- 1Key Laboratory for Advanced Materials and Feringa Nobel Prize Scientist Joint Research Center, School of Chemistry and Molecular Engineering, Institute of Fine Chemicals, East China University of Science and Technology, Shanghai, China
- 2Department of Medicinal Chemistry, School of Pharmacy, Fudan University, Shanghai, China
Real-time and accurate detection of endogenous hydrogen sulfide is of great biomedical significance. Here, a FRET-based fluorescent probe for ratiometric detection of H2S was designed to comprise an AIE luminophore TPE as an energy donor and a monochlorinated BODIPY dye as an energy acceptor and H2S-responsive site. Such a designed probe showed H2S-dependent ratiometric and light-up NIR-II emission, enabling accurate imaging of H2S-rich cancer cells and identification of H2S-rich tumors with high resolution.
Introduction
Endogenous hydrogen sulfide is an important signaling molecule, mainly derived from the enzymatic hydrolysis of L-cysteine (Chiku et al., 2009; Singh et al., 2009). Studies have found that H2S is associated with many pathological processes, while an abnormal level of H2S may associated with some diseases, such as Alzheimer's disease, hypertension, and cardiac ischemia disease (Eto et al., 2002; Zhao et al., 2015; Shi et al., 2017). Therefore, real-time and accurate detection of hydrogen sulfide is of great biomedical significance. Until now, many fluorescence-based H2S probes have been reported (Jin et al., 2016; Wang et al., 2018, 2019b); however, the fluorescence of many probes generally locates in the visible or the near-infrared I region (650–900 nm), inevitably leading to some drawbacks of poor tissue penetration, severe background interference from living tissue (Zhou et al., 2014). Compared with the traditional NIR-I imaging (650–900 nm) (Li et al., 2018), fluorescent imaging in the second near-infrared window (NIR-II, 1,000–1,700 nm) has attracted more and more attention due to lower tissue absorption, stronger tissue penetration, and reduced autofluorescence (Hong et al., 2012; Dang et al., 2016; Shi et al., 2018; Xu et al., 2018). Another issue is the hydrophobic nature of most traditional fluorescent dyes, which generally triggers the aggregation in physiological conditions due to π-π stacking. Such a process can give rise to aggregation-caused quenching (ACQ) (Sun et al., 2014; Yuan et al., 2015) and thus compromise the accuracy of bioimaging. In comparison, fluorogens with AIE characteristics show enhanced fluorescence in the aggregate states, thus providing an alternative strategy for the design of fluorescent light up probes (Zhao et al., 2012; Mei et al., 2014, 2015; Zhang et al., 2015; Fu et al., 2019). Since the hydrophobic fluorescent probes undergo the intrinsic aggregation process in aqueous media, it is desirable to develop H2S-activatable probes with AIE characteristic for in vivo imaging.
Herein, we reported a H2S-responsive probe that showed ratiometric fluorescence and NIR-II emission light-up upon activation for in vitro and in vivo imaging (Scheme 1). Such a probe was designed by appending an AIE luminophore TPE, as an energy donor, to a monochlorinated BODIPY dye as an energy acceptor and H2S-responsive site. As compared to conventional intensity-based fluorescent probes (Huang et al., 2014; Tang et al., 2016), this Förster resonance energy transfer (FRET)-based ratiometric probe can enable accurate detection through elimination of the limitations of experimental conditions including probe concentration, light source, and background interference effects (Wang et al., 2019a). Such a design strategy is applicable to the design of various ratiometric probes for different targets. As expected, in the absence of H2S, due to the good spectral overlap between the emission spectra of the TPE and the absorption spectra of the BODIPY, efficient FRET occurs. In contrast, in the presence of H2S, the absorption spectra of BODIPY undergo an obvious red shift, resulting in a significant reduction of the overlap with the TPE emission. Correspondingly, the FRET process is significantly attenuated. More importantly, upon activation by H2S, the probe produces a new fluorescence light-up at 920 nm with the fluorescence tail extending to 1,300 nm, indicative of the suitability for fluorescent imaging in the second near-infrared (NIR-II).
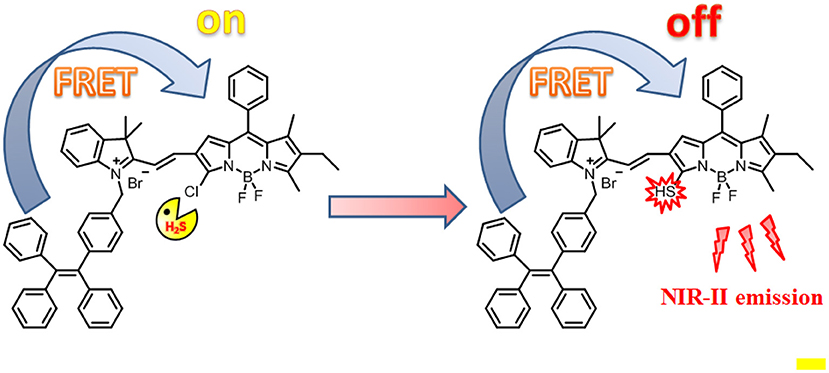
Scheme 1. Schematic illustration of the design of probe TPE-BODIPY-Cl and the mechanism for H2S-mediated ratiometric and NIR fluorescence light up.
Experimental
Synthesis
The TPE-BODIPY-Cl was obtained from the synthetic route of Scheme 2. Br-TPE and BODIPY were synthesized according to the literature procedure (Zhao et al., 2013, 2014). Animal experiments were performed in compliance with Chinese legislation on the Use and Care of Research Animals and guidelines by Fudan University Animal Studies Committee for the Care and Use of Laboratory Animals. All experimental procedures were approved by this committee.
Synthesis of Compound A
Br-TPE (165 mg, 0.39 mmol) and 2,3,3-trimethyl-3H-indole (62 mg, 0.39 mmol) were dissolved in 25 mL CH3CN and refluxed for 10 h. Then, the solvent was removed under reduced pressure, and the crude product was dissolved in CH2Cl2. The mixture was dropped into the ether solvent to precipitate white solid, which was used for next reaction without further purification. HRMS (ESI, m/z): calculated for C38H34N [M-Br]+: 504.2691, found: 504.2699.
Synthesis of Compound TPE-BODIPY-Cl
Compound A (100 mg, 0.17 mmol) and BODIPY (80 mg, 0.21 mmol) were dissolved in dry ethanol and refluxed for 4 h. Then, EtOH was evaporated and the crude product was purified by a silica gel column with CH2Cl2/MeOH (20/1, v/v) as eluent to give TPE-BODIPY-Cl (90 mg, 56%). 1H NMR (400 MHz, CDCl3) δ 8.00 (d, 1H, J = 12.00 Hz), 7.63–7.55 (m, 2H), 7.52–7.46 (m, 4H), 7.43–7.36 (m, 2H), 7.09–7.06 (m, 7H), 7.03–7.00 (m, 3H), 6.98–6.96 (m, 4H), 6.95–6.92 (m, 4H), 6.87–6.85 (m, 4H), 6.08 (s, 2H), 2.71 (s, 3H), 2.44-2.39 (q, 2H, J = 6.67 Hz), 1.78 (s, 6H), 1.52 (s, 3H), 1.09–1.05 (t, 3H, J = 8.00 Hz). 13C NMR (101 MHz, CDCl3) δ 144.43, 144.21, 143.73, 143.25, 143.06, 142.64, 141.75, 141.48, 139.85, 132.12, 131.79, 131.20, 130.44, 129.50, 129.38, 129.06, 128.96, 127.76, 127.68, 127.61, 126.62, 126.52, 126.39, 122.35, 115.31, 51.90, 31.94, 29.71, 29.67, 29.37, 27.60, 22.71, 17.29, 14.14, 13.94, 13.90, 12.81. HRMS (ESI, m/z): calculated for C58H50BF2N3Cl [M-Br]+: 872.3754, found: 872.3750.
Results and Discussion
TPE-BODIPY-Cl was prepared via a Knoevenagel condensation reaction. The synthesis and characterization are outlined in Scheme 2 and Supporting Information.
Spectroscopic Studies of TPE-BODIPY-Cl
With the probe in hand, we initially evaluated the photophysical properties. Because the probe contains the TPE AIEgen, we explored the AIE performance of the probe to obtain the best test conditions. As shown in Figure 1A, we tested the FRET process of the probe under different ratios of H2O/CH3CN. With the increasing water content, the degree of aggregation of the probe intensifies, accompanying the increase of TPE fluorescence while the occurrence of ACQ for BODIPY chromophore. As is well-known, a ratiometric fluorescence mode has higher accuracy than turn-on or turn-off fluorescence detection mode (Wang et al., 2015; Zhang et al., 2019), we here selected Tris/CH3CN buffer solution (0.5 M Tris, 40% CH3CN, pH = 7.4) as the next testing condition in order to obtain the ratiometric fluorescent responsiveness. The aggregation of our probe under this buffer solution was proven by dynamic light scattering (Figure S1).
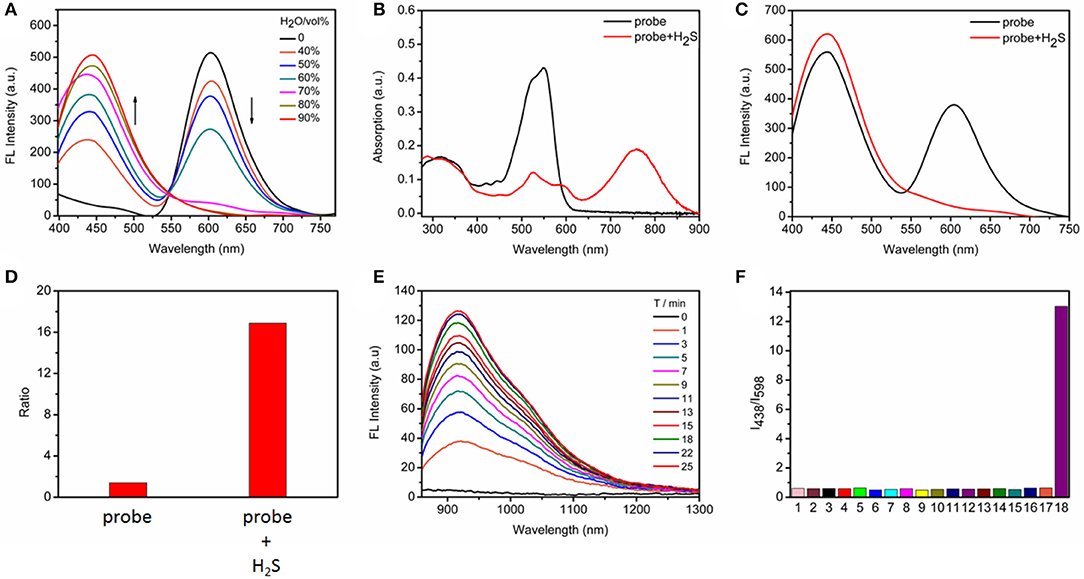
Figure 1. (A) Fluorescence changes of TPE-BODIPY-Cl (10 μM) in Tris/CH3CN mixtures with different water fractions. (B) Absorption and (C) fluorescence spectra in the absence and presence of 100 μM NaHS in Tris/CH3CN buffer solution (0.5 M Tris-HCl, 40% CH3CN, pH = 7.4), λex = 360 nm. (D) The fluorescence intensity ratio (I438/I598) in the absence and presence of 100 μM NaHS. (E) Time-dependent NIR-II emission spectra upon addition of 100 μM NaHS, λex = 760 nm. (F) Ratiometric fluorescence changes of TPE-BODIPY-Cl in the presence of 100 μM NaHS and other biologically relevant reactive sulfur and anions (1 mM) in Tris/CH3CN buffer solution (0.5 M Tris-HCl, 40% CH3CN, pH = 7.4): (1) Free; (2) F−; (3) Cl−; (4) Br−; (5) I−; (6) ; (7) ; (8) ; (9) ; (10) ; (11) ClO−; (12) H2O2; (13) −OAc; (14) S2; (15) GSH; (16) Cys; (17) Hcy; (18) NaHS.
Next, we evaluated the response capability of TPE-BODIPY-Cl toward H2S (Figure 1 and Figure S2). As shown in Figure 1B, the free probe showed strong absorption at 550 nm. The typical absorption band of the TPE around 300–360 nm was also noted. In the fluorescence spectrum, due to FRET process, we can observe two strong fluorescence peaks with maxima at 438 and 598 nm, corresponding to TPE and BODIPY, respectively. When treated with 100 μM H2S, the absorption band at 550 nm decreased significantly and a new absorption band appeared at 760 nm with a red-shift of 220 nm due to the formation of TPE-BODIPY-SH that was proven by HRMS analysis (Figure S3). Such treatment with NaHS attenuated the FRET, thus affording an enhancement of the fluorescence intensity ratio (I438/I598) by 12 times. This indicated that TPE-BODIPY-Cl was indeed a ratiometric fluorescent probe for H2S. Most importantly, H2S-triggered a new NIR-II fluorescence light up with a maximum emission of 920 nm (λex = 760 nm). These results demonstrated that TPE-BODIPY-Cl could be used as a H2S-activatable NIR-II fluorescent probe to enable high-resolution bioimaging with deep-tissue penetration. Utilizing the linear relation of fluorescence intensity ratio at 438 and 598 nm with H2S concentration (0–50 μM) (Figure S4), the detection limit was determined to be 6.5 × 10−7 M, indicating that TPE-BODIPY-Cl has high sensitivity for H2S detection. Of note, the probe exhibits minimal optical responsiveness to biologically related reactive sulfur (RSS), oxygen (ROS), and nitrogen species (RNS) and some ions, showing its high selectivity for H2S (Figure 1F and Figure S5). In addition, the good photostability of probe TPE-BODIPY-Cl, evidenced by minimal optical changes under continuous irradiation with light irradiation (Figure S6), indicated its suitability for bioimaging.
Imaging of H2S in Living Cells
Inspired by the promising response to H2S, we then assessed the ability of TPE-BODIPY-Cl for fluorescence imaging in living HCT116 cells that express high levels of H2S [20–100 μM, Szabo et al., 2013]. As shown in Figure 2, the incubation of HCT116 cells and 10 μM TPE-BODIPY-Cl for 30 min afforded the bright and stable fluorescence signal in the green channel and relatively weak fluorescence in the red channel. The ratio of the green to red channel is ~2.82. When a CBS inhibitor aminooxyacetic acid (AOAA) which can inhibit the H2S production was added, the ratio dropped to 0.70. In contrast, with the addition of an allosteric CBS activator S-adenosyl-L-methionine (SAM) to promote the production of H2S, the ratio increased to 3.59. These results indicated that TPE-BODIPY-Cl can efficiently enter living cells and serve as a potential sensor to detect endogenous hydrogen sulfide rapidly and specifically.
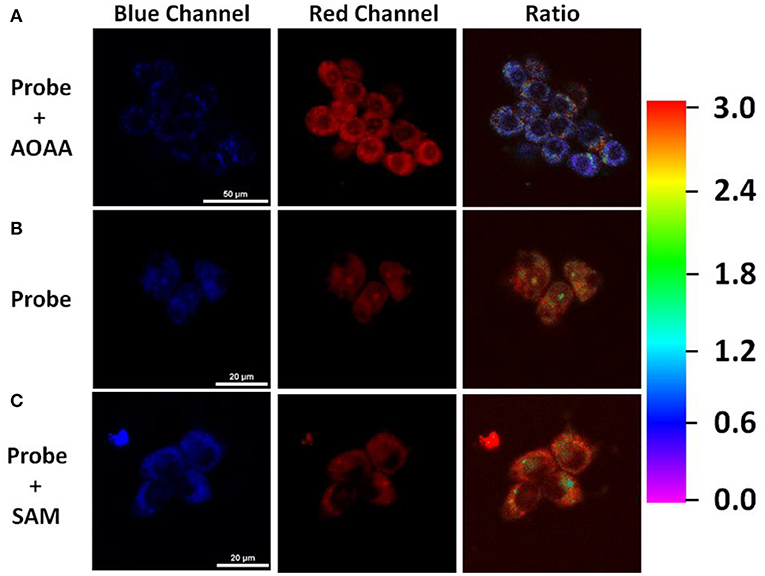
Figure 2. (A) HCT116 cells pretreated with 1 mM AOAA for 1 h, followed by incubation with TPE-BODIPY-Cl (10 μM) for 30 min. (B) HCT116 cells incubated with TPE-BODIPY-Cl for 30 min. (C) HCT116 cells pretreated with SAM (3 mM) for 1 h, followed by loading with TPE-BODIPY-Cl for 30 min.
Imaging of H2S in vivo
Finally, we explored the ability of the probe for visualizing H2S-rich cancers using HCT116 subcutaneous xenograft nude mice. TPE-BODIPY-Cl was administrated to nude mice through intratumoral injection. As shown in Figure 3, after the injection, obvious NIR-II fluorescence in the tumor region was observed and the signals gradually increased over time, producing a 14.8-fold enhancement at the time point of 60 min (Figure S7). These results indicated that the TPE-BODIPY-Cl could be activation of NIR-II fluorescence in H2S-rich colorectal cancers.
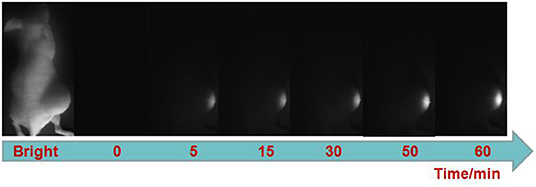
Figure 3. NIR-II fluorescent images of HCT116 subcutaneous xenograft nude mice. Images were taken at various time points after subcutaneous injection of TPE-BODIPY-Cl into tumor region.
Conclusion
In summary, we have designed a FRET based probe through appending the AIE luminophore TPE to the monochlorinated BODIPY dye for imaging of H2S-rich cancer cells and tumors, wherein TPE serves as an energy donor and BODIPY dye as an energy acceptor. This probe showed H2S-dependent FRET process, thus enabling the selective visualization of endogenous H2S in HCT116 cells. Furthermore, this probe displayed H2S specific activation of NIR II emission light up. By using this activatable NIR II emission, accurate identification of colorectal tumors was realized. We expect our design strategy here can help the development of a new activatable probe.
Data Availability Statement
The raw data supporting the conclusions of this manuscript will be made available by the authors, without undue reservation, to any qualified researcher.
Ethics Statement
The animal study was reviewed and approved by Fudan University.
Author Contributions
CZ and XG conceived the project and wrote the manuscript. RW conceived the molecule design. RW and WG prepared and characterized the small molecule. RW, TZ, and GX performed the optical characterization. RW and JG performed the living cells imaging, in vivo imaging, and analyzed the data.
Conflict of Interest
The authors declare that the research was conducted in the absence of any commercial or financial relationships that could be construed as a potential conflict of interest.
Acknowledgments
We gratefully acknowledge the financial support by the National Natural Science Foundation of China (21672062, 21874043, and 21572039).
Supplementary Material
The Supplementary Material for this article can be found online at: https://www.frontiersin.org/articles/10.3389/fchem.2019.00778/full#supplementary-material
References
Chiku, T., Padovani, D., Zhu, W., Singh, S., Vitvitsky, V., and Banerjee, R. J. (2009). H2S biogenesis by human cystathionine γ-lyase leads to the novel sulfur metabolites lanthionine and homolanthionine and is responsive to the grade of hyperhomocysteinemia. Biol. Chem. 284, 11601–11612. doi: 10.1074/jbc.M808026200
Dang, X., Gu, L., Qi, J., Correa, S., Zhang, G., Belcher, A. M., et al. (2016). Layer-by-layer assembled fluorescent probes in the second near-infrared window for systemic delivery and detection of ovarian cancer. Proc. Natl. Acad. Sci. U.S.A. 113, 5179–5184. doi: 10.1073/pnas.1521175113
Eto, K., Asada, T., Arima, K., Makifuchi, T., and Kimura, H. (2002). Brain hydrogen sulfide is severely decreased in Alzheimer's disease. Biochem. Biophys. Res. Commun. 293, 1485–1488. doi: 10.1016/S0006-291X(02)00422-9
Fu, W., Yan, C., Zhang, Y., Ma, Y., Guo, Z., and Zhu, W.-H. (2019). Near-infrared aggregation-induced emission-active probe enables in situ and long-term tracking of endogenous b-galactosidase activity. Front. Chem. 7:291. doi: 10.3389/fchem.2019.00291
Hong, G., Lee, J. C., Robinson, J. T., Raaz, U., Xie, L., Huang, N. F., et al. (2012). Multifunctional in vivo vascular imaging using near-infrared II fluorescence. Nat. Med. 18, 1841–1846. doi: 10.1038/nm.2995
Huang, C., Jia, T., Tang, M., Yin, Q., Zhu, W., Zhang, C., et al. (2014). Selective and ratiometric fluorescent trapping and quantification of protein vicinal dithiols and in situ dynamic tracing in living cells. J. Am. Chem. Soc. 136, 14237–14244. doi: 10.1021/ja5079656
Jin, X., Wu, S., She, M., Jia, Y., Hao, L., Yin, B., et al. (2016). A novel fluorescein-based fluorescent probe for detecting H2S and its real applications in blood plasma and biological imaging. Anal. Chem. 88, 11253–11260. doi: 10.1021/acs.analchem.6b04087
Li, H., Yao, Q., Xu, F., Xu, N., Sun, W., Long, S., et al. (2018). Lighting-up tumor for assisting resection via spraying NIR fluorescent probe of g-glutamyltranspeptidas. Front. Chem. 6:485. doi: 10.3389/fchem.2018.00485
Mei, J., Hong, Y., Lam, J. W., Qin, A., Tang, Y., and Tang, B. Z. (2014). Aggregation-induced emission: the whole is more brilliant than the parts. Adv. Mater. 26, 5429–5479. doi: 10.1002/adma.201401356
Mei, J., Leung, N. L., Kwok, R. T., Lam, J. W., and Tang, B. Z. (2015). Aggregation-induced emission: together we shine, united we soar!. Chem. Rev. 115, 11718–11940. doi: 10.1021/acs.chemrev.5b00263
Shi, B., Gu, X., Fei, Q., and Zhao, C. (2017). Photoacoustic probes for real-time tracking of endogenous H2S in living mice. Chem. Sci. 8, 2150–2155. doi: 10.1039/C6SC04703C
Shi, B., Yan, Q., Tang, J., Xin, K., Zhang, J., Zhu, Y., et al. (2018). Hydrogen sulfide-activatable second near-infrared fluorescent nanoassemblies for targeted photothermal cancer therapy. Nano Lett. 18, 6411–6416. doi: 10.1021/acs.nanolett.8b02767
Singh, S., Padovani, D., Leslie, R. A., Chiku, T., and Banerjee, R. J. (2009). Relative contributions of cystathionine β-synthase and γ-cystathionase to H2S biogenesis via alternative trans-sulfuration reactions. Biol. Chem. 284, 22457–22466. doi: 10.1074/jbc.M109.010868
Sun, X., Xu, Q., Kim, G., Flower, S. E., Lowe, J. P., Yoon, J., et al. (2014). A water-soluble boronate-based fluorescent probe for the selective detection of peroxynitrite and imaging in living cells. Chem. Sci. 5, 3368–3373. doi: 10.1039/C4SC01417K
Szabo, C., Coletta, C., Chao, C., Módis, K., Szczesny, B., Papapetropoulos, A., et al. (2013). Tumor-derived hydrogen sulfide, produced by cystathionine-β-synthase, stimulates bioenergetics, cell proliferation, and angiogenesis in colon cancer. Proc. Natl. Acad. Sci. U.S.A. 110, 12474–12479. doi: 10.1073/pnas.1306241110
Tang, M., Wu, L., Wu, D., Huang, C., Zhu, W., Xu, Y., et al. (2016). An “off–on” fluorescent probe for the detection of cysteine /homocysteine and its imaging in living cells. RSC Adv. 6, 34996–35000. doi: 10.1039/C6RA00832A
Wang, F., Xu, G., Gu, X., Wang, Z., Wang, Z., Shi, B., et al. (2018). Realizing highly chemoselective detection of H2S in vitro and in vivo with fluorescent probes inside core-shell silica nanoparticles. Biomaterials 159, 82–90. doi: 10.1016/j.biomaterials.2018.01.009
Wang, F., Zhu, Y., Zhou, L., Pan, L., Cui, Z., Fei, Q., et al. (2015). Fluorescent in situ targeting probes for rapid imaging of ovarian-cancer-specific g-glutamyltranspeptidase. Angew. Chem. Int. Ed. 54, 7349–7353. doi: 10.1002/anie.201502899
Wang, R., Chen, J., Gao, J., Chen, J.-A., Xu, G., Zhu, T., et al. (2019a). A molecular design strategy toward enzymeactivated probes with near-infrared I and II fluorescence for targeted cancer imaging. Chem. Sci. 10, 7222–7227. doi: 10.1039/C9SC02093D
Wang, R., Dong, K., Xu, G., Shi, B., Zhu, T., Shi, P., et al. (2019b). Activatable near-infrared emission-guided on-demand administration of photodynamic anticancer therapy with a theranostic nanoprobe. Chem. Sci. 10, 2785–2790. doi: 10.1039/C8SC04854A
Xu, G., Yan, Q., Lv, X., Zhu, Y., Xin, K., Shi, B., et al. (2018). Imaging of colorectal cancers using activatable nanoprobes with second near-infrared window emission. Angew. Chem. Int. Ed. 57, 3626–3630. doi: 10.1002/anie.201712528
Yuan, Y., Zhang, C.-J., Gao, M., Zhang, R., Tang, B. Z., and Liu, B. (2015). Specific light-up bioprobe with aggregation-induced emission and activatable photoactivity for the targeted and image-guided photodynamic ablation of cancer cells. Angew. Chem. Int. Ed. 54, 1780–1786. doi: 10.1002/anie.201408476
Zhang, W., Kang, J., Li, P., Wang, H., and Tang, B. (2015). Dual signaling molecule sensor for rapid detection of hydrogen sulfide based on modified tetraphenylethylene. Anal. Chem. 87, 8964–8969. doi: 10.1021/acs.analchem.5b02169
Zhang, X., Zhang, L.i., Ma, W.-W., Zhou, Y., Lu, Z.-N., and Xu, S. (2019). A near-infrared ratiometric fluorescent probe for highly selective recognition and bioimaging of cysteine. Front. Chem. 7:32. doi: 10.3389/fchem.2019.00032
Zhao, C., Zhang, J., Wang, X., and Zhang, Y. (2013). Pyridone fused boron-dipyrromethenes: synthesis and properties. Org. Biomol. Chem. 11, 372–377. doi: 10.1039/C2OB26791H
Zhao, C., Zhang, X., Li, K., Zhu, S., Guo, Z., Zhang, L., et al. (2015). FRET-switchable self-assembled micellar nanoprobe: ratiometric fluorescent trapping of endogenous H2S generation via fluvastatin-stimulated upregulation. J. Am. Chem. Soc. 137, 8490–8498. doi: 10.1021/jacs.5b03248
Zhao, Y., Wu, Y., Yan, G., and Zhang, K. (2014). Aggregation-induced emission block copolymers based on ring-opening metathesis polymerization. RSC Adv. 4, 51194–51200. doi: 10.1039/C4RA08191A
Zhao, Z., Lam, J. W. Y., and Tang, B. Z. (2012). Tetraphenylethene: a versatile AIE building block for the construction of efficient luminescent materials for organic light-emitting diodes. J. Mater. Chem. 22, 23726–23740. doi: 10.1039/c2jm31949g
Keywords: ratiometric, light up, FRET, AIE, NIR-II imaging
Citation: Wang R, Gao W, Gao J, Xu G, Zhu T, Gu X and Zhao C (2019) A Förster Resonance Energy Transfer Switchable Fluorescent Probe With H2S-Activated Second Near-Infrared Emission for Bioimaging. Front. Chem. 7:778. doi: 10.3389/fchem.2019.00778
Received: 30 July 2019; Accepted: 28 October 2019;
Published: 25 November 2019.
Edited by:
Zhen Shen, Nanjing University, ChinaReviewed by:
Chusen Huang, Shanghai Normal University, ChinaShaomin Ji, Guangdong University of Technology, China
Copyright © 2019 Wang, Gao, Gao, Xu, Zhu, Gu and Zhao. This is an open-access article distributed under the terms of the Creative Commons Attribution License (CC BY). The use, distribution or reproduction in other forums is permitted, provided the original author(s) and the copyright owner(s) are credited and that the original publication in this journal is cited, in accordance with accepted academic practice. No use, distribution or reproduction is permitted which does not comply with these terms.
*Correspondence: Xianfeng Gu, eGZndUBmdWRhbi5lZHUuY24=; Chunchang Zhao, emhhb2NjaGFuZ0BlY3VzdC5lZHUuY24=
†These authors have contributed equally to this work