- Translational Nanobiomaterials and Imaging Group, Department of Radiology, Leiden University Medical Center, Leiden, Netherlands
Fluorescence imaging in the second near infrared window (NIR-II, 1,000–1,700 nm) has been widely used in cancer diagnosis and treatment due to its high spatial resolution and deep tissue penetration depths. In this work, recent advances in rare-earth-doped nanoparticles (RENPs)—a novel kind of NIR-II nanoprobes—are presented. The main focus of this study is on the modification of RENPs and their applications in NIR-II in vitro and in vivo imaging and cancer theranostics. Finally, the perspectives and challenges of NIR-II RENPs are discussed.
Introduction
Cancer is one of the world's most lethal diseases, and there are no particularly effective treatments to date. Cancer patients must endure chemotherapy and radiotherapy, followed by long-term medications, which are a great burden on their body and mind. For people not to be afflicted by cancer, it is necessary to diagnose the disease in an early stage and personalize treatments based on each patient's individual variability and medical profile (Rubin et al., 2014). Molecular imaging modalities can be useful for the comprehensive evaluation of essential biomolecules and can facilitate the non-invasive visualization of cell function and biochemical processes in biological systems (Kuimova et al., 2009; Weissleder et al., 2016; Yang et al., 2017a). They are well-recognized as powerful techniques that provide more comprehensive anatomical, physiological and functional information in early cancer detection, drug delivery, as well as monitoring treatment effectiveness (Quon and Gambhir, 2005; Weissleder and Pittet, 2008; Willmann et al., 2008). Currently, varieties of molecular imaging techniques are widely used in the medical field, including magnetic resonance imaging (MRI), X-ray computed tomography (CT), positron emission tomography (PET), single-photon emission tomography (SPECT), and optical fluorescent light imaging (FLI) (Figure 1). However, these methods have some disadvantages. For example, CT and MRI often require high doses of contrast agents; PET and SPECT require radioactive tracers that can put both patients and operators in danger (O'Leary et al., 1999; Mariani et al., 2001, 2002; Tsien, 2003). Also, they need to be optimized to obtain more accurate information due to their long scanning time and low sensitivity/spatial resolution (Toussaint et al., 1996; Paulus et al., 2000).
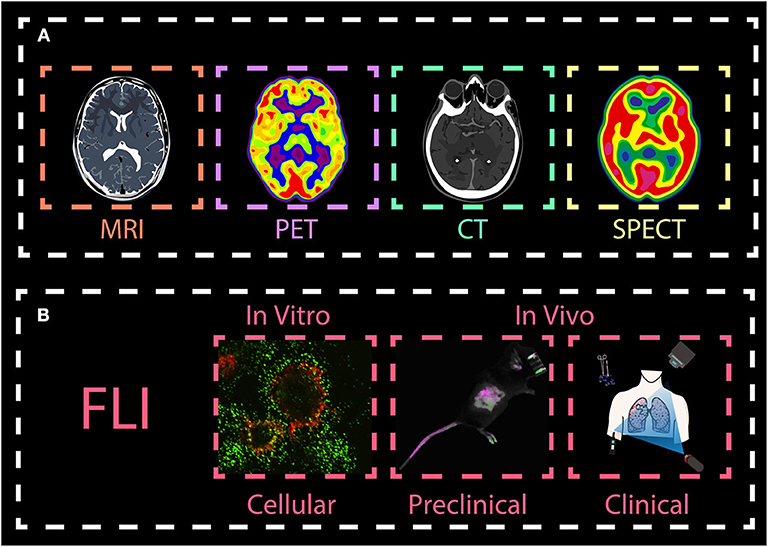
Figure 1. Overview molecular imaging techniques. The main imaging methods are (A) magnetic resonance imaging (MRI), X-ray computed tomography (CT), positron emission tomography (PET), single-photon emission tomography (SPECT), and (B) optical fluorescent light imaging (FLI). While MRI, PET, CT, and SPECT are widely used in the clinics, FLI techniques are mainly used in biomedical preclinical research in vitro and in vivo, with the exception of fluorescence image-guided surgery, a medical imaging technique used to detect fluorescently labeled structures during surgery. This review, we will focus on introducing FLI from both in vitro and in vivo imaging.
In recent years, optical imaging has attracted much attention in various fields, predominantly preclinical research because it provides excellent real-time visualization, high sensitivity and spatial resolution, especially in early detection and diagnosis of cancer. Generally, most of the conventional imaging agents operate in the short-wavelength region (e.g., the ultraviolet (UV) and visible regions). In these regions, light signals are easily absorbed and scattered by certain biological tissues (such as muscle, skin and body fluids). This leads to high autofluorescence, low signal-to-background ratio and low tissue penetration (Yang et al., 2017b,c). Besides, high-energy light can lead to photo-toxicity damage in biological tissues. To circumvent these problems, optical imaging in the near-infrared (NIR) region, which is located in the so-called “biological window,” has gained much attention (Figure 2). Imaging agents in the first near-infrared window (NIR-I, 700–900 nm) are gradually being known by researchers, and can provide deep and sensitive bioimaging. However, their limited tissue penetration depth (less to 1 cm) and large photon scattering losses in biological samples still restrict their use further in biomedical diagnosis and therapy. To address these challenges, novel materials that enable fluorescent imaging in the NIR-II window (10,00–1,700 nm) for biomedical applications have been developed. They show better resolution because they have deeper penetration (~1.8 cm) and lower autofluorescence. Therefore, there is need to synthesize the novel NIR-II agents with high efficiency and resolution for biological imaging application (Fan and Zhang, 2019).
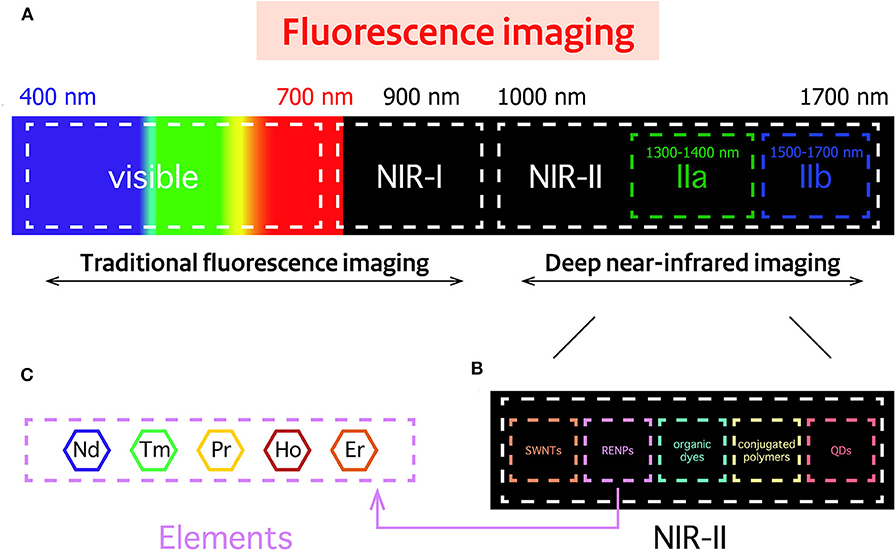
Figure 2. Spectral overview fluorescence imaging techniques. (A) Spectral ranges of traditional fluorescence imaging techniques, including the near-infrared (NIR)-I region, and the deep NIR-II region. (B) Imaging probes commonly used in the NIR-II region: Single walled nanotubes (SWNTs), rare-earth-doped nanoparticles (RENPs), organic dyes, conjugated polymers, and quantum dots (QDs). (C) Neodymium (Nd)-, thulium (Tm)-, praseodymium (Pr)-, holmium (Ho)-, and erbium (Er)-containing RENPs have attracted wide attention.
So far, many types of fluorescent agents with emission in the NIR-II region have been extensively applied for effective bio-sensing and real-time in vitro and in vivo imaging of living species (Figure 2). They include single-walled carbon nanotubes (SWNTs) (Gong et al., 2013; Liang et al., 2014), organic dyes (Lei et al., 2019; Wang et al., 2019a), conjugated polymers (Hong et al., 2014), quantum dots (QDs) (Li C. et al., 2014), and rare-earth-doped nanoparticles (RENPs) (Fan et al., 2019; Wang et al., 2019b). However, most of them have some disadvantages, such as the broad emission bandwidths of SWNTs, short accumulation time of organic dyes, high toxicity, low quantum yield and low solubility of QDs. These disadvantages will vastly inhibit their further applications in NIR-II imaging. RENPs are good candidates for NIR-II optical imaging, because they show minimal photo-bleaching, superior luminescent lifetimes, excellent tunable emission wavelengths and low biotoxicity (Rocha et al., 2014; Wang et al., 2014; Dong et al., 2015; Hemmer et al., 2016; Jiang et al., 2016; Kamimura et al., 2017).
Rare earth elements constitute a class of lanthanide ions found in the 6th row of the periodic table (La, Ce, Pr, Nd, Pm, Sm, Eu, Gd, Tb, Dy, Ho, Er, Tm, Yb, Lu), as well as two other elements closely related to the lanthanides—yttrium (Y) and scandium (Sc). Due to the incompletely filled 4f shell and the spin-orbital coupling of 4f free ions, they possess extremely complex optical properties. One of the most interesting features of these ions is their photoluminescence. The luminescence of the trivalent lanthanide ions arises from f-f transitions of the 4f shell and f-d transitions in the 4f-5d shell. The f-f transitions also provide the lanthanide elements with rich energy level structures in the UV, VIS and NIR ranges. As they can be tuned from the UV to the NIR region, most nanomaterials made of rare-earth elements can be classified into two major categories: Upconversion nanoparticles (UCNPs) and downconversion nanoparticles (DCNPs). DCNPs can downconvert a high energy photon into two or more low-energy photons. In contrast, UCNPs can convert long-wavelength light (low energy) to short wavelength (high energy). Unfortunately, due to the unique anti-Stokes optical properties of UCNPs, most of the NIR-II nanoprobes belong to the category of DCNPs. Until now, a large number of studies have shown that by using suitable sensitizers, UCNPs can obtain longer excitation wavelength for NIR-II imaging (Zhang et al., 2020). For example, emissions in the NIR-II region of Ho3+ and Nd3+ could be obtained from Er3+ sensitized UCNPs (Liu et al., 2018).
Based on the excellent characteristics of rare-earth ions, such as their low photobleaching, various absorption and emission wavelengths, and low energy losses, NIR light-mediated RENPs have been widely used in in vitro and in vivo imaging of biomolecules. Commonly, this kind of downconverting nanoparticle combines rare-earth ions and an inorganic crystalline host lattice (e.g., NaYF4, NaLuF4, and CaF2). The host can also provide an environment for energy transfer from a sensitizer to a rare-earth dopant resulting in NIR-II fluorescence.
This review mainly introduces the recent advances in RENPs fluorescent imaging in the NIR-II region. In particular, we focus on the modification of these nanoparticles by lipids or dyes, and their use in cancer diagnosis and therapy. Then, the challenges and prospects of RENPs are discussed.
Main Kinds of RENPs
Based on the energy level of the rare earth elements, most RENPs possess upconversion and downconversion properties. Up to date, thanks to the effort of many researchers, five of them are reported and extensively explored as activators emitting in NIR-II regions, having excellent downconversion emission (1,060/1,300 nm for Nd3+, 1,470 nm for Tm3+, 1,310 nm for Pr3+, 1,185 nm for Ho3+, and 1,525 nm for Er3+) (Liu et al., 2016) (Figure 2).
Nd-Doped Nanoparticles
According to recent studies, Nd3+ has gained attention for bioimaging applications due to its special illumination at 808 nm and deep tissue penetration (Wang et al., 2013). With strong absorption at 730 nm, 808 nm or 860 nm, Nd3+ can transfer photons with the generation of electrons from the 4I9/2 ground state to the 4F7/2, 4F5/2, or 4F3/2; then the electrons move back to the 4F3/2 state, which can reduce the overheating effect of tissues usually caused by 980 nm light. As a result of the two transitions, the emission corresponds to 1,060 nm (4F3/2→4I11/2) and 1,330 nm (4F3/2→4I13/2) in the NIR-II region. Thus, it provides a good way to avoid autofluorescence of tissue.
Earlier attempts of using Nd-doped nanomaterials as NIR-II biomedical imaging agents have been described (Villa et al., 2015; Yu et al., 2018). In 2002, Stouwdam et al. first realized that Nd3+ doped LaF3 nanoparticles can be utilized as a polymer-based optical component under 514 nm laser excitation (Stouwdam and van Veggel, 2002). Then, Wang et al. developed the synthesized method of LaF3: Nd3+. It was carried out in aqueous solution at low temperature, and showed great NIR-II emission under 802 nm laser excitation (Wang et al., 2006). In 2014, LaF3: Nd3+ nanoparticles were used to obtain both in vitro and in vivo images in cancer cells and mice by Rocha et al. The results showed that LaF3: Nd3+ nanoparticles are a very promising fluorescent nanoprobe for bioimaging in the second NIR window (Rocha et al., 2014). One year later, Villa and his group did an exciting work on high-contrast in vivo imaging in the second biological window (Villa et al., 2015). This work showed how to produce autofluorescence free, high contrast in vivo fluorescence imaging with 1340 nm emission band of SrF2: Nd3+ nanoparticles. They found that autofluorescence of animal diet can extend up to about 1,100 nm, which demonstrated that food-related infrared autofluorescence has an impact on the study of reliable biodistribution. In the past 3 years, some new host matrices have been reported, such as LiYF4 (Jiang et al., 2016), GdPO4 (Yang et al., 2018), CaTiO3 (Li et al., 2015), and NaDyF4 (Liu et al., 2017). As we know, higher Nd3+ doping will result in severe quenching of concentration, so to induce great fluorescence signals, the concentration of Nd3+ should be controlled between 1 and 5%. Thanks to intensive research, most of these new Nd3+ doped systems are nowadays not only used in vivo NIR-II imaging but also in X-ray CT bioimaging or MRI. Owing to the large X-ray absorption coefficient of Gd3+, Dy3+, dual-mode molecular imaging has become a new trend in bioimaging, such as NIR-II imaging/CT, NIR-II imaging/MRI, NIR-II imaging/PET.
Despite the efforts made so far as seen above, low optical effects are still a major drawback. However, sensitizers and core-shell structures that can be used to increase the signal-to-noise ratio are gradually becoming more known in the field of NIR-II bioimaging, disease detection and treatment. For example, NaGdF4: Nd3+, Yb3+, Tm3+ is a novel nanomaterial which uses Gd3+ as bridge ions and finally traps energy by the initial activator ions (Nd3+) (Zhang et al., 2015). Other previous studies also showed that co-doping with Y3+ effectively reduced the aggregation of Nd3+ in CaF2, resulting in a greater luminescence enhancement of Nd3+ (Yu et al., 2018). Chen et al. synthesized high quantum yield core/shell NaGdF4: 3%Nd3+@NaGdF4 nanoparticles with an average size of 15 nm. An in vitro and in vivo NIR-II bioimaging was obtained by loading HeLa cells with NaGdF4: 3%Nd3+@NaGdF4 nanoparticles and transferring NaGdF4: 3%Nd3+@NaGdF4 nanoparticles in a nude mouse model (Chen et al., 2012). CaF2 was also used as the shell material to make NaYF4: Yb, Nd@CaF2 core/shell nanoparticles, which resulted in high contrast multiplexed in vivo imaging in the NIR-II region (Ortgies et al., 2018). In 2018, inspired by Chen's work, Wang et al. fabricated NaGdF4: 5%Nd3+@NaGdF4 by the successive layer-by-layer (SILAR) method. To obtain DCNPs-L1-FSHβ nanoprobes via an EDC/NHS reaction, image-guided surgery for metastatic ovarian cancer could be improved. Utilizing these novel nanoprobes, metastases with ≤ 1 mm can be completely resected under the guidance of NIR-II imaging (Wang P. et al., 2018). A recent report showed that the ultra-small NaGdF4: 5 %Nd@NaGdF4 (4.38 ± 0.57 nm) nanoparticles can be applied in the precise inflammation bioimaging by ROS (reactive oxygen species)-responsive cross-linking after modification with GSH (Glu-Cys-Gly) (Wang et al., 2014; Zhao et al., 2019). An interesting work based on supramolecular self-assembly strategy is developed for NIR-II imaging assembly and disassembly through NaGdF4: 10%Y, 25%Yb, 0.5%Tm@NaGdF4 UCNP@azobenzene and NaGdF4: 5%Nd@NaGdF4 DCNP@β-cyclodextrin. The new strategy allows flexible assembly and disassembly of nanoparticles by controlling different NIR-lasers, which can reduce the background of biological imaging and long-term cytotoxicity, while providing technical support for further accurate image-guided tumor surgery (Zhao M. et al., 2018). As only a few NIR-II fluorophores can be used directly for bone imaging without linking to targeted ligands, He et al. demonstrated DSPE-mPEG encapsulated with β-phase NaYF4: 7%Nd@NaYF4 can be used for bone and vascular imaging, even real-time image-guided lymph node mapping and resection (He et al., 2019) (Table 1).
Er-Doped Nanoparticles
With the rapid development of the RENPs, Er3+ doped nanoprobes are mainly synthesized as upconversion nanomaterials and applied in the VIS and NIR-I regions. In 2011, Y2O3: Yb, Er nanoparticles modified by PEG-b-PVBP and PEG-PO3H2 showed NIR emission at 1,550 nm in organs of live mice (Kamimura et al., 2011). Then people considered Er3+ as a better dopant since it can exhibit strong downconversion luminescence in NIR-IIb region. Nanoprobes employed in the NIR-IIb region are better for bioimaging, owing to their deeper tissue penetration, higher spatial and temporal resolution and lower autofluorescence than those in the NIR-IIa region; but rare-earth based nanoprobes with high spatial and temporal resolution imaged in NIR-IIb region are still very scarce. There is no doubt that the special characteristic of Er3+ solves the main problem that has plagued researchers for a long time. Two years later, Naczynski et al. first used NaYF4: Yb, Ln (Ln: Er, Ho, Tm or Pr) for in vivo imaging of tumors. They demonstrated that Er3+ doped nanoprobes were the brightest one. Especially, by encapsulating RENPs with albumin, they provided a good method to improve tumor accumulation (Naczynski et al., 2013). Then, Er3+ codoped Yb3+ nanoprobes have attracted increasing attention due to their special application potential. Polyacrylic acid (PAA) modified NaYF4: Gd/Yb/Er nanoprobes have been synthesized and have opened the opportunities for NIR-IIb in vivo imaging, non-invasive brain vessel imaging and tiny tumor detection guided by optical imaging (Xue et al., 2018). In 2016, Dang et al. used the well-established technology, Layer-by-Layer (LbL) to design a NIR-II based theranostic platform by NaYF4: Yb, Er-PLA/DXS/PLA/HA nanoprobes, which can accumulate in diseased sites and demonstrate diagnostic capabilities within an ovarian tumor mouse model. This study demonstrated that these nanoprobes can serve as a promising theranostic platform to monitor the progression and treatment of serous ovarian cancer (Dang et al., 2016). Indeed, core-shell is well known for its unique ability to enhance the Er3+ emission at NIR-II region. This special structure does not only delay the degradation of dopant but also decreases the quenching effects and strengthens fluorescence. Simple NaYF4: Yb/Er@NaYF4 nanoprobes have been prepared to realize real-time surveillance of metastatic lesions (Kantamneni et al., 2017). Deng et al. proposed Sc-based probes (KSc2F7: Yb3+/Er3+), which are significantly different from the traditional NaYF4 host. After modification with PAA, they showed a ~1.70-fold stronger fluorescence than the PAA-NaYF4 nanocrystals under 980 nm excitation. On this basis, they performed the first case of through-skull fluorescence imaging of brain vessels with KSc2F7: Yb3+/Er3+ probes (Deng et al., 2018). Normally, Yb3+ can transfer energy to Er 4I11/2 level to release non-radiative photons to the 4I13/2 level, and then radiate to the 4I15/2 level to produce the 1,550 nm downconversion emission. During this process, upconversion and quenching effects will decrease the intensity of Er3+ downconversion emission. As an alternative, Ce3+ is developed as a doping element in Er-doped nanoparticles to improve the NIR-II downconversion emission by efficiently accelerating non-radiative relaxation of Er 4I11/2→4I13/2. NaYbF4: 2%Er, 2%Ce@NaYF4 nanoparticles have been made to prove Ce3+ can highly suppress the upconversion with the downconversion pathway boosted by about 9-fold. This can lead to fast NIR-II cerebral-vasculatures imaging by modified PMF-PEG (Zhong et al., 2017). The synthesis of NaCeF4: Er/Yb@NaCeF4 has further verified the efficient energy transfer of Yb3+-Er3+-Ce3+. Surface modification with DSPE-PEG2000-COOH proved to be a useful method to detect uric acid and can be a key approach in a physiological survey and clinical diagnosis (Lei et al., 2018). Interesting research has been done to design and implant QR codes into a mouse by incorporating NaYF4: Tm3+/Er3+@NaYF4 into polydimethylsiloxane (PDMS) matrices. The QR code consists of black squares arranged in a square grid on a white background according to certain rules, and the imaging device can read the data from the horizontal and vertical components of the image. It provides a possibility for NIR-II in vivo information storage and decoding (Zhang et al., 2019). A core/multishell structure (NaGdF4@NaGdF4: Yb/Er@NaYF4: Yb@NaNdF4: Yb) has also been used for breast cancer diagnostics in vivo (Fan et al., 2018). Recent progress has focused on the diversification of Er-doped rare-earth nanoparticles. NaYF4: Er nanoparticles conjugated with the indocyanine green dye (ICG) have been applied to bioimaging in the NIR-II window because of their high spatial resolution. Due to high absorption cross-section of ICG, excitation efficiency of Er3+ is increased by the energy transfer mechanism and has proved the potential of ICG-NaYF4: Er nanoconjugates for multimodal theranostics (Wang D. et al., 2018) (Figure 3). Since NIR-II imaging-guided photothermal therapy (PTT) is rarely explored, Liu et al. have successfully developed a core-shell structured NaLuF4: Gd/Yb/Er NRs@PDA as a nanoplatform that can simultaneously be used to diagnose and treat tumors. It can not only be used to realize NIR-II imaging but also to enable image-guided PTT (Li X. et al., 2019).
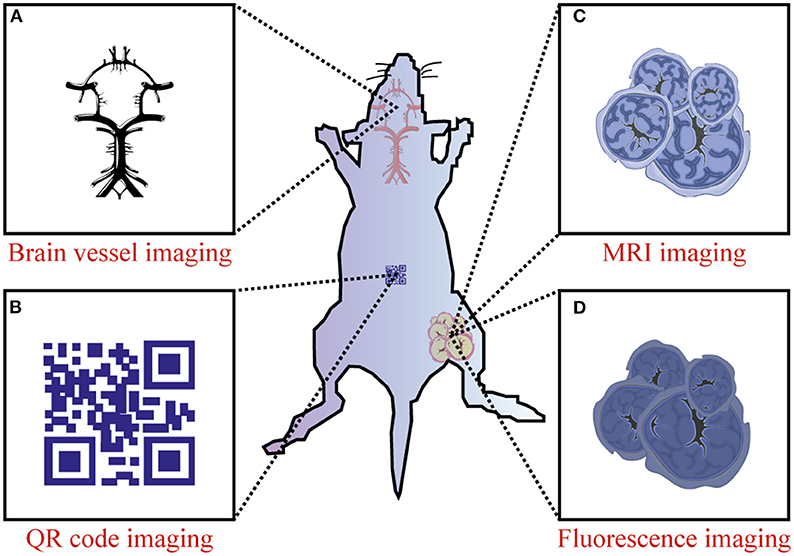
Figure 3. Preclinical application of Er-RENPs in the NIR-II region. (A) NIR-II brain vessel imaging, (B) NIR-II QR code imaging and (C,D) dual mode MRI imaging/ NIR-II fluorescence imaging.
Synthesis and Modification
At present, RENPs are synthesized by a solvothermal method, which is technically mature. Solvothermal synthesis is a solution chemistry method that crystallizes nanomaterials of different sizes and morphologies directly from solution under a certain temperature and pressure. To synthesize RENPs with uniform size and morphology, good dispensability and high luminous efficiency, the size and morphology have to be controlled by adjusting the ratio of raw materials, temperature and solvents (Mai et al., 2007; Zhang et al., 2007; Tan et al., 2009, 2013; Wang et al., 2010; Yuan et al., 2013). On the other hand, because this process cannot precisely control the distribution of dopants, the local relative enrichment of the dopants usually occurs, resulting in the reduction of luminescent efficiency. In order to avoid the deficiency, one pot successive layer-by-layer (SLBL) strategy is used to synthesize homogeneous doping core (HOC) nanoparticles by growing uniform shells (Li X. et al., 2014, 2019). However, RENPs prepared with oleic acid as reagent are hardly soluble in water and difficult to attach to biomolecules. This limits their application in cell labeling and fluorescent imaging. It is therefore necessary to convert a hydrophobic group into a hydrophilic group by surface modification (for example, -COOH, -NH2, or -SH). Alternatively, Dong et al. have reported the oleate ligands attached to the UCNPs surface can be replaced by nitrosonium tetrafluoroborate (NOBF4) (Dong et al., 2011). Currently, the main surface modification methods used are ligand oxidation, ligand exchange and layer-by-layer self-assembly (Wang and Liu, 2009; Li and Lin, 2010). The nanoparticle size does not increase after the water-soluble modification using the ligand exchange method, and it is not easy to control the exchange efficiency and effect of water solubility. After using ligand oxidation for modification, due to the shortening of the ligand carbon chain, polar solvent water cannot be effectively suppressed not to quench the fluorescence, and also the fluorescence intensity is much weaker. This method is only suitable for the oxidation of ligands containing carbon-carbon double bonds (C=C). Therefore, it is still a hot Research Topic to select effective water-soluble modification methods to obtain RENPs with small particle size, good water solubility and high fluorescence intensity. The following is a brief summary to the currently used surface modification methods (Figure 4).
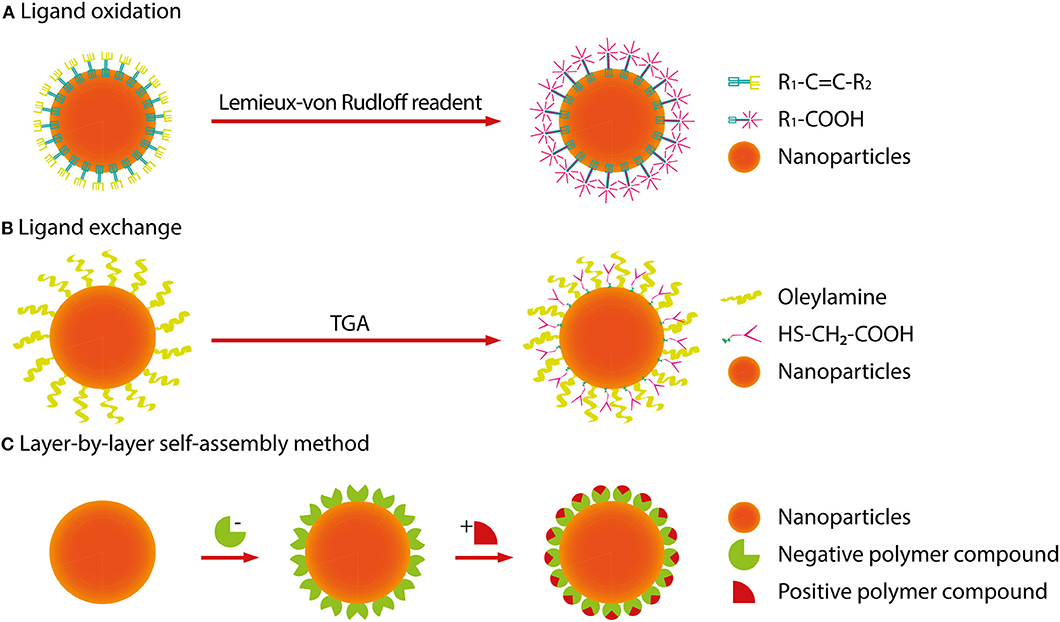
Figure 4. Surface modification methods of RENPs. (A) The ligand oxidation method requires a strong oxidizing agent (Lemieux-von Rudloff reagent, etc.) to oxidize the carbon-carbon double bond to a carboxyl group. (B) The ligand exchange method is used to replace an organic ligand having weak coordination and hydrophobicity with a strong hydrophilic organic ligand. (C) The layer-by-layer self-assembly method relies mainly on the attractive force between oppositely charged molecules, alternately deposits dense monolayers of charged molecules onto oppositely charged surfaces.
Ligand Oxidation
The ligand oxidation method requires a strong oxidizing agent (Lemieux-von Rudloff reagent, etc.) to oxidize the carbon-carbon double bond to a carboxyl group, which is on the surface-coated oleic acid ligand. This reaction can obtain hydrophilic, carboxylic acid-functional RENPs. Thus, the RENPs not only have good water solubility, but also can be directly coupled with diverse biomolecules.
Ligand Exchange
The ligand exchange method is used to replace an organic ligand having weak coordination and hydrophobicity with a strong hydrophilic organic ligand on the surface of the material. This process makes the RENPs hydrophilic and water-soluble.
Layer-by-Layer Self-Assembly Method
The principle of the layer-by-layer self-assembly method (Wang et al., 2002) is to first wrap a layer of polymer compound with a certain charge on the surface of hydrophobic RENPs. When it is added to a solution of an opposite charged polymer compound, another opposite charged polymer compound can be attracted to the first layer. In this way, the layers are adsorbed, and the upper fluorescent group can be converted, which is possible by alternating self-assembled layers into a polymer layer on the surface of the RENPs. The thickness of the polymer layer can be regulated by changing the number of self-assembled layers so that the RENPs can be stably dispersed in water and have good biocompatibility.
Cancer Theranostics With NIR-II RENPs
Currently, clinically approved indocyanine green (ICG) and methylene blue (MB) have been used as contrast agents to depict tumor margins in preclinical cancer models and human patients (Winer et al., 2010; Vahrmeijer et al., 2013; Wang et al., 2015). However, these rapidly excreted probes possess short tumor retention times and affect the process of cancer treatment. Due to the renal filtration threshold of ~40 kD, most fluorescent probes accumulate largely in the organs of reticuloendothelial system, such as the liver and spleen, leading to long-term safety concerns. Therefore, NIR-II RENPs probes with long tumor retention times, high signal-to-background ratio and deep tissue penetration have aroused great interest in investigating their applications for cancer theranostics. To reduce the retention time of nanoparticles in the reticuloendothelial system, excretable NIR-II nanoparticles, RENPs@Lips, have been developed for medical imaging and surgical navigation. Under the guidance of NIR-II imaging, RENPs@Lips showed excellent performance in intraoperative identification of orthotopic tumor vessels and embolization surgery, and could be used in sentinel lymph node biopsies in tumor-bearing mice (Li D. et al., 2019). CXCR-4-targeted functional nanoprobes (fReANC) have been demonstrated to detect up to 10.5 mm of deep-seeded subtissue microlesions in lung metastatic models of breast cancer, providing a reliable platform for the detection of targeted subtissue cancerous lesions (Zevon et al., 2015). At the same time, Dang et al. compared several available LbL NIR-II probes, found that rare-earth-based down-conversion nanoparticles can define vascular and skeletal structures, and were evaluated as diagnostic probes for high-grade serous ovarian cancer with the highest resolution out of all tested probes (Dang et al., 2016). NIR-II RENPs, with the least interference from scattering and autofluorescence, seemed to represent a promising tool for photothermal therapy (PTT) and photodynamic therapy (PDT). He et al. have designed a unique NaGdF4: Nd@NaGdF4@NaGdF4: Yb, Er@NaGdF4: Yb@NaNdF4: Yb (LDNPs-5) structure by attaching Au25 clusters and poly(ethylene glycol) (PEG) molecules on nanostructure. Under 808 nm light irradiation, the special LDNPs can efficiently kill tumor cells in vitro and in vivo due to a synergistic effect arising from the combination of PTT effect generated from Nd3+ with PDT (He et al., 2016). In addition, a three-layer core-shell-shell nanocomposite (NaYF4: Nd3+@NaLuF4@PDA18) showed an excellent PTT effect in ablation tumors (Dai et al., 2017). Recently, dual-mode SWIR imaging and MRI guided PTT was performed in a nude mouse model by using NaErF4@NaGdF4 (Er@Gd), which can effectively be used to ablate tumors and provide a new way for cancer theranostics (Ma et al., 2018). NaErF4@NaYF4@NaNdF4@Prussian blue (PB) encapsulated in a phospholipid PEG micelle (PEG-CSS@PB) served as an efficient theranostic agent for NIR-II-image guided PTT. In this study, tumors treated with PTT shrank ~12-fold compared with untreated tumors (Wang et al., 2019c). To achieve accurate tumor localization and a high cancer therapeutic efficacy, Liu et al. developed an ultrasmall pH-responsive photothermal gallic acid-iron complex-modified NaDyF4: Nd nanoprobe to enhance cancer theranostic by in situ aggregation (Liu et al., 2017). In addition, a theranostic nanoparticle based on RENPs has been developed for gene therapy. Polyethylenimine (PEI) coated β-NaY0.78F4:Yb0.20,Er0.02@NaYF4 was designed to deliver genetic cargo in an in vitro cancer model and detected tumor lesions in a lung metastases model of breast cancer. This strategy will make it possible to develop a nanotheranostic platform based on NIR-II RENPs for gene therapy (Zhao Z. et al., 2018). In summary, NIR-II RENPs have great potential in cancer theranostics.
Discussion
Overall, RENPs are promising candidates for NIR-II biomedical imaging due to their low toxicity, high photostability, deep tissue penetration, and tunable pharmacokinetic behavior. Despite these successful gains, challenges still remain in the bioimaging applications of NIR-II RENPs. One of them is the limitation of the emission center, which is the fluorescence core of the RENPs. As we know, five rare earth elements (Nd3+, Tm3+, Pr3+, Ho3+, Er3+) can be the emission centers in NIR-II RENPs, which are excited by 808 nm or 980 nm lasers. However, the RENPs that have been developed are still mainly based on Nd3+ and Er3+ as the emission centers, which greatly limits the development and application of near-infrared probe types. Although other rare earth elements have also been presented, such efforts should be devoted to design novel NIR-II RENPs probes. For example, Liu et al. used Er3+ as a sensitizer and Ho3+ as an emitter to make a core-shell structured NaErF4: Ho@NaYF4 nanoparticle, which emitted at 1,180 nm (Liu et al., 2018). Besides that, the size of the RENPs has always been a concern in bioimaging. Smaller size nanoparticles can effectively enter biological tissues, even cells, but the luminescence intensity of nanomaterials will decrease. Although the commonly used core-shell structure can enhance the luminescence intensity, it will increase the size, making it difficult for the nanoparticles to gain entry into biological tissues and the digestion time will become longer. Designing suitable size nanoparticles is still an essential task to promote the NIR-II bioimaging applications of RENPs. All of these studies in the past decades have pointed out that RENPs will play an important role in drug delivery tracking and multispectral molecular imaging in the near future.
Author Contributions
ZY, CE, and LC structured the review. ZY wrote the review. CE and LC revised the text. All authors contributed to the article and approved the submitted version.
Funding
ZY was supported by the CSC scholarship. CE was supported by the research program VENI with project number 916.181.54, which is (partly) financed by the Dutch Research Council (NWO). LC was supported by project grants from the European Commission H2020-MSCA-RISE (644373—PRISAR), H2020-MSCA-RISE (777682—CANCER), H2020-WIDESPREAD-05-2017-Twinning (807281—ACORN), H2020-WIDESPREAD-2018-03 (852985—SIMICA), H2020-SCA-RISE-2016 (734684—CHARMED), and MSCA-ITN-2015-ETN (675743-ISPIC), 861190 (PAVE), 857894 (CAST), 859908 (NOVA-MRI); 860173 (RISE-WELL); 872860 (PRISAR2) and research program VIDI (project number 723.012.110) of Dutch Research Council (NWO).
Conflict of Interest
The authors declare that the research was conducted in the absence of any commercial or financial relationships that could be construed as a potential conflict of interest.
Acknowledgments
We would like to thank E. L. Kaijzel for proof-reading of the manuscript.
References
Chen, G., Ohulchanskyy, T. Y., Liu, S., Law, W. C., Wu, F., Swihart, M. T., et al. (2012). Core/shell NaGdF4: Nd3+/NaGdF4 nanocrystals with efficient near-infrared to near-infrared downconversion photoluminescence for bioimaging applications. ACS Nano. 6, 2969–2977. doi: 10.1021/nn2042362
Dai, Y., Yang, D., Yu, D., Cao, C., Wang, Q., Xie, S., et al. (2017). Mussel-inspired polydopamine-coated lanthanide nanoparticles for NIR-II/CT dual imaging and photothermal therapy. ACS Appl. Mater. Interf. 9, 26674–26683. doi: 10.1021/acsami.7b06109
Dang, X., Gu, L., Qi, J., Correa, S., Zhang, G., Belcher, A. M., et al. (2016). Layer-by-layer assembled fluorescent probes in the second near-infrared window for systemic delivery and detection of ovarian cancer. Proc. Natl. Acad. Sci U.S.A. 113, 5179–5184. doi: 10.1073/pnas.1521175113
Deng, Z., Li, X., Xue, Z., Jiang, M., Li, Y., Zeng, S., et al. (2018). A high performance sc-based nanoprobe for through-skull fluorescence imaging of brain vessels beyond 1500 nm. Nanoscale 10, 9393–9400. doi: 10.1039/C8NR00305J
Dong, A., Ye, X., Chen, J., Kang, Y., Gordon, T., Kikkawa, J. M., et al. (2011). A generalized ligand-exchange strategy enabling sequential surface functionalization of colloidal nanocrystals. J. Am. Chem. Soc. 133, 998–1006. doi: 10.1021/ja108948z
Dong, H., Du, S. R., Zheng, X. Y., Lyu, G. M., Sun, L. D., Li, L. D., et al. (2015). Lanthanide Nanoparticles: from Design toward Bioimaging and Therapy. Chem. Rev. 115, 10725–10815. doi: 10.1021/acs.chemrev.5b00091
Fan, Y., Wang, P., Lu, Y., Wang, R., Zhou, L., Zheng, X., et al. (2018). Lifetime-engineered NIR-II nanoparticles unlock multiplexed in vivo imaging. Nat. Nanotechnol. 13, 941–946. doi: 10.1038/s41565-018-0221-0
Fan, Y., Wang, S., and Zhang, F. (2019). Optical multiplexed bioassays for improved biomedical diagnostics. Angew. Chem. Int. Ed. 58, 13208–13219. doi: 10.1002/anie.201901964
Fan, Y., and Zhang, F. (2019). A new generation of NIR-II probes: lanthanide-based nanocrystals for bioimaging and biosensing. Adv. Opt. Mater. 7:1801417. doi: 10.1002/adom.201801417
Gong, H., Peng, R., and Liu, Z. (2013). Carbon nanotubes for biomedical imaging: the recent advances. Adv. Drug Deliv. Rev. 65, 1951–1963. doi: 10.1016/j.addr.2013.10.002
He, F., Feng, L., Yang, P., Liu, B., Gai, S., Yang, G., et al. (2016). Enhanced up/down-conversion luminescence and heat: simultaneously achieving in one single core-shell structure for multimodal imaging guided therapy. Biomaterials.105, 77–88. doi: 10.1016/j.biomaterials.2016.07.031
He, S., Chen, S., Li, D., Wu, Y., Zhang, X., Liu, J., et al. (2019). High affinity to skeleton rare earth doped nanoparticles for near-infrared II imaging. Nano Lett.19, 2985–2992. doi: 10.1021/acs.nanolett.9b00140
Hemmer, E., Benayas, A., Légar, É, F., and Vetrone, F. (2016). Exploiting the biological windows: current perspectives on fluorescent bioprobes emitting above 1000 nm. Nanoscale Horiz. 1, 168–184. doi: 10.1039/C5NH00073D
Hong, G., Zou, Y., Antaris, A. L., Diao, S., Wu, D., Cheng, K., et al. (2014). Ultrafast fluorescence imaging in vivo with conjugated polymer fluorophores in the second near-infrared window. Nat. Commun. 5, 1–9. doi: 10.1038/ncomms5206
Jiang, X., Cao, C., Feng, W., and Li, F. (2016). Nd3+-doped LiYF4 nanocrystals for bio-imaging in the second near-infrared window. J. Mater. Chem. B 4, 87–95. doi: 10.1039/C5TB02023A
Kamimura, M., Kanayama, N., Tokuzen, K., Soga, K., and Nagasaki, Y. (2011). Near-infrared (1550 nm) in vivo bioimaging based on rare-earth doped ceramic nanophosphors modified with PEG-b-poly (4-vinylbenzylphosphonate). Nanoscale 3, 3705–3713. doi: 10.1039/c1nr10466g
Kamimura, M., Matsumoto, T., Suyari, S., Umezawa, M., and Soga, K. K. (2017). Ratiometric near-infrared fluorescence nanothermometry in the OTN-NIR (NIR II/III) biological window based on rare-earth doped β-NaYF4 nanoparticles, J. Mater. Chem. B 5, 1917–1925. doi: 10.1039/C7TB00070G
Kantamneni, H., Zevon, M., Donzanti, M. J., Zhao, X., Sheng, Y., Barkund, S. R., et al. (2017). Surveillance nanotechnology for multi-organ cancer metastases. Na. Biomed Eng. 1, 993–1003. doi: 10.1038/s41551-017-0167-9
Kuimova, M. K., Botchway, S. W., Parker, A. W., Balaz, M., Collins, H. A., Anderson, H. L., et al. (2009). Imaging intracellular viscosity of a single cell during photoinduced cell death. Nat. Chem. 1, 69–73. doi: 10.1038/nchem.120
Lei, X., Li, R., Tu, D., Shang, X., Liu, Y., You, W., et al. (2018). Intense near-infrared-II luminescence from NaCeF4: Er/Yb nanoprobes for in vitro bioassay and in vivo bioimaging. Chem. Sci. 9, 4682–4688. doi: 10.1039/C8SC00927A
Lei, Z., Sun, C., Pei, P., Wang, S., Li, D., Zhang, X., et al. (2019). Stable, wavelength-tunable fluorescent dyes in the NIR-II region for in vivo high-contrast bioimaging and multiplexed biosensing. Angew. Chem. 131, 8250–8255. doi: 10.1002/ange.201904182
Li, C., and Lin, J. (2010). Rare earth fluoride nano-/microcrystals: synthesis, surface modification and application. J. Mater. Chem. 20, 6831–6847. doi: 10.1039/c0jm00031k
Li, C., Zhang, Y., Wang, M., Zhang, Y., Chen, G., Li, L., et al. (2014). In vivo real-time visualization of tissue blood flow and angiogenesis using Ag2S quantum dots in the NIR-II window. Biomaterials 35, 393–400. doi: 10.1016/j.biomaterials.2013.10.010
Li, D., He, S., Wu, Y., Liu, J., Liu, Q., Chang, B., et al. (2019). Excretable lanthanide nanoparticle for biomedical imaging and surgical navigation in the second near-infrared window. Adv. Sci. 6:1902042. doi: 10.1002/advs.201902042
Li, X., Jiang, M., Zeng, S., and Liu, H. (2019). Polydopamine coated multifunctional lanthanide theranostic agent for vascular malformation and tumor vessel imaging beyond 1500 nm and imaging-guided photothermal therapy. Theranostics. 9:3866. doi: 10.7150/thno.31864
Li, X., Shen, D., Yang, J., Yao, C., Che, R., Zhang, F., et al. (2013). Successive layer-by-layer strategy for multi-shell epitaxial growth: shell thickness and doping position dependence in upconverting optical properties. Chem. Mater. 25, 106–112. doi: 10.1021/cm3033498
Li, X., Wang, R., Zhang, F., and Zhao, D. (2014). Engineering homogeneous doping in single nanoparticle to enhance upconversion efficiency. Nano Lett. 14, 3634–3639. doi: 10.1021/nl501366x
Li, X., Zhang, Q., Ahmad, Z., Huang, J., Ren, Z., Weng, W., et al. (2015). Near-infrared luminescent CaTiO3: Nd3+ nanofibers with tunable and trackable drug release kinetics. J. Mater. Chem. B 3, 7449–7456. doi: 10.1039/C5TB01158B
Liang, C., Diao, S., Wang, C., Gong, H., Liu, T., Hong, G., et al. (2014). Tumor metastasis inhibition by imaging-guided photothermal therapy with single-walled carbon nanotubes. Adv. Mater. 26, 5646–5652. doi: 10.1002/adma.201401825
Liu, L., Wang, S., Zhao, B., Pei, P., Fan, Y., Li, X., et al. (2018). Er3+ sensitized 1530 nm to 1180 nm second near-infrared window upconversion nanocrystals for in vivo biosensing. Angew. Chem. Int. Ed. 57, 7518–7522. doi: 10.1002/anie.201802889
Liu, T. M., Conde, J., Lipinski, T., Bednarkiewicz, A., and Huang, C. C. (2016). Revisiting the classification of NIR-absorbing/emitting nanomaterials for in vivo bioapplications. NPG Asia Mater. 8, e295–e295. doi: 10.1038/am.2016.106
Liu, Y., Fan, H., Guo, Q., Jiang, A., Du, X., and Zhou, J. (2017). Ultra-small pH-responsive Nd-doped NaDyF4 nanoagents for enhanced cancer theranostic by in situ aggregation. Theranostics 7:4217. doi: 10.7150/thno.21557
Ma, L., Liu, Y., Liu, L., Jiang, A., Mao, F., Liu, D., et al. (2018). Simultaneous activation of short-wave infrared (SWIR) light and paramagnetism by a functionalized shell for high penetration and spatial resolution theranostics. Adv. Func. Mater. 28:1705057. doi: 10.1002/adfm.201705057
Mai, H. X., Zhang, Y. W., Sun, L. D., and Yan, C. H. (2007). Size-and phase-controlled synthesis of monodisperse NaYF4: yb, Er nanocrystals from a unique delayed nucleation pathway monitored with upconversion spectroscopy. J. Phys. Chem. C 111, 13730–13739. doi: 10.1021/jp073919e
Mariani, G., Gipponi, M., Moresco, L., Villa, G., Bartolomei, M., Mazzarol, G., et al. (2002). Radioguided sentinel lymph node biopsy in malignant cutaneous melanoma. J. Nucl. Med. 43, 811–827.
Mariani, G., Moresco, L., Viale, G., Villa, G., Bagnasco, M., Canavese, G., et al. (2001). Radioguided sentinel lymph node biopsy in breast cancer surgery. J Nucl. Med. 42, 1198–1215.
Naczynski, D. J., Tan, M. C., Zevon, M., Wall, B., Kohl, J., Kulesa, A., et al. (2013). Rare-earth-doped biological composites as in vivo shortwave infrared reporters. Nat. Commun. 4:2199. doi: 10.1038/ncomms3199
O'Leary, D. H., Polak, J. F., Kronmal, R. A., Manolio, T. A., Burke, G. L., Wolfson, S. K., et al. (1999). Carotid-artery intima and media thickness as a risk factor for myocardial infarction and stroke in older adults. N. Engl. J. Med. 340, 14–22. doi: 10.1056/NEJM199901073400103
Ortgies, D. H., Tan, M., Ximendes, E. C., Del Rosal, B., Hu, J., Xu, L., et al. (2018). Lifetime-encoded infrared-emitting nanoparticles for in vivo multiplexed imaging. ACS Nano. 12, 4362–4368. doi: 10.1021/acsnano.7b09189
Paulus, M. J., Gleason, S. S., Kennel, S. J., Hunsicker, P. R., and Johnson, D. K. (2000). High resolution X-ray computed tomography: an emerging tool for small animal cancer research. Neoplasia 2, 62–70. doi: 10.1038/sj.neo.7900069
Quon, A., and Gambhir, S. S. (2005). FDG-PET and beyond: molecular breast cancer imaging. J. Clin. Oncol. 23, 1664–1673. doi: 10.1200/JCO.2005.11.024
Rocha, U., Kumar, K. U., Jacinto, C., Villa, I., Sanz-Rodríguez, F., de la Cruz, M. D. C. I., et al. (2014). Neodymium-doped LaF3 nanoparticles for fluorescence bioimaging in the second biological window. Small 10, 1141–1154. doi: 10.1002/smll.201301716
Rubin, E. H., Allen, J. D., Nowak, J. A., and Bates, S. E. (2014). Developing precision medicine in a global world. Clin. Cancer Res. 20, 1419–1427. doi: 10.1158/1078-0432.CCR-14-0091
Stouwdam, J. W., and van Veggel, F. C. (2002). Near-infrared emission of redispersible Er3+, Nd3+, and Ho3+ doped LaF3 nanoparticles. Nano Lett. 2, 733–737. doi: 10.1021/nl025562q
Tan, M. C., Kumar, G. A., Riman, R. E., Brik, M. G., Brown, E., and Hommerich, U. (2009). Synthesis and optical properties of infrared-emitting YF3: Nd nanoparticles. J. Appl. Phys. 106:063118. doi: 10.1063/1.3168442
Tan, M. C., Naczynski, D. J., Moghe, P. V., and Riman, R. E. E. (2013). Engineering the design of brightly-emitting luminescent nanostructured photonic composite systems. Aust. J. Chem. 66, 1008–1020. doi: 10.1071/CH13221
Toussaint, J. F., Lamuraglia, G. M., Southern, J. F., Fuster, V., and Kantor, H. L. (1996). Magnetic resonance images lipid, fibrous, calcified, hemorrhagic, and thrombotic components of human atherosclerosis in vivo. Circulation 94, 932–938. doi: 10.1161/01.CIR.94.5.932
Tsien, R. Y. (2003). Imagining imaging's future. Nat. Rev. Mol. Cell Biol. (Suppl.). 4:SS16-SS21. doi: 10.1038/nrm1196
Vahrmeijer, A. L., Hutteman, M., Van Der Vorst, J. R., Van De Velde, C. J., and Frangioni, J. V. (2013). Image-guided cancer surgery using near-infrared fluorescence. Nat. Rev. Clin. Oncol. 10, 507–518. doi: 10.1038/nrclinonc.2013.123
Villa, I., Vedda, A., Cantarelli, I. X., Pedroni, M., Piccinelli, F., Bettinelli, M., et al. (2015). 1.3 μm emitting SrF2: Nd3+ nanoparticles for high contrast in vivo imaging in the second biological window. Nano Res. 8, 649–665. doi: 10.1007/s12274-014-0549-1
Wang, D., Rogach, A.L., and Caruso, F. (2002). Nanocrystal-labeled biofunctional colloids. Nano Lett. 2:857. doi: 10.1021/nl025624c
Wang, D., Wang, D., Kuzmin, A., Pliss, A., Shao, W., Xia, J., et al. (2018). ICG-sensitized NaYF4: er nanostructure for theranostics. Adv. Opt. Mater. 6:1701142. doi: 10.1002/adom.201701142
Wang, F., and Liu, X. (2009). Recent advances in the chemistry of lanthanide-doped upconversion nanocrystals. Chem. Soc. Rev. 38, 976–989. doi: 10.1039/b809132n
Wang, F., Zhang, Y., Fan, X., and Wang, M. (2006). Facile synthesis of water-soluble LaF3: Ln 3+ nanocrystals. J. Mater. Chem.16, 1031–1034. doi: 10.1039/b518262j
Wang, H., Agarwal, P., Zhao, S., Yu, J., Lu, X., and He, X. (2015). A biomimetic hybrid nanoplatform for encapsulation and precisely controlled delivery of theranostic agents. Nat. Commun. 6, 1–13. doi: 10.1038/ncomms10081
Wang, P., Fan, Y., Lu, L., Liu, L., Fan, L., Zhao, M., et al. (2018). NIR-II nanoprobes in-vivo assembly to improve image-guided surgery for metastatic ovarian cancer. Nat. Commun. 9:2898. doi: 10.1038/s41467-018-05113-8
Wang, Q., Tan, M. C., Zhuo, R., Kumar, G. A., and Riman, R. E. (2010). A solvothermal route to size-and phase-controlled highly luminescent NaYF4: Yb, Er up-conversion nanocrystals. J. Nanosci. Nanotechnol. 10, 1685–1692. doi: 10.1166/jnn.2010.2120
Wang, R., Li, X., Zhou, L., and Zhang, F. (2014). Epitaxial seeded growth of rare-earth nanocrystals with efficient 800 nm near-infrared to 1525 nm short-wavelength infrared downconversion photoluminescence for in vivo bioimaging. Angew. Chem. Int. Ed. 53, 12086–12090. doi: 10.1002/anie.201407420
Wang, S., Fan, Y., Li, D., Sun, C., Lei, Z., Lu, L., et al. (2019a). Anti-quenching NIR-II molecular fluorophores for in vivo high-contrast imaging and pH sensing. Nat. Commun. 10, 1–11. doi: 10.1038/s41467-019-09043-x
Wang, S., Liu, L., Fan, Y., El-Toni, A. M., Alhoshan, M. S., Li, D., et al. (2019b). In vivo high-resolution ratiometric fluorescence imaging of inflammation using NIR-II nanoprobes with 1550 nm emission. Nano Lett. 19, 2418–2427. doi: 10.1021/acs.nanolett.8b05148
Wang, X., Li, H., Li, F., Han, X., and Chen, G. (2019c). Prussian blue-coated lanthanide-doped core/shell/shell nanocrystals for NIR-II image-guided photothermal therapy. Nanoscale 11, 22079–22088. doi: 10.1039/C9NR07973D
Wang, Y. F., Liu, G. Y., Sun, L. D., Xiao, J. W., Zhou, J. C., and Yan, C. H. (2013). Nd3+-sensitized upconversion nanophosphors: efficient in vivo bioimaging probes with minimized heating effect. ACS Nano. 7, 7200–7206. doi: 10.1021/nn402601d
Weissleder, R., and Pittet, M. J. (2008). Imaging in the era of molecular oncology. Nature 452, 580–589. doi: 10.1038/nature06917
Weissleder, R., Schwaiger, M. C., Gambhir, S. S., and Hricak, H. (2016). Imaging approaches to optimize molecular therapies. Sci. Transl. Med. 8:355ps16. doi: 10.1126/scitranslmed.aaf3936
Willmann, J. K., Van Bruggen, N., Dinkelborg, L. M., and Gambhir, S. S. (2008). Molecular imaging in drug development. Nat. Rev. Drug Discov. 7, 591–607. doi: 10.1038/nrd2290
Winer, J. H., Choi, H. S., Gibbs-Strauss, S. L., Ashitate, Y., Colson, Y. L., and Frangioni, J. V. (2010). Intraoperative localization of insulinoma and normal pancreas using invisible near-infrared fluorescent light. Ann. Surg. Oncol. 17, 1094–1100. doi: 10.1245/s10434-009-0868-8
Xue, Z., Zeng, S., and Hao, J. (2018). Non-invasive through-skull brain vascular imaging and small tumor diagnosis based on NIR-II emissive lanthanide nanoprobes beyond 1500 nm. Biomaterials 171, 153–163. doi: 10.1016/j.biomaterials.2018.04.037
Yang, Q., Li, X., Xue, Z., Li, Y., Jiang, M., and Zeng, S. (2018). Short-wave near-infrared emissive GdPO4: Nd 3+ theranostic probe for in vivo bioimaging beyond 1300 nm. RSC Adv. 8, 12832–12840. doi: 10.1039/C7RA12864A
Yang, Y., Aw, J., and Xing, B. (2017c). Nanostructures for NIR light-controlled therapies. Nanoscale 9, 3698–3718. doi: 10.1039/C6NR09177F
Yang, Y., Chao, Y., Liu, J., Dong, Z., He, W., Zhang, R., et al. (2017a). Core-shell and co-doped nanoscale metal-organic particles (NMOPs) post-synthesis cation exchange for multimodal imaging and synergistic thermo-radiotherapy. NPG Asia Mater. 9:e344. doi: 10.1038/am.2016.205
Yang, Y., Mu, J., and Xing, B. (2017b). Photoactivated drug delivery and bioimaging. Wiley Interdiscip. Rev. Nanomed. Nanobiotechnol. 9:e1408. doi: 10.1002/wnan.1408
Yu, Z. F., Shi, J. P., Li, J. L., Li, P. H., and Zhang, H. W. (2018). Luminescence enhancement of CaF2: Nd3+ nanoparticles in the second near-infrared window for in vivo imaging through Y3+ doping. J. Mater. Chem. B 6, 1238–1243. doi: 10.1039/C7TB03052E
Yuan, D., Tan, M. C., Riman, R. E., and Chow, G. M. (2013). Comprehensive study on the size effects of the optical properties of NaYF4: Yb, Er nanocrystals. J. Phys. Chem. C 117, 13297–13304. doi: 10.1021/jp403061h
Zevon, M., Ganapathy, V., Kantamneni, H., Mingozzi, M., Kim, P., Adler, D., et al. (2015). CXCR-4 targeted, short wave infrared (SWIR) emitting nanoprobes for enhanced deep tissue imaging and micrometastatic cancer lesion detection. Small 11, 6347–6357. doi: 10.1002/smll.201502202
Zhang, F., Wan, Y., Yu, T., Zhang, F., Shi, Y., Xie, S., et al. (2007). Uniform nanostructured arrays of sodium rare-earth fluorides for highly efficient multicolor upconversion luminescence. Angew. Chem., Int. Ed. 46, 7976–7979. doi: 10.1002/anie.200702519
Zhang, H., Chen, Z. H., Liu, X., and Zhang, F. (2020). A mini-review on recent progress of new sensitizers for luminescence of lanthanide doped nanomaterials. Nano Res. 1-15. doi: 10.1007/s12274-020-2661-8
Zhang, H., Fan, Y., Pei, P., Sun, C., Lu, L., and Zhang, F. (2019). Tm3+-Sensitized NIR-II Fluorescent Nanocrystals for in vivo Information Storage and Decoding. Angew. Chem. 131, 10259–10263. doi: 10.1002/ange.201903536
Zhang, X., Zhao, Z., Zhang, X., Cordes, D. B., Weeks, B., Qiu, B., et al. (2015). Magnetic and optical properties of NaGdF4: Nd3+, Yb3+, Tm3+ nanocrystals with upconversion/downconversion luminescence from visible to the near-infrared second window. Nano Res. 8, 636–648. doi: 10.1007/s12274-014-0548-2
Zhao, M., Li, B., Wang, P., Lu, L., Zhang, Z., Liu, L., et al. (2018). Supramolecularly engineered NIR-II and upconversion nanoparticles in vivo assembly and disassembly to improve bioimaging. Adv. Mater. 30:1804982. doi: 10.1002/adma.201804982
Zhao, M., Wang, R., Li, B., Fan, Y., Wu, Y., Zhu, X., et al. (2019). Precise in vivo inflammation imaging using in situ responsive cross-linking of glutathione-modified ultra-small NIR-II lanthanide nanoparticles. Angew. Chem. 131, 2072–2076. doi: 10.1002/ange.201812878
Zhao, Z., Kantamneni, H., He, S., Pelka, S., Venkataraman, A. S., Kwon, M., et al. (2018). Surface-modified shortwave-infrared-emitting nanophotonic reporters for gene-therapy applications. ACS Biomater. Sci. Eng. 4, 2350–2363. doi: 10.1021/acsbiomaterials.8b00378
Keywords: NIR-II, rare-earth-doped nanoparticles, modification, in vitro and in vivo imaging, cancer theranostics
Citation: Yu Z, Eich C and Cruz LJ (2020) Recent Advances in Rare-Earth-Doped Nanoparticles for NIR-II Imaging and Cancer Theranostics. Front. Chem. 8:496. doi: 10.3389/fchem.2020.00496
Received: 24 March 2020; Accepted: 14 May 2020;
Published: 17 June 2020.
Edited by:
Jianhua Zhang, Tianjin University, ChinaCopyright © 2020 Yu, Eich and Cruz. This is an open-access article distributed under the terms of the Creative Commons Attribution License (CC BY). The use, distribution or reproduction in other forums is permitted, provided the original author(s) and the copyright owner(s) are credited and that the original publication in this journal is cited, in accordance with accepted academic practice. No use, distribution or reproduction is permitted which does not comply with these terms.
*Correspondence: Luis J. Cruz, bC5qLmNydXpfcmljb25kbyYjeDAwMDQwO2x1bWMubmw=