- 1Department of Inorganic and Analytical Chemistry, University of Geneva, Geneva, Switzerland
- 2Laboratory of Crystallography, University of Geneva, Geneva, Switzerland
- 3Department of Inorganic Chemistry, University of Granada, Unidad de Excelencia de Química (UEQ), Granada, Spain
A series of highly emissive inert and chiral CrIII complexes displaying positive and negative circularly polarized luminescence (CPL) within the near-infrared (NIR) region at room temperature have been prepared and characterized to decipher the effect of ligand substitution on the photophysical properties, more specifically on the chiroptical properties. The helical homoleptic [Cr(dqp-R)2]3+ (dqp = 2,6-di(quinolin-8-yl)pyridine; R = Ph, ≡-Ph, DMA, ≡-DMA (DMA = N,N-dimethylaniline)) and heteroleptic [Cr(dqp)(L)]3+ (L = 4-methoxy-2,6-di(quinolin-8-yl)pyridine (dqp-OMe) or L = N2,N6-dimethyl-N2,N6-di(pyridin-2-yl)pyridine-2,6-diamine (ddpd)) molecular rubies were synthesized as racemic mixtures and then resolved and isolated into their respective pure PP and MM enantiomeric forms by chiral stationary phase HPLC. The corresponding enantiomers show two opposite polarized emission bands within the 700–780 nm range corresponding to the characteristic metal-centered Cr(2E’→4A2) and Cr(2T1’→4A2) transitions with large glum ranging from 0.14 to 0.20 for the former transition. In summary, this study reports the rational use of different ligands on CrIII and their effect on the chiroptical properties of the complexes.
1 Introduction
Chiral chromophores displaying intense circularly polarized luminescence (CPL) signal and high CPL brightness (BCPL) are up-and-coming candidates for applications as molecular probes in biological systems (Carr et al., 2012; Staszak et al., 2019), in bio-imaging (Heffern et al., 2014), in light-emitting devices (e.g., CP-OLEDs) (Brandt et al., 2017; Zinna et al., 2017; Furlan et al., 2024), and in counterfeiting agents (e.g., security inks) (MacKenzie and Pal, 2021). The dissymmetry factor glum is used to estimate the excess of emitted right- or left-circularly polarized light in an isotropic solution. It is deduced from IL and IR, which represent the emission intensities of left and right circularly polarized light, respectively (Equation 1 center, Richardson, 1979; Arrico et al., 2020). This parameter is directly related to the electric dipole (
An important number of photons must be detected to apply chiral chromophores in CPL materials. To account for that, Zinna and coworkers introduced the CPL brightness (BCPL), which combines the molar absorption extinction coefficient (ελexc), the photoluminescence quantum yield (ϕPL), and the dissymmetry factor in Equation 2 (Arrico et al., 2020).
Improving BCPL is a current challenge as commonly bright luminescent molecules (displaying high ελexc and ϕPL) are usually accompanied with weak glum, as rationalized by Equation 1 because intense ED-allowed transitions (ligand-to-metal charge transfer, metal-to-ligand charge transfer, or π* → π transitions) imply
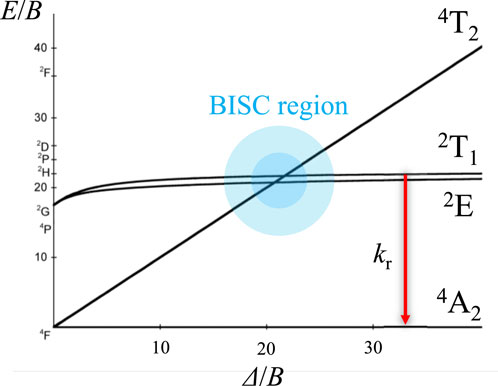
Figure 1. Simplified Tanabe–Sugano diagram for a d3 electronic configuration in an octahedral field with C/B = 4.5. Two deexcitation pathways are shown: radiative (kr, red arrow) and back intersystem crossing (BISC, blue domain). A strong LFS prevents the non-radiative deexcitation of the excited state through BISC.
Despite uniting the searched intrinsic kinetic inertness with emissive spin-flip transitions, the design of chiral CrIII complexes with adapted absorption and emission properties remains scarce. Recently, the meridional coordination of bis-terdentate six-membered chelates rings to CrIII has been demonstrated to be an interesting approach for inducing intense spin-flip chiroptical responses (Jimenez et al., 2019; Jimenez et al., 2023). In contrast, introducing chiral centers in the ligand scaffold did not lead to strong CPL emission in related complexes (Poncet et al., 2021; Cheng et al., 2023). In this context, the recent chiral resolution of the inert emissive complexes [Cr(dqp)2]3+ (dqp = 2,6-di(quinolin-8-yl)pyridine) (Jimenez et al., 2019) and [Cr(ddpd)2]3+ (ddpd = N2,N6-dimethyl-N2,N6-di(pyridin-2-yl)pyridine-2,6-diamine) (Dee et al., 2019) displaying large dissymmetry factors reactivated the interest for this topic. Concomitant high quantum yields could be obtained, making cheap and earth-abundant chromium assemblies promising candidates for CPL applications.
This work follows this strategy with the synthesis of four novel homoleptic complexes [Cr(dqp-R)2]3+ (R = Ph (1), ≡-Ph (2), DMA (3), and ≡-DMA (4), DMA = N,N-dimethylaniline). The photophysics of these complexes can be compared with those of the heteroleptic complexes [Cr(dqp)(dqp-OMe)]3+ (5, dqp-OMe = 4-methoxy-2,6-di(quinolin-8-yl)pyridine) and [Cr(dqp)(ddpd)]3+ (6) (Jimenez et al., 2020) The enantiomeric resolutions proved to be successful for all complexes, which paved the way for addressing their chiroptical properties.
2 Results and discussion
The ligands dqp-OMe, ddpd, and dqp; the salt Cr(CF3SO3)2·2H2O; and the heteroleptic complexes 5 and 6 were prepared according to published methods (Supplementary Appendix 1; Cantuel et al., 2002; Otto et al., 2015; Jimenez et al., 2019; Jiménez et al., 2020). The preparation of the homoleptic complexes [Cr(dqp-R)2]3+ is achieved by mixing one equivalent of CrII precursor and two equivalents of the corresponding ligands dqp-R (dqp-Ph for 1, dqp-≡-Ph for 2, dqp-DMA for 3, and dqp-≡-DMA for 4) under anaerobic conditions at room temperature. The formed rac-[CrII(dqp-R)2]2+ are oxidized to the kinetically inert rac-[CrIII(dqp-R)2]3+ using AgSO3CF3, affording the desired complexes in good-to-excellent yields (74%–96%, Figure 2).
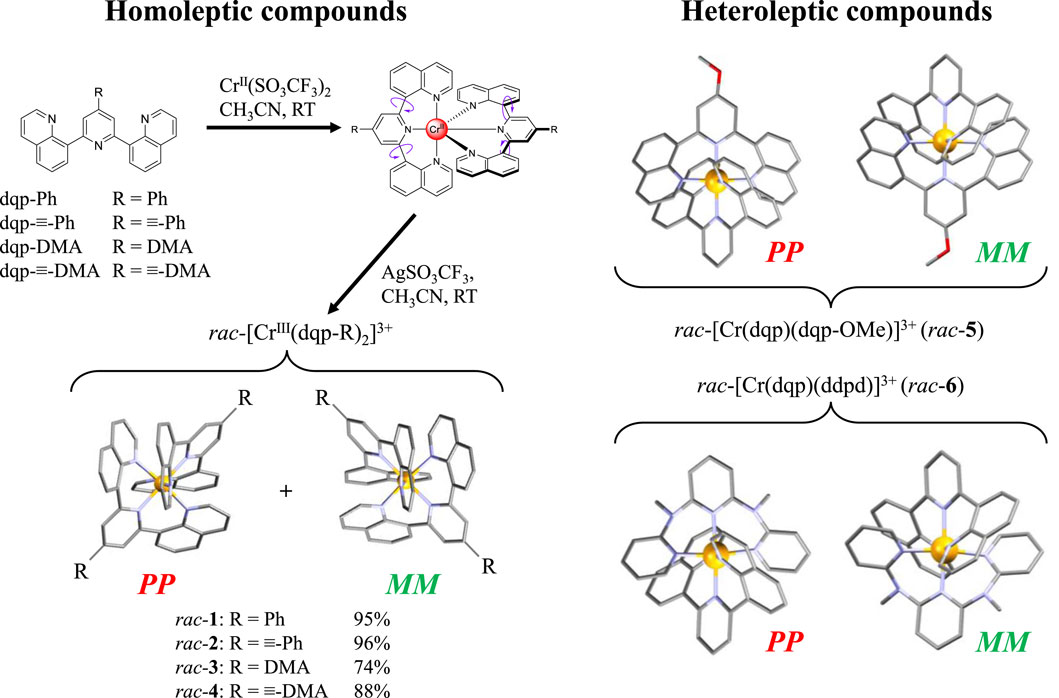
Figure 2. Synthesis of homoleptic complexes rac-[Cr(dqp-R)2]3+. Racemic mixtures of the PP and MM enantiomers are isolated for both homoleptic and heteroleptic complexes.
Single crystals suitable for X-ray diffraction analysis (Supplementary Tables S1–S13; Supplementary Figures S1–S7 in the Supplementary Material) were obtained through slow diffusion of diethyl ether into a concentrated methanol solution for 1, 2, 3 (with triflate counterions), and 4 (with chlorine counterions). The dqp derivatives systematically adopt the thermodynamically more stable meridional binding around the kinetic labile CrII intermediate, leading to meridional arrangement in the final CrIII complexes after oxidation. Under acidic conditions, the tertiary amine groups in 3 and 4 can be further protonated to yield [Cr(dqp-DMAH)2]5+ (H2-3, DMAH = N,N-dimethylanilininum) and [Cr(dqp-≡-DMAH)2]5+ (H2-4). Single crystals suitable for X-ray diffraction of both protonated complexes could also be successfully isolated by crystallization. The semi-flexible nature of the dqp backbone upon mer binding joined with the kinetic inertness of CrIII results in the formation of racemic mixtures of helical PP and MM enantiomers of D2-symmetry in the crystals (Figure 2). As previously reported, the two instances of intramolecular, interligand π-stacking between the aromatic quinolines produce stabilizing interactions compatible with the exclusive formation of the PP and MM enantiomers (Jimenez et al., 2021). No meso PM complex could be observed, likely due to steric hindrance, as simulated in the parent [Cr(dqp)2]3+ (Jimenez et al., 2021). To better appreciate the intramolecular interligand π-stacking, the interplanar angle is calculated as the angle between the mean plane of the 10 atoms of each quinoline (Supplementary Figures S8–S15). The closer the angle is to 0, the more parallel to each other the quinolines are. The calculated values for the complexes 1–5, [H2-3] and [H2-4] lie within the 15.54°–16.68° range. In the heteroleptic complex [Cr(dqp)(ddpd)]3+ (6), the corresponding quinoline–pyridine interplanar angle reaches 31.48°, far larger than in the other compounds, but yet smaller than the parent [Cr(ddpd)2]3+ (46.52°) (Otto et al., 2015). The transoid bite angles N-Cr-N are in the 176.0(1)°–177.7(8)° range for the homoleptic complexes (1–4, H2-3, and H2-4) and in the 175.5(9)°–176.1(10)° range for the heteroleptic complexes (5 and 6). In addition, to evaluate the structural distortion with respect to a perfect octahedron, the following parameter
The absorption spectra of the homoleptic complexes (1–4) were recorded in water at room temperature. Complexes H2-3 and H2-4 were recorded in acidic media (aqueous HCl 1 M) to ensure full protonation of the terminal tertiary amine (Figure 3).
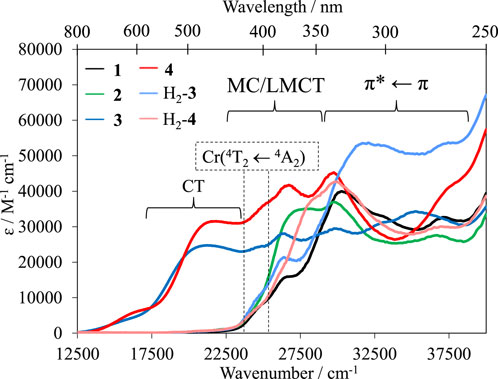
Figure 3. Absorption spectra of complexes 1–4 in H2O and H2-3 and H2-4 in aqueous HCl (1 M) at 293 K.
The maxima observed within the 345–250 nm range (29,000–40,000 cm−1) are associated with π*←π transitions located on the ligands. Ligand-to-metal charge transfers (LMCTs) are observed at lower energies from 435 nm to 345 nm (23,000–29,000 cm−1). Additionally, a shoulder is observed within the 420–400 nm range (23,800–25,000 cm−1), which has been assigned to the spin-allowed, parity-forbidden metal-centered (MC) Cr(4T2←4A2) transition according to TD-DFT calculations performed on the parent [Cr(dqp)2]3+ complex (Jimenez et al., 2019). Because Δ = E(Cr(4T2←4A2)) in octahedral complexes, the extracted energy values point to similar ligand-field splitting for all complexes within the 24,272–24,876 cm−1 range (Supplementary Table S14). Complexes 3 and 4 display an additional intense broad absorption band in the visible range of the electromagnetic spectrum 800–435 nm (12,500–23,000 cm−1) assigned to the intraligand charge transfer (ILCT) from the terminal nitrogen of the aniline to the trivalent chromium center, as observed in related terpyridine-based complexes (Barbour et al., 2017). Ensuring full protonation using acidic conditions (aqueous 1 M HCl) removes the CT band in H2-3 and H2-4 (Figure 3). Moving down in energy and using an increased concentration of complexes, the spin-forbidden/parity-forbidden spin-flip (SF) transitions Cr(2T1,2E←4A2) can be observed with low molar extinction coefficients ranging from 0.1 M−1 cm−1 to 0.6 M−1 cm−1.
Because of the slightly distorted geometry going from Oh to D2, a splitting of the two expected bands produces five distinct excited energy levels. The Cr(2T1) splits into three non-degenerated energy levels, and the Cr(2E) splits into two (Figure 4; Supplementary Figures S16–S19) (Jimenez et al., 2023). Recording the SF bands was impossible in complexes 3 and 4 because of the overlap with the intense charge transfer absorption band in the visible region of the spectrum. Individual assignments of the absorption bands together with experimental radiative rate constants krad, radiative lifetimes τrad, oscillator strengths fexp, and dipole strengths Dexp are compiled in Supplementary Table S14. From the absorption spectra and the calculated energies of the accessible excited levels, the ligand-field parameter Δ and the Racah parameters B and C can be estimated using Equations 3–6 (Supplementary Table S15) (Jorgensen, 1963; Witzke, 1971; Chong et al., 2022).
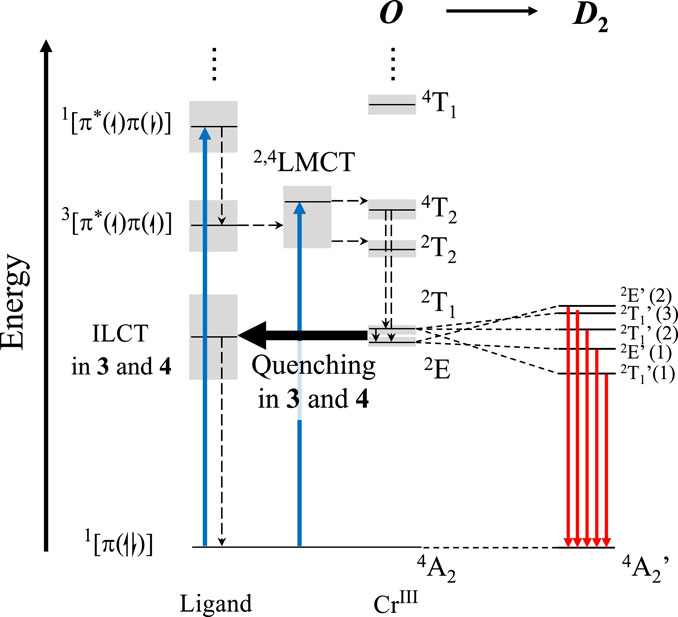
Figure 4. Perrin–Jablonski diagram for a CrIII in O geometry. Excitation (blue arrows), internal conversion (dashed arrows), ILCT quenching in 3 and 4 (black arrow), and expected emission in a D2 distorted geometry (red arrow) are represented. The splitting of the 2T1 and 2E energy levels leads to five expected optically active energy levels in D2 symmetry.
For all studied complexes, B ranges from 611 cm−1 to 655 cm−1, and C ranges from 2,743 cm–−1 to 2,885 cm−1, which implies a weak impact of the extension of the π-delocalized conjugated system contrariwise to a previously reported substitution of methoxy groups in the same position in the homoleptic [Cr(dqp-OMe)2]3+ (Jimenez et al., 2021). We note that for the [Cr(dqp)2]3+, the ratio C/B equals 4.7, but the ratio is only 3.1 for [Cr(dqp-OMe)2]3+. Typical values are in the range of 4.2–4.9 and are sometimes assumed to be 4.7 (Adachi, 2024).
Upon UV–VIS excitation (λexc = 350–435 nm) at room temperature, the typical sharp NIR dual emissions (FWHM ≈200 cm−1) observed in complexes 1 and 2 (Figure 5A) can be assigned to the radiative relaxation of the Cr(2E) and Cr(2T1) excited state levels to the Cr(4A2) ground state level in approximate O symmetry (Jimenez et al., 2019; Jimenez et al., 2021). The most intense band is attributed to the Cr(2T1’→4A2) SF transition (maxima 13,227 cm−1 (756 nm) in 1 and 2) and the less intense to Cr(2E’→4A2) (maxima at 13,698 cm−1 (730 nm) for 1 and 13,661 cm−1 (732 nm) for 2). Contrariwise, the luminescence of complexes 3 and 4 is completely quenched, likely due to energy back transfer into the non-emissive CT levels (Figure 4). Upon protonation of the anilines (H2-3 and H2-4), a weak luminescence is retrieved with an overall photoluminescence quantum yield of ΦPL ≤ 0.0011% (Supplementary Table S16; Figure 5A), in contrast to the non-luminescent terpyridine analog (Barbour et al., 2017).
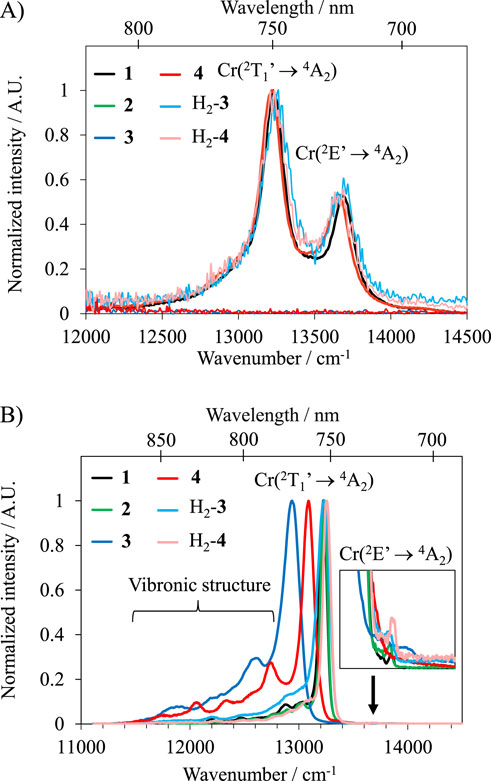
Figure 5. Near-infrared emission spectra with λexc = 435 nm of (A) Complexes 1–4 in H2O and H2-3 and H2-4 in aqueous 1 M HCl at 293 K and (B) Complexes 1–4 in DMSO/H2O 1:1 and H2-3 and H2-4 in aqueous 1 M HCl at 77 K.
Upon changing the temperature, the Boltzmann distribution of the thermally equilibrated Cr(2E’) and Cr(2T1’) levels is modified, and 77 K measurements result in a close to single emission assigned to Cr(2T1’→4A2) (Figure 5B). Interestingly, the total (3 and 4) and partial (H2-3 and H2-4) quenching pathways of the luminescence happening at 293 K vanish at 77 K, and strong luminescence is recovered (Figure 5B). For both H2-3 and H2-4 at 77 K, the main emission band is blue-shifted compared to the non-protonated 3 and 4 complexes. The room temperature ΦPL values determined in aerobic and anaerobic conditions (CH3CN, λexc = 435 nm) are gathered in Supplementary Table S16. The obtained values in oxygen-free solutions are 12.4%, making complexes 1 and 2 in the same range as the previously reported record-holding deuterium-free CrIII complexes (Jimenez et al., 2019; Jimenez et al., 2021). The origin of the high ΦPL observed is attributed to (i) the weak trigonal distortion forming the octahedral geometry, preventing the non-radiative deexcitation pathways (Kitzmann et al., 2022) and (ii) the strong LFS induced by the dqp-type ligands, avoiding BISC to the Cr(4T2) excited state level (Figure 1).
Time-resolved experiments were conducted and displayed mono-exponential decays, resulting in
Additionally, the rate of energy transfer from Cr(2E’/2T1’) to O2, kq, can be estimated in 1 and 2 with the relationship kq = 1/[O2]⋅(1/τair −1/τAr) (Burgin et al., 2022), in which [O2] is the oxygen concentration in the solvent at the experimental temperature (2.42 mM in CH3CN at 293 K), τair is the Cr(2E’/2T1’) lifetime under air-equilibrated conditions, and τAr is the respective lifetime under deaerated conditions. The obtained values of 6.23∙106 s−1 (1) and 7.59∙106 s−1 (2) demonstrate the effectiveness of O2 quenching (Supplementary Table S16).
The thermal equilibrium of the Cr(2T1’) and Cr(2E’) levels was confirmed by the recording of identical excited state lifetimes at both maxima. The sensitization efficiency for transferring the energy from the ligand to the Cr(2E’/2T1’) excited state
Chiral stationary phase high-performance liquid chromatography (CSP-HPLC) was proven to be effective, straightforward, and quick in the separation of the racemic mixture of d-block complexes such as RuIII, CrIII, and CoIII (Yoshida et al., 2013; Cortijo et al., 2017; Dee et al., 2019). Isocratic elution using a CH2Cl2/CH3CH3OH/triethylamine/trifluoroacetic acid 50/49.2/0.5/0.3 (v:v) mixture resulted in the separation of the complexes 1–6 (Figures 6A–F). For complexes 1, 2, and 5, the elutions of MM enantiomers tail and slightly overlap with the elution peaks of the PP enantiomers. As a result, a small amount of contamination was observed upon reinjection in the analytical column (Supplementary Figures S30–S32). Nevertheless, thorough integrations of the chromatogram reveal that ≤0.5% mol was present in the sample. Therefore, the chiroptical studies were carried out while considering these contaminations as negligible. The obtention of a mirror image of the circular dichroism (CD) and CPL signals confirmed the adequacy of the latter assumption. Note that the efficient separation of 3 and 4 would allow a larger scale separation.
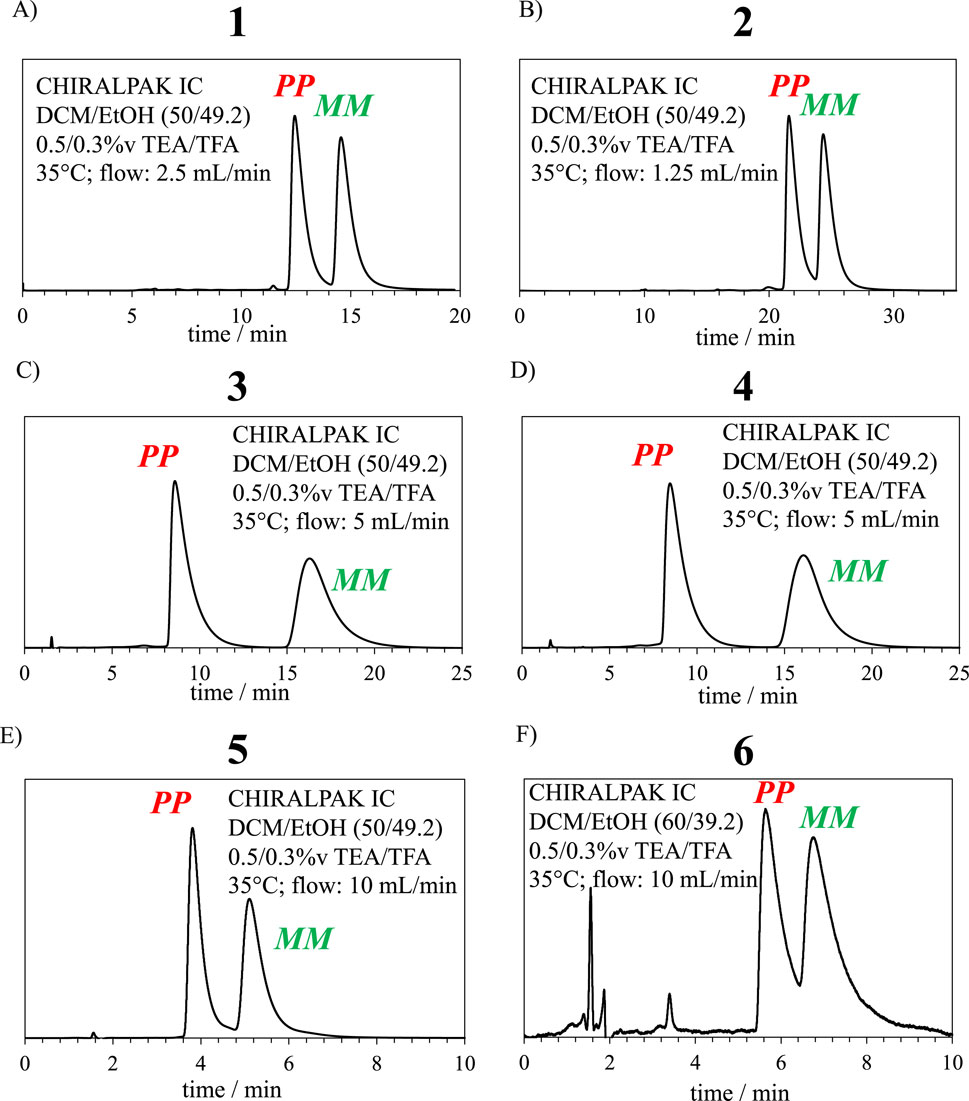
Figure 6. CSP-HPLC conditions required for the enantiomeric resolution of complexes (A) 1, (B) 2, (C) 3, (D) 4, (E) 5, and (F) 6.
The CD was recorded, and mirror images were systematically obtained for all complexes 1–6, H2-3, and H2-4 (Figures 7, 8). The study of the analogous crystallized MM-[Cr(dqp)2]3+ in CD allowed the assignment of the measured complexes (Jimenez et al., 2019). In all complexes except for 6 (Figure 8D), a strong Cotton effect could be observed in the 410–430 nm range, corresponding to the MC Cr(4T2←4A2) transition. The UV range is also dominated by considerable Cotton effect matching with π*←π transitions located on the ligands. Despite the significant absorption of the CT bands in complexes 3 and 4 (ε = 25,000–32000 M−1cm−1), little to no Cotton effect was observed, confirming the low gabs for these specific transitions (Figures 7A, C). Because 3 and 4 could be protonated in solution, their conversion to the respective protonated species H2-3 and H2-4 could be followed by CD upon successive addition of hydrochloric acid (0.05 M) in the aqueous solution (Figures 7E, F). The MC ligand-field Cr(4T2←4A2) transition is less affected by the protonation of the ligands than the π*←π transitions, which display important changes in the 250–400 nm range.
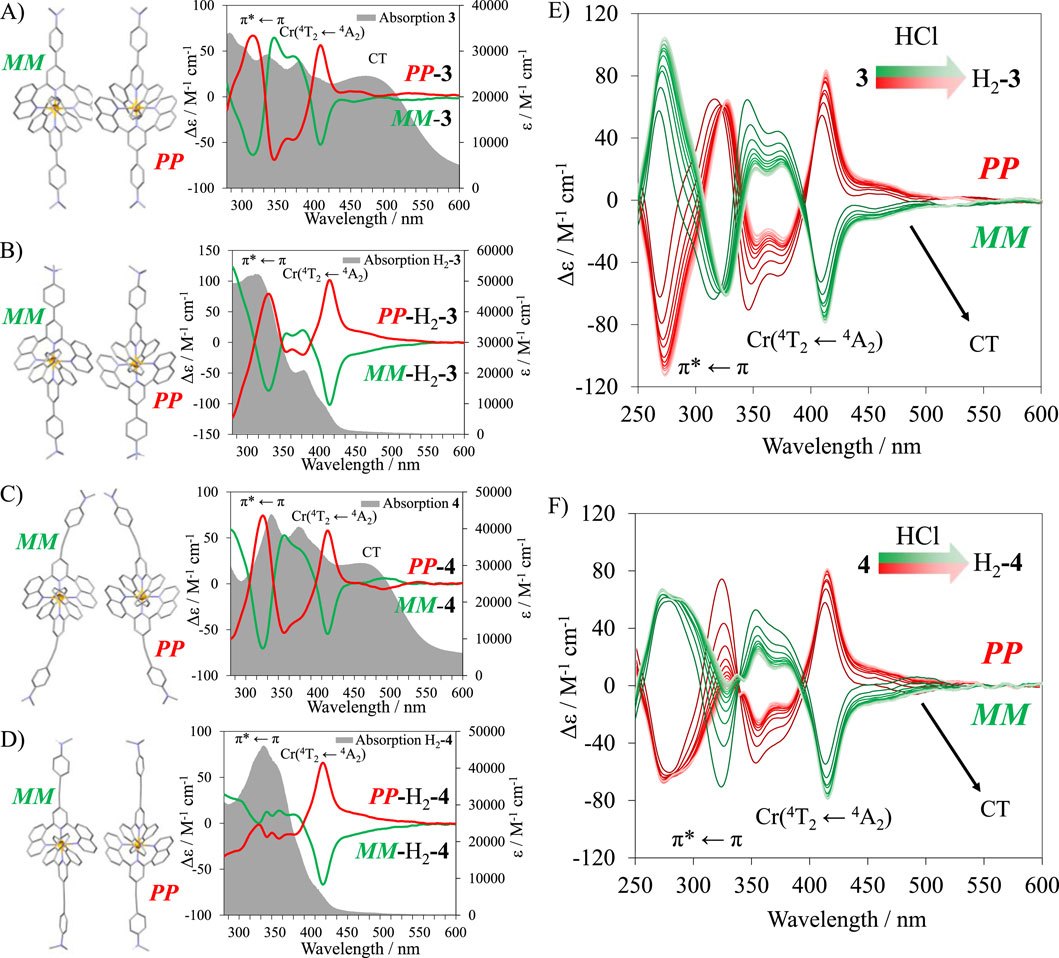
Figure 7. Crystalline structures of PP and MM enantiomers and the corresponding absorption (gray traces) and CD spectra (red and green traces) for (A) 3, (B) H2-3, (C) 4, and (D) H2-4. CD titration of complexes (E) 3 to H2-3 and (F) 4 to H2-4 in aqueous media.
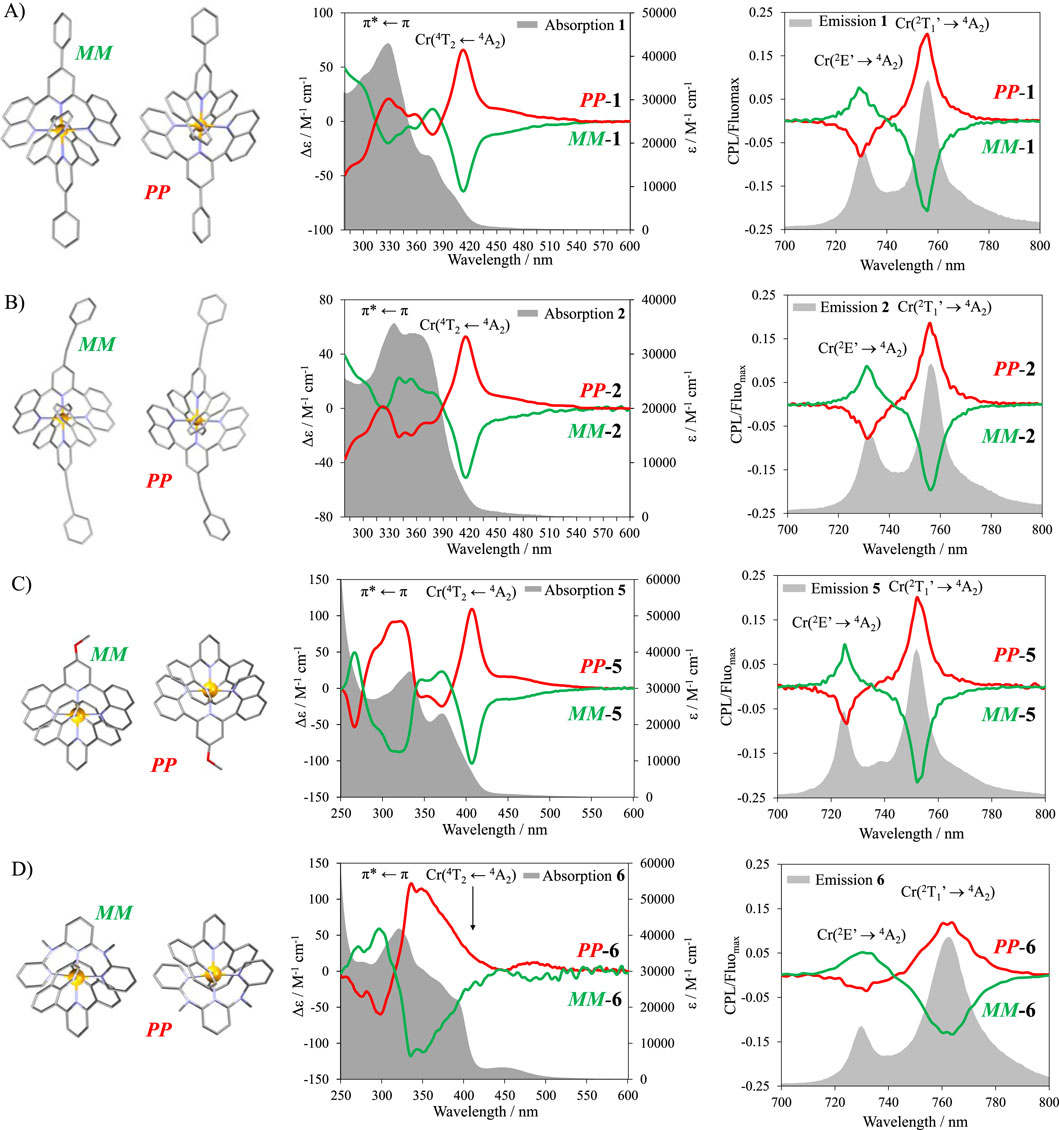
Figure 8. Crystalline structure of the PP and MM enantiomers and the corresponding CD (middle, red, and green traces) and CPL (λexc = 340 nm, right, (red and green traces)) spectra for (A) 1, (B) 2, (C) 5, and (D) 6. The experimental bandwidth (EBW) for the CPL spectra in A–C is 0.5 nm and 5 nm for (D). The gray traces show the absorption (middle) and emission (right) spectra.
Circularly polarized luminescence (CPL) measurements were recorded on each enantiomer of the emissive complexes 1, 2, 5, and 6 (Figures 8A–D). The low photoluminescence quantum yield of H2-3 and H2-4 and the non-emissive character of 3 and 4 prevented CPL measurements. To ensure a correct determination of the dissymmetry factor, the highest resolution of the CPL spectra is ensured with the smallest emission slit aperture settings available at the cost of the signal intensity (Sickinger et al., 2024). The experimental bandwidth (EBW) used in these experiments is 0.5 nm for 1, 2, and 5 and 5 nm for 6. A better signal-to-noise ratio can be obtained by opening the slits (larger EBW), but the resolution of the spectra must be sacrificed, skewing the results and the value of glum. It is, therefore, more correct to measure the sample at the smallest slit aperture available and divide the CPL spectra by the maximum read value of the corresponding emission. Under unpolarized excitation (λexc = 340 nm), complexes 1, 2, 5, and 6 (Figures 8A–D) displayed strong circularly polarized emission within the 720–780 nm range. The dissymmetry factors |glum| obtained for the Cr(2E’→4A2) transition reach 0.07–0.08 for all four complexes (Supplementary Figures S33–S36). Interestingly, for the Cr(2T1’→4A2) transition, complexes 1, 2, and 5 display |glum| = 0.20, whereas complex 6 reaches only |glum| = 0.14.
Putting these results in perspective with those of the previously reported homoleptic complexes of [Cr(ddpd)2]3+ and [Cr(dqp-R)2]3+ (R = H, Br, OMe, C≡CH, Table 1) (Otto et al., 2015; Jimenez et al., 2019; Jimenez et al., 2021), a trend emerges for the Cr(2T1’→4A2) transitions. Indeed, all complexes containing two dqp-based ligands display the same dissymmetry factor of 0.2, whereas the more flexible [Cr(ddpd)2]3+ culminated at 0.093 (Dee et al., 2019). By exploiting the chemical inertness of CrIII, one of each ligand could be implemented in the same complex to give the heteroleptic complex [Cr(dqp)(ddpd)]3+ (6), for which |glum| = 0.14 corresponds to the average of the dissymmetry factors of the two parent homoleptic complexes. As an attempt to rationalize this result, a hypothetical “ligand dissymmetry factor”
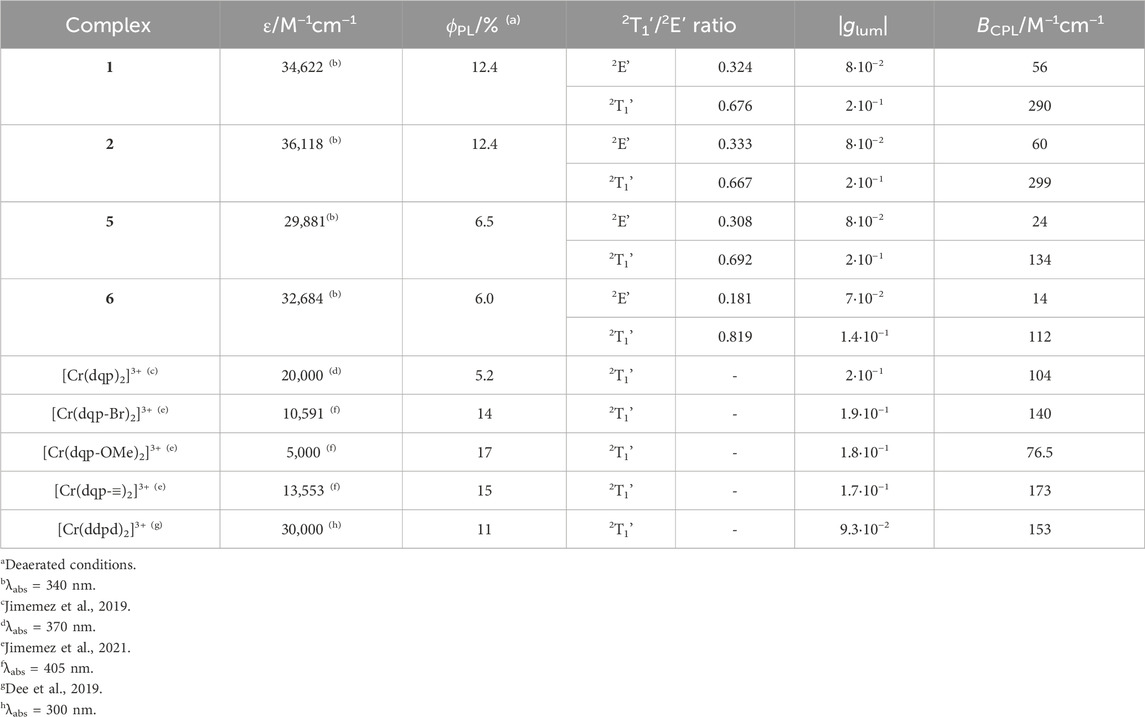
Table 1. CPL brightness (BCPL) calculation for each emissive transition in complexes 1, 2, 5, and 6.
For 2, one obtains
The BCPL of the emissive complexes can be calculated using Equation 2. Because the glum values associated with the two observed emissions Cr(2T1’→4A2) and Cr(2E’→4A2) are of opposite signs in the same enantiomer, BCPL must be similarly split into two components. Accordingly, the ϕPL must be split relative to the intensity of each band at the temperature measurement (293 K). The emission spectrum of the corresponding complex is approximated as two Gaussian curves, deconvoluted as such, and the ratio between them is calculated (idealized Cr(2T1→4A2) and Cr(2E→4A2) transitions, Supplementary Figures S37–S40). The calculated values of BCPL are compiled in Table 1 and range from 299 M−1cm−1 to 122 M−1cm−1 for the Cr(2T1’→4A2) transition and 60–14 M−1cm−1 for the Cr(2E’→4A2) transition, which are among the highest reported values for CPL active compounds (Arrico et al., 2020). A higher BCPL can be achieved by exciting the maxima of the absorption band to increase the value of ε in the BCPL calculation. The ϕPL is considered invariant with the excitation wavelength.
3 Conclusion
A series of new chiral homoleptic and heteroleptic CrIII chromophores with dqp-based ligands with para functionalization of the central pyridine have been synthesized and characterized. The addition of N,N-dimethylaniline to the complex resulted in a large increase in absorbance in the VIS region (CT) accompanied by quenching of the luminescence. Interestingly, weak luminescence is retrieved upon protonation of the aniline (ΦPL ≤ 0.0011%). The highly luminescent complexes [Cr(dqp-Ph)2]3+ and [Cr(dqp-≡-Ph)2]3+ are promising candidates for use as chiral luminescent probes. Enantiomeric resolution of all six racemic complexes could be achieved by CSP-HPLC. Implementing N,N-dimethylaniline as a substituent to the complex resulted in a baseline separation of the two enantiomers, allowing a potential large-scale separation. Moreover, the evolution of the circular dichroism from the non-protonated to the protonated species in [Cr(dqp-DMA)2]3+/[Cr(dqp-DMAH)2]5+ and [Cr(dqp-≡-DMA)2]3+/[Cr(dqp-≡-DMAH)2]5+ could be followed. Near-perfect octahedral geometries are obtained with the help of the six-membered chelate rings, providing long excited state lifetime and high overall photoluminescence quantum yields at room temperature. Dual circularly polarized luminescence arises from the Cr(2T1’) and Cr(2E’) excited level to the Cr(4A2) ground state within the 720–780 nm range. The observed |glum| for the emissive complexes amounts to 0.2 except for [Cr(dqp)(ddpd)]3+, for which the |glum| was measured to be in between the two corresponding parent homoleptic complexes [Cr(dqp)2]3+ (|glum| = 0.2) and [Cr(ddpd)2]3+ (|glum| = 0.093). High BCPL values, ranging from 299 −1cm−1 to 122 M−1cm−1 for the Cr(2T1’→4A2) transition and 60–14 M−1cm−1 for the Cr(2E’→4A2) transition, were obtained, reaching the typical range of 4f-based chiral chromophores with the added value of the inertness and low cost of trivalent chromium.
Data availability statement
The original contributions presented in the study are included in the article/Supplementary Material, further inquiries can be directed to the corresponding authors.
Author contributions
MP: conceptualization, formal analysis, investigation, and writing–original draft. CB: formal analysis and writing–review and editing. LG: formal analysis and writing–review and editing. J-RJ: conceptualization, supervision, validation, and writing–review and editing. CP: conceptualization, funding acquisition, supervision, validation, and writing–review and editing.
Funding
The author(s) declare that financial support was received for the research, authorship, and/or publication of this article. This work is supported through grants from the Swiss National Science Foundation (grant 200020_207313). Financial support from the Swiss National Science Foundation is gratefully acknowledged. J-RJ acknowledges the funding received from the grant TED2021.129598A.I00 funded by MCIN/AEI/10.13039/501100011033 and by European Union NextGenerationEU/PRTR and is grateful to the Ministerio de Ciencia Innovación y Universidades for a Ramón y Cajal contract (grant RYC 2022-037255-I) funded by MCIN/AEI/10.13039/501100011033 and FSE+.
Acknowledgments
K. L. Buchwalder is acknowledged for performing elemental analysis.
Conflict of interest
The authors declare that the research was conducted in the absence of any commercial or financial relationships that could be construed as a potential conflict of interest.
Publisher’s note
All claims expressed in this article are solely those of the authors and do not necessarily represent those of their affiliated organizations, or those of the publisher, the editors, and the reviewers. Any product that may be evaluated in this article, or claim that may be made by its manufacturer, is not guaranteed or endorsed by the publisher.
Supplementary material
The Supplementary Material for this article can be found online at: https://www.frontiersin.org/articles/10.3389/fchem.2024.1472943/full#supplementary-material
References
Adachi, S. (2024). Practical consideration on Racah parameter and Tanabe−Sugano diagram analyses for Mn4+ and Cr3+-activated phosphors. J. Luminescence 273, 120628. doi:10.1016/j.jlumin.2024.120628
Alazaly, A. M. M., Clarkson, G. J., Ward, M. D., and Abdel-Shafi, A. A. (2023). Mechanism of oxygen quenching of the excited states of heteroleptic chromium(III) phenanthroline derivatives. Inorg. Chem. 62, 16101–16113. doi:10.1021/acs.inorgchem.3c02343
Albano, G., Pescitelli, G., and Di Bari, L. (2020). Chiroptical properties in thin films of π-conjugated systems. Chem. Rev. 120, 10145–10243. doi:10.1021/acs.chemrev.0c00195
Arrico, L., Di Bari, L., and Zinna, F. (2020). Quantifying the overall efficiency of circularly polarized emitters. Chem. – A Eur. J. 27, 2920–2934. doi:10.1002/chem.202002791
Barbour, J. C., Kim, A. J. I., deVries, E., Shaner, S. E., and Lovaasen, B. M. (2017). Chromium(III) bis-arylterpyridyl complexes with enhanced visible absorption via incorporation of intraligand charge-transfer transitions. Inorg. Chem. 56, 8212–8222. doi:10.1021/acs.inorgchem.7b00953
Brandt, J. R., Salerno, F., and Fuchter, M. J. (2017). The added value of small-molecule chirality in technological applications. Nat. Rev. Chem. 1, 0045. doi:10.1038/s41570-017-0045
Burgin, T. H., Glaser, F., and Wenger, O. S. (2022). Shedding light on the oxidizing properties of spin-flip excited states in a Cr(III) polypyridine complex and their use in photoredox catalysis. J. Am. Chem. Soc. 144, 14181–14194. doi:10.1021/jacs.2c04465
Cantuel, M., Bernardinelli, G., Imbert, D., Bünzli, J.-C. G., Hopfgartner, G., and Piguet, C. (2002). A kinetically inert and optically active Cr(III) partner in thermodynamically self-assembled heterodimetallic non-covalent d-f podates. J. Chem. Soc. Dalton Trans., 1929–1940. doi:10.1039/B200011C
Carr, R., Evans, N. H., and Parker, D. (2012). Lanthanide complexes as chiral probes exploiting circularly polarized luminescence. Chem. Soc. Rev. 41, 7673–7686. doi:10.1039/C2CS35242G
Cheng, Y., He, J., Zou, W., Chang, X., yang, Q., and Lu, W. (2023). Circularly polarized near-infrared phosphorescence of chiral chromium(III) complexes. Chem. Commun. 59, 1781–1784. doi:10.1039/D2CC06548G
Chong, J., Besnard, C., Cruz, C. M., Piguet, C., and Jimenez, J. R. (2022). Heteroleptic mer-[Cr(NNN)(CN)3] complexes: synthetic challenge, structural characterization and photophysical properties. Dalton Trans. 51, 4297–4309. doi:10.1039/d2dt00126h
Cortijo, M., Viala, C., Reynaldo, T., Favereau, L., Fabing, I., Srebro-Hooper, M., et al. (2017). Synthesis, spectroelectrochemical behavior, and chiroptical switching of tris(β-diketonato) complexes of ruthenium(III), chromium(III), and cobalt(III). Inorg. Chem. 56, 4555–4567. doi:10.1021/acs.inorgchem.6b03094
Dee, C., Zinna, F., Kitzmann, W. R., Pescitelli, G., Heinze, K., Di Bari, L., et al. (2019). Strong circularly polarized luminescence of an octahedral chromium(III) complex. Chem. Comm. 55, 13078–13081. doi:10.1039/c9cc06909g
Furlan, F., Moreno-Naranjo, J. M., Gasparini, N., Feldmann, S., Wade, J., and Fuchter, M. J. (2024). Chiral materials and mechanisms for circularly polarized light-emitting diodes. Nat. Photonics 18, 658–668. doi:10.1038/s41566-024-01408-z
Gauthier, E. S., Abella, L., Hellou, N., Darquié, B., Caytan, E., Roisnel, T., et al. (2020). Long-lived circularly polarized phosphorescence in helicene-NHC rhenium(I) complexes: the influence of helicene, halogen, and stereochemistry on emission properties. Angew. Chem. Int. Ed. 59, 8394–8400. doi:10.1002/anie.202002387
Hara, N., Okuda, K., Shizuma, M., Tajima, N., and Imai, Y. (2019). Control of circularly polarised luminescence using a suitable wired structure connecting a binaphthyl with two pyrenes. ChemistrySelect 4, 10209–10213. doi:10.1002/slct.201902176
Heffern, M. C., Matosziuk, L. M., and Meade, T. J. (2014). Lanthanide probes for bioresponsive imaging. Chem. Rev. 114, 4496–4539. doi:10.1021/cr400477t
Helm, L., and Merbach, A. E. (2005). Inorganic and bioinorganic solvent exchange mechanisms. Chem. Rev. 105, 1923–1960. doi:10.1021/cr030726o
Homberg, A., Brun, E., Zinna, F., Pascal, S., Górecki, M., Monnier, L., et al. (2018). Combined reversible switching of ECD and quenching of CPL with chiral fluorescent macrocycles. Chem. Sci. 9, 7043–7052. doi:10.1039/C8SC02935K
Jimenez, J. R., Doistau, B., Cruz, C. M., Besnard, C., Cuerva, J. M., Campana, A. G., et al. (2019). Chiral molecular ruby [Cr(dqp)2](3+) with long-lived circularly polarized luminescence. J. Am. Chem. Soc. 141, 13244–13252. doi:10.1021/jacs.9b06524
Jimenez, J. R., Miguez-Lago, S., Poncet, M., Ye, Y. T., Ruiz, C. L., Cruz, C. M., et al. (2023). Eu-III functionalized silica nanoparticles encapsulating chiral Cr-III complexes with simultaneous unpolarized red and polarized NIR-I luminescence. J. Mater. Chem. C 11, 2582–2590. doi:10.1039/d2tc05344f
Jiménez, J.-R., Poncet, M., Doistau, B., Besnard, C., and Piguet, C. (2020). Luminescent polypyridyl heteroleptic CrIII complexes with high quantum yields and long excited state lifetimes. Dalton Trans. 49, 13528–13532. doi:10.1039/D0DT02872J
Jimenez, J. R., Poncet, M., Miguez-Lago, S., Grass, S., Lacour, J., Besnard, C., et al. (2021). Bright long-lived circularly polarized luminescence in chiral chromium(III) complexes. Angew. Chem. Int. Ed. 60, 10095–10102. doi:10.1002/anie.202101158
Jorgensen, C. K. (1963). Spectroscopy of transition-group complexes. Adv. Chem. Phys. 5, 33–146. doi:10.1002/9780470143513.ch2
Kirk, A. D. (1999). Photochemistry and photophysics of chromium(III) complexes. Chem. Rev. 99, 1607–1640. doi:10.1021/cr960111+
Kitzmann, W. R., Ramanan, C., Naumann, R., and Heinze, K. (2022). Molecular ruby: exploring the excited state landscape. Dalton Trans. 51, 6519–6525. doi:10.1039/d2dt00569g
Lunkley, J. L., Shirotani, D., Yamanari, K., Kaizaki, S., and Muller, G. (2008). Extraordinary circularly polarized luminescence activity exhibited by cesium tetrakis(3-heptafluoro-butylryl-(+)-camphorato) Eu(III) complexes in EtOH and CHCl3 solutions. J. Am. Chem. Soc. 130, 13814–13815. doi:10.1021/ja805681w
MacKenzie, L. E., and Pal, R. (2021). Circularly polarized lanthanide luminescence for advanced security inks. Nat. Rev. Chem. 5, 109–124. doi:10.1038/s41570-020-00235-4
Maiman, T. H. (1960). Stimulated optical radiation in ruby. Nature 187, 493–494. doi:10.1038/187493a0
Mori, T. (2020). Circularly polarized luminescence of isolated small organic molecules. Singapore: Springer. doi:10.1007/978-981-15-2309-0
Nagata, Y., and Mori, T. (2020). Irreverent nature of dissymmetry factor and quantum yield in circularly polarized luminescence of small organic molecules. Front. Chem. 8, 448. doi:10.3389/fchem.2020.00448
Nakanishi, S., Nakabayashi, K., Mizusawa, T., Suzuki, N., Guo, S., Fujiki, M., et al. (2016). Cryptochiral binaphthyl–bipyrene luminophores linked with alkylene esters: intense circularly polarised luminescence, but ultraweak circular dichroism. RSC Adv. 6, 99172–99176. doi:10.1039/C6RA20342F
Otto, S., Grabolle, M., Förtser, C., Kreitner, C., Resh-Genger, U., and Heinze, K. (2015). [Cr(ddpd)2]3+: a molecular, water-soluble, highly NIR-emisssive Ruby analogue. Angew. Chem. Int. Ed. 54, 11572–11576. doi:10.1002/anie.201504894
Poncet, M., Benchohra, A., Jimenez, J. R., and Piguet, C. (2021). Chiral chromium(III) complexes as promising candidates for circularly polarized luminescence. Chemphotochem 5, 880–892. doi:10.1002/cptc.202100146
Reiné, P., Justicia, J., Morcillo, S. P., Abbate, S., Vaz, B., Ribagorda, M., et al. (2018). Pyrene-containing ortho-Oligo(phenylene)ethynylene foldamer as a ratiometric probe based on circularly polarized luminescence. J. Org. Chem. 83, 4455–4463. doi:10.1021/acs.joc.8b00162
Richardson, F. S. (1979). Theory of optical activity in the ligand-field transitions of chiral transition metal complexes. Chem. Rev. 79, 17–42. doi:10.1021/cr60317a003
Richens, D. T. (2005). Ligand substitution reactions at inorganic centers. Chem. Rev. 105, 1961–2002. doi:10.1021/cr030705u
Saleh, N., Shen, C., and Crassous, J. (2014). Helicene-based transition metal complexes: synthesis, properties and applications. Chem. Sci. 5, 3680–3694. doi:10.1039/C4SC01404A
Sang, Y., Han, J., Zhao, T., Duan, P., and Liu, M. (2020). Circularly polarized luminescence in nanoassemblies: generation, amplification, and application. Adv. Mater. 32, 1900110. doi:10.1002/adma.201900110
Sato, S., Yoshii, A., Takahashi, S., Furumi, S., Takeuchi, M., and Isobe, H. (2017). Chiral intertwined spirals and magnetic transition dipole moments dictated by cylinder helicity. Proc. Natl. Acad. Sci. 114, 13097–13101. doi:10.1073/pnas.1717524114
Sickinger, A., Baguenard, B., Bensalah-Ledoux, A., Guyot, Y., Guy, L., Pointillart, F., et al. (2024). Impact of the experimental bandwidth on circularly polarized luminescence measurements of lanthanide complexes: the case of erbium(iii). J. Mater. Chem. C 12, 4253–4260. doi:10.1039/D3TC04717B
Sinha, N., Jimenez, J. R., Pfund, B., Prescimone, A., Piguet, C., and Wenger, O. S. (2021). A near-infrared-II emissive chromium(III) complex. Angew. Chem. Int. Ed. 60, 23722–23728. doi:10.1002/anie.202106398
Song, J., Xiao, H., Fang, L., Qu, L., Zhou, X., Xu, Z.-X., et al. (2022). Highly phosphorescent planar chirality by bridging two square-planar platinum(II) complexes: chirality induction and circularly polarized luminescence. J. Am. Chem. Soc. 144, 2233–2244. doi:10.1021/jacs.1c11699
Staszak, K., Wieszczycka, K., Marturano, V., and Tylkowski, B. (2019). Lanthanides complexes – chiral sensing of biomolecules. Coord. Chem. Rev. 397, 76–90. doi:10.1016/j.ccr.2019.06.017
Ward, M. D. (2010). Mechanisms of sensitization of lanthanide(III)-based luminescence in transition metal/lanthanide and anthracene/lanthanide dyads. Coord. Chem. Rev. 254, 2634–2642. doi:10.1016/j.ccr.2009.12.001
Witzke, H. (1971). Semi-empirical evaluations of the Racah B and C parameters from the crystal field spectra of chromium(III) complexes. Theor. Chim. Acta 20, 171–185. doi:10.1007/BF00569263
Yoshida, J., Watanabe, G., Kakizawa, K., Kawabata, Y., and Yuge, H. (2013). Tris(β-diketonato) Ru(III) complexes as chiral dopants for nematic liquid crystals: the effect of the molecular structure on the helical twisting power. Inorg. Chem. 52, 11042–11050. doi:10.1021/ic401240f
Yoshida, K., Kajiwara, M., Okazaki, Y., Véronique, L., Zinna, F., Sojic, N., et al. (2024). Modulation of circularly polarized luminescence by swelling of microgels functionalized with enantiopure [Ru(bpy)3]2+ luminophores. Chem. Commun. 60, 1743–1746. doi:10.1039/D3CC04391F
Keywords: chromium, chirality, emission, circularly polarized luminescence, circular dichroism, chiral HPLC
Citation: Poncet M, Besnard C, Guénée L, Jiménez J-R and Piguet C (2024) Tuning the circularly polarized luminescence in homoleptic and heteroleptic chiral CrIII complexes. Front. Chem. 12:1472943. doi: 10.3389/fchem.2024.1472943
Received: 30 July 2024; Accepted: 20 September 2024;
Published: 09 October 2024.
Edited by:
Fabio Piccinelli, University of Verona, ItalyReviewed by:
Marco M. Allard, La Sierra University, United StatesJatish Kumar, Indian Institute of Science Education and Research, India
Yu-Wu Zhong, Chinese Academy of Sciences (CAS), China
Copyright © 2024 Poncet, Besnard, Guénée, Jiménez and Piguet. This is an open-access article distributed under the terms of the Creative Commons Attribution License (CC BY). The use, distribution or reproduction in other forums is permitted, provided the original author(s) and the copyright owner(s) are credited and that the original publication in this journal is cited, in accordance with accepted academic practice. No use, distribution or reproduction is permitted which does not comply with these terms.
*Correspondence: Claude Piguet, Y2xhdWRlLnBpZ3VldEB1bmlnZS5jaA==; Juan-Ramón Jiménez, anJqaW1lbmV6QHVnci5lcw==