- 1Department of Biochemistry and Molecular Biology, University of Miami School of Medicine, Miami, FL, United States
- 2Institute of Physiotherapy and Health Sciences, The Jerzy Kukuczka Academy of Physical Education, Katowice, Poland
Drug delivery across the blood–brain barrier (BBB) has several challenges, especially toward targeting neurological diseases, due to tight and selective barrier function of the BBB. Several structural and functional components of this barrier contribute to restricting drug entry, such as interendothelial tight junctions (TJs), efflux transporters, drug-metabolizing enzymes, and crosstalk between the cells of the neurovascular unit. Among different strategies to overcome BBB resistance to therapeutic drug delivery, the use of extracellular vesicles (EVs) gained attention in recent years. This review discusses the BBB structural and functional resistance, as well as potential avenues to overcome this challenge using EVs as drug delivery vehicles into the brain.
1 The Blood–Brain Barrier
The blood–brain barrier (BBB) represents a microvascular interface between the circulatory system and the extracellular space of the brain (Toborek et al., 2005; Palmer and Alavijeh 2013; Osborne et al., 2020; Naranjo et al. 2021). The primary roles of this physical and metabolic entity are regulating central nervous system (CNS) homeostasis and providing the brain with a unique protection against endogenous and foreign agents (Weiss et al., 2009). Multiple cell types are interconnected at the endothelial cell lining of brain microvessels, which represent the anatomical site of the BBB. In terms of length, the BBB is the largest brain barrier, measuring approximately 650 km, and a surface of 10–20 m2. Certain substances such as water, oxygen, and small lipids from the bloodstream cross the BBB by transcellular pathways or a paracellular pathway. The transcellular pathway includes a variety of mechanisms such as passive diffusion, receptor-mediated transport, and transcytosis. For the paracellular pathway, ions and solutes use concentration gradients to pass the BBB by passive diffusion (Dong, 2018). Although the BBB protects the brain’s homeostasis, it may also interfere with therapeutic drug delivery into the CNS. This BBB resistance may occur via several mechanisms involving different structural and functional aspects of the BBB.
1.1 The Neurovascular Unit
Solute filtration at the BBB is a highly selective process that involves multiple cell types. The cells that play a role in the integrity of the BBB are collectively known as the neurovascular unit (NVU) (Abbott and Friedman, 2012). The primary component of the NVU is the brain microvascular endothelial cell (BMEC), which is a flat, sheet-like cell that forms the single-cell layer wall of brain microvessels (Kadry et al., 2020). BMECs and peripheral endothelial cells have similar functions, such as regulating exchanges between the bloodstream and other surrounding cells. However, endothelial cells assembling the microvascular lining of the BBB lack fenestrations or small breaches in the outer membrane. They also have tight intercellular junctions. As a result, diffusion of proteins and small molecules is highly limited (Cohen et al., 2001; Stamatovic et al., 2008; Campos-Bedolla et al., 2014; Kadry et al., 2020; Walter et al., 2021). Both peripheral and brain endothelial cells have substantial proinflammatory properties (Toborek et al., 1995; Andras et al., 2005).
Astrocytes, a glial cell type, also play a critical role in the formation of the BBB. Astrocytes present projections known as astrocytic end-feet that extend to the walls of the blood vessels of the BBB (Kubotera et al., 2019). Working jointly, astrocytes and BMECs mediate signals that prompt the formation of TJs and other cell adhesion molecules necessary to fortify BBB integrity (Abbott and Friedman, 2012).
Pericytes, embedded between the parenchyma and external lamina of the BMEC, are also part of the NVU. These cells are susceptible to injury and viral infections (Nakagawa et al., 2012; Bertrand et al., 2019). They are separated from the parenchyma by the basal lamina, a thin layer that also interposes between the pericyte and endothelial cells (Bergers and Song, 2005). Pericytes have several functions; they are thought to limit angiogenesis and provide microvascular stability by inhibiting the growth of capillaries. Pericytes also possess contractile functions, which regulate capillary diameter. Effectively, the size of the diameter will have an impact on oxygen and nutrient diffusion (Kadry et al., 2020).
Surrounding neurons remain closely associated with capillaries and connect with astrocytic endfeet in the vicinity of the BBB. In addition to controlling blood flow and microvascular permeability, neurons regulate angiogenesis by releasing factors that stimulate growth of new blood vessels. Furthermore, neurons assist in the synthesis and localization of tight junction molecules in brain endothelial cell culture (Savettieri et al., 2000).
1.2 Accessory Cells of the Blood–Brain Barrier
Cells that also play a role in BBB integrity are microglia (Kovac et al., 2009), leukocytes (Engelhardt 2006), and, according to some reports, surrounding neurons of the NVU (Sonar and Lal, 2018). They typically induce an inflammatory response to stress, infection, and other altercations in the brain. Microglial cells facilitate the inflammatory response after chronic and acute central nervous system disorders, including Alzheimer’s disease (AD) and Parkinson’s disease. They work in conjunction with the neurovascular unit and function in BBB-sensor homeostasis, so any disturbance within the brain causes BBB dysfunction and neuroinflammation.
1.3 Interendothelial Junctions of the Blood–Brain Barrier
The unregulated passage of polar molecules, toxins, and other substances between blood and brain is highly limited. It is primarily regulated by interendothelial junctions composed of protein complexes of tight junctions (TJ), adherence junctions (AJ), and gap junctions (GJ) (Komarova et al. 2017; Dong, 2018). TJs are transmembrane protein complexes that prohibit the interendothelial flux of solutes and ions (Hartsock and Nelson, 2008). AJs are linked to intracellular actin filaments of ECs and mainly initiate and stabilize cell–cell adhesion. Both AJs and TJs are intermembrane structures that function as seals to paracellular pathways of BMECs. Thus, the decrease in the integrity of these protein complexes results in inflammation, edema, and neuropathologies. Lastly, GJs are intercellular channels that direct electric and chemical communication between BMECs. Like TJs, GJs also regulate cell–cell transfer of ions and small molecules (Goodenough and Paul, 2009).
1.4 Efflux Transporters
An intact BBB is crucial for normal brain functions. Protecting the brain from potentially harmful endogenous and exogenous substances are physiological components such as efflux transporters and drug metabolizing enzymes. Efflux transporters of the BBB, such as P-glycoprotein (P-gp) (Cordon-Cardo et al., 1989), breast cancer resistance protein (BCRP) (Eisenblatter and Galla, 2002), and organic anion-transporting polypeptide (OATP) (Gao et al., 2000), are drug transporter proteins expressed at the luminal and abluminal BMEC membranes. P-gp and BCRP are specific membrane transporters known as multidrug resistance pumps. The role of these transporters is to detoxify the BMECs by actively pumping out compounds, such as xenobiotics, back into the blood stream (Kadry et al., 2020). P-gp can actively transport various compounds out of the cell by using ATP. Transported drugs, however, increase the enzymatic activity of ATPase by several folds. Due to their hydrophobic nature, most drugs will travel from the cytosol to the inner leaflet of the pump located in the lipid bilayer. Once inside, ATP must bind to the interior nucleotide-binding domains causing P-gp to undergo a dramatic conformational change that extrudes the drug to extracellular space. In a study involving Pgp inhibition in a rodent model, it was shown that knockout of one of the two genes that express P-gp in rodents (mdr1a) can increase drug penetration up to 100-fold but can sometimes lead to toxic consequences (Loscher and Potschka, 2005). Administration of P-gp inhibitors (e.g., PSC833 and GF120918) could also enhance brain entry of anticancer therapeutics (Loscher and Potschka, 2005). These data demonstrate that efflux pumps at the BBB level have a major role in the BBB resistance to therapeutic intervention.
1.5 Drug Metabolizing Enzymes of the Blood–Brain Barrier
Solute clearance is further enhanced by the presence of drug-metabolizing cytochrome P450 (CYP450) enzymes, a super-family of enzymes that are classified as monooxygenases. Located in the endoplasmic reticulum or within mitochondrial membranes of BMECs, CYP450 enzymes are responsible for the metabolism of xenobiotics and endogenous compounds, such as fatty acids in the brain microvascular area (Ghersi-Egea et al., 1994; Zanger and Schwab 2013). This group of enzymes can also cause oxidation of a large group of drugs, including antiepileptic drugs (Kadry et al., 2020).
2 Overcoming Blood–Brain Barrier Resistance: Extracellular Vesicle-Mediated Delivery Into the Brain
The integrated defense systems of the BBB impose a major challenge for effective drug delivery and the treatment of many brain diseases (Banks, 2016). Over the past decade, multiple strategies to improve drug delivery across the BBB are focused on noninvasive techniques. One of the most effective solutions to improve delivery efficiency relies on the use of extracellular vesicles (EVs).
2.1 Extracellular Vesicles
EVs are membrane vesicles from cellular origin that contain a lipid bilayer with a uniquely interactive surface area that can establish contact with surrounding cells and molecules of the extracellular microenvironment (Ratajczak et al., 2006; Zwi-Dantsis et al., 2020). The surface diameter of EVs expands from 20 nm to as large as 10 μm. The mean diameter is approximately 30–150 nm (Subedi et al., 2019). Additional morphological characteristics of EVs, such as shape, are less versatile. They are round but can take on an elongated appearance that is not energetically favorable; therefore, it is only temporary or reversible (Zabeo et al., 2017). The general EV properties are presented in Table 1. The biological properties of EVs have been subject of several publications from our laboratory (Andras et al., 2017; András et al., 2020a; Cho et al., 2021). We also characterized the proteome of EVs derived from brain endothelial cells (András et al., 2020b). EVs have three main subtypes: microvesicles (MVs), exosomes (EXOs), and apoptotic bodies (Borges, Reis, and Schor 2013; Yanez-Mo et al., 2015; Zaborowski et al., 2015; Yu et al., 2019). MVs are released from the cell surface via budding mechanisms and attach to other cells where they may have surface–surface interactions (Ratajczak et al., 2006). They contain adhesion molecules, such as integrins, that can influence the diffusion of vesicles. Different proteins and lipids are involved in the vesicle trafficking processes, which in turn influence membrane curvature and rigidity (Skog et al., 2008).
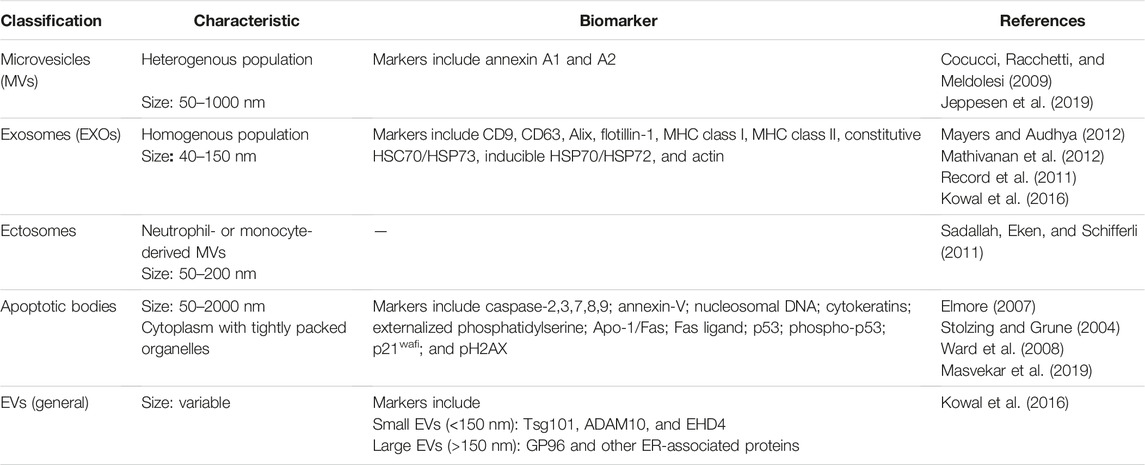
TABLE 1. Summary of EV Properties (modified from Lee et al., 2012).
A specific pool of vesicles is generated after the disassembly of apoptotic cells. These membrane-surrounded fragments are referred to as apoptotic bodies. Initially, it was believed that the primary role of vesicles pertaining to this category was harboring cellular debris of disassembled cells, and occurred spontaneously (Wickman et al., 2012; Xu et al., 2019). It is now becoming increasingly clear that these vesicles play a larger role in cellular apoptosis and, in fact, contain a wide variety of components such as micronuclei, chromatin remnants, cytosol portions, degraded proteins, DNA fragments, and intact organelles (Ma et al., 2021). Brain endothelial cell death may occur in both physiological and pathological conditions. In a study on zebrafish larvae, some brain endothelial cell apoptosis could be attributed to remodeling of the brain vasculature (Zhang et al., 2018). Another study reported that the increased presence of acid sphingomyelinase in old mice prompted apoptosis in brain endothelial cells (Park et al., 2018). In pathological conditions such as stroke, rapid and also late endothelial cell death was demonstrated (Zille et al., 2019). Programmed brain endothelial cell death can be correlated to low perfusion in capillaries (Park et al., 2018). Therefore, apoptotic bodies resulting from these processes may profoundly impact the surrounding cells of the neurovascular unit.
Smaller vesicles, such as exosomes (EXOs), are produced by multiple vesicular endosomes that undergo invagination, resulting in their release into the intraluminal or extracellular space of the cell. Exosomes act as shuttle vectors or signal transducers that can deliver specific biological information and mediate nearby or long-distance intercellular communication. Furthermore, EXOs and other EV subtypes are non-cytotoxic and exhibit a low immunogenic profile. These characteristics place them as promising candidates for the next generation of nanomedicine for both diagnostic and therapeutic purposes (Alvarez-Erviti et al., 2011; Jang et al., 2013; Yang et al., 2015; Khongkow et al., 2019).
Throughout the article, we will use the terms EVs (extracellular vesicles in general), EXOs (exosomes), MVs (microvesicles), and apoptotic bodies.
2.2 Extracellular Vesicle Transport and Uptake
EVs can cross the BBB from the blood into the brain and from the brain into the blood. True BBB transendothelial transport of vesicles in both directions and quantitative uptake of EVs was presented in a highly cited paper (Banks et al., 2020). According to the calculations presented in this study, all EXOs crossed the BBB with an influx rate from 0.044 µL/g-min to 0.524 µL/g-min (Banks et al., 2020). While the routes embarked by EVs remain unclear, there is evidence that EVs may cross the BBB using a variety of mechanisms depending on their origins. The mechanisms employed for this crossing are likely to involve endocytic and transcytotic pathways, such as adsorptive transcytosis (Jarmalaviciute and Pivoriunas, 2016; Banks et al., 2020). The available evidence that EXOs indeed cross the BBB primarily by transcytotic mechanisms suggests that there may be some connections between the mechanisms of pathways used by immune cells, infectious agents such as viruses, some large proteins, as well as nanoparticles for crossing the BBB (Vorbrodt and Trowbridge, 1991; Banks et al., 2012). Their convergence with viral pathways has been reviewed, summarizing how herpesviruses can merge with MVs pathways. Some proteins that are utilized for EXOs production by herpesviruses serve as functional release agents. The convergence of these pathways could explain the observation of virus-like particles, which could potentially be EXOs containing viral proteins or nucleic acids (Wurdinger et al., 2012). In addition, CD46 and mannose-6-phosphate (M6P) may also be involved in the EV crossing the BBB (Banks et al., 2020). Confocal microscopy showed that EXOs are internalized by brain endothelial cells through endocytosis, colocalize with endosomes, in effect primarily utilizing the transcellular route of crossing (Chen et al., 2016).
Receptor-mediated endocytosis via brain endothelial cells may be categorized into clathrin-mediated and non-clathrin-mediated (El-Sayed and Harashima, 2013). A proposed mechanism of clathrin-dependent-receptor-mediated endocytosis of EVs (Figure 1) is a ligand-receptor interaction between C-type lectin and its receptor (Hao et al., 2007). Many C-type lectins participate in receptor-mediated endocytosis to transport soluble bound ligands to lysosomes (Cummings and McEver, 2015). Lectins are glycoprotein-bound receptor domains that interact with and bind to carbohydrates and glycan moieties. Among the functions of this broad subset of proteins is cell-to-cell communication, adhesion, and intracellular transport. Three categories of lectins exist, all that have been linked to EVs. They are as follows: transmembrane lectins and selectins, transmembrane sialic acid-binding immunoglobulin-like lectins (SIGLECS), and galectins found in the cytosol (Gonda et al., 2019). Selectins, however, are the best-known lectin type found to regulate the uptake of EXOs and other EVs (Johannes et al., 2016). C-type lectin receptors have been identified on both dendritic cells and brain endothelial cells. Using antibodies that bind to cellular C-type lectin receptors, it was demonstrated that the internalization of macrophage-derived EXOs occurs via the interaction between C-type lectin and its receptor (Hao et al., 2007). The interaction of selectins and C-type lectins with EXOs suggests an emerging area of research into the intercellular communication that enhances immune cell–antigen recognition and movement (Gonda et al., 2019). The ligand–receptor complex triggers a signaling cascade, which causes clathrin molecules to home in the surrounding area of the cell membrane. Additional stabilizing factors then pull in the lipid bilayer, allowing entry of the C-type lectin ligand into the cytosol. The following step is the formation of a clathrin-coated vesicle because of the invagination of the membrane (Kaksonen and Roux, 2018). The fate of the cargo-containing vesicle ultimately depends on the content it delivers. For example, a cargo with a pH of 4–5 is directed toward the lysosome, where the membrane is degraded, freeing its content. Vesicles can also be recycled into endosomes and repurposed to trigger signals within the cell or in surrounding ones (El-Sayed and Harashima, 2013).
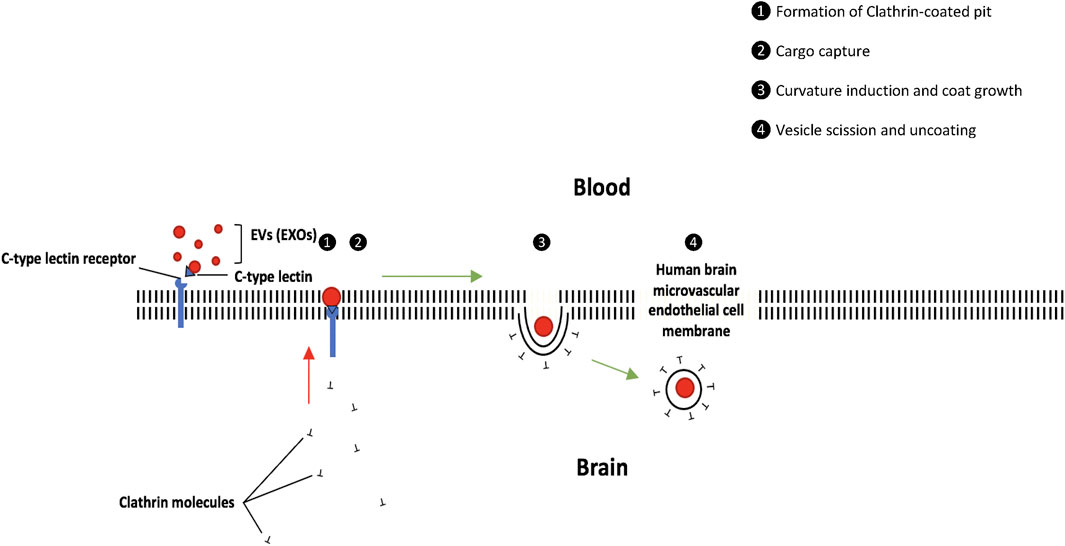
FIGURE 1. EV uptake via receptor-mediated endocytosis (clathrin-dependent). The ligand–receptor complex triggers a signaling cascade, which causes clathrin molecules to home in the surrounding area of the cell membrane. The following step is the formation of a clathrin-coated vesicle. The cargo-containing vesicle may be directed toward the lysosome for degradation or can be recycled into endosomes.
Conversely, clathrin-independent endocytosis takes place in lipid rafts that are enriched in caveolin, which play important roles as vesicle traffic mediators and signal transducers (Zhong et al., 2008). The rafts are small, fluid domains of the lipid bilayer composed of sphingolipids and cholesterol connected to phospholipids and membrane-associated proteins. The essential membrane proteins include: 1) proteins attached to glycosylphosphatidylinositol-anchored proteins (GPI-AP) that are inserted in the outer leaflet of the membrane, 2) proteins attached to the inner leaflet of the membrane, and 3) transmembrane proteins that have a cytoplasmic domain in addition to an outer domain that is exposed on the cell surface (Simons and Ikonen, 1997; El-Sayed and Harashima, 2013). In endothelial cells, caveolae-mediated endocytosis takes place in lipid rafts that are enriched in caveolin and the resulting vesicles are stabilized by cavin. Scission of the vesicles from the cell membrane takes place via the action of dynamin (El-Sayed and Harashima, 2013). A study (Segura et al., 2005) demonstrated a possible mechanism of uptake for dendritic cell (DC) derived EXOs into B lymphocytes. Specifically, a decrease in DC-EXO uptake was observed after a blockage of intercellular adhesion molecule 1 (ICAM-1), a surface glycoprotein expressed on DC-EXO membranes, and its corresponding cell surface receptor, lymphocyte function-associated antigen 1 (LFA-1) (Walling and Kim, 2018), suggesting the involvement of these molecules in EV uptake (Figure 2).
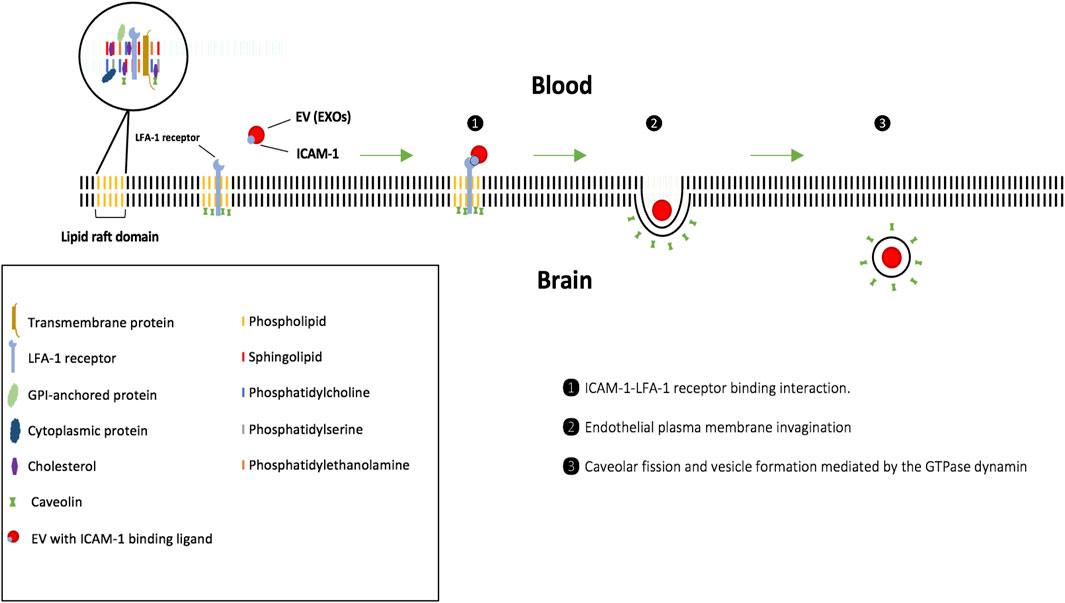
FIGURE 2. EV uptake via receptor-mediated endocytosis involving lipid rafts and caveolae (clathrin-independent). 1) Upon binding to the cell surface, EXOs travel along the endothelial plasma membrane toward the caveolae invaginations, where they are maintained through ICAM-1 ligand-LFA1 receptor interactions. 2) Fission of the caveolae from the membrane, mediated by the GTPase dynamin, then generates the cytosolic caveolar vesicle. 3) Caveosome is formed and does not undergo lysosomal degradation, and, therefore, may be advantageous for EXOs carrying cargo highly sensitive to enzymes.
Characterized by its uptake at a smaller scale, micropinocytosis occurs when an intracellular vacuole of size less than 0.2 µm (Anzinger et al., 2010) forms from the invagination of the plasma membrane. EXOs interact with the surface of the recipient cell via surface receptor molecules and ligands (Muthu et al., 2021), and micropinocytosis plays an important role in internalizing EXOs on the cell surface. Interestingly, micropinocytosis is being seen to play a larger role in LDL uptake (Anzinger et al., 2010), where it accounts for 40% of LDL uptake. In addition, it was demonstrated in a zebrafish model that nanoparticles were able to cross the BBB with the aid of micropinocytosis uptake (Zhao et al., 2020). Hence, EXOs encapsulating nanoparticles can pave routes toward nanoparticle drug delivery across the BBB via micropinocytosis uptake.
In a recent paper, high-resolution electron microscopy imaging of the BBB in vitro revealed nanovesicles bound to the brain endothelial plasma membrane surface. These membrane-bound vesicles appeared to impact the formation of thin nanotubes in the paracellular space between the brain endothelial cells. These nanotubes may have a crucial role in the paracellular space alignment and sealing (Mentor and Fisher 2021). We can speculate that EVs may cross the BBB in pathological conditions when the BBB permeability is increased via the altered paracellular pathway involving these nanovesicles.
2.3 Targeting of Extracellular Vesicles for Drug Delivery
EVs can carry versatile cargo loads, including both hydrophilic and hydrophobic drugs, nucleic acids like miRNA, siRNA, and recombinant proteins, or even solid-state nanoparticles. Substantial effort has concentrated on developing EXOs as a drug delivery system as they possess the ability to undergo modification to improve delivery capacity and targeting specificity of nanomaterials. An initial report demonstrated EXO-mediated delivery of siRNA to the mouse brain by intravenous injection (Alvarez-Erviti et al., 2011). This was achieved by genetically modifying EV-producing cells to produce a targeting fusion protein, followed by loading the EVs with the siRNA cargo. The fusion protein was composed of Lamp2b, a surface protein found in the membrane of EXOs, and rabies virus glycoprotein (RVG), a neuronal cell-targeted protein.
Phosphatidylserine (PS), one of the most common phospholipids and abundantly found on the surface of EVs (Pirisinu et al., 2020), is a key player in decreasing EV time in circulation (Miyanishi et al., 2007). Along with its receptor, phosphatidylserine receptor (PSR), the PS–PSR complex serves as a surface marker and is recognized by phagocytes as apoptotic cells (Hoffmann et al., 2005). Based on this, EVs could be potentially targeted to phagocytes and used for therapeutic purposes. For example, EVs carrying this marker and a drug could be engulfed by brain macrophages thus having a potential for targeting HIV reservoirs in the brain. When EXOs secreted by primary oligodendrocytes were exposed to liposomes containing PS (Fitzner et al., 2011), their uptake via macropinocytosis was reduced. This was due to competition with the liposomes containing PS, underscoring a role of PS in EV uptake. Additionally, it was demonstrated that EXO-PS can facilitate the recognition and internalization of neuronal EXOs by microglia (Yuyama et al., 2012).
EVs may have a potential as delivery vehicles or tracking tools in pathological conditions. In vitro work investigating the interactions between EXOs and brain endothelial cells under conditions that mimic the healthy and inflamed BBB in vivo demonstrated that their transport involved endocytotic processes (Chen et al., 2016). Transwell assays revealed that luciferase-carrying EXOs can cross a brain endothelial monolayer under stroke-like, tumor necrosis factor alpha activated inflamed conditions but not under normal conditions. Confocal microscopy demonstrated that EXOs are internalized by brain endothelial cells through endocytosis, colocalize with endosomes, in effect primarily utilizing the transcellular route of crossing (Chen et al., 2016).
Extracellular vesicle-mediated repair of damaged endothelial cells in an amyotrophic lateral sclerosis (ALS) in vitro model was demonstrated recently. Human bone marrow endothelial progenitor cell-derived EVs ameliorated mouse brain endothelial damage, and this effect appeared to be mediated via EV uptake into the endothelial cells (Garbuzova-Davis et al., 2020).
Moreover, EVs derived from rat brain endothelial cells in combination with tissue plasminogen activator (tPA) were reported to have a beneficial effect on stroke outcomes by reducing neurovascular damage. These EVs improved BBB integrity, reduced infarct volume, and improved neurological outcomes in rats. Ultrastructural data from TEM images clearly showed that intravenously administered EVs crossed the BBB and were internalized by astrocytes and injured neurons (Li et al., 2021). A recent study also demonstrated that EXOs can play a protective role when delivering specific toxic viral proteins into the brain. In a study engineering EXOs containing HIV-1 Tat (EXO-Tat), Tat neurotoxicity was greatly reduced both in vitro and in vivo (Tang et al., 2020). EXO-Tat could reactivate latent HIV-1 infection but neurotoxicity was inhibited by eliminating Tat’s ability to penetrate the neuronal cell membrane.
2.3.1 Intranasal Delivery of Extracellular Vesicles
EV drug delivery via the intranasal administration is gaining popularity due to its ability to easily bypass the BBB and retain itself at sites of injury better than the intravenous route. Two cranial nerves, olfactory and trigeminal, innervate the nasal cavity and provide direct access to the brain (Hanson and Frey, 2008). More specifically, drug administration via the intranasal route is narrowed down to intracellular and extracellular pathways (Crowe et al., 2018). In the intracellular pathway, the drug is engulfed via endocytosis by olfactory sensory cells, migrates via axonal transport, and is exocytosed in the olfactory bulb of the brain. In the extracellular pathway, the drug translocates through the tight TJs of the nasal epithelium into the lamina propria, travels externally along axons via bulk processes into the CNS.
This route of delivery was explored in successful treatment of brain inflammation induced by administration of lipopolysaccharide, myelin oligodendrocyte glycoprotein (MOG)-induced experimental autoimmune encephalitis (EAE) and in glioma-26 tumor model (Zhuang et al., 2011). Specifically, antioxidant curcumin was encapsulated in EXOs and delivered via the intranasal route. Intranasal drug delivery via EXOs was also applied toward rectifying spinal cord injury (SCI) (Guo et al., 2019). SCI results in limited axonal growth and function due to the adult central nervous system neuron’s maladaptive ability to regenerate from injury. In addition, SCI ensues major inflammation, myelin-associated inhibitors, glial components, and major blood loss. While attempts to correct spinal cord damage via permissive substrate grafting have proven to be ineffective (Griffin and Bradke, 2020), mesenchymal stem cell-derived EXOs (MSC-EXO) loaded with PTEN-siRNA were employed for intranasal administration to target spinal cord lesions. The PTEN gene normally functions as a tumor suppressor gene. The results indicated that EXOs migrated to the injured T10 spinal segment, suggesting a possibility of novel therapeutic approaches.
2.3.2 Extracellular Vesicles and the Choroid Plexus
The choroid plexus is characterized by its ability to secrete the majority of the central nervous system’s cerebrospinal fluid (CSF), which presents potential in emerging methods of drug delivery. Injection of drugs from the bloodstream is transferred to the CSF across the choroid plexus, while in the BBB or brain capillary endothelium, drug is transferred into the interstitial fluid (ISF) from the blood, due to different endothelial/epithelial barriers (Pardridge 2011). The blood–CSF barrier is formed by TJs of the ependymal epithelium lining the ventricles. The choroid plexus contains many capillaries, but they are leaky allowing for large volume flow in the brain as opposed to the capillaries with TJs found within the BBB (Abbott, 2004). When comparing brain penetration of drugs to CSF penetration, CLogD is used to measure lipophilicity of certain compounds (Abbott et al., 2018). It turned out that brain penetration increases as drugs become more lipid-soluble (increased CLogD), but CSF penetration significantly decreases with more lipid-soluble drugs (Abbott et al., 2018). The reason is that the aqueous CSF is less favorable for lipophylic compounds than the brain, where the compounds directly meet the lipid cell membranes. In reference to efflux transporters, such as P-glycoprotein, uptake of substrates for this transporter is generally closer at any given CLogD in wild-type mice that contain the P-glycoprotein transporter (Abbott et al., 2018). In the KO mice where P-glycoprotein is missing, brain entry is increased because the drug is not effluxed back to blood. Therefore, the presence of any compound in CSF cannot be a true measure of its brain level, especially for lipophilic drugs that interact with efflux transporters (Abbott et al., 2018).
Regarding the role of the choroid plexus in the EV-mediated brain pathologies, it was previously reported that peripheral inflammation evoked increased choroid plexus epithelial cell-derived EVs release at the blood–cerebrospinal fluid (CSF) interface into the CSF (Balusu et al., 2016). Later, the same group studied choroid plexus-mediated EV release in AD pathogenesis. They observed increased EV levels in the CSF of young transgenic APP/PS1 mice which correlated with high amyloid beta (Aβ) CSF levels. If they injected Aβ oligomers into the brain ventricles of wild-type mice, a significant increase of EVs in the CSF occurred and these EVs originated from the choroid plexus (Vandendriessche et al., 2021). Recently, it was also demonstrated that choroid plexus and CSF EVs might play a role in the pathogenesis of Niemann–Pick type C disease. Specifically, in NPC1−/− mice, enlarged CSF-EVs were observed. It turned out that EVs derived from NPC1−/− choroid plexus explants could induce typical brain pathology like microgliosis and astrogliosis (Van Hoecke et al., 2021).
2.3.3 Extracellular Vesicle-Based Brain Cancer Therapy
Several EV-based therapeutic strategies have been employed in experimental treatment of primary brain tumors, such as glioblastoma multiforme (GBM). Temozolomide (TMZ), an alkylating agent used as a first-line adjuvant drug, is at the frontline of GBM treatment; however, not all forms of GBM are sensitive to this drug. Because microRNA-9 (miR-9) is highly expressed in GBM cells that are resistant to TMZ, a strategy has been explored to deliver anti-miR-9 to TMZ-resistant GBM cells. This approach successfully sensitized the GBM cells to TMZ (Munoz et al., 2013).
Another miR, miR-146b, has also been explored as a potential contributor toward GBM cancer therapy. MiR-146b can inhibit glioma cell invasion, migration, viability, and expression of EGFR (Katakowski et al., 2013). In this context, bone marrow stromal cell-derived EXOs were tested to serve as a vehicle for miR-146b delivery into GBM cells. Administration of EXOs loaded with miR-146b via intra-tumor injection resulted in a significant reduction in 9 L glioma xenograft growth in a rat model (Katakowski et al., 2013).
2.3.4 Combined Extracellular Vesicle-Nanoparticle Delivery Into the Brain
Inorganic nanoparticles have an overall higher delivery efficiency compared with nanomaterials from organic origin. This is expected due to the vast range of tunable properties that inorganic nanoparticles possess, such as size, controlled release mechanisms, or active targeting (Patra et al., 2018). To improve the clinical translation of nanomedicine as an effective treatment for neurological diseases, it is important to consider these properties. The delivery efficiency of nanoparticles exhibiting neutral zeta potentials tends to be higher than that of nanoparticles with positive or negative zeta potentials. For a nanoparticle to efficiently reach the targeted tumor site, it must selectively interact with tumor cells while avoiding interaction with other cell types. If the designed nanoparticles exhibit a negative zeta potential charge, they may be repelled from the negatively charged tumor cell membrane, for example. Conversely, designing a positively charged surface for nanoparticles may allow for better interaction with the tumor cells, but may increase interaction with unwanted cell types (Gumustas et al., 2017). Furthermore, delivery methods, such as active targeting and passive targeting, are also considered. In passive targeting, nanoparticles take advantage of the enhanced permeability retention (EPR) effect, where they cross the tumor vascular membrane through intercellular gaps and undergo a longer retention period due to the impaired lymphatic drainage of the tumor (Shi et al., 2020). Alternatively, active targeting is a more efficient targeting method, which relies on attaching targeting ligands to the surface of nanoparticles. This method potentially reduces delivery time of nanoparticles, which decreases the risk of potential phagocytosis from immunogenic cells and enhances binding specificity, since the ligands in the nanoparticle surface are functionalized to target the corresponding receptors in the tumor site (Attia et al., 2019).
Similar approaches using EVs in combination with nanoparticles can be applied to the field of brain cancer. For example, EVs can be genetically modified to produce a fusion protein containing gelonin, a cancer targeting protein (Cheng et al., 2018). Following this modification, EVs can be loaded with nanoparticles such as iron oxide, gold nanoparticles, or zinc oxide. Iron is used in various magnetic applications because it contains four unpaired electrons in its d orbital that contribute to its magnetic potential. However, it is commonly used in the form of iron oxide because it is more stable than pure iron. Iron oxide is also biocompatible and was considered nontoxic to the human body, facilitating its use in biomedical applications (Teja and Koh 2009). However, iron oxide nanoparticles can also have some toxic effects involving oxidative stress (Mahmoudi et al., 2012; Soenen et al., 2012). When administered intranasally, they evoked oxidative stress and microglial activation in the olfactory bulb, hippocampus, and striatum (Kumari et al., 2013). In a rat model, these iron oxide nanoparticles induced oxidative stress, inflammation, and apoptosis in neurons (Kim et al., 2013). Once iron oxide is delivered to the cancer cell, it can induce magnetic hyperthermia or it can serve other purposes, such as magnetic resonance imaging (MRI) and photodynamic therapy (Estelrich and Busquets, 2018). Loaded inside EVs, gold nanoparticles can also serve as agents of magnetic resonance imaging (Khongkow et al., 2019). Zinc oxide nanoparticles encapsulated inside EVs can also be efficiently internalized by cancer cells, and particularly, may trigger apoptosis (Bai et al., 2017).
3 Challenges of Using Extracellular Vesicles for Brain Drug Delivery
Despite described advantages that expand EVs’ potential clinical use in cancer and CNS diseases, EV-mediated drug delivery methods are still at early stages and there are many obstacles yet to overcome. For example, achieving a large-scale production of EVs for clinical use is challenging. This process is affected by limited resources and requires cell culturing, followed by EV isolation, which potentially causes issues with vesicle purification. EVs have a complex structure as revealed by proteomic, RNA seq, and lipidomic studies, which is difficult to control, and they have the ability to manipulate cell microenvironment. For example, EVs derived from GBM cells can change the angiogenic phenotype in brain endothelial cells, increase the proliferation of GBM cells within the surrounding area, and increase tumor growth as a result of vesicle internalization. Specifically, these EVs contain angiogenic proteins, such as angiogenin, FGFα, IL-6, IL-8, TIMP-1, VEGF, and TIMP-2, and thus stimulate tumor vascularization promoting tumor growth. The presence of low pH levels in germinating tumors may facilitate lysis of EVs, leading to increased bioavailability of intravesicular proteins. In addition, angiogenesis-promoting proteins, such as angiogenin, require membrane transport to exert their biological activity, which could be also facilitated by EVs. Therefore, tumor-derived EVs may potentially also serve as a targeted product delivery vehicle, carrying multiple components, including mRNA, miRNA, and proteins, to communicate genetic information and signaling proteins to cells within proximity (Skog et al., 2008).
Another important factor to consider is the EV half-life in different biological compartments. EV blood levels reflect a balance between secretion and clearance rates (Matsumoto et al., 2020). After intravenous administration of EVs in a mouse model, EVs had an estimated half-life of 30 min and most EVs were cleared in 6 h (Lai et al., 2014). When crossing the BBB from the blood into the brain, EV clearance from the blood occurred rapidly (1.51–7.29 min) or slowly, independent of the EVs origin. These clearance patterns reached a prolonged steady state indicative of uptake by the periphery and exchange between the circulation and the peripheral tissues (Banks et al., 2020).
4 Conclusion
Although its functional and structural integrity is vital in maintaining the homeostasis of the brain microenvironment, the BBB compromises the effectiveness of many CNS treatments. Among the explored strategies to overcome this challenge, EVs are becoming increasingly promising candidates to cross or bypass the BBB by their innate capability to act as efficient drug delivery vehicles alone or in combination with nanoparticles. Nevertheless, several obstacles in using EVs for drug delivery remain to be addressed, including a large-scale production of EVs for clinical use or the biological impact of their cargo, which originates from the parent cells.
Author Contributions
JD, NS, IA and MT wrote or contributed to the writing of the manuscript; JD created all figures. MT provided funding.
Funding
This study was supported by the Florida Department of Health grant 8AZ24; the National Institutes of Health (NIH) grants MH128022, MH122235, MH072567, HL126559, DA044579, DA039576, DA040537, DA050528, and DA047157; and by the NSC grant 2019/35/B/NZ7/03155.
Conflict of Interest
The authors declare that the research was conducted in the absence of any commercial or financial relationships that could be construed as a potential conflict of interest.
Publisher’s Note
All claims expressed in this article are solely those of the authors and do not necessarily represent those of their affiliated organizations, or those of the publisher, the editors, and the reviewers. Any product that may be evaluated in this article, or claim that may be made by its manufacturer, is not guaranteed or endorsed by the publisher.
References
Abbott, N. J., and Friedman, A. (2012). Overview and Introduction: the Blood-Brain Barrier in Health and Disease. Epilepsia 53 (Suppl. 6), 1–6. doi:10.1111/j.1528-1167.2012.03696.x
Abbott, N. J., Pizzo, M. E., Preston, J. E., Janigro, D., and Thorne, R. G. (2018). The Role of Brain Barriers in Fluid Movement in the CNS: Is There a 'glymphatic' System? Acta Neuropathol. 135 (3), 387–407. doi:10.1007/s00401-018-1812-4
Abbott, N. J. (2004). Evidence for Bulk Flow of Brain Interstitial Fluid: Significance for Physiology and Pathology. Neurochem. Int. 45 (4), 545–552. doi:10.1016/j.neuint.2003.11.006
Alvarez-Erviti, L., Seow, Y., Yin, H., Betts, C., Lakhal, S., and Wood, M. J. A. (2011). Delivery of siRNA to the Mouse Brain by Systemic Injection of Targeted Exosomes. Nat. Biotechnol. 29 (4), 341–345. doi:10.1038/nbt.1807
András, I. E., Pu, H., Tian, J., Deli, M. A., Nath, A., Hennig, B., et al. (2005). Signaling Mechanisms of HIV-1 Tat-Induced Alterations of Claudin-5 Expression in Brain Endothelial Cells. J. Cereb. Blood Flow Metab. 25 (9), 1159–1170. doi:10.1038/sj.jcbfm.9600115
András, I. E., Leda, A., Contreras, M. G., Bertrand, L., Park, M., Skowronska, M., et al. (2017). Extracellular Vesicles of the Blood-Brain Barrier: Role in the HIV-1 Associated Amyloid Beta Pathology. Mol. Cell Neurosci. 79, 12–22. doi:10.1016/j.mcn.2016.12.006
András, I. E., Garcia-Contreras, M., Yanick, C., Perez, P., Sewell, B., Durand, L., et al. (2020a). Extracellular Vesicle-Mediated Amyloid Transfer to Neural Progenitor Cells: Implications for RAGE and HIV Infection. Mol. Brain 13 (1), 21. doi:10.1186/s13041-020-0562-0
András, I. E., Sewell, B. B., and Toborek, M. (2020b). HIV-1 and Amyloid Beta Remodel Proteome of Brain Endothelial Extracellular Vesicles. Ijms 21 (8), 2741. doi:10.3390/ijms21082741
Anzinger, J. J., Chang, J., Xu, Q., Buono, C., Li, Y., Leyva, F. J., et al. (2010). Native Low-Density Lipoprotein Uptake by Macrophage colony-stimulating Factor-Differentiated Human Macrophages Is Mediated by Macropinocytosis and Micropinocytosis. Atvb 30 (10), 2022–2031. doi:10.1161/ATVBAHA.110.210849
Attia, M. F., Anton, N., Wallyn, J., Omran, Z., and Vandamme, T. F. (2019). An Overview of Active and Passive Targeting Strategies to Improve the Nanocarriers Efficiency to Tumour Sites. J. Pharm. Pharmacol. 71 (8), 1185–1198. doi:10.1111/jphp.13098
Bai, D.-P., Zhang, X.-F., Zhang, G.-L., Huang, Y.-F., and Gurunathan, S. (2017). Zinc Oxide Nanoparticles Induce Apoptosis and Autophagy in Human Ovarian Cancer Cells. Ijn 12, 6521–6535. doi:10.2147/IJN.S140071
Balusu, S., Van Wonterghem, E., De Rycke, R., Raemdonck, K., Stremersch, S., Gevaert, K., et al. (2016). Identification of a Novel Mechanism of Blood-Brain Communication during Peripheral Inflammation via Choroid Plexus-Derived Extracellular Vesicles. EMBO Mol. Med. 8 (10), 1162–1183. doi:10.15252/emmm.201606271
Banks, W. A., Niehoff, M. L., Ponzio, N. M., Erickson, M. A., and Zalcman, S. S. (2012). Pharmacokinetics and Modeling of Immune Cell Trafficking: Quantifying Differential Influences of Target Tissues versus Lymphocytes in SJL and Lipopolysaccharide-Treated Mice. J. Neuroinflamm. 9, 231. doi:10.1186/1742-2094-9-231
Banks, W. A., Sharma, P., Bullock, K. M., Hansen, K. M., Ludwig, N., and Whiteside, T. L. (2020). Transport of Extracellular Vesicles across the Blood-Brain Barrier: Brain Pharmacokinetics and Effects of Inflammation. Ijms 21 (12), 4407. doi:10.3390/ijms21124407
Banks, W. A. (2016). From Blood-Brain Barrier to Blood-Brain Interface: New Opportunities for CNS Drug Delivery. Nat. Rev. Drug Discov. 15 (4), 275–292. doi:10.1038/nrd.2015.21
Bergers, G., and Song, S. (2005). The Role of Pericytes in Blood-Vessel Formation and Maintenance. Neuro Oncol. 7 (4), 452–464. doi:10.1215/S1152851705000232
Bertrand, L., Cho, H. J., and Toborek, M. (2019). Blood-brain Barrier Pericytes as a Target for HIV-1 Infection. Brain 142 (3), 502–511. doi:10.1093/brain/awy339
Borges, F. T., Reis, L. A., and Schor, N. (2013). Extracellular Vesicles: Structure, Function, and Potential Clinical Uses in Renal Diseases. Braz. J. Med. Biol. Res. 46 (10), 824–830. doi:10.1590/1414-431X20132964
Campos-Bedolla, P., Walter, F. R., Veszelka, S., and Deli, M. A. (2014). Role of the Blood-Brain Barrier in the Nutrition of the central Nervous System. Arch. Med. Res. 45 (8), 610–638. doi:10.1016/j.arcmed.2014.11.018
Chen, C. C., Liu, L., Ma, F., Wong, C. W., Guo, X. E., Chacko, J. V., et al. (2016). Elucidation of Exosome Migration across the Blood-Brain Barrier Model In Vitro. Cel. Mol. Bioeng. 9 (4), 509–529. doi:10.1007/s12195-016-0458-3
Cheng, G., Li, W., Ha, L., Han, X., Hao, S., Wan, Y., et al. (2018). Self-Assembly of Extracellular Vesicle-like Metal-Organic Framework Nanoparticles for Protection and Intracellular Delivery of Biofunctional Proteins. J. Am. Chem. Soc. 140 (23), 7282–7291. doi:10.1021/jacs.8b03584
Cho, H. J., Velichkovska, M., Schurhoff, N., András, I. E., and Toborek, M. (2021). Extracellular Vesicles Regulate gap junction-mediated Intercellular Communication and HIV-1 Infection of Human Neural Progenitor Cells. Neurobiol. Dis. 155, 105388. doi:10.1016/j.nbd.2021.105388
Cocucci, E., Racchetti, G., and Meldolesi, J. (2009). Shedding Microvesicles: Artefacts No More. Trends Cel Biol. 19 (2), 43–51. doi:10.1016/j.tcb.2008.11.003
Cohen, C. J., Shieh, J. T. C., Pickles, R. J., Okegawa, T., Hsieh, J.-T., and Bergelson, J. M. (2001). The Coxsackievirus and Adenovirus Receptor Is a Transmembrane Component of the Tight junction. Proc. Natl. Acad. Sci. 98 (26), 15191–15196. doi:10.1073/pnas.261452898
Cordon-Cardo, C., O'Brien, J. P., Casals, D., Rittman-Grauer, L., Biedler, J. L., Melamed, M. R., et al. (1989). Multidrug-resistance Gene (P-Glycoprotein) Is Expressed by Endothelial Cells at Blood-Brain Barrier Sites. Proc. Natl. Acad. Sci. 86 (2), 695–698. doi:10.1073/pnas.86.2.695
Crowe, T. P., Greenlee, M. H. W., Kanthasamy, A. G., and Hsu, W. H. (2018). Mechanism of Intranasal Drug Delivery Directly to the Brain. Life Sci. 195, 44–52. doi:10.1016/j.lfs.2017.12.025
Cummings, R. D., and McEver, R. P. (2015). “C-Type Lectins,” in Essentials of Glycobiology. Editor A. Varki, R. D. Cummings, J. D. Esko, P. Stanley, G. W. Hart, M. Aebiet al. (NY: Cold Spring Harbor), 435–452.
Dong, X. (2018). Current Strategies for Brain Drug Delivery. Theranostics 8 (6), 1481–1493. doi:10.7150/thno.21254
Eisenblätter, T., and Galla, H.-J. (2002). A New Multidrug Resistance Protein at the Blood-Brain Barrier. Biochem. Biophysical Res. Commun. 293 (4), 1273–1278. doi:10.1016/S0006-291X(02)00376-5
Elmore, S. (2007). Apoptosis: a Review of Programmed Cell Death. Toxicol. Pathol. 35 (4), 495–516. doi:10.1080/01926230701320337
El-Sayed, A., and Harashima, H. (2013). Endocytosis of Gene Delivery Vectors: from Clathrin-dependent to Lipid Raft-Mediated Endocytosis. Mol. Ther. 21 (6), 1118–1130. doi:10.1038/mt.2013.54
Engelhardt, B. (2006). Molecular Mechanisms Involved in T Cell Migration across the Blood-Brain Barrier. J. Neural Transm. 113 (4), 477–485. doi:10.1007/s00702-005-0409-y
Estelrich, J., and Busquets, M. (2018). Iron Oxide Nanoparticles in Photothermal Therapy. Molecules 23 (7), 1567. doi:10.3390/molecules23071567
Fitzner, D., Schnaars, M., van Rossum, D., Krishnamoorthy, G., Dibaj, P., Bakhti, M., et al. (2011). Selective Transfer of Exosomes from Oligodendrocytes to Microglia by Macropinocytosis. J. Cel Sci. 124 (Pt 3), 447–458. doi:10.1242/jcs.074088
Gao, B., Hagenbuch, B., Kullak-Ublick, G. A., Benke, D., Aguzzi, A., and Meier, P. J. (2000). Organic Anion-Transporting Polypeptides Mediate Transport of Opioid Peptides across Blood-Brain Barrier. J. Pharmacol. Exp. Ther. 294 (1), 73–79.
Garbuzova-Davis, S., Willing, A. E., Ehrhart, J., Wang, L., Sanberg, P. R., and Borlongan, C. V. (2020). Cell-Free Extracellular Vesicles Derived from Human Bone Marrow Endothelial Progenitor Cells as Potential Therapeutics for Microvascular Endothelium Restoration in ALS. Neuromol Med. 22 (4), 503–516. doi:10.1007/s12017-020-08607-1
Ghersi-Egea, J. F., Leninger-Muller, B., Suleman, G., Siest, G., and Minn, A. (1994). Localization of Drug-Metabolizing Enzyme Activities to Blood-Brain Interfaces and Circumventricular Organs. J. Neurochem. 62 (3), 1089–1096. doi:10.1046/j.1471-4159.1994.62031089.x
Gonda, A., Kabagwira, J., Senthil, G. N., and Wall, N. R. (2019). Internalization of Exosomes through Receptor-Mediated Endocytosis. Mol. Cancer Res. 17 (2), 337–347. doi:10.1158/1541-7786.MCR-18-0891
Goodenough, D. A., and Paul, D. L. (2009). Gap Junctions. Cold Spring Harbor Perspect. Biol. 1 (1), a002576. doi:10.1101/cshperspect.a002576
Griffin, J. M., and Bradke, F. (2020). Therapeutic Repair for Spinal Cord Injury: Combinatory Approaches to Address a Multifaceted Problem. EMBO Mol. Med. 12 (3), e11505. doi:10.15252/emmm.201911505
Gumustas, M., Sengel-Turk, C. T., Gumustas, A., Ozkan, S. A., and Uslu, B. (2017). “Effect of Polymer-Based Nanoparticles on the Assay of Antimicrobial Drug Delivery Systems,” in Multifunctional Systems for Combined Delivery, Biosensing and Diagnostics. Editor A. M. Grumezescu (Bucharest, RO: Elsevier), 67–108. Available at: https://www.sciencedirect.com/science/article/pii/B9780323527255000058. doi:10.1016/B978-0-323-52725-5.00005-8
Guo, S., Perets, N., Betzer, O., Ben-Shaul, S., Sheinin, A., Michaelevski, I., et al. (2019). Intranasal Delivery of Mesenchymal Stem Cell Derived Exosomes Loaded with Phosphatase and Tensin Homolog siRNA Repairs Complete Spinal Cord Injury. ACS Nano 13 (9), 10015–10028. doi:10.1021/acsnano.9b01892
Hanson, L. R., and Frey, W. H. (2008). Intranasal Delivery Bypasses the Blood-Brain Barrier to Target Therapeutic Agents to the central Nervous System and Treat Neurodegenerative Disease. BMC Neurosci. 9 Suppl 3 (Suppl. 3), S5. doi:10.1186/1471-2202-9-S3-S5
Hao, S., Bai, O., Li, F., Yuan, J., Laferte, S., and Xiang, J. (2007). Mature Dendritic Cells Pulsed with Exosomes Stimulate Efficient Cytotoxic T-Lymphocyte Responses and Antitumour Immunity. Immunology 120 (1), 90–102. doi:10.1111/j.1365-2567.2006.02483.x
Hartsock, A., and Nelson, W. J. (2008). Adherens and Tight Junctions: Structure, Function and Connections to the Actin Cytoskeleton. Biochim. Biophys. Acta (Bba) - Biomembr. 1778 (3), 660–669. doi:10.1016/j.bbamem.2007.07.012
Hoffmann, P. R., Kench, J. A., Vondracek, A., Kruk, E., Daleke, D. L., Jordan, M., et al. (2005). Interaction between Phosphatidylserine and the Phosphatidylserine Receptor Inhibits Immune Responses In Vivo. J. Immunol. 174 (3), 1393–1404. doi:10.4049/jimmunol.174.3.1393
Jang, S. C., Kim, O. Y., Yoon, C. M., Choi, D.-S., Roh, T.-Y., Park, J., et al. (2013). Bioinspired Exosome-Mimetic Nanovesicles for Targeted Delivery of Chemotherapeutics to Malignant Tumors. ACS Nano 7 (9), 7698–7710. doi:10.1021/nn402232g
Jarmalavičiūtė, A., and Pivoriūnas, A. (2016). Exosomes as a Potential Novel Therapeutic Tools against Neurodegenerative Diseases. Pharmacol. Res. 113 (Pt B), 816–822. doi:10.1016/j.phrs.2016.02.002
Jeppesen, D. K., Fenix, A. M., Franklin, J. L., Higginbotham, J. N., Zhang, Q., Zimmerman, L. J., et al. (2019). Reassessment of Exosome Composition. Cell 177 (2), 428–445. doi:10.1016/j.cell.2019.02.029
Johannes, L., Wunder, C., and Shafaq-Zadah, M. (2016). Glycolipids and Lectins in Endocytic Uptake Processes. J. Mol. Biol. 428, 4792–4818. doi:10.1016/j.jmb.2016.10.027
Kadry, H., Noorani, B., and Cucullo, L. (2020). A Blood-Brain Barrier Overview on Structure, Function, Impairment, and Biomarkers of Integrity. Fluids Barriers CNS 17 (1), 69. doi:10.1186/s12987-020-00230-3
Kaksonen, M., and Roux, A. (2018). Mechanisms of Clathrin-Mediated Endocytosis. Nat. Rev. Mol. Cel. Biol. 19 (5), 313–326. doi:10.1038/nrm.2017.132
Katakowski, M., Buller, B., Zheng, X., Lu, Y., Rogers, T., Osobamiro, O., et al. (2013). Exosomes from Marrow Stromal Cells Expressing miR-146b Inhibit Glioma Growth. Cancer Lett. 335 (1), 201–204. doi:10.1016/j.canlet.2013.02.019
Khongkow, M., Yata, T., Boonrungsiman, S., Ruktanonchai, U. R., Graham, D., and Namdee, K. (2019). Surface Modification of Gold Nanoparticles with Neuron-Targeted Exosome for Enhanced Blood-Brain Barrier Penetration. Sci. Rep. 9 (1), 8278. doi:10.1038/s41598-019-44569-6
Kim, Y., Kong, S. D., Chen, L.-H., Pisanic, T. R., Jin, S., and Shubayev, V. I. (2013). In Vivo nanoneurotoxicity Screening Using Oxidative Stress and Neuroinflammation Paradigms. Nanomed. Nanotechnol. Biol. Med. 9 (7), 1057–1066. doi:10.1016/j.nano.2013.05.002
Komarova, Y. A., Kruse, K., Mehta, D., and Malik, A. B. (2017). Protein Interactions at Endothelial Junctions and Signaling Mechanisms Regulating Endothelial Permeability. Circ. Res. 120 (1), 179–206. doi:10.1161/CIRCRESAHA.116.306534
Kovac, A., Zilkova, M., Deli, M. A., Zilka, N., and Novak, M. (2009). Human Truncated Tau Is Using a Different Mechanism from Amyloid-β to Damage the Blood-Brain Barrier. Jad 18 (4), 897–906. doi:10.3233/JAD-2009-1197
Kowal, J., Arras, G., Colombo, M., Jouve, M., Morath, J. P., Primdal-Bengtson, B., et al. (2016). Proteomic Comparison Defines Novel Markers to Characterize Heterogeneous Populations of Extracellular Vesicle Subtypes. Proc. Natl. Acad. Sci. USA 113 (8), E968–E977. doi:10.1073/pnas.1521230113
Kubotera, H., Ikeshima-Kataoka, H., Hatashita, Y., Allegra Mascaro, A. L., Pavone, F. S., and Inoue, T. (2019). Astrocytic Endfeet Re-cover Blood Vessels after Removal by Laser Ablation. Sci. Rep. 9 (1), 1263. doi:10.1038/s41598-018-37419-4
Kumari, M., Rajak, S., Singh, S. P., Murty, U. S. N., Mahboob, M., Grover, P., et al. (2013). Biochemical Alterations Induced by Acute Oral Doses of Iron Oxide Nanoparticles in Wistar Rats. Drug Chem. Toxicol. 36 (3), 296–305. doi:10.3109/01480545.2012.720988
Lai, C. P., Mardini, O., Ericsson, M., Prabhakar, S., Maguire, C. A., Chen, J. W., et al. (2014). Dynamic Biodistribution of Extracellular Vesicles In Vivo Using a Multimodal Imaging Reporter. ACS Nano 8 (1), 483–494. doi:10.1021/nn404945r
Lee, Y., El Andaloussi, S., and Wood, M. J. (2012). Exosomes and Microvesicles: Extracellular Vesicles for Genetic Information Transfer and Gene Therapy. Hum. Mol. Genet. 21 (R1), R125–R134. doi:10.1093/hmg/dds317
Li, C., Wang, C., Zhang, Y., Alsrouji, O. K., Chebl, A. B., Ding, G., et al. (2021). Cerebral Endothelial Cell-Derived Small Extracellular Vesicles Enhance Neurovascular Function and Neurological Recovery in Rat Acute Ischemic Stroke Models of Mechanical Thrombectomy and Embolic Stroke Treatment with tPA. J. Cereb. Blood Flow Metab. 41 (8), 0271678X2199298–2104. doi:10.1177/0271678X21992980
Löscher, W., and Potschka, H. (2005). Blood-brain Barrier Active Efflux Transporters: ATP-Binding Cassette Gene Family. Neurotherapeutics 2 (1), 86–98. doi:10.1602/neurorx.2.1.86
Ma, Q., Liang, M., Wu, Y., Luo, F., Ma, Z., Dong, S., et al. (2021). Osteoclast-derived Apoptotic Bodies Couple Bone Resorption and Formation in Bone Remodeling. Bone Res. 9 (1), 5. doi:10.1038/s41413-020-00121-1
Mahmoudi, M., Hofmann, H., Rothen-Rutishauser, B., and Petri-Fink, A. (2012). Assessing the In Vitro and In Vivo Toxicity of Superparamagnetic Iron Oxide Nanoparticles. Chem. Rev. 112 (4), 2323–2338. doi:10.1021/cr2002596
Masvekar, R., Wu, T., Kosa, P., Barbour, C., Fossati, V., and Bielekova, B. (2019). Cerebrospinal Fluid Biomarkers Link Toxic Astrogliosis and Microglial Activation to Multiple Sclerosis Severity. Mult. Scler. Relat. Disord. 28, 34–43. doi:10.1016/j.msard.2018.11.032
Mathivanan, S., Fahner, C. J., Reid, G. E., and Simpson, R. J. (2012). ExoCarta 2012: Database of Exosomal Proteins, RNA and Lipids. Nucleic Acids Res. 40 (Database issue), D1241–D1244. doi:10.1093/nar/gkr828
Matsumoto, A., Takahashi, Y., Chang, H. Y., Wu, Y. W., Yamamoto, A., Ishihama, Y., et al. (2020). Blood Concentrations of Small Extracellular Vesicles Are Determined by a Balance between Abundant Secretion and Rapid Clearance. J. Extracell. Vesicles 9 (1), 1696517. doi:10.1080/20013078.2019.1696517
Mayers, J. R., and Audhya, A. (2012). Vesicle Formation within Endosomes: An ESCRT marks the Spot. Commun. Integr. Biol. 5 (1), 50–56. doi:10.4161/cib.18208
Mentor, S., and Fisher, D. (2021). High-Resolution Insights into the In Vitro Developing Blood-Brain Barrier: Novel Morphological Features of Endothelial Nanotube Function. Front. Neuroanat. 15, 661065. doi:10.3389/fnana.2021.661065
Miyanishi, M., Tada, K., Koike, M., Uchiyama, Y., Kitamura, T., and Nagata, S. (2007). Identification of Tim4 as a Phosphatidylserine Receptor. Nature 450 (7168), 435–439. doi:10.1038/nature06307
Munoz, J. L., Bliss, S. A., Greco, S. J., Ramkissoon, S. H., Ligon, K. L., and Rameshwar, P. (2013). Delivery of Functional Anti-miR-9 by Mesenchymal Stem Cell-Derived Exosomes to Glioblastoma Multiforme Cells Conferred Chemosensitivity. Mol. Ther. - Nucleic Acids 2, e126. doi:10.1038/mtna.2013.60
Muthu, S., Bapat, A., Jain, R., Jeyaraman, N., and Jeyaraman, M. (2021). Exosomal Therapy-A New Frontier in Regenerative Medicine. Stem Cel Investig. 8, 7. doi:10.21037/sci-2020-037
Nakagawa, S., Castro, V., and Toborek, M. (2012). Infection of Human Pericytes by HIV-1 Disrupts the Integrity of the Blood-Brain Barrier. J. Cel. Mol. Med. 16 (12), 2950–2957. doi:10.1111/j.1582-4934.2012.01622.x
Naranjo, O., Osborne, O., Torices, S., and Toborek, M. (2021). In Vivo Targeting of the Neurovascular Unit: Challenges and Advancements. Cell Mol. Neurobiol. doi:10.1007/s10571-021-01113-3
Osborne, O., Peyravian, N., Nair, M., Daunert, S., and Toborek, M. (2020). The Paradox of HIV Blood-Brain Barrier Penetrance and Antiretroviral Drug Delivery Deficiencies. Trends Neurosci. 43 (9), 695–708. doi:10.1016/j.tins.2020.06.007
Palmer, A. M., and Alavijeh, M. S. (2013). Overview of Experimental Models of the Blood-Brain Barrier in CNS Drug Discovery. Curr. Protoc. Pharmacol. 62, 7.15.1–7.15.30. doi:10.1002/0471141755.ph0715s62
Pardridge, W. M. (2011). Drug Transport in Brain via the Cerebrospinal Fluid. Fluids Barriers CNS 8 (1), 7. doi:10.1186/2045-8118-8-7
Park, M. H., Lee, J. Y., Park, K. H., Jung, I. K., Kim, K.-T., Lee, Y.-S., et al. (2018). Vascular and Neurogenic Rejuvenation in Aging Mice by Modulation of ASM. Neuron 100 (3), 762. doi:10.1016/j.neuron.2018.10.038
Patra, J. K., Das, G., Fraceto, L. F., Campos, E. V. R., Rodriguez-Torres, M. D. P., Acosta-Torres, L. S., et al. (2018). Nano Based Drug Delivery Systems: Recent Developments and Future Prospects. J. Nanobiotechnol. 16 (1), 71. doi:10.1186/s12951-018-0392-8
Pirisinu, M., Pham, T. C., Zhang, D. X., Hong, T. N., Nguyen, L. T., and Le, M. T. (2020). Extracellular Vesicles as Natural Therapeutic Agents and Innate Drug Delivery Systems for Cancer Treatment: Recent Advances, Current Obstacles, and Challenges for Clinical Translation. Semin. Cancer Biol 80, 340–355. doi:10.1016/j.semcancer.2020.08.007
Ratajczak, J., Miekus, K., Kucia, M., Zhang, J., Reca, R., Dvorak, P., et al. (2006). Embryonic Stem Cell-Derived Microvesicles Reprogram Hematopoietic Progenitors: Evidence for Horizontal Transfer of mRNA and Protein Delivery. Leukemia 20 (5), 847–856. doi:10.1038/sj.leu.2404132
Record, M., Subra, C., Silvente-Poirot, S., and Poirot, M. (2011). Exosomes as Intercellular Signalosomes and Pharmacological Effectors. Biochem. Pharmacol. 81 (10), 1171–1182. doi:10.1016/j.bcp.2011.02.011
Sadallah, S., Eken, C., and Schifferli, J. A. (2010). Ectosomes as Modulators of Inflammation and Immunity. Clin. Exp. Immunol. 163 (1), 26–32. doi:10.1111/j.1365-2249.2010.04271.x
Savettieri, G., Liegro, I. D., Catania, C., Licata, L., Pitarresi, G. L., DʼAgostino, S., et al. (2000). Neurons and ECM Regulate Occludin Localization in Brain Endothelial Cells. Neuroreport 11 (5), 1081–1084. doi:10.1097/00001756-200004070-00035
Segura, E., Nicco, C., Lombard, B., Véron, P., Raposo, G., Batteux, F., et al. (2005). ICAM-1 on Exosomes from Mature Dendritic Cells Is Critical for Efficient Naive T-Cell Priming. Blood 106 (1), 216–223. doi:10.1182/blood-2005-01-0220
Shi, Y., van der Meel, R., Chen, X., and Lammers, T. (2020). The EPR Effect and beyond: Strategies to Improve Tumor Targeting and Cancer Nanomedicine Treatment Efficacy. Theranostics 10 (17), 7921–7924. doi:10.7150/thno.49577
Simons, K., and Ikonen, E. (1997). Functional Rafts in Cell Membranes. Nature 387 (6633), 569–572. doi:10.1038/42408
Skog, J., Würdinger, T., van Rijn, S., Meijer, D. H., Gainche, L., Curry, W. T., et al. (2008). Glioblastoma Microvesicles Transport RNA and Proteins that Promote Tumour Growth and Provide Diagnostic Biomarkers. Nat. Cel Biol. 10 (12), 1470–1476. doi:10.1038/ncb1800
Soenen, S. J., De Cuyper, M., De Smedt, S. C., and Braeckmans, K. (2012). Investigating the Toxic Effects of Iron Oxide Nanoparticles. Methods Enzymol. 509, 195–224. doi:10.1016/B978-0-12-391858-1.00011-3
Sonar, S. A., and Lal, G. (2018). Blood-brain Barrier and its Function during Inflammation and Autoimmunity. J. Leukoc. Biol. 103 (5), 839–853. doi:10.1002/JLB.1RU1117-428R
Stamatovic, S., Keep, R., and Andjelkovic, A. (2008). Brain Endothelial Cell-Cell Junctions: How to “Open” the Blood Brain Barrier. Cn 6 (3), 179–192. doi:10.2174/157015908785777210
Stolzing, A., and Grune, T. (2004). Neuronal Apoptotic Bodies: Phagocytosis and Degradation by Primary Microglial Cells. FASEB j. 18 (6), 743–745. doi:10.1096/fj.03-0374fje
Subedi, P., Schneider, M., Philipp, J., Azimzadeh, O., Metzger, F., Moertl, S., et al. (2019). Comparison of Methods to Isolate Proteins from Extracellular Vesicles for Mass Spectrometry-Based Proteomic Analyses. Anal. Biochem. 584, 113390. doi:10.1016/j.ab.2019.113390
Tang, X., Lu, H., and Ramratnam, B. (2020). Neurotoxicity of HIV-1 Tat Is Attributed to its Penetrating Property. Sci. Rep. 10 (1), 14002. doi:10.1038/s41598-020-70950-x
Teja, A. S., and Koh, P.-Y. (2009). “Synthesis, Properties, and Applications of Magnetic Iron Oxide Nanoparticles,” in Progress in Crystal Growth and Characterization of Materials. Editor J. B. Mullin (Poole, Dorset, UK: Elsevier), 22–45. Available at: https://www.sciencedirect.com/science/article/pii/S0960897408000168. doi:10.1016/j.pcrysgrow.2008.08.003
Toborek, M., Barger, S. W., Mattson, M. P., McClain, C. J., and Hennig, B. (1995). Role of Glutathione Redox Cycle in TNF-α-Mediated Endothelial Cell Dysfunction. Atherosclerosis 117 (2), 179–188. doi:10.1016/0021-9150(95)05568-h
Toborek, M., Lee, Y. W., Flora, G., Pu, H., András, I. E., Wylegala, E., et al. (2005). Mechanisms of the Blood-Brain Barrier Disruption in HIV-1 Infection. Cel Mol. Neurobiol. 25 (1), 181–199. doi:10.1007/s10571-004-1383-x
Van Hoecke, L., Van Cauwenberghe, C., Dominko, K., Van Imschoot, G., Van Wonterghem, E., Castelein, J., et al. (2021). Involvement of the Choroid Plexus in the Pathogenesis of Niemann-Pick Disease Type C. Front. Cel. Neurosci. 15, 757482. doi:10.3389/fncel.2021.757482
Vandendriessche, C., Balusu, S., Van Cauwenberghe, C., Brkic, M., Pauwels, M., Plehiers, N., et al. (2021). Importance of Extracellular Vesicle Secretion at the Blood-Cerebrospinal Fluid Interface in the Pathogenesis of Alzheimer's Disease. Acta Neuropathol. Commun. 9 (1), 143. doi:10.1186/s40478-021-01245-z
Vorbrodt, A. W., and Trowbridge, R. S. (1991). Ultrastructural Study of Transcellular Transport of Native and Cationized Albumin in Cultured Sheep Brain Microvascular Endothelium. J. Neurocytol. 20 (12), 998–1006. doi:10.1007/BF01187917
Walling, B. L., and Kim, M. (2018). LFA-1 in T Cell Migration and Differentiation. Front. Immunol. 9, 952. doi:10.3389/fimmu.2018.00952
Walter, F. R., Santa-Maria, A. R., Mészáros, M., Veszelka, S., Dér, A., and Deli, M. A. (2021). Surface Charge, Glycocalyx, and Blood-Brain Barrier Function. Tissue Barriers 9 (3), 1904773. doi:10.1080/21688370.2021.1904773
Ward, T. H., Cummings, J., Dean, E., Greystoke, A., Hou, J. M., Backen, A., et al. (2008). Biomarkers of Apoptosis. Br. J. Cancer 99 (6), 841–846. doi:10.1038/sj.bjc.6604519
Weiss, N., Miller, F., Cazaubon, S., and Couraud, P.-O. (2009). The Blood-Brain Barrier in Brain Homeostasis and Neurological Diseases. Biochim. Biophys. Acta (Bba) - Biomembr. 1788 (4), 842–857. doi:10.1016/j.bbamem.2008.10.022
Wickman, G., Julian, L., and Olson, M. F. (2012). How Apoptotic Cells Aid in the Removal of Their Own Cold Dead Bodies. Cell Death Differ 19 (5), 735–742. doi:10.1038/cdd.2012.25
Wurdinger, T., Gatson, N. N., Balaj, L., Kaur, B., Breakefield, X. O., and Pegtel, D. M. (2012). Extracellular Vesicles and Their Convergence with Viral Pathways. Adv. Virol. 2012, 1–12. doi:10.1155/2012/767694
Xu, X., Lai, Y., and Hua, Z.-C. (2019). Apoptosis and Apoptotic Body: Disease Message and Therapeutic Target Potentials. Biosci. Rep. 39 (1), BSR20180992. doi:10.1042/BSR20180992
Yáñez-Mó, M., Siljander, P. R.-M., Andreu, Z., Bedina Zavec, A., Borràs, F. E., Buzas, E. I., et al. (2015). Biological Properties of Extracellular Vesicles and Their Physiological Functions. J. Extracell. Vesicles 4, 27066. doi:10.3402/jev.v4.27066
Yang, T., Martin, P., Fogarty, B., Brown, A., Schurman, K., Phipps, R., et al. (2015). Exosome Delivered Anticancer Drugs across the Blood-Brain Barrier for Brain Cancer Therapy in Danio rerio. Pharm. Res. 32 (6), 2003–2014. doi:10.1007/s11095-014-1593-y
Yu, S., Zhao, Z., Xu, X., Li, M., and Li, P. (2019). Characterization of Three Different Types of Extracellular Vesicles and Their Impact on Bacterial Growth. Food Chem. 272, 372–378. doi:10.1016/j.foodchem.2018.08.059
Yuyama, K., Sun, H., Mitsutake, S., and Igarashi, Y. (2012). Sphingolipid-modulated Exosome Secretion Promotes Clearance of Amyloid-β by Microglia. J. Biol. Chem. 287 (14), 10977–10989. doi:10.1074/jbc.M111.324616
Zabeo, D., Cvjetkovic, A., Lässer, C., Schorb, M., Lötvall, J., and Höög, J. L. (2017). Exosomes Purified from a Single Cell Type Have Diverse Morphology. J. Extracell. Vesicles 6 (1), 1329476. doi:10.1080/20013078.2017.1329476
Zaborowski, M. P., Balaj, L., Breakefield, X. O., and Lai, C. P. (2015). Extracellular Vesicles: Composition, Biological Relevance, and Methods of Study. Bioscience 65 (8), 783–797. doi:10.1093/biosci/biv084
Zanger, U. M., and Schwab, M. (2013). Cytochrome P450 Enzymes in Drug Metabolism: Regulation of Gene Expression, Enzyme Activities, and Impact of Genetic Variation. Pharmacol. Ther. 138 (1), 103–141. doi:10.1016/j.pharmthera.2012.12.007
Zhang, Y., Xu, B., Chen, Q., Yan, Y., Du, J., and Du, X. (2018). Apoptosis of Endothelial Cells Contributes to Brain Vessel Pruning of Zebrafish during Development. Front. Mol. Neurosci. 11, 222. doi:10.3389/fnmol.2018.00222
Zhao, Y., Xiong, S., Liu, P., Liu, W., Wang, Q., Liu, Y., et al. (2020). Polymeric Nanoparticles-Based Brain Delivery with Improved Therapeutic Efficacy of Ginkgolide B in Parkinson's Disease. Ijn Vol. 15, 10453–10467. doi:10.2147/IJN.S272831
Zhong, Y., Smart, E. J., Weksler, B., Couraud, P.-O., Hennig, B., and Toborek, M. (2008). Caveolin-1 Regulates Human Immunodeficiency Virus-1 Tat-Induced Alterations of Tight junction Protein Expression via Modulation of the Ras Signaling. J. Neurosci. 28 (31), 7788–7796. doi:10.1523/JNEUROSCI.0061-08.2008
Zhuang, X., Xiang, X., Grizzle, W., Sun, D., Zhang, S., Axtell, R. C., et al. (2011). Treatment of Brain Inflammatory Diseases by Delivering Exosome Encapsulated Anti-inflammatory Drugs from the Nasal Region to the Brain. Mol. Ther. 19 (10), 1769–1779. doi:10.1038/mt.2011.164
Zille, M., Ikhsan, M., Jiang, Y., Lampe, J., Wenzel, J., and Schwaninger, M. (2019). The Impact of Endothelial Cell Death in the Brain and its Role after Stroke: A Systematic Review. Cst 3 (11), 330–347. doi:10.15698/cst2019.11.203
Keywords: blood–brain barrier, brain endothelial cells, extracellular vesicles, nanoparticles, drug delivery
Citation: Dardet JP, Serrano N, András IE and Toborek M (2022) Overcoming Blood-Brain Barrier Resistance: Implications for Extracellular Vesicle-Mediated Drug Brain Delivery. Front. Drug. Deliv. 2:855017. doi: 10.3389/fddev.2022.855017
Received: 14 January 2022; Accepted: 03 March 2022;
Published: 24 March 2022.
Edited by:
Shinya Dohgu, Fukuoka University, JapanCopyright © 2022 Dardet, Serrano, András and Toborek. This is an open-access article distributed under the terms of the Creative Commons Attribution License (CC BY). The use, distribution or reproduction in other forums is permitted, provided the original author(s) and the copyright owner(s) are credited and that the original publication in this journal is cited, in accordance with accepted academic practice. No use, distribution or reproduction is permitted which does not comply with these terms.
*Correspondence: Ibolya Edit András, SUFuZHJhc0BtZWQubWlhbWkuZWR1; Michal Toborek, bXRvYm9yZWtAbWVkLm1pYW1pLmVkdQ==