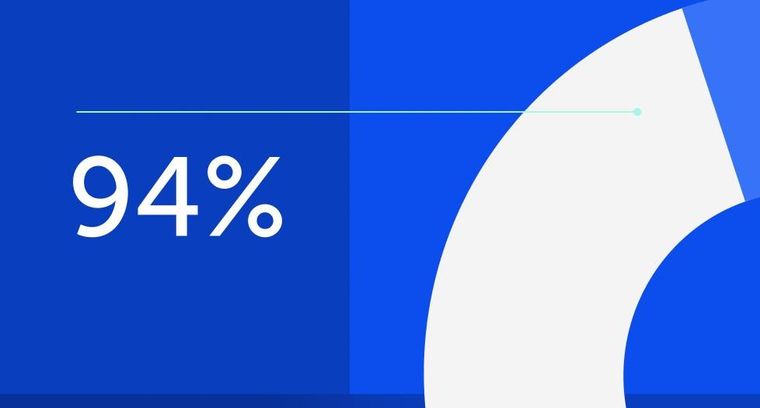
94% of researchers rate our articles as excellent or good
Learn more about the work of our research integrity team to safeguard the quality of each article we publish.
Find out more
ORIGINAL RESEARCH article
Front. Commun. Netw., 21 March 2025
Sec. Wireless Communications
Volume 6 - 2025 | https://doi.org/10.3389/frcmn.2025.1560311
This article is part of the Research TopicAntennas on the Engineered-Materials Substrate for Biomedical and Wireless Communication ApplicationsView all articles
The advancement of wireless technologies has led to significant progress in antenna design in order to meet the continuously increasing demands. Liquid antennas have gained significant interest in research owing to their distinctive properties, such as being small, flexible, transparent, and capable of reconfiguration. Recently, graphene liquid has been considered for various applications because of its affordability, excellent conductivity, flexibility, transparency, and easy processing. This paper presents a beam-reconfigurable graphene liquid antenna. The movement of the graphene liquid within the microfluidic channel enables beam reconfiguration. The antenna is realized in a rectangular microfluidic channel made of polymethyl methacrylate over a liquid crystal polymer substrate. The proposed antenna performs beam-steering up to
Over the past few years, wireless communication systems have undergone significant revolutionary advancements. Fifth-generation (5G) wireless communications networks have already been deployed with the aim of providing high data rates (Chowdhury et al., 2020). In particular, 5G has been formally commercialized since 2019, using the millimeter wave (mmWave) and sub-6 GHz bands. However, the rapid growth in the number of smart devices and the emergence of the Internet of Everything (IoE) applications, which require energy-efficient, ultra-reliable and low-latency communications, will substantially burden the 5G wireless networks (Alsabah et al., 2021; Chowdhury et al., 2020). Therefore, the 5G paradigm will be further developed and expanded under sixth-generation (6G) technologies that will pursue wider coverage, higher rates, more connections, ultra-low latency, ultra-high positioning accuracy, integration of communications and sensing, more intelligence, more security, and better substitutability (Chowdhury et al., 2020). The mmWave communication systems offer significant advantages in terms of high data rates and bandwidth, spectrum availability, beamforming capabilities, high spatial resolution, and reduced latency, making them essential for the 5G wireless communication networks (Hong et al., 2021). However, wireless communications over the mmWave bands are susceptible by bad weather conditions and obstacles due to the signal’s smaller wavelengths. Therefore, efficient antennas that overcome these limitations are important for the effective operation of future wireless systems.
In the present era of wireless communication systems, adaptability and diverse functionality stand out as the most attractive features in any communication device. Therefore, since the antenna is essential to these systems, the rapid progression towards 5G technologies necessitates the design of efficient antennas. Nevertheless, conventional antennas, usually made of conductive metals on stiff substrates, perform well but are not mechanically flexible. Moreover, exceeding certain limits in bending or stretching these antennas leads to irreversible structural deformations and even destruction (Alzoubi et al., 2011). As a consequence, metallic antennas’ rigidity limits their use in application where flexibility is required. This motivates the need for more flexible and adaptable antennas, such as antennas using metallic liquids (Huang et al., 2021). Metallic liquids are a perfect substitute for flexible antenna applications due to their flowing properties and lack of deformation limits. Moreover, liquid metals in microfluidic channels maintain exceptional flexibility and mechanical stability without compromising their electrical properties (So et al., 2009). Therefore, metallic liquid antennas are perfect for antenna applications because of their exceptional flexibility, deformability, and high conductivity. Modern methods of fabricating antennas take advantage of the fluidic qualities of metallic liquids, such as 3D printing, injecting, or spraying metallic liquid onto rigid or flexible substrates. Liquid antennas can readily achieve reconfigurability through electrochemically controlled capillary action or micro pumping, in contrast to traditional techniques like high-frequency switching. Given these important benefits, there has been significant interest recently on the beneficial role of liquid antennas in wireless communication systems (Psomas et al., 2023; Wong et al., 2021). The basis for developing efficient liquid antennas is found in the special qualities of liquid materials, which have a major impact on antenna performance and design.
Liquid antennas capitalize on the mechanical properties of fluids, leveraging their ability to change shape and flow to create flexible, reconfigurable, and adaptable antenna structures for various applications (Huang et al., 2021). The presence of metallic liquid in fluidic channels allows the fluidic channel to take shape due to its low viscosity (Choi, 2014). Flexible substrates allow for the bending, folding, stretching, and twisting of liquid antennas, thereby withstanding various forms of mechanical deformation. However, due to their high degree of reversibility, they can instantly regain their original form (So et al., 2009). Because of their intrinsic flexibility, liquid materials serve as a viable substitute for rigid or solid conductors in the realm of flexible electronics (Varnava, 2019). The development of metallic liquid antennas is made possible by the fluidic properties of metallic liquids. By using metallic liquids as radiative elements instead of solid conductors like copper, it is possible to create antennas that are much more flexible and reconfigurable. Moreover, the high conductivity inherent in metallic liquids makes them particularly well-suited for antenna applications. Indeed, the fluidic properties of metallic liquids have enabled a wide range of metallic liquid antennas (Kosta and ChaIurvedi, 1989; Dey et al., 2016; Hayes et al., 2012; So et al. 2009; Morishita et al., 2013). Even when radiative elements are installed on rigid substrates, more flexibility and reconfigurability can be achieved due to the fluidic nature of liquid materials. Metallic liquids have been used to develop flexible and reconfigurable antennas since the late 80s (Kosta and ChaIurvedi, 1989). One notable example of a metallic liquid is mercury (Hg). The fluidic and conductive nature of mercury allows for the design of reconfigurable metallic liquid antennas (Dey et al., 2016). However, the toxic nature and high cost of mercury impose limitations on its use for antennas. Alternative metallic liquid materials typically manifest as alloys composed of conductive nanoparticles. A well-known alloy for liquid antenna consists of gallium and indium has been explored in several antenna designs (Hayes et al., 2012; So et al., 2009; Morishita et al., 2013).
Recently, metallic liquid antennas employing graphene liquid, a novel metallic liquid material, have been designed (Dash et al., 2023). In comparison to traditional metallic liquid antennas made of mercury and gallium indium alloy (EGaIn), graphene-based liquid antennas exhibit superior electromagnetic performance. Since its discovery in 2004 Novoselov et al. (2004), the superior properties of graphene, including high electrical conductivity (=
Table 1 presents the material properties of graphene liquid in comparison to conventional metallic liquids such as mercury and EGaIn. The comparison highlights that graphene liquid is a promising candidate for liquid antennas, offering advantages over traditional metallic liquids. Graphene’s higher electrical conductivity and optical transparency provide significant advantages over EGaIn, particularly in applications where both high conductivity and optical transparency are required. Graphene’s higher conductivity makes it ideal for ultra-fast electronics, flexible circuits, and antennas. Graphene is nearly transparent, absorbing only 2.3% of visible light, making it an excellent material for transparent conductive films, touchscreens, and optoelectronic devices. EGaIn is completely opaque, limiting its use in applications requiring optical transparency. Moreover, graphene liquid proves to be safe for industrial use and is environmentally friendly. As a result, graphene liquid has many applications in various industries (Marlinda et al., 2023). The extensive research focus in recent years has led to the utilization of graphene inks for flexible electronics, wireless connectivity, and Internet of Things (IoT) applications (Yang and Wang, 2016; Pan et al., 2018). In addition to its flexibility, the stability and biocompatibility of graphene have gained interest as a promising candidate for applications in neuroscience, cardiac science, and biomedical engineering (Garcia-Cortadella et al., 2021; Gao et al., 2024). Therefore, it is no surprise that, during the last decade, graphene has been proven to be a well-known material for efficient antenna design (Dash et al., 2020; Dash and Patnaik, 2021). Nevertheless, designs for graphene-based liquid antennas remain unexplored. To the authors’ knowledge, graphene conductive liquid for beam reconfigurable antenna is considered in this work for the first time. Compared to conventional metallic liquid antennas that use mercury and EGaIn, graphene liquid antenna performs better in terms of gain, bandwidth, reflection coefficient, as well as radiation efficiency (Dash et al., 2023). Additionally, it offers reconfigurability and the potential for integration into advanced communication systems. These advantages make it a compelling alternative, particularly for applications requiring flexibility and dynamic reconfiguration. In this work, we introduce a new method for designing a beam-reconfigurable antenna that uses graphene liquid inside a microfluidic channel. The various states and reconfigurations of the proposed liquid antenna are made possible by the fluidic property of the graphene liquid. In the present work, the main beam is reconfigured in different directions by moving the graphene liquid to different locations. The movement of the graphene liquid within the microfluidic channel is used to investigate a reconfiguration mechanism for the antenna beams. The antenna is realized in a rectangular-shaped poly methyl methacrylate microfluidic channel over a liquid crystal polymer substrate. The primary contributions of the paper are provided below:
We design and numerically analyze the proposed graphene-based liquid antenna over mmWave frequency bands. The antenna consists the graphene liquid (
Figure 1 illustrate the proposed microfluidically graphene-based liquid antenna. The dimensions of the antenna are optimized for the operating frequency of 28 GHz. Table 2 displays the geometrical dimensions of antenna. The proposed antenna is designed, analyzed and its performance is validated using the FEM-based Ansoft HFSS software by ANSYS HFSS (2021). The center-fed single probe method is used to excite the antenna. The antenna’s ground plane (bottom layer) is a metal sheet that is electrically connected to an SMA connector’s external conductor. The feeding probe is electrically connected to the SMA’s internal conductor and inserts into the metallic liquid from its bottom center. In six locations P1, P2, P3, P4, P5, and P6, six SMA connectors are connected. The volume of liquid has a role in the frequency reconfiguration of the antenna and the radiation pattern is reconfigured by the movement of liquid at different locations within the microfluidic channel. In order to achieve antenna beam reconfigurability, the position of the graphene liquid relocates into different positions in the microfluidic channel.
Figure 1. Schematic of the proposed graphene-based liquid antenna. (a) 3D view. (b) Cross-sectional view.
Since this graphene liquid antenna is excited by only one port at a time, the antenna evaluates only S11 for the active port. The S11 parameter has been evaluated for return loss analysis, ensuring that reflection at the excited port is minimized for efficient radiation at the desired frequencies. S12 typically represents transmission between two simultaneously active ports, which does not apply in this case because, at any given time, only one port is excited. However, when the graphene liquid moves and shifts excitation to a second port, a new S11 measurement is performed for that configuration. This means that each state of the antenna has its own S11 evaluation, but no direct S12 measurement exists. In this design, where a single port is active at any time, the essential performance metrics include: S11 for impedance matching at each excitation state, radiation patterns to analyze beam steering effectiveness, gain and efficiency to evaluate antenna performance.
The Ansys HFSS, an FEM-based electromagnetic (EM) solver, is used to validate the proposed designed graphene-based liquid antenna with a resonant frequency of 28 GHz ANSYS HFSS (2021). The antenna is realized by considering a fixed volume (
For the modelling of the graphene liquid in the FEM-based EM solver, it is essential to model the conductive liquid with the surface conductivity
where
The graphene liquid flows in the microfluidic channel from one position to another. In the present work, six positions of graphene liquid in the microfluidic channel are considered. The center-fed single probe method is used to excite the antenna. The antenna is excited at the centre of graphene liquid radiating elements, like dipoles. In order to feed the graphene liquid antenna in a microfluidic channel, the external conductor of the SMA connector connected to the ground plane. The feeding probe is electrically connected to the inner conductor of the SMA and inserted into the graphene liquid from the bottom center, as shown in Figure 1b. To attain six working modes and beam reconfigurability, six feeding ports are created in six positions. Impedance matching must be accomplished in order to guarantee the antenna’s maximum radiation. Figure 3 illustrates the behavior of antenna impedance matching. It is evident that for each of the six graphene liquid positions, the antenna has a well-matched resonant frequency at 28 GHz.
The fabrication feasibility of the proposed graphene-based liquid antenna can be explained using Figure 2. The graphene liquid antenna can be mechanically supported by a silicon wafer sample. The graphene-based liquid antenna can be realized by injecting graphene liquid into a poly methyl methacrylate microfluidic channel (
Reconfigurability is one of the important advantages of the proposed graphene-based liquid antenna. The antenna’s operating frequency can be tuned by varying the volume and shape of the graphene liquid. The volume of liquid has a role in the frequency reconfiguration of the antenna and the radiation pattern is reconfigured by the movement of liquid at various positions within the microfluidic channel. By utilizing the micropump unit to alter the graphene liquid configuration inside the microfluidic channel, the antenna achieves beam reconfigurability.
The reflection coefficient of the microfluidically graphene-based liquid antenna at six different positions P1, P2, P3, P4, P5 and P6 are shown in Figure 3. It can be noticed that the proposed antenna resonates at 28 GHz in six different positions P1, P2, P3, P4, P5 and P6. The antenna resonant frequency remains the same for all six positions in the microfluidic channel. The antenna offers a wideband of 10-dB impedance bandwidth of
The volume of the graphene liquid in a microfluidic channel plays a significant role in operating at different frequencies. Figure 4 shows the reflection coefficient of antenna at different liquid volumes to illustrate how the liquid volume affects the antenna performance. The reflection performance of the graphene liquid antenna is analyzed for different volumes, with Lm varying between 2 mm and 5 mm. The antenna operates at different frequencies between 24 GHz and 30 GHz by varying the volume of the liquid in the microfluidic channel from Lm = 2 mm–5 mm, which is clearly marked in Figure 4. Furthermore, it can be noticed that an antenna with a smaller liquid volume leads to higher operational frequency, whereas an antenna with a higher liquid volume operates at a lower frequency. The antenna resonates at 30 GHz, 28 GHz, 26 GHz, and 24 GHz when Lm = 2 mm, 3 mm, 4 mm and 5 mm are considered, respectively. The resonant frequency decreases with the volume of liquid and can be dynamically controlled in a wide frequency range.
The proposed graphene-based liquid antenna at 28 GHz attains a unidirectional symmetrical radiation pattern with a gain of 7 dBi, as illustrated in Figure 5. The antenna’s gain and radiation efficiency over the frequency bands 10–45 GHz are shown in Figure 6. Over the considered frequency bands, the antenna’s radiation efficiency exceeds 60%. Figure 8 shows the normalized radiation patterns of the proposed graphene liquid antenna at 28 GHz. The proposed antenna provides a reduced back lobe radiation with front-to-back ratio of 10 dB, which can be observed in Figure 8. Beam reconfiguration and six different operation states are made possible by the flow of the graphene liquid into six distinct locations within the microfluidic channel. The proposed graphene-based liquid antenna with six beams is presented in Figure 7. The antenna’s normalized radiation patterns in six modes are shown in Figure 8. By appropriately choosing the location of the graphene liquid within the microfluidic channel, the antenna can be directed in D2
Figure 6. Radiation Gain and Radiation efficiency of the antenna over the frequency band 10–45 GHz. (a) Radiation Gain. (b) Radiation efficiency.
Figure 8. The normalized radiation pattern of the graphene-based liquid antenna in six working modes at 28 GHz.
With the growth of wireless communication networks, there have been significant technological advancements in antenna design in order to meet the ever-growing requirements of users Kumar et al. (2020). Therefore, the utilization of high-performance antennas to increase coverage and reduce the complexity of a system is required (Alibakhshikenari et al., 2022; Marasco et al., 2022; Marasco Parchin et al., 2023; Hasan et al., 2022). It is thus expected that 5G antennas will be effective in terms of polarization, gain/directivity, bandwidth, efficiency, etc.
Applications requiring mechanically flexible antennas can benefit from the use of liquid antennas. The conductive metals used to make conventional antennas, like copper, make them extremely effective but unfortunately rigid. On the other hand, liquid antennas are capable of providing the required flexibility and reconfigurability. For this reason, they have recently gained significant interest in the research community of wireless communications. Fluidic and conductivity characteristics are the primary determinants of metallic liquid antenna performance. As such, a graphene-based liquid antenna performs better than the EGaIn and Mercury liquid antenna counterparts, in terms of gain, bandwidth, reflection coefficient, and radiation efficiency (Dash et al., 2023).
Graphene-based liquid antennas represent a revolutionary advancement in the field of wireless communications and antenna technology. Graphene, a single layer of carbon atoms arranged in a hexagonal lattice, offers exceptional electrical, mechanical, and thermal properties. When graphene is in liquid form, it has highly conductive and flexible behaviour that can be used to create antennas with unprecedented characteristics. Graphene production techniques have advanced significantly, leading to more cost-effective methods for large-scale production. Additionally, the liquid nature of these antennas simplifies manufacturing processes and reduces material waste, further enhancing their cost-effectiveness and scalability compared to conventional antennas. Graphene-based liquid antennas can be easily modelled into different shapes and allow for seamless integration into a wide range of devices and structures. Graphene’s exceptional electronic characteristics allow for the development of antennas that can operate at a wide range of frequencies. This wideband performance is crucial for modern communication systems that must support multiple wireless standards and frequencies simultaneously. Graphene exhibits exceptional electrical conductivity and low electromagnetic losses, resulting in antennas with high efficiency and minimal signal degradation. This characteristic is particularly important for applications requiring long-range communications or operation in challenging environments with high interference or attenuation.
The overall electromagnetic performance of a liquid antenna is influenced by multiple parameters, including losses, flexibility, adaptability, reconfigurability, density, oxidation effect, and residue formation. Beyond conductivity, graphene-based liquid antennas offer additional advantages, such as lower density, higher mechanical flexibility, transparency, environmental stability and the ability to be easily processed in microfluidic channels. Graphene metallic liquids also offer advantages in terms of oxidation mitigation and reduced residue formation, which helps maintain stable electrical properties, ensuring better long-term reliability and environmental stability. Furthermore, graphene’s tunable conductivity enables dynamic impedance matching and beam reconfiguration, making it particularly well-suited for reconfigurable antenna applications. In contrast, EGaIn has certain limitations, such as higher density, susceptibility to oxidation, and potential toxicity, which can affect long-term performance. The high density and oxidation-prone nature of EGaIn alloys can degrade the antenna performance over time. Thus, graphene-based liquid antennas provide superior overall performance making them more suitable for next-generation reconfigurable and flexible wireless communication systems. The reconfigurability of graphene-based liquid antennas in a microfluidic channel is an additional benefit. Additional degrees of freedom are made possible by the fluidic nature of liquid materials, which improves reconfigurability. In graphene liquid antennas, frequency reconfiguration and beam reconfiguration can be accomplished by adjusting the liquid volume and liquid movement within the microfluidic channel at different locations. This tunability allows for adaptive antenna designs that can optimize performance based on network dynamics (e.g., due to mobility), changing environmental conditions or communication requirements. In this way, graphene-based liquid antennas address the need for high-performance, flexible, and adaptable antennas in modern communication systems. Their unique combination of properties offers significant advantages over conventional antennas, paving the way for 5G mmWave wireless communication system.
A microfluidically beam-reconfigurable directional antenna using graphene liquid for the mmWave wireless communication system was presented in this work. The reconfiguration mechanism of the proposed antenna is based on the movement of the graphene liquid inside the microfluidic channel. The antenna is realized in a rectangular-shaped poly methyl methacrylate microfluidic channel over a liquid crystal polymer substrate. The antenna is reconfigured in its radiation direction, covering up to 360° angles with six beams (
The original contributions presented in the study are included in the article/supplementary material, further inquiries can be directed to the corresponding author.
SD: Conceptualization, Data curation, Formal Analysis, Investigation, Methodology, Software, Supervision, Validation, Writing–original draft, Writing–review and editing. CP: Project administration, Resources, Supervision, Writing–review and editing, Conceptualization, Formal Analysis, Methodology, Validation, Visualization. IK: Funding acquisition, Project administration, Resources, Supervision, Visualization, Writing–review and editing, Conceptualization, Formal Analysis, Methodology, Validation.
The author(s) declare that financial support was received for the research, authorship, and/or publication of this article. This work has received funding from the European Union’s Horizon-JU-SNS research and innovation programme under the projects 6G-LEADER (Grant agreement No. 101192080) and iSEE-6G (Grant agreement No. 101139291). It has also received funding from the European Research Council (ERC) under the European Union’s Horizon 2020 research and innovation programme (Grant agreement No. 819819).
The authors declare that the research was conducted in the absence of any commercial or financial relationships that could be construed as a potential conflict of interest.
The author(s) declared that they were an editorial board member of Frontiers, at the time of submission. This had no impact on the peer review process and the final decision.
The author(s) declare that no Generative AI was used in the creation of this manuscript.
All claims expressed in this article are solely those of the authors and do not necessarily represent those of their affiliated organizations, or those of the publisher, the editors and the reviewers. Any product that may be evaluated in this article, or claim that may be made by its manufacturer, is not guaranteed or endorsed by the publisher.
Alibakhshikenari, M., Virdee, B. S., Benetatos, H., Ali, E. M., Soruri, M., Dalarsson, M., et al. (2022). An innovative antenna array with high inter element isolation for sub-6 GHz 5G MIMO communication systems. Sci. Rep. 12, 7907–7913. doi:10.1038/s41598-022-12119-2
Alsabah, M., Naser, M. A., Mahmmod, B. M., Abdulhussain, S. H., Eissa, M. R., Al-Baidhani, A., et al. (2021). 6G wireless communications networks: a comprehensive survey. IEEE Access 9, 148191–148243. doi:10.1109/access.2021.3124812
Alzoubi, K., Hamasha, M. M., Schadt, M., Lu, S., Sammakia, B., and Poliks, M. (2011). “Effect of lamination on the bending fatigue life of copper coated PET substrate,” in Advances in display technologies; and E-papers and flexible displays (San Francisco, CA: International Society for Optics and Photonics SPIE), 7956, 79560X. doi:10.1117/12.874226
Bazzi, A., and Chafii, M. (2025). Low dynamic range for ris-aided bistatic integrated sensing and communication. IEEE J. Sel. Areas Commun., 1. doi:10.2139/ssrn.5126060
Choi, J. (2014). “Materials for flexible and printed electronics,” in Materials matter, aldrich materials science sigma-aldrich Co. LLC.
Chowdhury, M. Z., Shahjalal, M., Ahmed, S., and Jang, Y. M. (2020). 6G wireless communication systems: applications, requirements, technologies, challenges, and research directions. IEEE Open J. Commun. Soc. 1, 957–975. doi:10.1109/ojcoms.2020.3010270
Dash, S., Liaskos, C., Akyildiz, I. F., and Pitsillides, A. (2020). “Nanoantennas design for thz communication: material selection and performance enhancement,” in Proceedings of the 7th ACM international conference on nanoscale computing and communication (New York, NY: Association for Computing Machinery)
Dash, S., and Patnaik, A. (2021). “Advancements in terahertz antenna design and their performances,” in Next generation terahertz wireless communication networks (USA: Taylor and Francis Group, CRC Press).
Dash, S., Psomas, C., and Krikidis, I. (2023). Selection of metallic liquid in sub-6 GHz antenna design for 6G networks. Sci. Rep. 13, 20551. doi:10.1038/s41598-023-47870-7
Dey, A., Guldiken, R., and Mumcu, G. (2016). Microfluidically reconfigured wideband frequency-tunable liquid-metal monopole antenna. IEEE Trans. Antennas Propag. 64, 2572–2576. doi:10.1109/tap.2016.2551358
Dhote, C., Sharma, P. K., and Singh, A. (2023). “Graphene-based reconfigurable intelligent surface for 6g wireless communication,” in 2023 IEEE microwaves, antennas, and propagation conference (MAPCON), 1–6.
Gao, H., Wang, Z., Yang, F., Wang, X., Wang, S., Zhang, Q., et al. (2024). Graphene-integrated mesh electronics with converged multifunctionality for tracking multimodal excitation-contraction dynamics in cardiac microtissues. Nat. Commun. 15, 2321. doi:10.1038/s41467-024-46636-7
Garcia-Cortadella, R., Schwesig, G., Jeschke, C., Illa, X., Gray, A. L., Savage, S., et al. (2021). Graphene active sensor arrays for long-term and wireless mapping of wide frequency band epicortical brain activity. Nat. Commun. 12, 211. doi:10.1038/s41467-020-20546-w
Gusynin, V., Sharapov, S. G., and Carbotte, J. P. (2006). Magneto-optical conductivity in graphene. J. Phys. Cond. Matter 19, 026222. doi:10.1088/0953-8984/19/2/026222
Hasan, M. M., Islam, M. T., Samsuzzaman, M., Baharuddin, M. H., Soliman, M. S., Alzamil, A., et al. (2022). Gain and isolation enhancement of a wideband mimo antenna using metasurface for 5G sub-6 GHz communication systems. Sci. Rep. 12, 9433–9517. doi:10.1038/s41598-022-13522-5
Hayes, G. J., So, J.-H., Qusba, A., Dickey, M. D., and Lazzi, G. (2012). Flexible liquid metal alloy (EGaIn) microstrip patch antenna. IEEE Trans. Antennas Propag. 60, 2151–2156. doi:10.1109/tap.2012.2189698
Hong, W., Jiang, Z. H., Yu, C., Hou, D., Wang, H., Guo, C., et al. (2021). The role of millimeter-wave technologies in 5g/6g wireless communications. IEEE J. Microwaves 1, 101–122. doi:10.1109/jmw.2020.3035541
Huang, Y., Xing, L., Song, C., Wang, S., and Elhouni, F. (2021). Liquid antennas: past, present and future. IEEE Open J. Antennas Propag. 2, 473–487. doi:10.1109/ojap.2021.3069325
Kosta, Y. P., ChaIurvedi, B. K., et al. (1989). “A liquid patch microwave antenna,” in Proceedings of NACONECS-1989, dept. Of electronics and computer engineering, university of roorkee (India: Tata McGrawhill), 43–47.
Kumar, S., Dixit, A. S., Malekar, R. R., Raut, H. D., and Shevada, L. K. (2020). Fifth Generation antennas: a comprehensive review of design and performance enhancement techniques. IEEE Access 8, 163568–163593. doi:10.1109/access.2020.3020952
Ling, K., Kim, H. K., Yoo, M., and Lim, S. (2015). Frequency-switchable metamaterial absorber injecting eutectic gallium-indium (EGaIn) liquid metal alloy. Sensors 15, 28154–28165. doi:10.3390/s151128154
Marasco, I., Niro, G., Mastronardi, V. M., Rizzi, F., De Vittorio, M., Grande, M., et al. (2022). A compact evolved antenna for 5G communications. Sci. Rep. 12, 10327–10411. doi:10.1038/s41598-022-14447-9
Marasco Parchin, N. O., Mohamed, H. G., Moussa, K. H., See, C. H., Abd-Alhameed, R. A., and Alwadai, A. A. S. N. M. (2023). An efficient antenna system with improved radiation for multi-standard/multi-mode 5G cellular communications. Sci. Rep. 13, 4179–4215. doi:10.1038/s41598-023-31407-z
Marlinda, A. R., Thien, G. S. H., Shahid, M., Ling, T. Y., Hashem, A., Chan, K.-Y., et al. (2023). Graphene as a lubricant additive for reducing friction and wear in its liquid-based form. Lubricants 11, 29–27. doi:10.3390/lubricants11010029
Molero, C., Palomares-Caballero, Ã., Alex-Amor, A., Parellada-Serrano, I., Gamiz, F., Padilla, P., et al. (2021). Metamaterial-based reconfigurable intelligent surface: 3d meta-atoms controlled by graphene structures. IEEE Commun. Mag. 59, 42–48. doi:10.1109/mcom.001.2001161
Morishita, A. M., Kitamura, C. K. Y., Ohta, A. T., and Shiroma, W. A. (2013). A liquid-metal monopole array with tunable frequency, gain, and beam steering. IEEE Antennas Wirel. Propag. Lett. 12, 1388–1391. doi:10.1109/lawp.2013.2286544
Novoselov, K. S., Geim, A. K., Morozov, S. V., Jiang, D., Zhang, Y., Dubonos, S. V., et al. (2004). Electric field effect in atomically thin carbon films. Science 306, 666–669. doi:10.1126/science.1102896
Pan, K., Fan, Y., Leng, T., Li, J., Xin, Z., Zhang, J., et al. (2018). Sustainable production of highly conductive multilayer graphene ink for wireless connectivity and iot applications. Nat. Commun. 9, 5197. doi:10.1038/s41467-018-07632-w
Psomas, C., Smith, P. J., Suraweera, H., and Krikidis, I. (2023). Continuous fluid antenna systems: modeling and analysis. IEEE Commun. Lett. 27, 3370–3374. doi:10.1109/lcomm.2023.3330157
Rodrigo, D., Jofre, L., and Cetiner, B. A. (2012). Circular beam-steering reconfigurable antenna with liquid metal parasitics. IEEE Trans. Antennas Propag. 60, 1796–1802. doi:10.1109/tap.2012.2186235
So, H., Thelen, J., Qusba, A., Hayes, G. J., Lazzi, G., and Dickey, M. D. (2009). Reversibly deformable and mechanically tunable fluidic antennas. Adv. Funct. Mater. 19, 3632–3637. doi:10.1002/adfm.200900604
Sruti, A. N., and Jagannadham, K. (2010). Electrical conductivity of graphene composites with in and In-Ga alloy. J. Electron. Mater. 39, 1268–1276. doi:10.1007/s11664-010-1208-2
Varnava, C. (2019). Liquid metals take stretchable circuits to new heights. Nat. Electron. 2, 52. doi:10.1038/s41928-019-0217-2
Wan, Z., Zeng, H., and Feinerman, A. (2006). Reversible electrowetting of liquid-metal droplet. J. Fluids Eng. 129, 388–394. doi:10.1115/1.2436582
Wang, D., Bazzi, A., and Chafii, M. (2024). “Ris-enabled integrated sensing and communication for 6g systems,” in 2024 IEEE wireless communications and networking conference (WCNC), 1–6.
Wong, K.-K., Shojaeifard, A., Tong, K.-F., and Zhang, Y. (2021). Fluid antenna systems. IEEE Trans. Wirel. Commun. 20, 1950–1962. doi:10.1109/twc.2020.3037595
Xia, Y., and Whitesides, G. M. (1998). Soft lithography. Angew. Chem. Int. Ed. 37, 550–575. doi:10.1002/(sici)1521-3773(19980316)37:5<550::aid-anie550>3.3.co;2-7
Keywords: graphene liquid, microfluidic, antenna, mmwave, beam reconfiguration, wireless communications
Citation: Dash S, Psomas C and Krikidis I (2025) Graphene-based beam-reconfigurable liquid antenna for 5G mmWave wireless systems. Front. Commun. Netw. 6:1560311. doi: 10.3389/frcmn.2025.1560311
Received: 14 January 2025; Accepted: 12 March 2025;
Published: 21 March 2025.
Edited by:
Muhammad Ikram, King Abdullah University of Science and Technology, Saudi ArabiaReviewed by:
Ahmad Bazzi, New York University Abu Dhabi, United Arab EmiratesCopyright © 2025 Dash, Psomas and Krikidis. This is an open-access article distributed under the terms of the Creative Commons Attribution License (CC BY). The use, distribution or reproduction in other forums is permitted, provided the original author(s) and the copyright owner(s) are credited and that the original publication in this journal is cited, in accordance with accepted academic practice. No use, distribution or reproduction is permitted which does not comply with these terms.
*Correspondence: Sasmita Dash, c2FzbWl0YWRhc2gzMEBnbWFpbC5jb20=
Disclaimer: All claims expressed in this article are solely those of the authors and do not necessarily represent those of their affiliated organizations, or those of the publisher, the editors and the reviewers. Any product that may be evaluated in this article or claim that may be made by its manufacturer is not guaranteed or endorsed by the publisher.
Research integrity at Frontiers
Learn more about the work of our research integrity team to safeguard the quality of each article we publish.