- School of Pharmacy, Weifang Medical University, Weifang, China
A novel and efficient C3-H vinylation reaction with quinoxalin-2(1H)-one as the substrate, in the presence of alkenes, under metal-free conditions, is reported herein. The reaction leads to the formation of new carbon–carbon bonds that exhibit moderate to good reactivities. The vinylation of quinoxalin-2(1H)-ones, in the presence of alkenes, is an attractive process that can be potentially utilized to produce biologically active 3-vinylated quinoxalin-2(1H)-ones.
Introduction
For Recent years have seen, the emergence of cross-dehydrocoupling (CDC) reaction, between two different molecules, as a prominent research topic (Girard et al., 2014; Huang et al., 2019; Mane et al., 2019; Liu et al., 2020; Xu et al., 2020). These reactions exploit the C-H bonds of various substrates, during the dehydrogenation coupling reactions under oxidizing reaction conditions, to form C-C bonds (Niu et al., 2015; Cheng et al., 2017; Yuan et al., 2018; Xie et al., 2020). The cross-dehydrocoupling reactions provide shorter synthetic routes and new research ideas for the direct and efficient synthesis of complex organic materials from simple raw materials. High atom efficiency can also be achieved (Scheuermann, 2010; Moon et al., 2012; Jiang et al., 2014; Parvatkar et al., 2019).
Quinoxalin-2(1H)-ones are important nitrogen-containing fused heterocycles, that form the core structure of numerous biologically active compounds. The biological activity of quinoxalin-2(1H)-one is significantly affected by the substituents present in the core structure of the molecule. The 3-functional quinoxalin-2(1H)-ones have been widely studied because they exhibit excellent biological activities (they possess. anti-angiogenic, anti-tumor, and anti-inflammatory properties, among others) (Willardsen et al., 2004; Khattab et al., 2015).
The Armido Studer' group through a visible-light-initiated to synthesize α-perfluoroalkyl-β-heteroarylation of various alkenes with perfluoroalkyl iodides and quinoxalin-2(1H)-ones (Scheme 1A) (Zheng and Studer, 2019). The Dipankar Koley' group explored a strategy to synthesize α-sulfono-β-heteroaryl scaffolds using alkenes with aryl sulfinic acids and quinoxalin-2(1H)-ones (Scheme 1B) (Sekhar Dutta et al., 2019). Afterwards, Pengfei Zhang' group reported a hypervalent Iodine(III)-promoted rapid cascade reaction of quinoxalinones with unactivated alkenes and TMSN3 (Scheme 1C) (Shen et al., 2019). Recently, Wei Wei' group synthesize 3-trifluoroalkylated quinoxalin-2(1H)-ones via K2S2O8-mediated unactivated alkenes with quinoxalin-2(1H)-ones and CF3SO2Na (Scheme 1D) (Meng et al., 2020). To the best of our knowledge, the CDC reaction has not been utilized yet to synthesize quinoxalin-2(1H)-one derivatives bearing alkene substituents at the C3 position.
Herein, we report the C-H functionalization of quinoxalin-2(1H)-one, in the presence of alkenes, for the direct synthesis of 3-vinylated quinoxalin-2(1H)-ones. In the absence of metal/ligand, the reaction was oxidized with ammonium persulfate [(NH4)2S2O8] to obtain the target products (Scheme 1E).
Results and Discussion
When the reaction was carried out with 1-methylquinoxalin-2(1H)-one 1a and styrene 2a as the-substrates, in the presence of PIFA (oxidant), in DMSO, the desired product was not obtained. Different oxidants, such as PhI(OAc)2, TBHP, TBDP, (NH4)2S2O8 and K2S2O8 were screened for the reaction. (NH4)2S2O8 proved to be the best oxidizing agent, and the final compound was obtained in 45% yield when (NH4)2S2O8 was used for oxidation (Table 1, entries 2–6). The target compound was not obtained when the reaction was carried out in the absence of the oxidant (Table 1, entry 7). The reaction was carried out in different solvents such as toluene, EtOAc, acetone, H2O, DMF, and CH3CN to determine the optimal reaction solvent (Table 1, entries 8–13). The reactions did not proceed smoothly when these solvents were used as the reaction solvents, and the desired products were obtained in significantly low yields. Following this, the effects of different additives, such as CuBr and CuSO4, on the product yields were investigated. It was observed that, in the presence of these additives, the products were produced in significantly low yields (Table 1, entries 14, 15). The reaction condition was also optimized with respect to bases to obtain better yields of the products (Table 1, entries 16–20). The experiments revealed that Cs2CO3 was the most effective in promoting the reactions. Significantly low product yields were obtained when other bases (such as TEA, K2CO3, NaOH, and NaH) were used to drive the reactions. Following this, the effect of temperature on the product yields was also investigated. When the reaction was carried out at higher or lower temperatures, a decrease in the yield of 3a was observed (Table 1, entries 21–23). Thus, the reaction conditions were optimized and the maximum yield of the product was obtained when the reaction was carried out in DMSO (0.1 M) with 1a (0.25 mmol) and 2a (0.75 mmol) as the substrates, in the presence of (NH4)2S2O8 as the oxidant (1 mmol) and Cs2CO3 (0.75 mmol) as the base, under atmospheric conditions at 80°C for 10 h.
After determining the optimal reaction conditions, we focused on expanding the scope of the reaction. We used various substituted aryl olefins as the substrates to carry out the reactions under the optimal reaction conditions (Scheme 2). The results showed that good functional group tolerance could be achieved under the optimized reaction conditions. A series of olefins bearing electron-withdrawing (4-F, 4-Cl, 4-Br, 3-F, 3-Br, and 2-Br) and electron-donating [4-C(CH3)3, 4- Me, and 4-Ph] substituents, with groups attached to the phenyl ring, proved to be good substrates for this reaction. The corresponding 3-vinylated quinoxalin-2(1H)-ones were produced in moderate yields (3b–3p). The product yields decreased when substrates bearing electron donating groups were used for carrying out the reactions. The product yield was dictated by the strength of the electron donating groups. We also observed that the reaction proceeded smoothly when a strong electron-withdrawing group (4-CF3) was present on the phenyl ring. The corresponding product (3l) was obtained in 41% yield. We replaced different heterocyclic rings and investigated the effect of such a change on the yields of the products. The target product 3m was obtained when the ring of choice was naphthalene. Subsequently, we also investigated the influence of quinoxalin-2(1H)-ones, bearing different substituents, on the applicability of the reaction (Scheme 2). The results revealed that the applicability of the method was extensive. The reactions were carried out with derivatives of quinoxalin-2(1H)-one derivatives with different N-substituted groups, such as N-ethyl, N-pentyl, N-vinyl, N-ethynyl, N-esteryl, N-(2-oxo-2-phenylethyl), and N-[2-oxo-2-(4-nitrophenyl)], could generate target compounds 3m–3s in moderate to good yields. The reactions progressed smoothly when the reactions were carried out with the quinoxalin-2(1H)-benzene ring, bearing halogen atoms at different positions, and the desired products in moderate yields (3t–3v). It is worth mentioning that the corresponding target compound 3w was obtained in 51% yield, even when a relatively strong electron-withdrawing group was present on the benzene ring. The target compound 3x was obtained in 50% yield, when the unsubstituted quinoxalin-2(1H)-one was used as the substrate. Regrettably, when replacing styrene with methyl acrylate and allylbenzene, the corresponding product 3y and 3z were not obtained. Surprisingly, by replacing the substrate with quinoxaline, the corresponding product 3A can be obtained in 41% yield.
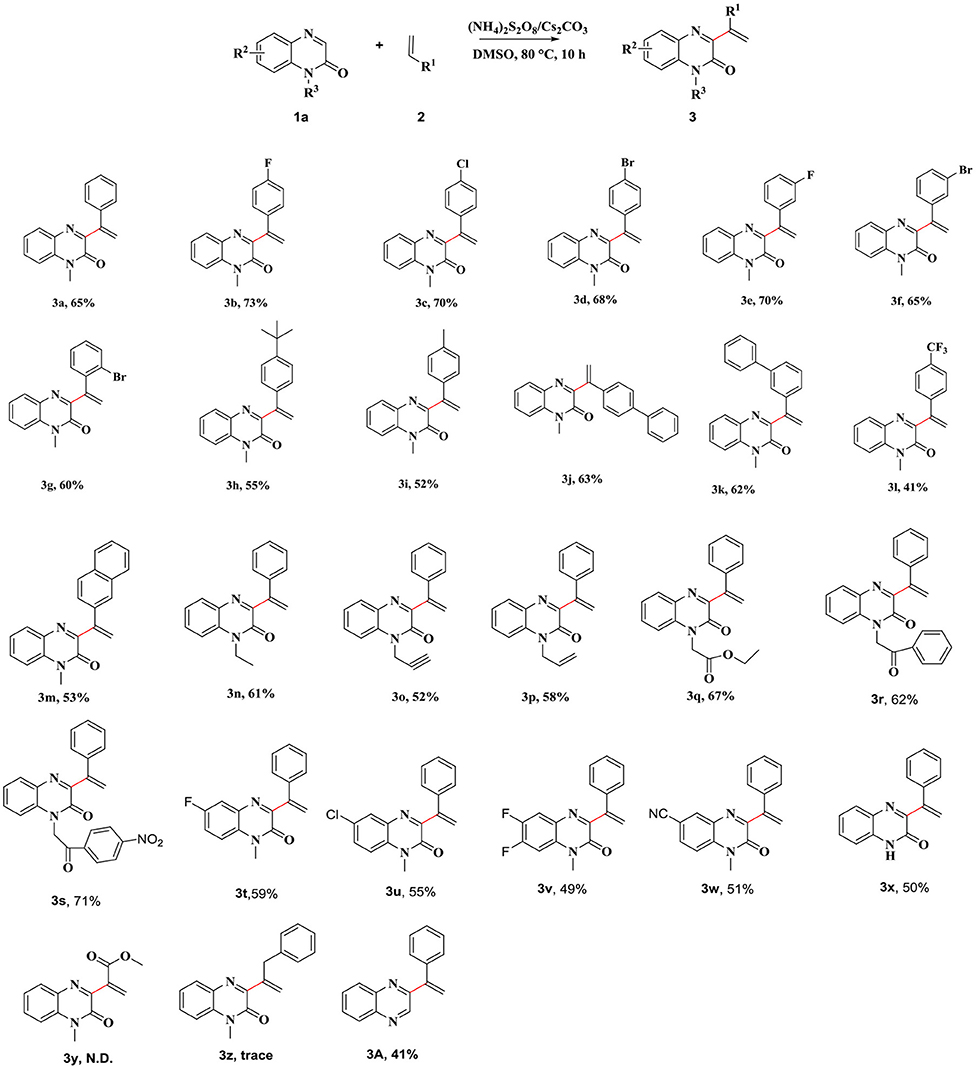
Scheme 2. Coupling Reaction of Quinoxalin-2(1H)-ones and Alkenes. Reaction conditions: 1a (0.25 mmol), 2a (0.75 mmol), Oxidant (1 mmol), base (0.75 mmol), and solvent at 80°C for 10 h under air.
Encouraged by this reaction and sustainable synthesis, we conducted scale-up experiments to investigate the synthetic utility of the reaction. When 7.0 mmol of 1-methylquinoxalin-2(1H)-one 1a was treated with 21.0 mmol styrene (2a), the corresponding product 3a was obtained with a yield of 52%, although an extended reaction time was required (Scheme 3).
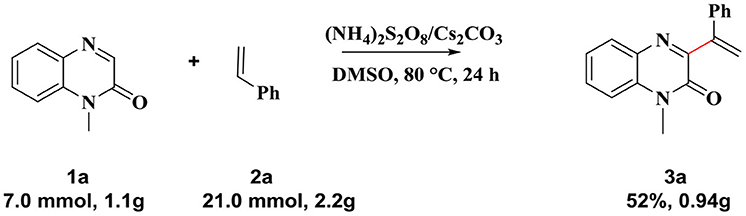
Scheme 3. Large scale experiment: 1a (7.0 mmol), 2a (21.0 mmol), (NH4)2S2O8(19.6 mmol), Cs2CO3 (10.3 mmol) in 20 mL of DMSO, 80°C, 24 h. Product 3a was isolated in 52% yield.
Control reactions were carried out to investigate the reaction mechanism. The introduction of a radical inhibitor (TEMPO or BHT) into the model reaction mixture, significantly inhibited the progress of the reaction. The corresponding 3-vinylated quinoxalin-2(1H)-ones was not obtained (Scheme 4, Equations 1, 2). This, indicated that the reaction proceeded through a radical mechanism. The reaction proceeded smoothly in the presence of deuterated styrene, producing the corresponding target compound (Scheme 4, Equation 3), which indicated that the hydrogen of the terminal double bond in the substrate was not involved in the reaction process. If styrene was replaced with β-methylstyrene, the target product couldn't be obtained under standard conditions, and it was probably due to the spatial site resistance that the reaction did not proceed (Scheme 4, Equation 4). When the amount of TMPEO was reduced, the captured intermediate structure was detected by liquid-phase mass spectrometry, indicating that the reaction mechanism may have gone through this process (Scheme 4, Equation 5).
We proposed the possible reaction mechanism based on these observations and the results presented in literature reports (Bag and Maiti, 2016; Gupta et al., 2017; Fu et al., 2018; Toonchue et al., 2018; Wei et al., 2018; Jin et al., 2019; Sekhar Dutta et al., 2019; Shen et al., 2019; Xie et al., 2019a,b; Zheng and Studer, 2019; Meng et al., 2020; Shi and Wei, 2020; Xie et al., 2020; Ali et al., 2021) (Scheme 5). Initially, alkene 2 reacts with sulfate radical anion (generated in situ) to generate the alkyl radical A. The addition of alkyl radical A to quinoxalin-2(1H)-one 1 produces the nitrogen radical B. The intermediate radical B generates free radical C under heating conditions. The bisulfate anion is released during the process. Single-electron transfer (SET), in the presence of S2, produces the nitrogen cation intermediate D from the radical C. Finally, the intermediate D is deprotonated under base conditions to produce the final product 3.
Conclusion
In summary, we have reported a simple and efficient C3-H vinylation reaction with quinoxalin-2(1H)-one as the substrate, in the presence of alkenes and absence of metals. A series of 3-vinylated quinoxalin-2(1H)-ones with potential biological activities can be obtained when the reactions are carried out in the presence of (NH4)2S2O8. Further research to determine the applicability of the synthetic procedure is presently underway in our laboratory.
Data Availability Statement
The original contributions presented in the study are included in the article/Supplementary Material, further inquiries can be directed to the corresponding author/s.
Author Contributions
YLv and JH were responsible for designing the experiments. RD, YLi, and YC performed the experimentations. JH, YLv, and JY analyzed the results and wrote the publication. All authors contributed to the article and approved the submitted version.
Funding
The authors thank the National Natural Science Foundation of China (Grant No. 81903469) and Weifang Science and Technology Development Plan Project (Grant No. 2019GX029).
Conflict of Interest
The authors declare that the research was conducted in the absence of any commercial or financial relationships that could be construed as a potential conflict of interest.
Supplementary Material
The Supplementary Material for this article can be found online at: https://www.frontiersin.org/articles/10.3389/fchem.2021.672051/full#supplementary-material
References
Ali, W., Prakash, G., and Maiti, D. (2021). Recent development in transition metal-catalysed C–H olefination. Chem. Sci. 2735–2759. doi: 10.1039/D0SC05555G
Bag, S., and Maiti, D. (2016). Palladium-catalyzed olefination of aryl C–H bonds by using directing scaffolds. Synthesis 48, 804–815. doi: 10.1055/s-0035-1561321
Cheng, D., Wu, L., Lv, H., Xu, X., and Yan, J. (2017). CDC reaction and subsequent cyclization for the synthesis of 2-Hydroxy-3-alkyl-1,4-naphthoquinones and pyranonaphthoquinones. J. Org. Chem. 82, 1610–1617. doi: 10.1021/acs.joc.6b02787
Fu, J., Yuan, J., Zhang, Y., Xiao, Y., Mao, P., Diao, X., et al. (2018). Copper-catalyzed oxidative coupling of quinoxalin-2(1H)-ones with alcohols: access to hydroxyalkylation of quinoxalin-2(1H)-ones. Org. Chem. Front. 5, 3382–3390. doi: 10.1039/C8QO00979A
Girard, S. A., Knauber, T., and Li, C. J. (2014). The cross-dehydrogenative coupling of Csp3-H bonds: a versatile strategy for C-C bond formations. Angew Chem. Int. Ed. Engl. 53, 74–100. doi: 10.1002/anie.201304268
Gupta, A., Deshmukh, M. S., and Jain, N. (2017). Iodine-Catalyzed C–N bond formation: synthesis of 3-aminoquinoxalinones under ambient conditions. J. Org. Chem. 82, 4784–4792. doi: 10.1021/acs.joc.7b00464
Huang, C. Y., Kang, H., Li, J., and Li, C. J. (2019). En route to intermolecular cross-dehydrogenative coupling reactions. J. Org. Chem. 84, 12705–12721. doi: 10.1021/acs.joc.9b01704
Jiang, Q., Xu, B., Zhao, A., Jia, J., Liu, T., and Guo, C. (2014). Transition-metal-free oxidative α-C–H amination of ketones via a radical mechanism: mild synthesis of α-amino ketones. J. Org. Chem. 79, 8750–8756. doi: 10.1021/jo5015855
Jin, C., Yan, Z., Sun, B., and Yang, J. (2019). Visible-Light-Induced regioselective alkylation of coumarins via decarboxylative coupling with N-hydroxyphthalimide esters. Org. Lett. 21, 2064–2068. doi: 10.1021/acs.orglett.9b00327
Khattab, S. N., Abdel Moneim, S. A. H., Bekhit, A. A., Massry, A. M. E., Hassan, S. Y., El-Faham, A., et al. (2015). Exploring new selective 3-benzylquinoxaline-based MAO-A inhibitors: design, synthesis, biological evaluation and docking studies. Eur. J. Med. Chem. 93, 308–320. doi: 10.1016/j.ejmech.2015.02.020
Liu, J., Yu, D., Yang, Y., You, H., Sun, M., Wang, Y., et al. (2020). Free-radical-promoted dehydrogenative coupling of polyfluorinated alcohol with quinone, chromone, and coumarin. Org. Lett. 22, 4844–4847. doi: 10.1021/acs.orglett.0c01671
Mane, K. D., Mukherjee, A., Vanka, K., and Suryavanshi, G. (2019). Metal-Free regioselective cross dehydrogenative coupling of cyclic ethers and aryl carbonyls. J. Org. Chem. 84, 2039–2017. doi: 10.1021/acs.joc.8b03048
Meng, N., Wang, L., Liu, Q., Li, Q., Lv, Y., Yue, H., et al. (2020). Metal-Free trifluoroalkylation of quinoxalin-2(1H)-ones with unactivated alkenes and langlois' reagent. J. Org. Chem. 85, 6888–6896. doi: 10.1021/acs.joc.9b03505
Moon, Y., Kwon, D., and Hong, S. (2012). Palladium-Catalyzed dehydrogenation/oxidative cross-coupling sequence of β-heteroatom-substituted ketones. Angew. Chem. Int. Ed. Engl. 51, 11333–11336. doi: 10.1002/anie.201206610
Niu, B., Zhao, W., Ding, Y., Bian, Z., Pittman, C. U., Zhou, A., et al. (2015). Regioselective cross-couplings of coumarins and flavones with ethers via C(sp3)–H functionalization. J. Org. Chem. 80, 7251–7257. doi: 10.1021/acs.joc.5b00800
Parvatkar, P. T., Manetsch, R., and Banik, B. K. (2019). Metal-Free cross-dehydrogenative coupling (CDC): molecular iodine as a versatile catalyst/reagent for CDC reactions. Chem. Asian J. 14, 6–30. doi: 10.1002/asia.201801237
Scheuermann, C. J. (2010). Beyond traditional cross couplings: the scope of the cross dehydrogenative coupling reaction. Chem Asian J. 5, 436–451. doi: 10.1002/asia.200900487
Sekhar Dutta, H., Ahmad, A., Khan, A. A., Kumar, M., Raziullah and Koley, D. (2019). Metal free benzylation and alkylation of quinoxalin-2(1H)-ones with alkenes triggered by sulfonyl radical generated from sulfinic acids. Adv. Synth. Catal. 361, 5534–5539. doi: 10.1002/adsc.201901212
Shen, J., Xu, J., Huang, L., Zhu, Q., and Zhang, P. (2019). Hypervalent Iodine(III)-promoted rapid cascade reaction of quinoxalinones with unactivated alkenes and TMSN3. Adv. Synth. Catal. 362, 230–241. doi: 10.1002/adsc.201901314
Shi, J. W., and Wei, W. (2020). External photocatalyst-free visible-light-induced C3-acylation of quinoxalin-2(1H)-ones. Chin. J. Org. Chem. 40, 2170–2172. doi: 10.6023/cjoc202000041
Toonchue, S., Sumunnee, L., Phomphrai, K., and Yotphan, S. (2018). Metal-free direct oxidative C-C bond coupling of pyrazolones and quinoxalinones. Org. Chem. Front. 5, 1928–1932. doi: 10.1039/C8QO00328A
Wei, W., Wang, L., Bao, P., Shao, Y., Yue, H., Yang, D., et al. (2018). Metal-Free C(sp2)–H/N–H cross-dehydrogenative coupling of quinoxalinones with aliphatic amines under visible-light photoredox catalysis. Org. Lett. 20, 7125–7130. doi: 10.1021/acs.orglett.8b03079
Willardsen, J. A., Dudley, D. A., Cody, W. L., Chi, L., McClanahan, T. B., Mertz, T. E., et al. (2004). Design, synthesis, and biological activity of potent and selective inhibitors of blood coagulation factor Xa. J. Med. Chem. 47, 4089–4099. doi: 10.1021/jm0497491
Xie, L. Y., Jiang, L. L., Tan, J. X., Wang, Y., Xu, X. Q., Zhang, B., et al. (2019a). Visible-Light-Initiated decarboxylative alkylation of quinoxalin-2(1H)-ones with phenyliodine(III) dicarboxylates in recyclable ruthenium(II) catalytic system. ACS Sust. Chem. Eng. 7, 14153–14160. doi: 10.1021/acssuschemeng.9b02822
Xie, L. Y., Liu, Y. S., Ding, H. R., Gong, S. F., Tan, J. X., He, J. Y., et al. (2020). C(sp2)–H/O–H cross-dehydrogenative coupling of quinoxalin-2(1H)-ones with alcohols under visible-light photoredox catalysis. Chin. J. Catal. 41, 1168–1173. doi: 10.1016/S1872-2067(19)63526-6
Xie, L. Y., Peng, S., Fan, T. G., Liu, Y. F., Sun, M., Jiang, L. L., et al. (2019b). Metal-free C3-alkoxycarbonylation of quinoxalin-2(1H)-ones with carbazates as ecofriendly ester sources. Sci. China Chem. 460–464. doi: 10.1007/s11426-018-9446-1
Xu, W. L., Hu, W., Zhao, W. M., Wang, M., Chen, J., and Zhou, L. (2020). Copper(I)/DDQ-mediated double-dehydrogenative diels-alder reaction of aryl butenes with 1,4-diketones and indolones. Org. Lett. 22, 7169–7174. doi: 10.1021/acs.orglett.0c02486
Yuan, J., Fu, J., Yin, J., Dong, Z., Xiao, Y., Mao, P., et al. (2018). Transition-metal-free direct C-3 alkylation of quinoxalin-2(1H)-ones with ethers. Org. Chem. Front. 5, 2820–2828. doi: 10.1039/C8QO00731D
Keywords: vinylation, cross-dehydrocoupling, ammonium persulfate, alkenes, C–H functionalization
Citation: Ding R, Li Y, Chang Y, Liu Y, Yu J, Lv Y and Hu J (2021) Metal-Free Direct C–H Functionalization of Quinoxalin-2(1H)-Ones to Produce 3-Vinylated Quinoxalin-2(1H)-Ones in the Presence of Alkenes. Front. Chem. 9:672051. doi: 10.3389/fchem.2021.672051
Received: 25 February 2021; Accepted: 06 April 2021;
Published: 30 April 2021.
Edited by:
Guigen Li, Texas Tech University, United StatesReviewed by:
Da-Gang Yu, Sichuan University, ChinaDaoshan Yang, Qingdao University of Science and Technology, China
Copyright © 2021 Ding, Li, Chang, Liu, Yu, Lv and Hu. This is an open-access article distributed under the terms of the Creative Commons Attribution License (CC BY). The use, distribution or reproduction in other forums is permitted, provided the original author(s) and the copyright owner(s) are credited and that the original publication in this journal is cited, in accordance with accepted academic practice. No use, distribution or reproduction is permitted which does not comply with these terms.
*Correspondence: Yanna Lv, bHlubHluYSYjeDAwMDQwOzE2My5jb20=; Jinxing Hu, amlueGluZ2h1MjAxMyYjeDAwMDQwO3dmbWMuZWR1LmNu