouzandehmehr, M. (2019). Personalised deposition maps for micro- and nanoparticles targeting an atherosclerotic plaque: attributions to the receptor-mediated adsorption on the inflamed endothelial cells. Biomechanics Model. Mechanobiol. 18, 813–828. doi:10.1007/s10237-018-01116-y
Sharma, M., Alessandro, P., Cheriyamundath, S., and Lopus, M. (2024). Therapeutic and diagnostic applications of carbon nanotubes in cancer: recent advances and challenges. J. Drug Target, 1–13. doi:10.1080/1061186X.2024.2309575
Sharma, S., Katiyar, V. K., and Singh, U. (2015). Mathematical modelling for trajectories of magnetic nanoparticles in a blood vessel under magnetic field. J. Magnetism Magnetic Mater. 379, 102–107. doi:10.1016/j.jmmm.2014.12.012
Shazri, S., and Idres, M. (2017). Numerical investigation of magnetic nanoparticles trajectories for magnetic drug targeting. IOP Conf. Ser. Mater. Sci. Eng. 184, 012061. doi:10.1088/1757-899X/184/1/012061
Shetake, N. G., Ali, M., Kumar, A., Bellare, J., and Pandey, B. N. (2022). Theranostic magnetic nanoparticles enhance DNA damage and mitigate doxorubicin-induced cardio-toxicity for effective multi-modal tumor therapy. Biomater. Adv. 142, 213147. doi:10.1016/j.bioadv.2022.213147
Shi, X. H., Bussche, A. V. D., Hurt, R. H., Kane, A. B., and Gao, H. J. (2011). Cell entry of one-dimensional nanomaterials occurs by tip recognition and rotation. Nat. Nanotechnol. 6, 714–719. doi:10.1038/nnano.2011.151
Shim, M. K., Yang, S., Park, J., Yoon, J. S., Kim, J., Moon, Y., et al. (2022). Preclinical development of carrier-free prodrug nanoparticles for enhanced antitumor therapeutic potential with less toxicity. J. Nanobiotechnology 20, 436. doi:10.1186/s12951-022-01644-x
Singh, N., Sen Gupta, R., and Bose, S. (2024). A comprehensive review on singlet oxygen generation in nanomaterials and conjugated polymers for photodynamic therapy in the treatment of cancer. Nanoscale. doi:10.1039/d3nr05801h
Skiadopoulos, A., Neofytou, P., and Housiadas, C. (2017). Comparison of blood rheological models in patient specific cardiovascular system simulations. J. Hydrodynamics 29, 293–304. doi:10.1016/s1001-6058(16)60739-4
Song, B., Yuan, H., Pham, S. V., Jameson, C. J., and Murad, S. (2012). Nanoparticle permeation induces water penetration, ion transport, and lipid flip-flop. Langmuir 28, 16989–17000. doi:10.1021/la302879r
Song, K., Tang, Z., Song, Z., Meng, S., Yang, X., Guo, H., et al. (2022a). Hyaluronic acid-functionalized mesoporous silica nanoparticles loading simvastatin for targeted therapy of atherosclerosis. Pharmaceutics 14, 1265. doi:10.3390/pharmaceutics14061265
Song, P. P., Han, X. Q., Zheng, R. X., Yan, J., Wu, X. Q., Wang, Y. J., et al. (2022b). Upregulation of MHC-I and downregulation of PD-L1 expression by doxorubicin and deferasirox codelivered liposomal nanoparticles for chemoimmunotherapy of melanoma. Int. J. Pharm. 624, 122002. doi:10.1016/j.ijpharm.2022.122002
Spivak, M. Y., Bubnov, R. V., Yemets, I. M., Lazarenko, L. M., Tymoshok, N. O., and Ulberg, Z. R. (2013). Development and testing of gold nanoparticles for drug delivery and treatment of heart failure: a theranostic potential for PPP cardiology. EPMA J. 4, 20. doi:10.1186/1878-5085-4-20
Starodumov, I. O., Sokolov, S. Y., Blyakhman, F. S., Zubarev, A. Y., Fedotov, S. P., and Alexandrov, D. V. (2023). In silico study of magnetic nanoparticles transport in channels of various diameters in the presence of a constant magnetic field. Eur. Phys. Journal-Special Top. 232, 1207–1217. doi:10.1140/epjs/s11734-023-00859-9
Subbotina, J., Rouse, I., and Lobaskin, V. (2023). In silico prediction of protein binding affinities onto core–shell PEGylated noble metal nanoparticles for rational design of drug nanocarriers. Nanoscale 15, 13371–13383. doi:10.1039/d3nr03264g
Sun, G., and Mao, J. J. (2012). Engineering dextran-based scaffolds for drug delivery and tissue repair. Nanomedicine (Lond) 7, 1771–1784. doi:10.2217/nnm.12.149
Sun, M., Hao Pontius, M. H., Yang, S., Pendekanti, T., Raghunathan, S., Shavit, J. A., et al. (2023). Direct delivery of plasmin using clot-anchoring thrombin-responsive nanoparticles for targeted fibrinolytic therapy. J. Thromb. Haemost. 21, 983–994. doi:10.1016/j.jtha.2022.11.037
Sun, M., Miyazawa, K., Pendekanti, T., Razmi, A., Firlar, E., Yang, S., et al. (2020). Combination targeting of 'platelets + fibrin' enhances clot anchorage efficiency of nanoparticles for vascular drug delivery. Nanoscale 12, 21255–21270. doi:10.1039/d0nr03633a
Suo, J., Tong, S., Mcdaniel, M., Samady, H., Taylor, W. T., Βao, G., et al. (2012). “Numerical simulation of magnetic nanoparticles targeted at an atherosclerotic lesion in the left coronary artery of patient,” in ASME 2012 Summer Bioengineering Conference, Fajardo, Puerto Rico, USA, June 20–23, 2012.
Tadayon, A., Jamshidi, R., and Esmaeili, A. (2015). Delivery of tissue plasminogen activator and streptokinase magnetic nanoparticles to target vascular diseases. Int. J. Pharm. 495, 428–438. doi:10.1016/j.ijpharm.2015.09.008
Tan, J. F., Keller, W., Sohrabi, S., Yang, J., and Liu, Y. L. (2016). Characterization of nanoparticle dispersion in red blood cell suspension by the lattice Boltzmann-immersed boundary method. Nanomaterials 6, 30. doi:10.3390/nano6020030
Tang, T. Y., Muller, K. H., Graves, M. J., Li, Z. Y., Walsh, S. R., Young, V., et al. (2009). Iron oxide particles for atheroma imaging. Arterioscler. Thromb. Vasc. Biol. 29, 1001–1008. doi:10.1161/ATVBAHA.108.165514
Tassa, C., Shaw, S. Y., and Weissleder, R. (2011). Dextran-coated iron oxide nanoparticles: a versatile platform for targeted molecular imaging, molecular diagnostics, and therapy. Acc. Chem. Res. 44, 842–852. doi:10.1021/ar200084x
Tavanti, F., and Menziani, M. C. (2015). A closer look into the ubiquitin corona on gold nanoparticles by computational studies. New J. Chem. 39, 2474–2482. doi:10.1039/c4nj01752h
Tietze, R., Lyer, S., Durr, S., Struffert, T., Engelhorn, T., Schwarz, M., et al. (2013). Efficient drug-delivery using magnetic nanoparticles--biodistribution and therapeutic effects in tumour bearing rabbits. Nanomedicine 9, 961–971. doi:10.1016/j.nano.2013.05.001
Tietze, R., Zaloga, J., Unterweger, H., Lyer, S., Friedrich, R. P., Janko, C., et al. (2015). Magnetic nanoparticle-based drug delivery for cancer therapy. Biochem. Biophys. Res. Commun. 468, 463–470. doi:10.1016/j.bbrc.2015.08.022
Todaro, B., Moscardini, A., and Luin, S. (2022). Pioglitazone-loaded PLGA nanoparticles: towards the most reliable synthesis method. Int. J. Mol. Sci. 23, 2522. doi:10.3390/ijms23052522
Tokarev, A. A., Butylin, A. A., and Ataullakhanov, F. I. (2011). Platelet adhesion from shear blood flow is controlled by near-wall rebounding collisions with erythrocytes. Biophysical J. 100, 799–808. doi:10.1016/j.bpj.2010.12.3740
Tripathi, J., Vasu, B., Bég, O. A., Gorla, R. S. R., and Kameswaran, P. K. (2021). Computational simulation of rheological blood flow containing hybrid nanoparticles in an inclined catheterized artery with stenotic, aneurysmal and slip effects. Comput. Biol. Med. 139, 105009. doi:10.1016/j.compbiomed.2021.105009
Trouki, C., Barcaro, G., and Monti, S. (2022). Exploring the mechanisms of drug-delivery by decorated ZnO nanoparticles through predictive ReaxFF molecular dynamics simulations. Nanoscale 14, 13123–13131. doi:10.1039/d2nr03941a
Tsimpoukis, A., Cicha, I., and Neofytou, P. (2023). “Simulation of medical nanoparticle deposition in an in-vitro setup,” in 19th Multiphase Flow Workshop - Conference and Short Course, Dresden, June 19 - 23, 2023.
Unterweger, H., Tietze, R., Janko, C., Zaloga, J., Lyer, S., Durr, S., et al. (2014). Development and characterization of magnetic iron oxide nanoparticles with a cisplatin-bearing polymer coating for targeted drug delivery. Int. J. Nanomedicine 9, 3659–3676. doi:10.2147/IJN.S63433
Van Der Meel, R., Vehmeijer, L. J., Kok, R. J., Storm, G., and Van Gaal, E. V. (2013). Ligand-targeted particulate nanomedicines undergoing clinical evaluation: current status. Adv. Drug Deliv. Rev. 65, 1284–1298. doi:10.1016/j.addr.2013.08.012
Van Der Valk, F. M., Van Wijk, D. F., Lobatto, M. E., Verberne, H. J., Storm, G., Willems, M. C., et al. (2015). Prednisolone-containing liposomes accumulate in human atherosclerotic macrophages upon intravenous administration. Nanomedicine 11, 1039–1046. doi:10.1016/j.nano.2015.02.021
Vangijzegem, T., Lecomte, V., Ternad, I., Van Leuven, L., Muller, R. N., Stanicki, D., et al. (2023). Superparamagnetic iron oxide nanoparticles (SPION): from fundamentals to state-of-the-art innovative applications for cancer therapy. Pharmaceutics 15, 236. doi:10.3390/pharmaceutics15010236
Von Smoluchowski, M. (1917). Versuch einer mathematischen Theorie der Koagulationskinetic kolloider Losungen. Z. Phys. Chem. 92, 129–168. doi:10.1515/zpch-1918-9209
Wahajuddin, , and Arora, S. (2012). Superparamagnetic iron oxide nanoparticles: magnetic nanoplatforms as drug carriers. Int. J. Nanomedicine 7, 3445–3471. doi:10.2147/IJN.S30320
Wang, K., Yu, C., Liu, Y., Zhang, W., Sun, Y., and Chen, Y. (2018). Enhanced antiatherosclerotic efficacy of statin-loaded reconstituted high-density lipoprotein via ganglioside GM1 modification. ACS Biomater. Sci. Eng. 4, 952–962. doi:10.1021/acsbiomaterials.7b00871
Wang, L., Wang, J., Hao, J., Dong, Z., Wu, J., Shen, G., et al. (2021a). Guiding drug through interrupted bloodstream for potentiated thrombolysis by C-shaped magnetic actuation system in vivo. Adv. Mater 33, e2105351. doi:10.1002/adma.202105351
Wang, Q., Wang, Y., Liu, S., Sha, X., Song, X., Dai, Y., et al. (2021b). Theranostic nanoplatform to target macrophages enables the inhibition of atherosclerosis progression and fluorescence imaging of plaque in ApoE(-/-) mice. J. Nanobiotechnology 19, 222. doi:10.1186/s12951-021-00962-w
Wang, T., Zhou, T., Xu, M., Wang, S., Wu, A., Zhang, M., et al. (2022). Platelet membrane-camouflaged nanoparticles carry microRNA inhibitor against myocardial ischaemia‒reperfusion injury. J. Nanobiotechnology 20, 434. doi:10.1186/s12951-022-01639-8
Wang, X., Song, Y., Yu, L., Xue, X., Pang, M., Li, Y., et al. (2023a). Co-delivery of hesperetin and cisplatin via hyaluronic acid-modified liposome for targeted inhibition of aggression and metastasis of triple-negative breast cancer. ACS Appl. Mater Interfaces 15, 34360–34377. doi:10.1021/acsami.3c03233
Wang, Y., Jian, C., Long, Y., Xu, X., Song, Y., and Yin, Z. (2023b). H(2)O(2)-triggered "off/on signal" nanoparticles target P-selectin for the non-invasive and contrast-enhanced theranostics for arterial thrombosis. Acta Biomater. 158, 769–781. doi:10.1016/j.actbio.2022.12.026
Weller, F. F. (2008). Platelet deposition in non-parallel flow: influence of shear stress and changes in surface reactivity. J. Math. Biol. 57, 333–359. doi:10.1007/s00285-008-0163-5
Wilhelm, S., Tavares, A. J., Dai, Q., Ohta, S., Audet, J., Dvorak, H. F., et al. (2016). Analysis of nanoparticle delivery to tumours. Nat. Rev. Mater. 1, 16014. doi:10.1038/natrevmats.2016.14
Wu, J. (2021). The enhanced permeability and retention (EPR) effect: the significance of the concept and methods to enhance its application. J. Pers. Med. 11, 771. doi:10.3390/jpm11080771
Wu, J., Liao, S., Hu, Q., Wu, S., Qiu, S., Cheng, G., et al. (2021). Effects of liposomal simvastatin nanoparticles on vascular endothelial function and arterial smooth muscle cell apoptosis in rats with arteriosclerotic occlusive disease of lower limb via P38 mitogen-activated protein kinase nuclear factor kappa-B pathway. J. Nanosci. Nanotechnol. 21, 1169–1175. doi:10.1166/jnn.2021.18632
Xia, Q. Y., Li, H. X., and Xiao, K. (2016). Factors affecting the pharmacokinetics, biodistribution and toxicity of gold nanoparticles in drug delivery. Curr. Drug Metab. 17, 849–861. doi:10.2174/1389200217666160629114941
Xie, Y. Z., Ren, Z. H., Chen, H. Y., Tang, H., Zhu, M., Lv, Z., et al. (2023). A novel estrogen-targeted PEGylated liposome co-delivery oxaliplatin and paclitaxel for the treatment of ovarian cancer. Biomed. Pharmacother. 160, 114304. doi:10.1016/j.biopha.2023.114304
Xu, Z. L., and Kleinstreuer, C. (2018). Direct nanodrug delivery for tumor targeting subject to shear-augmented diffusion in blood flow. Med. Biol. Eng. Comput. 56, 1949–1958. doi:10.1007/s11517-018-1818-z
Yang, F., Xue, J., Wang, G., and Diao, Q. (2022). Nanoparticle-based drug delivery systems for the treatment of cardiovascular diseases. Front. Pharmacol. 13. doi:10.3389/fphar.2022.999404
Yang, H., Xiong, X., Zhang, L., Wu, C., and Liu, Y. (2011). Adhesion of bio-functionalized ultrasound microbubbles to endothelial cells by targeting to vascular cell adhesion molecule-1 under shear flow. Int. J. Nanomedicine 6, 2043–2051. doi:10.2147/IJN.S24808
Yang, H., Zhao, F., Li, Y., Xu, M., Li, L., Wu, C., et al. (2013). VCAM-1-targeted core/shell nanoparticles for selective adhesion and delivery to endothelial cells with lipopolysaccharide-induced inflammation under shear flow and cellular magnetic resonance imaging in vitro. Int. J. Nanomedicine 8, 1897–1906. doi:10.2147/IJN.S44997
Ye, H. L., Shen, Z. Q., and Li, Y. (2019a). Shape-dependent transport of microparticles in blood flow: from margination to adhesion. J. Eng. Mech. 145. doi:10.1061/(asce)em.1943-7889.0001597
Ye, M., Zhou, J., Zhong, Y., Xu, J., Hou, J., Wang, X., et al. (2019b). SR-A-Targeted phase-transition nanoparticles for the detection and treatment of atherosclerotic vulnerable plaques. ACS Appl. Mater Interfaces 11, 9702–9715. doi:10.1021/acsami.8b18190
Younis, N. K., Ghoubaira, J. A., Bassil, E. P., Tantawi, H. N., and Eid, A. H. (2021). Metal-based nanoparticles: promising tools for the management of cardiovascular diseases. Nanomedicine 36, 102433. doi:10.1016/j.nano.2021.102433
Yu, J., Ju, Y., Zhao, L., Chu, X., Yang, W., Tian, Y., et al. (2015). Multistimuli-regulated photochemothermal cancer therapy remotely controlled via Fe5C2 nanoparticles. ACS Nano 10, 159–169. doi:10.1021/acsnano.5b04706
Yun, X., Maximov, V. D., Yu, J., Zhu, H., Vertegel, A. A., and Kindy, M. S. (2013). Nanoparticles for targeted delivery of antioxidant enzymes to the brain after cerebral ischemia and reperfusion injury. J. Cereb. Blood Flow. Metab. 33, 583–592. doi:10.1038/jcbfm.2012.209
Zhang, D., Liu, L., Wang, J., Zhang, H., Zhang, Z., Xing, G., et al. (2022a). Drug-loaded PEG-PLGA nanoparticles for cancer treatment. Front. Pharmacol. 13, 990505. doi:10.3389/fphar.2022.990505
Zhang, H., Qu, H., He, Q., Gao, L., Zhang, H., Wang, Y., et al. (2021). Thrombus-targeted nanoparticles for thrombin-triggered thrombolysis and local inflammatory microenvironment regulation. J. Control Release 339, 195–207. doi:10.1016/j.jconrel.2021.06.043
Zhang, N., Li, J., Gao, W., Zhu, W., Yan, J., He, Z., et al. (2022b). Co-delivery of doxorubicin and anti-PD-L1 peptide in lipid/PLGA nanocomplexes for the chemo-immunotherapy of cancer. Mol. Pharm. 19, 3439–3449. doi:10.1021/acs.molpharmaceut.2c00611
Zhang, W. W., Xu, Y. G., Shi, X. Q., Huang, X. F., Chen, R., Xu, H. P., et al. (2022c). Nanoparticle albumin-bound paclitaxel is superior to liposomal paclitaxel in the neoadjuvant treatment of breast cancer. Nanomedicine 17, 683–694. doi:10.2217/nnm-2022-0025
Zhang, X., Teodoro, J. G., and Nadeau, J. L. (2015). Intratumoral gold-doxorubicin is effective in treating melanoma in mice. Nanomedicine 11, 1365–1375. doi:10.1016/j.nano.2015.04.001
Zhang, Y., Li, W., Ou, L., Wang, W., Delyagina, E., Lux, C., et al. (2012). Targeted delivery of human VEGF gene via complexes of magnetic nanoparticle-adenoviral vectors enhanced cardiac regeneration. PLoS One 7, e39490. doi:10.1371/journal.pone.0039490
Zhao, Y., Xie, R., Yodsanit, N., Ye, M., Wang, Y., and Gong, S. (2020). Biomimetic fibrin-targeted and H(2)O(2)-responsive nanocarriers for thrombus therapy. Nano Today 35, 100986. doi:10.1016/j.nantod.2020.100986
Zheng, K. H., Schoormans, J., Stiekema, L. C. A., Calcagno, C., Cicha, I., Alexiou, C., et al. (2019). Plaque permeability assessed with DCE-MRI associates with USPIO uptake in patients with peripheral artery disease. JACC Cardiovasc Imaging 12, 2081–2083. doi:10.1016/j.jcmg.2019.04.014
Zhou, J., Guo, D., Zhang, Y., Wu, W., Ran, H., and Wang, Z. (2014). Construction and evaluation of Fe₃O₄-based PLGA nanoparticles carrying rtPA used in the detection of thrombosis and in targeted thrombolysis. ACS Appl. Mater Interfaces 6, 5566–5576. doi:10.1021/am406008k
Zhu, Y., Xu, Y., Han, D., Zhang, X., Qin, C., Liu, J., et al. (2023). Scavenger receptor-AI targeted theranostic nanoparticles for regression of atherosclerotic plaques via ABCA1 modulation. Nanomedicine 50, 102672. doi:10.1016/j.nano.2023.102672
Zolghadr, A. R., and Moosavi, S. S. (2019). Interactions of neutral gold nanoparticles with DPPC and POPC lipid bilayers: simulation and experiment. RSC Adv. 9, 5197–5205. doi:10.1039/c8ra06777e
Zolnik, B. S., Gonzalez-Fernandez, A., Sadrieh, N., and Dobrovolskaia, M. A. (2010). Nanoparticles and the immune system. Endocrinology 151, 458–465. doi:10.1210/en.2009-1082
Keywords: drug delivery nanocarriers, atomistic molecular modelling, coarse-grained molecular modeling, nanoparticle protein corona, fluid-nanocarrier interaction modelling, computational fluid-particle dynamics
Citation: Buchete N-V, Cicha I, Dutta S and Neofytou P (2024) Multiscale physics-based in silico modelling of nanocarrier-assisted intravascular drug delivery. Front. Drug Deliv. 4:1362660. doi: 10.3389/fddev.2024.1362660
Received: 28 December 2023; Accepted: 08 February 2024;
Published: 04 March 2024.
Edited by:
Surya Nauli, University of California, Irvine, United StatesReviewed by:
Hanan Kassab, University of Baghdad, IraqGuankui Wang, SalioGen Therapeutics, United States
Muhammad Imran Sajid, University of Central Punjab, Pakistan
Copyright © 2024 Buchete, Cicha, Dutta and Neofytou. This is an open-access article distributed under the terms of the Creative Commons Attribution License (CC BY). The use, distribution or reproduction in other forums is permitted, provided the original author(s) and the copyright owner(s) are credited and that the original publication in this journal is cited, in accordance with accepted academic practice. No use, distribution or reproduction is permitted which does not comply with these terms.
*Correspondence: Nicolae-Viorel Buchete, bmljb2xhZS12aW9yZWwuYnVjaGV0ZUB1Y2QuaWU=; Iwona Cicha, SXdvbmEuQ2ljaGFAdWstZXJsYW5nZW4uZGU=; Panagiotis Neofytou, cGFub3NuQGlwdGEuZGVtb2tyaXRvcy5ncg==