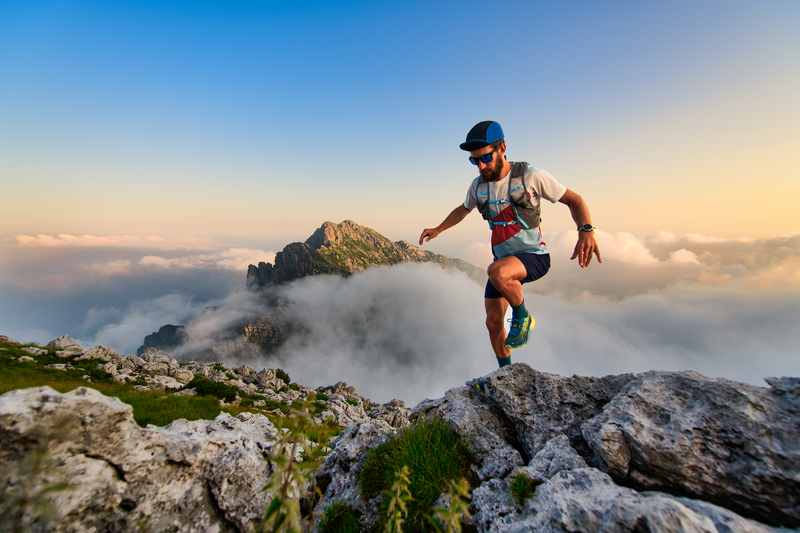
95% of researchers rate our articles as excellent or good
Learn more about the work of our research integrity team to safeguard the quality of each article we publish.
Find out more
MINI REVIEW article
Front. Chem. Eng. , 20 February 2024
Sec. Materials Process Engineering
Volume 6 - 2024 | https://doi.org/10.3389/fceng.2024.1334816
This study presents an overview of and innovations in reverse osmosis (RO) membrane processes for rejecting charged metal ions in wastewater in relation to the main problems associated with purification methods. It also explains the emergence of nanomaterials and the different methods applied for RO membrane modification to improve performance. Membrane regeneration and retentate management are also considered. The study concludes with an economic feasibility study for the industrial scale-up of the methodology.
Both the environment and human health are under serious pressure from inorganic and organic micropollutants due to their non-biodegradable nature, persistence, toxicity, bioaccumulation in the food chain, and biomagnification in higher organisms (Feng et al., 2021; Peng and Bartzas, 2021). Micropollutants are contaminating flora and fauna, and the air, atmosphere, water, and soil; they have become progressively more pronounced over the past decade as a result of global population growth (Peng and Bartzas, 2021).
In ecological terms, any metal or metalloid that causes environmental pollution or has no biological interest in the organism can be considered a heavy metal (HM) (Herrera-Estrella and Guevara-Garcia, 2001). Some of these metals are micronutrients necessary for plant growth (e.g., Zn, Cu, Mn, Ni, and Co), while others have unknown biological functions and are toxic (e.g., Cd, Pb, and Hg) According to Feng et al. (2021) and Herrera-Estrella and Guevara-Garcia (2001), a “heavy metal” is a metal or metalloid element that causes environmental pollution, has no vital function, and is toxic at low concentrations (such as Pb and Hg), or has a vital function but is harmful to organisms at high concentrations (such as Cu and Mo). Heavy metals have been categorized as toxic metals (Hg, Cr, Zn, Cu, Ni, Cd, As, Co, Sn, etc.), precious metals (Pd, Pt, Ag, Au, Ru, etc.), and radionuclides (U, Th, Ra, Am, etc.) (Wang and Chen, 2009). The most common are Pb, Zn, Hg, Ni, Cd, Cu, Cr, and As, which are always dangerous, even when detected at trace levels. Table 1 below lists the main sources of heavy metals, their health effects, and their permissible drinking water standard. Other metals frequently present in wastewater, such as Ag, Fe, Mn, Mo, B, Ca, Sb, and Co, must be completely eliminated (Qasem et al., 2021).
TABLE 1. Typical heavy metals present in wastewater (WHO, 2017; Qasem et al., 2021).
Heavy metals must be treated due to their non-biodegradable properties (Fu and Wang, 2011). Trace heavy metals can be toxic through the process of biomagnification, which can increase their concentration to a point where they become toxic (Khan et al., 2008). Several processes are used to remove heavy metals, including chemical precipitation and electrochemical treatment, but these are not effective for concentrated ions and produce excessive amounts of sludge. Ion selectivity is high in ion exchange treatment, but the cost of the resins is too high (Bashir et al., 2019). Adsorption uses either inorganic adsorbents—natural minerals, ores, clays and industrial solid waste such as bauxite red mud, slag, ash, water treatment sludge (alum), and seawater-neutralized red mud—or organic adsorbents—waste organic matter from plants or animals (Khan et al., 2008; Zhu et al., 2019). Sulfate-reducing bacteria (SRB) are used to biologically remove heavy metals, producing metal sulfide precipitates on a large scale (Perales-Vela et al., 2006). This treatment has weaknesses such as long residence times and the need for continuous feeding substrates and larger bioreactors (White et al., 1997; Perales-Vela et al., 2006). Moreover, microalgae are limited and do not purify effluent (Monteiro et al., 2012).
It has become imperative to look for new technologies to remove heavy metals, such as membrane technology. The right technology needs to be scalable, applicable to field conditions, economical, and capable of eliminating heavy metal concentrations to the established standard (Sheng et al., 2004). Membrane technologies are therefore of immediate interest for the quality of treated water. Table 2 below summarizes the strengths and weaknesses of biological, chemical precipitation, electrochemical, and pressure filtration processes.
TABLE 2. Strengths and weaknesses of biological and pressure filtration processes (Wang and Chen, 2009; Chen et al., 2018; Perpetuo et al., 2011; Monteiro et al., 2012; Bashir et al., 2019).
Many studies have reviewed the application of reverse osmosis (RO) membranes. For example, Abdullah et al. (2019) conducted a literature review of the use of membrane technologies for heavy metal removal. They discussed the performance and capabilities of different membrane processes and their advantages and disadvantages. Guo et al. (2022) studied the scale inhibition mechanism and modification strategy, which influences the biofouling of RO membranes. Xiang et al. (2022) reviewed the latest developments, discoveries, and prospective applications related to ultrafiltration (UF), nanofiltration, reverse osmosis, and electrodialysis, with an in-depth focus on heavy metal removal. They presented perspectives on opportunities and challenges in the field of membrane filtration. Saleh et al. (2022) evaluated removal processes by chemical precipitation, photocatalysis, flotation, ion exchange, remediation, electrochemical treatment, adsorption, membrane technologies, and coagulation/flocculation. Qasem et al. (2021) exhaustively and critically reviewed and discussed methods in terms of the agents/adsorbents used, metal ion removal efficiency, operating conditions, and the advantages and disadvantages of each method. In this study, we focus specifically on the different materials and specific methods for synthesizing RO membranes, as well as different wastewater treatment methods. The information available in this review will allow researchers to understand the available work and then consider the synthesis of new innovative RO membranes. It provides researchers with comprehensive data on metal and metalloid discharges and describes the performance of different membranes in terms of permeate flux and methods of heavy metal removal from wastewater, as well as the necessary information on chemical membrane cleaning and end-of-life membrane management.
This review also addresses the technical challenges of the existing membrane process and recommends future research for further improving membrane performance to make it the best alternative for treating water laden with heavy metals. This work is not the first on the subject to eliminate heavy metals. A great deal of work has been done to eliminate metal ions from water. For example, Dompé and Ahoulé, (2016) conducted a study on borehole water intended for consumption that was contaminated with arsenic. The removal of As (V) by reverse osmosis membrane (TW30) was 97.6% effective. A synthetic tannery effluent was first treated with commercial reverse osmosis membranes (BW30 and SW30). Next, a CS membrane on a polyethersulfone (PES) support (cs-PES MFO22) was prepared. The cs-PES MFO22 membrane was very effective at removing >99% of chromium. Removal of Cr, Ca, Mg, K, and Na on a BW 30 membrane ranged from 80%–90%, 85%–98%, 80%–97%, 60%–80%, and 60%–80%, respectively, and, on a SW30 membrane, 80%–98%, 80%–98%, 80%–98%, 78%–96%, and 78%–96%, respectively (Zakmout et al., 2020). Conidi et al. (2018) conducted a study to reduce the salinity of flue gas desulphurization (FGD) wastewater. After softening with Na2CO3 H2O and ultrafiltration, the wastewater was filtered through two commercial thin-film composite polyamide RO membranes (SWC-2540 and ESPA-2540 from Hydranautics). Experimental results indicated that the SWC-2540 membrane performed better in rejecting ions: Mg2+ ions were completely rejected, while the rejection of monovalent ions such as Na+ was approximately 95.5%. The ESPA-2540 membrane showed rejection of Ca2+ and Mg2+ higher than 86.5%, whilst the observed rejection of Na+ was 80%. For the SWC-2540 membrane, an increased rejection of Ca2+ and Na+ ions was observed by increasing the operating pressure in the range of 16–50 bar. Mg2+ ions were totally rejected independently by the operating pressure. MIL-101 (Cr) nanoparticles were doped into the dense layer of selective polyamide (PA) on the polysulfone (PS) ultrafiltration support to prepare a thin-film nanocomposite membrane for water desalination. sodium chloride (NaCl) removal on TFN-MIL-101 RO was greater than 99% (Xu et al., 2016).
Membrane separation technology is at the cutting edge of water purification (Friess et al., 2021). Inorganic, organic, and pharmaceutical compounds and salts dissolved in water are easily removed by reverse osmosis (Foureaux et al., 2019), making it a key technology for alleviating the water crisis (Shannon et al., 2008; Greenlee et al., 2009; Elimelech and Phillip, 2011; Chuang and Dudeney, 2018). Reverse osmosis is more effective at eliminating micropollutants and purifying effluent and is easy to scale-up. Cellulose acetate (CA) and thin-film composite (TFC) membranes are attracting industry interest, and the TFC membrane is attractive for its ability to reject solutes. CAs and aromatic polyamides (APs) are the active polymer derivatives used for RO membrane coatings (Sagle and Freeman, 2004; Ahmed et al., 2015; Makisha, 2019; Alanood et al., 2021).
These membranes have some advantages and disadvantages; CA membrane has high pH sensitivity, lower tolerance to high temperature, and it is more resistant to chlorine than TFC membrane. However, TFC membranes are much more efficient and effective than CA membranes. Interfacial polymerization, composite coating, and multilayer composite molding are the procedures commonly used for membrane preparation (Jackson and Hillmyer, 2010; Lalia et al., 2013; Ahmed et al., 2015; Sandu et al., 2022). TFC is the principal membrane used in the RO process and is characterized by a very thin active layer of PA that is formed on a porous substrate (Ismail et al., 2015; Xu et al., 2016). The thickness of the thin film is <0.2 μm with an interstitial void size ≤0.5 nm between the polymer chains (Maruf et al., 2012; Ismail et al., 2015; Gan et al., 2020; Zhang et al., 2022). The main determinant of the selectivity and water flux of the polyamide membrane is the selective layer formed by the interfacial polymerization reaction of aqueous amine and acyl chloride (Porter, 1989; Chuang and Huang, 2018).
The first research on RO dates back to the 1970s. For example, Kremen et al. (1977) demonstrated the possibility of purifying wastewater of various metal ions with an integrated process containing RO and precipitation units (Algieri et al., 2021). Zn2+ and Cu2+ ions were removed by a low-pressure RO process in the presence of a chelating agent (EDTA) (Ujang and Anderson, 2000). They found that permeate flux varied as a function of pressure, EDTA concentration, and temperature. The evolution of RO performance was demonstrated for Cu2+ and Cd2+ removal (Qdais and Moussa, 2004). The experiments were performed with polyamide membranes characterized by a spirally wound configuration (Algieri et al., 2021). Harharah et al. (2022) showed that permeate flux and Cu (II) removal were directly proportional to operating pressure and feed temperature. Pressure increased from 10 to 40 bars and removal increased from 89.98% to 94.21% on the Dow Polyamide TFC BW30XFR membrane. Pretreatment of real industrial wastewater by electrocoagulation, followed by reverse osmosis, revealed 99.89% removal of Cr3+ ions (Rasha et al., 2020). Coagulation/flocculation pretreatment of industrial and mining wastewater was followed by reverse osmosis on polyamide membrane (DOW™ FILMTEC™ BW30-440i); turbidity, total dissolved solid (TDS) concentrations, antimony, arsenic, nickel, zinc, and iron were reduced by 85%, 96%, 95%, 66%, 82%, 48%, and 10%, respectively, in the permeate (Samaei et al., 2020). Agboolo et al. (2021) have shown that TFC membranes can maintain selectivity only at low water permeance between 1–20 Lm−2 h−1 bar−1.
Membranes mixed/coated with various emerging nanomaterials such as carbon nanotubes (Farahbakhsh et al., 2019), graphene oxides (Chu et al., 2017; Chu et al., 2017), MXenes (Ding et al., 2017; Han et al., 2017), and metal–organic frameworks (MOFs) (Denny et al., 2016; Basu and Balakrishnan, 2017) have been synthesized. These have attracted interest from the water industry due to their outstanding characteristics, including selectivity, high tunable porosities and large accessible surface areas (Lu et al., 2014), enhanced hydrophilicity and resistance to fouling, high ability to easily combine particular species/features without changing the topology of the structure (Evans et al., 2014), and diverse potential applications (Furukawa et al., 2013).
Zeolite imidazolate frameworks (ZIF-8) were specifically selected because they are porous crystalline materials consisting of well-ordered pores (Pan et al., 2011). They have a high specific surface area, and their high thermal, chemical, and hydrothermal stability (Bhattacharjee et al., 2014; Şahin et al., 2017) and durable synthesis at room temperature have attracted the attention of scientists (Abbasi et al., 2020; Li et al., 2021). The unique structure of ZIF-8 resists chemical and thermal attack (Zhang et al., 2012; Wang et al., 2019)
Mixed-matrix membranes (MMMs) combine the advantages of organic membranes and inorganic materials, increasing permeability and reducing fouling (Zheng et al., 2017; Bi et al., 2018; Jeon et al., 2018). Castro-Muňoz et al. (2021) showed that metal ions are removed by mixed-matrix membranes.
However, chitosan (CS) has a hydrophobic character, is an important renewable natural biomass, and is one of the cheapest natural polysaccharides with abundant groups (Agnihotri et al., 2004; LogithKumar et al., 2016; Yang et al., 2016; Liu et al., 2019). Its properties of antibacterial activity, biocompatibility, non-toxicity, and good film-forming have made it advantageous for use as a selective coating (Kumar and Ioan, 2016; Reza et al., 2019; Pishnamazi et al., 2020). CS is known to be an excellent metal ligand, forming stable complexes with many metal ions (Chui et al., 1996). Due to the presence of hydroxyl and amine groups, CS is widely used to remove HM ions from aqueous solutions (Bozorgi et al., 2018; Haripriyan et al., 2022). However, the poor mechanical properties of CS limit its potential (Upadhyay et al., 2021).
Other materials used for heavy metal rejection, such as incorporating UiO-66-NH2 into PAN/chitosan nanofibers, have removed Pb (II), Cd (II), and Cr (VI) ions by 94%, 89%, and 85.5%, respectively (Jamshidifard et al., 2019). UiO-66-NH2 nanoparticle incorporated into the polyvinylidene fluoride (PVDF)/CS nanofiber membrane showed 95.6% rejection of Cr (VI) (Pishnamazi et al., 2020). The incorporation of graphitic carbon nitride nanosheets (g-C3N4) into PSF membranes showed rejections of 95%, 80%, and 70% for lead, cadmium, and arsenic, respectively (Akshatha et al., 2021). The mixed-metal nanoparticle Al–Ti2O6 has been used in the polysulfone membrane to remove heavy metals. Al–Ti2O6 was prepared by the precipitation method, and the membranes were prepared by diffusion-induced phase separation method with different Al–Ti2O6 compositions. The membrane showed a rejection of approximately 96% for As, 98% for Cd, and 99% for Pb (Sunil et al., 2018). The Al–Ti2O6 mixed-metal nanoparticle increased metal ion removal in contrast to the other nanoparticles cited above. Thin-film nanocomposite membranes were prepared by the interfacial polymerization (IP) between PIP and trimesoyl chloride, followed by post-treatment with polyethyleneimine (PEI) or PEI-polyethylene glycol conjugate and then the immobilization of Ag NP. The IP was conducted on a polyethersulfone/poly (methyl methacrylate)-co-poly (vinyl pyrollidone)/silver nanoparticle (Ag NP) blend ultrafiltration membrane support. The TFNC membranes exhibited >99% rejection of Pb2+, 91%–97% rejection of Cd2+, 90%–96% rejection of Co2+, and 95%–99% rejection of Cu2+ with permeate flux ∼40 Lm−2 h−1 at applied pressure 0.5 MPa (Bera et al., 2018).
Table 3 briefly explains the types of membranes and operating techniques applied in RO to remove heavy metals from wastewater.
It should be noted that the membrane operating techniques were conducted under differing laboratory conditions. However, Table 3 describes the variation in solute removal and permeability of the different membranes, depending mainly on the membrane synthesis and pore size, structure, and intensity. These results indicate that almost all PA TFC membranes succeeded in giving high rejection values of between 98%–100%, respectively. It should be noted that some PA TFC membranes such as BW30XFR, TW-30, and AD-SWRO showed rejection of B3+, Cu2+, and Cr6+ ions < 95% and were dependent on the feed concentration.
RO membrane performance is mainly determined by the different types of materials used in its manufacture. Lee et al. (2012) reported that RO membrane is typically designed on a porous PSF support on which an ultra-layer, a thin barrier layer, is deposited on the top surface. They suggest that the emergence of nanomaterials could offer an interesting alternative to polymeric materials.
The emergence of nanotechnology to improve TFC RO membranes has been applied by several researchers. Cohen-Tanugi and Grossman et al. (2015) conducted theoretical research on the addition of graphene in reverse osmosis membrane. They reported that the performance of RO membranes was improved by the addition of graphene, which exhibits pore size uniformity of 0.40 ± 0.24 nm diameter and high permeability up to 3 L/m2.h.bar. Chen et al. (2023) proposed improving the performance of TFC RO membranes by using a hydrophilic polycarboxylic acid–ZIF-8 hydrophobic nanoparticle bilayer interlayer on the PSF substrate prepared by the catechol autopolymerization reaction. The membrane exhibited 98.8% NaCl removal performance and the permeability was 4.2 L/m2h.bar. Guan et al. (2023) prepared a polyketone membrane as a porous substrate using a standard NIPS procedure and another membrane on the PSF porous support, and proposed using MPD—the monomer for IP formation of the PA layer. The membranes showed NaCl rejection from 96.6% to 98.6% in a 2000 ppm feed solution, with permeability varying, respectively, from 0.23 ± 0.13 L m−2 h−1 bar−1 to 0.18 ± 0.06 L m−2 h−1 bar−1. The polyethersulfone material was modified by three hydrophilic molecules—thioglycolic acid, DL-cysteine hydrochloride, and 2-(dimethylamino) ethanethiol hydrochloride—to form a series of new hydrophilic polyethersulfone copolymers (HPES-TGA, HPES-CYSAH, and HPES-DMAET) that offer the best performance in terms of water permeability and water/salt selectivity (Zhang et al., 2023).
The addition of nanomaterials and modification of the RO membrane synthesis provide high performance, but a number of challenges remain in terms of the economics of industrial-scale application. The incorporation of nanoparticles or nanomaterials improved membrane performance, and this comparison was made between mixed-matrix membranes and TFC PA.
Numerous investigations have been made into the performance of the RO membranes in terms of pressure, flow rate (Dimitriou et al., 2017), concentration, and temperature (Ruiz-Gracía et al., 2020).
Luis (2018) has shown that permeate flux is one of the most important factors in determining membrane performance, reflecting permeate quantity and species selectivity. The data from the other studies focused on permeate flux and solute release. The study by Armstrong et al. (2022) on the SWC4 seawater membrane and an ESPA3 brackish water membrane showed that membrane performance is evaluated on water-solute selectivity depending on the pressure applied during permeation tests. Qi et al. (2016) also found that water permeability and solute rejection are sensitive to temperature and applied pressure.
However, the introduction of graphitic carbon nitride (g-C3N4) nanosheets into PSF membranes improved the permeability and separation performance of PSF membranes at low transmembrane pressures ranging from 1 to 5 bar (Nadig et al., 2021).
The performance of the polysulfone composite membrane was improved by the addition of Al–Ti2O6 nanoparticles to remove heavy metal ions at low pressures of 200 kPa (Sunil et al., 2018).
It has been observed that the addition of nanomaterials to membranes enhances performance in terms of selectivity and water permeability at low operating pressures.
Many studies have demonstrated that the highwater flux is linked to better roughness (Al-Jeshi and Neville, 2006; Kong et al., 2010; Ma et al., 2018; Song et al., 2019a; Song et al., 2019b; Ma et al., 2019; Yan et al., 2019; Wu et al., 2020).
The selectivity of TFC membranes is excellent, but there is a trade-off with permeability. However, MMMs offer advantages such as low manufacturing costs, exceptional selectivity, and high packing density of polymeric materials with the long-term stability, high mechanical strength, and regenerative capacity of ceramic materials (Karan et al., 2015; Ayaz et al., 2019; Rezakazemi et al., 2019; Rozaini et al., 2019; Yang et al., 2019; Zhu et al., 2019). Table 3 also includes the performance of several RO membranes studied in the literature for metals removal.
Pore clogging or solute adsorption on the membrane surface adversely affects membrane fouling (Van der Bruggen et al., 2003; Lee et al., 2011; Malaeb and Ayoub, 2011). Pretreatment is recommended to prolong the lifetime of the membrane. Ultrafiltration or microfiltration (MF), scaling control such as softening, and acidification for pH regulation constitute the pretreatments currently considered (Abba et al., 2023). Either microbial growth (Speth et al., 2000; Abba et al., 2023), scaling to organics, or particle matter can form cakes (Gabelich et al., 2005), and the membrane becomes vulnerable to fouling. Membrane fouling can be reduced to the best possible roughness and high hydrophilicity (Vrijenhoek et al., 2001; Van der Bruggen et al., 2003; Mondal and wickramasinghe, 2008).
The underpressure filtration process is constrained by the phenomenon of concentration polarization, which affects its efficiency (Koseoglu et al., 2018). During operation, the solute concentration on the membrane surface increases due to selective transport across the membrane (Luis, 2018). In the case of pressurized membrane processes, the solute is generally retained by the membrane, leading to a concentration profile similar to that shown in Figure 1A. During filtration, the membrane retains the solute, leading to a concentration profile similar to Figure 1B as the component permeates more rapidly through the membrane, being in the boundary layer where the transport is limited by diffusion (Luis, 2018). This phenomenon is similar to capacitive deionization in that it removes ions from the feed concentration. However, when pressure is applied across an ion permselective membrane, the ICP electrokinetic phenomenon occurs. This method is based on ion depletion and enrichment, which are dynamic changes in ion concentration near the membrane to maintain electroneutrality (Rabiee et al., 2019).
FIGURE 1. Concentration representation with concentration polarization: (A) mass transfer limited by the membrane and (B) mass transfer limited to the membrane surface (Luis, 2018). Transmembrane flux decreases as a result of concentration polarization, low retention of low molecular weight solutes, and can lead to higher retention for mixtures of macromolecular solutes.
The pressurized membrane separation process is limited by the problem of fouling, which is unavoidable and reduces the performance of the process. The presence of inorganic and organic compounds in the water affects membrane fouling. Approximately 90% of suspended solids were eliminated in UF by Petrinic et al. (2015). To achieve this, Huang et al. suggested the need for a more advanced pretreatment process to avoid membrane fouling. RO membrane operation at low pressure with constant feed rate spares the membrane from vulnerability to fouling (Park and Kwon, 2018). Research by Lumami et al. (2022) has shown that changes in permeate flux depend on changes in pressure and affect membrane fouling.
Singhidi et al. (2021) reported that increased temperature and high pressure applied to the membrane alters the pore size, which leads to fouling. Li and Chen, (2010) cited membrane properties, feedwater quality, and ionic condition as the three factors influencing membrane fouling. Other parameters cited in the literature review include pressure, temperature, flow rate, and concentration—all of which influence membrane fouling.
Fouling slows down wastewater treatment using membrane processes (Ang et al., 2011), leading to a drop in productivity. Membrane cleaning is extremely important for removing undesirable matter from the membrane surface and renewing its functionalization (Wilson et al., 2022). In the literature, very little research seems devoted to membrane cleaning (Porcelli and Judd, 2010).
Qi et al. (2016) showed that a solution of ethylenediaminetetraacetic acid (EDTA), sodium hydroxide (NaOH), and citric acid was better at cleaning the RO membrane and had good chemical stability. The chemical stability of the membrane was further confirmed by cleaning with a solution of citric acid, NaOH, and EDTA (Wilson et al., 2022). Ang et al. (2011) proposed that the addition of sodium chloride to solution sodium hydroxide (NaOH), ethylenediaminetetraacetic acid (EDTA), and sodium dodecyl sulfate (SDS) was ideal for cleaning membranes resulting from the treatment of alkaline solutions, metal chelating agents, surfactants, and salt. They concluded that cleaning performance increased with an increasing pH of the cleaning solution.
Reverse osmosis retentate can have a number of environmental impacts. However, rational management and reuse of retentate is recommended. Heavy metals are not generally recycled, but recycling by sector is recommended. The best organized channels are obviously those where the largest masses are treated in order to recover valuable compounds. This study proposes two retentate treatment options. The first is recycling for the use of heavy metals, which requires additional investment in additional treatment by a metallize electrolysis reactor. Metals can be recovered by a reactor that uses the principle of electrolysis to cause charged particles, such as heavy metals, to agglomerate on the cathode. The metal agglomerate can then be recovered and recycled (Lenntech). The second option considers the circular economy of the process. For example, the use of retentate as mixing water to produce calcio-sulfoaluminate cement bricks for construction is recommended (Valdés et al., 2021).
The lifetime of the RO membrane depends on factors such as the RO membrane model, the type of pretreatment of the plant, the quality of the feedwater, the operating conditions, the location of the RO membrane, and the frequency of chemical cleaning (Kharraz et al., 2021). In view of the above, the average life has been estimated at between 5 and 10 years for pretreated feedwater (Kharraz et al., 2021); however, it is shorter at approximately 12 months when the feed solution is too loaded and if there is no pretreatment (Chang, 2006). At the end of RO membrane life, reuse in MF and NF is recommended by the literature. Khoo et al. (2021) converted RO membranes to end-of-life by modifying their microfiltration properties with KMnO4 treatment and showed that the end-of-life of RO membranes should considerably increase in coming years. The membrane converted to MF had a NaCl rejection of approximately 80%, and the permeability to pure water was 172.6 L/m.h.bar. In addition, RO has been cited as the process that generates environmental impacts following the production of solid waste (Luisa et al., 2006).
Senán-Salinas et al. (2021) have estimated that, by 2025, two million membrane modules will be discarded worldwide by the desalination sector in developed countries. Kharraz et al. (2021) revealed that membranes consist of plastic parts and that their recycling will reduce landfill disposal.
In addition, these membranes are made from petroleum-based polymers and are responsible for greenhouse gas emissions (Senán-Salinas et al., 2021). Rattanakul (2012) has shown that RO membranes contain 80% recycled components. However, plastics such as polyethylene terephthalate (PET), high-density polyethylene (HDPE), and polypropylene (PP) are recyclable and represent a step forward in reducing harmful impacts on the environment.
The RO membrane process is effective at removing organic and inorganic compounds from wastewater and in the simplicity of its scale-up. Removal of inorganic micropollutants varies between 95%–100%, depending on the type of polymer, materials, and operating conditions applied. However, the temperature rise applied in RO affects the membrane’s vulnerability to fouling.
The application of CS and ZIF-8 in the process improved selectivity and water permeability. It was observed that metal oxides also perform best in terms of selectivity and permeability. The emergence of nanomaterials in the membrane process has improved operation at very low pressure, as in the case of nanofiltration, with low operating and maintenance costs.
VK: conceptualization, methodology, validation, visualization, and writing–original draft. PL: conceptualization, funding acquisition, methodology, project administration, supervision, validation, and writing–review and editing.
The author(s) declare that no financial support was received for the research, authorship, and/or publication of this article.
The authors acknowledge the Department of Materials and Process Engineering (IMAP) at UCLouvain for their support. The authors would like to thank the Academy for Research and Higher Education (ARES) of the Wallonia-Brussels Federation for its financial support for the publication of this article.
The authors declare that the research was conducted in the absence of any commercial or financial relationships that could be construed as a potential conflict of interest.
All claims expressed in this article are solely those of the authors and do not necessarily represent those of their affiliated organizations, or those of the publisher, the editors, and the reviewers. Any product that may be evaluated in this article, or claim that may be made by its manufacturer, is not guaranteed or endorsed by the publisher.
BOD5, biological oxygen demand; ICP, inductively coupled plasma; Init. conc., initial concentration; CA, cellulose acetate; CNTs, carbon nanotubes; COD, chemical oxygen demand; CS, chitosan; GO, graphene oxide; HM, heavy metal; LPRO, low-pressure reverse osmosis; MF, microfiltration; MMMs, mixed-matrix membranes; Na2EDTA, disodium salt of ethylenediaminetetraacetic acid; NA, not available; NF, nanofiltration; ND, no data; PA, polyamide; PAA, polyacrylic acid; RO, reverse osmosis; SRB, sulfate-reducing bacteria; SDS, sodium dodecyl sulfate; SWRO, seawater reverse osmosis; TFC, thin-film composite; TDS, total dissolved solids; UF, ultrafiltration; WHO, World Health Organization; WW, wastewater.
Abba, S. I., Benaafi, M., and Aljundi, I. H. (2023). Intelligent process optimisation based on cutting-edge emotional learning for performance evaluation of NF/RO of seawater desalination plant. Desalination 550, 116376. ISSN 0011-9164. doi:10.1016/j.desal.2023.116376
Abbasi, Z., Cseri, L., Zhang, X., Ladewig, B. P., and Wang, H. (2020). “Metal-Organic Frameworks (MOFs) and MOF-derived porous carbon materials for sustainable adsorptive wastewater treatment,” in Sustainable nanoscale engineering: from materials design to chemical processing. Editors G. Szekely, and A Livingston. 1st ed. (Elsevier), 163–194. doi:10.1016/B978-0-12-814681-1.00007-2
Abdullah, N., Yusof, N., Lau, W. J., Jaafar, J., and Ismail, A. F. (2019). Recent trends of heavy metal removal from water/wastewater by membrane technologies. J. Industrial Eng. Chem. 76, 17–38. ISSN 1226-086X. doi:10.1016/j.jiec.2019.03.029
Agboolo, O., Fayomi, O. S. I., Ayodeji, A., Ayeni, A. O., Alagbe, E. E., Sanni, S. E., et al. (2021). A review on polymer nanocomposites and their effective applications in membranes and adsorbents for water treatment and gas separation. Membranes 11 (2), 139. doi:10.3390/membranes11020139
Agnihotri, S. A., Mallikarjuna, N. N., and Aminabhavi, T. M. (2004). Recent advances on chitosan-based micro-and nanoparticles in drug delivery. J. Control. Release 100, 5–28. doi:10.1016/j.jconrel.2004.08.010
Ahmed, F. E., Lalia, B. S., and Hashaikeh, R. (2015). A review on electrospinning for membrane fabrication: challenges and applications. Dessalement 356, 15–30. doi:10.1016/j.desal.2014.09.033
Akshatha, R. N., Naik, N. S., Padaki, M., Pai, R. K., and Déon, S. (2021). Impact of graphitic carbon nitride nanosheets in mixed-matrix membranes for removal of heavy metals from water. J. Water Process Eng. 41, 102026, 102026. ISSN 2214-7144. doi:10.1016/j.jwpe.2021.102026
Al-Alawy, A. F., and Salih, M. H. (2017). Comparative study between nanofiltration and reverse osmosis membranes for the removal of heavy metals from electroplating wastewater. Eng. J. 23, 1–21. doi:10.31026/j.eng.2017.04.01
Alanood, A. A., Al-Obaidi, M. A., Farag, S. K., Patel, R., and Mujtaba, I. M. (2021). Performance evaluation of a medium-scale industrial reverse osmosis brackish water desalination plant with different brands of membranes. A Simul. study, Desalination 503, 0011–9164. doi:10.1016/j.desal.2020.114927
Algieri, C., Chakraborty, S., and Candamano, S. (2021). A way to membrane-based environmental remediation for heavy metal removal. Environments 8 (6), 52. doi:10.3390/environments8060052
Algureiri, A. H., and Abdulmajeed, Y. R. (2016). Removal of heavy metals from industrial wastewater by using RO membrane. Iraqi J. Chem. Pet. Eng. 17, 125–136. doi:10.31699/ijcpe.2016.4.12
Al-Jeshi, S., and Neville, A. (2006). An investigation into the relationship between flux and roughness on RO membranes using scanning probe microscopy. Desalination 189, 221–228. ISSN 0011-9164. doi:10.1016/j.desal.2005.08.001
Ang, W. S., Yip, N. Y., and Tiraferri, A. (2011). Chemical cleaning of RO membranes fouled by wastewater effluent: achieving higher efficiency with dual-step cleaning. J. Membr. Sci. 382 (1–2), 100–106. ISSN 0376-7388. doi:10.1016/j.memsci.2011.07.047
Armstrong, M. D., Vickers, R., and Coronell, O. (2022). Trends and errors in reverse osmosis membrane performance calculations stemming from test pressure and simplifying assumptions about concentration polarization and solute rejection. J. Membr. Sci. 660 (2022), 120856. ISSN 0376-7388. doi:10.1016/j.memsci.2022.120856
Atès, N., and Uzal, N. (2018). Removal of heavy metals from aluminum anodic oxidation wastewaters by membrane filtration. Environ. Sci. Pollut. Res. Int. 25 (22), 22259–22272. doi:10.1007/s11356-018-2345-z
Ayaz, M., Muhammad, A., Younas, M., Khan, A. L., and Rezakazemi, M. (2019). Enhanced water flux by fabrication of polysulfone/alumina nanocomposite membrane for copper (II) removal. Remov. Macromol. Res. 27, 565–571. doi:10.1007/s13233-019-7086-4
Bashir, A., Malik, L. A., Ahad, S., Manzoor, T., Bhat, M. A., Dar, G. N., et al. (2019). Removal of heavy metal ions from aqueous system by ion-exchange and biosorption methods. Environ. Chem. Lett. 17, 729–754. doi:10.1007/s10311-018-00828-y
Basu, S., and Balakrishnan, M. (2017). Polyamide thin film composite membranes containing ZIF-8 for the separation of pharmaceutical compounds from aqueous streams. Sep. Purif. Technol. 179, 118–125. doi:10.1016/j.seppur.2017.01.061
Bera, A., Trivedi, J. S., Kumar, S. B., Chandel, A. K. S., Haldar, S., and Jewrajka, S. K. (2018). Anti-organic fouling and anti-biofouling poly(piperazineamide) thin film nanocomposite membranes for low pressure removal of heavy metal ions. J. Hazard. Mater. 343, 86–97. ISSN 0304-3894. doi:10.1016/j.jhazmat.2017.09.016
Bhattacharjee, S., Jang, M. S., Kwon, H. J., and Ahn, W. S. (2014). Zeolitic imidazolate frameworks: synthesis, functionalization, and catalytic/adsorption applications. Catal. Surv. Asia 18, 101–127. doi:10.1007/s10563-014-9169-8
Bi, R., Zhang, Q., Zhang, R., Su, Y., and Jiang, Z. (2018). Thin film nanocomposite membranes incorporated with graphene quantum dots for high flux and antifouling property. J. Membr. Sci. 553, 17–24. doi:10.1016/j.memsci.2018.02.010
Bozorgi, M., Abbasizadeh, S., Samani, F., and Mousavi, S. E. (2018). Performance of synthesized cast and electrospun PVA/chitosan/ZnO-NH2 nano-adsorbents in single and simultaneous adsorption of cadmium and nickel ions from wastewater. Environ. Sci. Pollut. Res. 25, 17457–17472. doi:10.1007/s11356-018-1936-z
Castro-Muňoz, R., Gonzalez-Melgoza, L. L., and García-Depraect, O. (2021). Ongoing progress on novel nanocomposite membranes for the separation of heavy metals from contaminated water. Chemosphere 270, 129421. ISSN 0045 - 6535. doi:10.1016/j.chemosphere.2020.129421
Chan, B. K. C., and Dudeney, A. W. L. (2008). Reverse osmosis removal of arsenic residues from bioleaching of refractory gold concentrates. Min. Eng. 21, 272–278. ISSN 0892-6875. doi:10.1016/j.mineng.2007.10.003
Chang, y. (2006). Life expectancy of POU membrane elements. Water Qual. Prod. https://www.wqpmag.com/life-expectancy-pou-membrane-elements.
Chen, K., Zhao, S., Tian, B., Li, F., Miao, C., Teng, D., et al. (2023). Hydrophilic-hydrophobic bilayer interlayer for high performance thin-film composite reverse osmosis membranes. J. Membr. Sci. 689, 122177. ISSN 0376-7388. doi:10.1016/j.memsci.2023.122177
Chen, Q., Yao, Y., Li, X., Lu, J., Zhou, J., and Huang, Z. (2018). Comparison of heavy metal removals from aqueous solutions by chemical precipitation and characteristics of precipitates. J. Water Process. Eng. 26, 289–300. doi:10.1016/j.jwpe.2018.11.003
Chu, K. H., Fathizadeh, M., Yu, M., Flora, J. R. V., Jang, A., Jang, M., et al. (2017). Evaluation of removal mechanisms in a graphene oxide-coated ceramic ultrafiltration membrane for retention of natural organic matter, pharmaceuticals, and inorganic salts. ACS Appl. Mat. Interfaces 9, 40369–40377. doi:10.1021/acsami.7b14217
Chu, K. H., Fathizadeh, M., Yu, M., Flora, J. R. V., Jang, A., Jang, M., et al. (2017). Evaluation of graphene oxide-coated ultrafiltration membranes for humic acid removal at different pH and conductivity conditions. Sep. Purif. Technol. 181, 139–147. ISSN 1383-5866. doi:10.1016/j.seppur.2017.03.026
Chuang, S. P., and Huang, S. J. (2018). The effect of environmental corporate social responsibility on environmental performance and business competitiveness: the mediation of green information technology capital. J. Bus. Ethics 150, 991–1009. doi:10.1007/s10551-016-3167-x
Chui, V. W. D., Mok, K. W., Ng, C. Y., Luong, B. P., and Ma, K. K. (1996). Removal and recovery of copper (II) chromium (III), and nickel (II) from solutions using crude shrimp chitin packed insmall columns. Environ. Int. 22 (4), 463–468. doi:10.1016/0160-4120(96)00034-7
Çimen, A., Kılıçel, F., and Arslan, G. (2014). Removal of chromium ions from waste waters using reverse osmosis AG and SWHR membranes. Russ. J. Phys. Chem. 88, 845–850. doi:10.1134/S0036024414050045
Cingolani, D., Fatone, F., Frison, N., Spinelli, M., and Eusebi, A. L. (2018). Pilot-scale multi-stage reverse osmosis (DT-RO) for water recovery from landfill leachate. Waste Manag. 76, 566–574. ISSN 0956-053X. doi:10.1016/j.wasman.2018.03.014
Cohen-Tanugi, D., and Grossman, J. C. (2015). Nanoporous graphene as a reverse osmosis membrane: recent insights from theory and simulation. Desalination 366, 59–70. ISSN 0011-9164. doi:10.1016/j.desal.2014.12.046
Conidi, C., Macedonio, F., Argurio, P., Cassano, A., and Drioli, E. (2018). Performance of reverse osmosis membranes in the treatment of flue-gas desulfurization (FGD) wastewaters. Environments 5, 71. doi:10.3390/environments5060071
Dang, T. T. H., Li, C. W., and Choo, K. H. (2016). Comparison of low-pressure reverse osmosis filtration and polyelectrolyte-enhanced ultrafiltration for the removal of Co and Sr from nuclear plant wastewater. Sep. Purif. Technol. 157, 209–214. ISSN 1383-5866. doi:10.1016/j.seppur.2015.11.019
Demiral, İ., Samdan, C., and Demiral, H. (2021). Enrichment of the surface functional groups of activated carbon by modification method. Surf. Interfaces 22, 100873, 100873. ISSN 2468-0230. doi:10.1016/j.surfin.2020.100873
Denny, M. S., Moreton, J. C., Benz, L., and Cohen, S. M. (2016). Metal-organic frameworks for membrane-based separations. Nat. Rev. Mater 1, 16078. doi:10.1038/natrevmats.2016.78
Dimitriou, E., Boutikos, P., Mohamed, E. S., Koziel, S., and Papadakis, G. (2017). Theoretical performance prediction of a reverse osmosis desalination membrane element under variable operating conditions. Desalination 419, 70–78. ISSN 0011-9164. doi:10.1016/j.desal.2017.06.001
Ding, L., Wei, Y. Y., Wang, Y. J., Chen, H. B., Caro, J., and Wang, H. H. (2017). A two-dimensional lamellar membrane: MXene nanosheet stacks. Angew. Chem. Int. Ed. 56, 1825–1829. doi:10.1002/anie.201609306
Dompé, G., and Ahoulé, M. (2016). Comparative performance of nanofiltration and reverse osmosis for arsenic contaminated water treatment in Burkina Faso. Process engineering. University of Montpellier; International Institute of Water and Environmental Engineering. French. ⟨NNT: 2016MONTS025⟩.
Duan, C., Ma, T., Wang, J., and Zhou, Y. (2020). Removal of heavy metals from aqueous solution using carbon-based adsorbents: a review. J. Water Process Eng. 37, 101339. ISSN 2214-7144. doi:10.1016/j.jwpe.2020.101339
Dydo, P., Nemś, I., and Turek, M. (2012). Boron removal and its concentration by reverse osmosis in the presence of polyol compounds. Sep. Purif. Technol. 89, 171–180. ISSN 1383-5866. doi:10.1016/j.seppur.2012.01.018
Elimelech, M., and Phillip, W. A. (2011). The future of seawater desalination: energy, technology, and the environment. Science 333, 712–717. doi:10.1126/science.1200488
Evans, J. D., Sumby, C. J., and Doonan, C. J. (2014). Post-synthetic metalation of metal-organic frameworks. Chem. Soc. Rev. 43, 5933–5951. doi:10.1039/C4CS00076E
Farahbakhsh, J., Delnavaz, M., and Vatanpour, V. (2019). Simulation and characterization of novel reverse osmosis membrane prepared by blending polypyrrole coated multiwalled carbon nanotubes for brackish water desalination and antifouling properties using artificial neural networks. J. Memb. Sci. 581, 123–138. doi:10.1016/j.memsci.2019.03.050
Feng, R. W., Zhao, P. P., Zhu, Y. M., Yang, J. G., Wei, X. Q., Yang, L., et al. (2021). Application of inorganic selenium to reduce accumulation and toxicity of heavy metals (metalloids) in plants: the main mechanisms, concerns, and risks. Sci. Total Environ. 771, 144776. doi:10.1016/j.scitotenv.2020.144776
Foureaux, A. F. S., Reis, E. O., Lebron, Y., Moreira, V., Santos, L. V., Amaral, M. S., et al. (2019). Rejection of pharmaceutical compounds from surface water by nanofiltration and reverse osmosis. Sep. Purif. Technol. 212, 171–179. ISSN 1383-5866. doi:10.1016/j.seppur.2018.11.018
Friess, K., Izák, P., Kárászová, M., Pasichnyk, M., Lanč, M., Nikolaeva, D., et al. (2021). A review on ionic liquid gas separation membranes. Membranes 11, 97. doi:10.3390/membranes11020097
Fu, F., and Wang, Q. (2011). Removal of heavy metal ions from wastewaters: a review. J. Environ. Manag. 92, 407–418. doi:10.1016/j.jenvman.2010.11.011
Furukawa, H., Cordova, K. E., O'Keeffe, M., and Yaghi, O. M. (2013). The chemistry and applications of metal-organic frameworks. Sci. (New York, N.Y.) 341 (6149), 1230444. doi:10.1126/science.1230444
Gabelich, C. J., Frankin, J. C., Gerringer, F. W., Ishida, K. P., and Suffet, I. H. (2005). Enhanced oxidation of polyamide membranes using monochloramine and ferrous iron. J. Membr. Sci. 258, 64–70. doi:10.1016/j.memsci.2005.02.034
Gan, B., Qi, S., Song, X., Yang, Z., Tang, C. Y., Cao, X., et al. (2020). Ultrathin polyamide nanofilm with an asymmetrical structure: a novel strategy to boost the permeance of reverse osmosis membranes. J. Membr. Sci. 612, 118402. ISSN 0376-7388. doi:10.1016/j.memsci.2020.118402
Greenlee, L. F., Lawler, D. F., Freeman, B. D., Marrot, B., and Moulin, P. (2009). Reverse osmosis desalination: water sources, technology, and today's challenges. Water Res. 43, 2317–2348. ISSN 0043-1354. doi:10.1016/j.watres.2009.03.010
Guan, K., Fang, S., Zhou, S., Fu, W., Li, Z., Gonzales, R. R., et al. (2023). Thin film composite membrane with improved permeance for reverse osmosis and organic solvent reverse osmosis. J. Membr. Sci. 688, 122104, 122104. ISSN 0376-7388. doi:10.1016/j.memsci.2023.122104
Guo, Y., Liu, C., Liu, H., Zhang, J., Li, H., and Zhang, C. (2022). Contemporary antibiofouling modifications ofreverse osmosis membranes: state-of-the-art insights on mechanisms and strategies. Chem. Eng. J. 429, 132400. ISSN 1385-8947. doi:10.1016/j.cej.2021.132400
Han, R. L., Ma, X. F., Xie, Y. L., Teng, D., and Zhang, S. H. (2017). Preparation of a new 2D M-Xene/PES composite membrane with excellent hydrophilicity and high flux. RSC Adv. 7, 56204–56210. doi:10.1039/C7RA10318B
Harharah, R. H., Abdalla, G. M. T., Elkhaleefa, A., Shigidi, I., and Harharah, H. N. (2022). A study of copper (II) ions removal by reverse osmosis under various operating conditions. Separations 9 (6), 155. doi:10.3390/separations9060155
Haripriyan, U., Gopinath, K. P., and Arun, J. (2022). Chitosan based nano adsorbents and its types for heavy metal removal. doi:10.1016/j.matlet.2022.131670
Herrera-Estrella, L. R., and Guevara-Garcia, A. A. (2001). “Heavy metal adaptation,” in Encyclopedia of life sciences (Hoboken, NJ, USA: John Wiley & Sons, Ltd.). Available at: https://www.lenntech.fr/metaux-lourds-haute-concentration.htm#ixzz8CiDsXJxZ (Accessed online on September 08, 2023). doi:10.1002/9780470015902
Ipek, U. (2005). Removal of Ni(II) and Zn(II) from an aqueous solution by reverse osmosis. Desalination 174, 161–169. ISSN 0011-9164. doi:10.1016/j.desal.2004.09.009
Ismail, A. F., Padaki, M., Hilal, N., Matsuura, T., and Lau, W. J. (2015). Thin film composite membrane — recent development and future potential. Desalination 356, 140–148. ISSN 0011-9164. doi:10.1016/j.desal.2014.10.042
Jackson, E. A., and Hillmyer, M. A. (2010). Nanoporous membranes derived from block copolymers: from drug delivery to water filtration. ACS Nano 4, 3548–3553. doi:10.1021/nn1014006
Jamshidifard, S., Koushkbaghi, S., Hosseini, S., Rezaei, S., Karamipour, A., Jafari, R. A., et al. (2019). Incorporation of UiO-66-NH2 MOF into the PAN/chitosan nanofibers for adsorption and membrane filtration of Pb(II), Cd(II) and Cr(VI) ions from aqueous solutions. J. Hazard. Mater. 368, 10–20. ISSN 0304-3894. doi:10.1016/j.jhazmat.2019.01.024
Jeon, S., Park, C. H., Park, S. H., Shin, M. G., Kim, H. J., Baek, K. Y., et al. (2018). Star polymer-assembled thin film composite membranes with high separation performance and low fouling. J. Membr. Sci. 555, 369–378. ISSN 0376-7388. doi:10.1016/j.memsci.2018.03.075
Jiang, Z., Karan, S., and Livingston, A. G. (2018). Water transport through ultrathin polyamide nanofilms used for reverse osmosis. Adv. Mat. 30, 1705973. doi:10.1002/adma.201705973
Karan, S., Jiang, Z., and Livingston, A. G. (2015). Sub–10 nm polyamide nanofilms with ultrafast solvent transport for molecular separation. Science 348, 1347–1351. doi:10.1126/science.aaa5058
Khan, M. J., Drochner, W., Steingass, H., and Islam, K. M. S. (2008). Nutritive evaluation of some tree leaves from Bangladesh for feeding ruminant animals. Indian J. Anim. Sci. 78, 1273–1277.
Kharraz, E. J., Almajraf, E. A., Alaoufi, A., Hinaai, D. A. A. R., Allison, J., Al-Busaidi, , et al. (2021). Management options for extending the lifecycle of reverse osmosis membranes and their environmental assessment. Towards a sustainable water future. January, 189–200. doi:10.1680/oicwe.65253.189
Khoo, Y. S., Lau, W. J., Hasan, S. W., Salleh, W. N. W., and Ismail, A. F. (2021). New approach of recycling end-of-life reverse osmosis membranes via sonication for microfiltration process. J. Environ. Chem. Eng. 9 (6), 106731. doi:10.1016/j.jece.2021.106731
Kong, C., Kanezashi, M., Yamomoto, T., Shintani, T., and Tsuru, T. (2010). Controlled synthesis of high-performance polyamide membrane with thin dense layer for water desalination. J. Membr. Sci. 362, 76–80. ISSN 0376-7388. doi:10.1016/j.memsci.2010.06.022
Koseoglu, H., Guler, E., Bilgehan, I. H., and Gonulsuz, E. (2018). Water flux and reverse salt flux, Membrane-Based Salin. Gradient Process. Water Treat. Power Generation, 57–86. Sarper Sarp, Nidal Hilal, ISBN 9780444639615. doi:10.1016/B978-0-444-63961-5.00002-X
Kremen, S. S., Hayes, C., and Dubos, M. (1977). Large-scale reverse osmosis processing of metal finishing rinse waters. Desalination 20, 71–80. doi:10.1016/s0011-9164(00)88209-0
Krishna Kumar, A. S., Jiang, S. J., and Tseng, W. L. (2015). Effective adsorption of chromium(vi)/Cr(iii) from aqueous solution using ionic liquid functionalized multiwalled carbon nanotubes as a super sorbent. J. Mat. Chem. A 3, 7044–7057. doi:10.1039/C4TA06948J
Kumar, V. T., and Ioan, S. V. (2016). Recent advances in cellulose and chitosan based membranes for water purification: a concise review. Carbohydr. Polym. 146, 148–165. ISSN 0144-8617. doi:10.1016/j.carbpol.2016.03.030
Lalia, B. S., Kochkodan, V., Hashaikeh, R., and Hilal, N. (2013). A review on membrane fabrication: structure, properties and performance relationship. Dessalement 326, 77–95. doi:10.1016/j.desal.2013.06.016
Lee, K. P., Arnot, T. C., and Mattia, D. (2011). A review of reverse osmosis membrane materials for desalination - development to date and future potential, J. Membr. Sci. 370 1–2, Pages 1–22. ISSN 0376-7388. doi:10.1016/j.memsci.2010.12.036
Li, H., and Chen, V. (2010). Chapter 10 - membrane fouling and cleaning in food and bioprocessing, Z. F. Cui, and H. S. Muralidhara, Membrane technology, Butterworth-Heinemann, 213–254. 9781856176323. doi:10.1016/B978-1-85617-632-3.00010-0
Li, Ke, Miwornunyuie, N., Chen, L., Jingyu, H., Amaniampong, S. P., Koomson, D. A., et al. (2021). Sustainable application of ZIF-8 for heavy-metal removal in aqueous solutions. Sustainability 13 (2), 984. doi:10.3390/su13020984
Li, Y., Xu, Z., Liu, S., Zhang, J., and Yang, X. (2017). Molecular simulation of reverse osmosis for heavy metal ions using functionalized nanoporous graphenes. Comput. Mat. Sci. 139 (65–74), 65–74. ISSN 0927-0256. doi:10.1016/j.commatsci.2017.07.032
Liu, L., Yang, W., Gu, D., Zhao, X., and Pan, Q. (2019). In situ preparation of chitosan/ZIF-8 composite beads for highly efficient removal of U(VI). Front. Chem. 7, 607. ISSN = 2296 - 2646. doi:10.3389/fchem.2019.00607
LogithKumar, R., KeshavNarayan, A., Dhivya, S., Chawla, A., Saravanan, S., and Selvamurugan, N. (2016). A review of chitosan and its derivatives in bone tissue engineering. Polym 151, 172–188. doi:10.1016/j.carbpol.2016.05.049
Lu, W. G., Wei, Z. W., Gu, Z. Y., Liu, T. F., Park, J., Park, J., et al. (2014). Tuning the structure and function of metal-organic frameworks via linker design. Chem. Soc. Rev. 43, 5561–5593. doi:10.1039/c4cs00003j
Luis, P. (2018). Fundamental modeling of membrane systems: membrane and process performance. Elsevier, 1–23. 9780128134832. doi:10.1016/B978-0-12-813483-2.00001-0
Lumami, K. V., Mar, G. A., Sang, S. V., Buleng, N. T. E., Ndikumana, T., Musibono, D.-D., et al. (2022). Evaluation of commercial reverse osmosis and nanofiltration membranes for the removal of heavy metals from surface water in the democratic republic of Congo. Clean. Technol. 4 (4), 1300–1316. doi:10.3390/cleantechnol4040080
Ma, X., Yang, Z., Yao, Z., Guo, H., Xu, Z., and Tang, C. Y. (2019). Tuning roughness features of thin film composite polyamide membranes for simultaneously enhanced permeability, selectivity and anti-fouling performance. J. Colloid Interface Sci. 540, 382–388. ISSN 0021 - 9797. doi:10.1016/j.jcis.2019.01.033
Ma, X.-H., Yao, Z.-K., Yang, Z., Guo, H., Xu, Z.-L., Tang, C. Y., et al. (2018). Nanofoaming of polyamide desalination membranes to tune permeability and selectivity. Environ. Sci. Technol. Lett. 5, 123–130. doi:10.1021/acs.estlett.8b00016
Makisha, N. (2019). Membrane bioreactors for treatment of galvanic wastewater. E3S Web Conf. 97, 05047. doi:10.1051/e3sconf/20199705047
Malaeb, L., and Ayoub, G. M. (2011). Reverse osmosis technology for water treatment: state of the art review. Desalination 267 (1), 1–8. ISSN 0011-9164. doi:10.1016/j.desal.2010.09.001
Marciniak, M., Goscianska, J., Frankowski, M., and Pietrzak, R. (2019). Optimal synthesis of oxidized mesoporous carbons for the adsorption of heavy metal ions. J. Mol. Liq. 276, 630–637. ISSN 0167-7322. doi:10.1016/j.molliq.2018.12.042
Maruf, S. H., Ahn, D. U., Pellegrino, J., Killgore, J. P., Greenberg, A. R., and Ding, Y. (2012). Correlation between barrier layer Tg and a thin-film composite polyamide membrane's performance: effect of chlorine treatment. J. Membr. Sci. 405, 167–175. doi:10.1016/j.memsci.2012.03.005
Mohsen-Nia, M., Montazeri, P., and Modarress, H. (2007). Removal of Cu2+ and Ni2+ from wastewater with a chelating agent and reverse osmosis processes. Desalination 217, 276–281. ISSN 0011-9164. doi:10.1016/j.desal.2006.01.043
Mondal, S., and Wickramasinghe, S. (2008). Produced water treatment by nanofiltration and reverse osmosis membranes. J. Membr. Sci. 322, 162–170. ISSN 0376 - 7388. doi:10.1016/j.memsci.2008.05.039
Monteiro, C. M., Castro, P. M. L., and Malcata, X. F. (2012). Metal uptake by microalgae: underlying mechanisms and practical applications. doi:10.1002/btpr.1504
Nadig, A. R., Naik, N. S., Padaki, M., Pai, R. K., and Déon, S. (2021). Impact of graphitic carbon nitride nanosheets in mixed-matrix membranes for removal of heavy metals from water. J. Water Process Eng. 41, 102026, 102026. ISSN 2214-7144. doi:10.1016/j.jwpe.2021.102026
Ngah, W. S. W., and Fatinathan, S. (2008). Adsorption of Cu (II) ions in aqueous solution using chitosan beads, chitosan-GLA beads and chitosan-alginate beads. Chem. Eng. J. 143, 62–72. ISSN 1385-8947. doi:10.1016/j.cej.2007.12.006
Öner, Ş. G., Kabay, N., Güler, E., Kitiş, M., and Yüksel, M. (2011). A comparative study for the removal of boron and silica from geothermal water by cross-flow flat sheet reverse osmosis method. Desalination 283, 10–15. ISSN 0011-9164. doi:10.1016/j.desal.2011.02.038
Owalude, S. O., and Tella, A. C. (2016). Removal of hexavalent chromium from aqueous solutions by adsorption on modified groundnut hull. Beni-Suef Univ. J. Basic Appl. Sci. 5, 377–388. ISSN 2314-8535. doi:10.1016/j.bjbas.2016.11.005
Ozaki, H., Sharma, K., and Saktaywin, W. (2002). Performance of an ultra-low-pressure reverse osmosis membrane (ULPROM) for separating heavy metal: effects of interference parameters. Desalination 144, 287–294. ISSN 0011-9164. doi:10.1016/S0011-9164(02)00329-6
Pan, Y., Liu, Y., Zeng, G., Zhao, L., and Lai, Z., (2011) Rapid synthesis of zeolitic imidazolate framework-8 (ZIF-8) nanocrystals in an aqueous system. doi:10.1039/C0CC05002D
Park, H. G., and Kwon, Y. N. (2018). Long-term stability of low-pressure reverse osmosis (RO) membrane operation-A pilot scale study. Water 10, 93. doi:10.3390/w10020093
Peng, L., and Bartzas, G. (2021). Editorial overview: heavy metals and metalloids: a serious threat to environment and human health. Curr. Opin. Environ. Sci. Health 23, 100287. 2468-5844. doi:10.1016/j.coesh.2021.100287
Perales-Vela, H. V., Pena-Castro, J. M., and Canizares-Villanueva, R. O. (2006). Heavy metal detoxification in eukaryotic microalgae. chemosphere 64 (1), 1–10. doi:10.1016/j.chemosphere.2005.11.024
Perpetuo, E. A., Souza, C. B., and Nascimento, C. A. O. (2011). “Engineering bacteria for bioremediation,” in Progress in molecular and environmental bioengineering-from analysis and modeling to technology applications (IntechOpen). doi:10.5772/19546
Petrinic, I., Korenak, J., Povodnik, D., and Hélix-Nielsen, C. (2015). A feasibility study of ultrafiltration/reverse osmosis (UF/RO)-based wastewater treatment and reuse in the metal finishing industry. J. Clean. Prod. 101, 292–300. doi:10.1016/j.jclepro.2015.04.022
Pires da Silva, J. R., Merçon, F., Guimarães Costa, C. M., and Benjo, D. R. (2016). Application of reverse osmosis process associated with EDTA complexation for nickel and copper removal from wastewater. Desalination Water Treat. 57 (41), 19466–19474. doi:10.1080/19443994.2015.1100554
Pishnamazi, M., Koushkbaghi, S., Hosseini, S. S., Darabi, M., Yousefi, A., and Irani, M. (2020). Metal organic framework nanoparticles loaded- PVDF/chitosan nanofibrous ultrafiltration membranes for the removal of BSA protein and Cr(VI) ions. J. Mol. Liq. 317, 113934. ISSN 0167-7322. doi:10.1016/j.molliq.2020.113934
Porcelli, N., and Judd, S. (2010). Chemical cleaning of potable water membranes: the cost benefit of optimisation. Water Res. 44 (5), 1389–1398. ISSN 0043-1354. doi:10.1016/j.watres.2009.11.020
Porter, M. C. (1989). “Handbook of industrial membrane technology” in Includes index. 1. Membranes (Technology) --Handbooks, manuals (Westwood, NJ, USA: Noyes Publications). etc. I. Porter, Mark C. TP159. M4H38 1988 880.2’842 88-17876 ISBN O-81 55-l 205-8.
Qasem, N. A. A., Mohammed, R. H., and Lawal, D. U. (2021). Removal of heavy metal ions from wastewater: a comprehensive and critical review. npj Clean. Water 4, 36. doi:10.1038/s41545-021-00127-0
Qdais, H. A., and Moussa, H. (2004). Removal of heavy metals from wastewater by membrane processes: a comparative study. Desalination 164 (105–110), 105–110. ISSN 0011-9164. doi:10.1016/S0011-9164(04)00169-9
Qi, S., Wang, R., Chaitra, G. K. M., Torres, J., Hu, X., and Fane, A. G. (2016). Aquaporin-based biomimetic reverse osmosis membranes: stability and long term performance. J. Membr. Sci. 508, 94–103. ISSN 0376-7388. doi:10.1016/j.memsci.2016.02.013
Rabiee, H., Khalilpour, K. R., Betts, J. M., and Tapper, N. (2019). Chapter 13 - nergy-water nexus: renewable-integrated hybridized desalination systems, Editor(s): K. Rajab Khalilpour, Polygeneration with polystorage for chemical and energy hubs, Academic Press, Pages 409–458. 9780128133064. doi:10.1016/B978-0-12-813306-4.00013-6
Rasha, H. S., Hassanain, A. H., Khalid, M., Abeda, A., Faiq, A.-A., Dhulfiqar, A. T., et al. (2020). Removal of chromium ions from a real wastewater of leather industry using electrocoagulation and reverse osmosis processes. AIP Conf. Proc. 2213, 020186. doi:10.1063/5.0000201
Rattanakul, S. (2012). “Concentrate and solid waste management in reverse osmosis plants,”. Master’s Thesis in Engineering in environmental engineering and management (Thailand: Asian Institute of Technology School of Environment, Resources and Development).
Reza, M. S. K., Rahimpour, A., Bakeri, G., and Abedini, R. (2019). Experimental and theoretical investigation of thin ZIF-8/chitosan coated layer on air gap membrane distillation performance of PVDF membrane. Desalination 450, 21–32. doi:10.1016/j.desal.2018.10.023
Rezakazemi, M., Dashti, A., Hajilary, N., and Shirazian, S. (2019). “Organic/Silica nanocomposite membranes applicable to green chemistry,” in Sustainable polymer composites and nanocomposites (Cham, Switzerland: Springer International Publishing), 629–652. doi:10.1007/978-3-030-05399-4_22
Ricci, B. C., Ferreira, C. D., Marques, L. S., Martins, S. S., Reis, B. G., and Amaral, M. C. S. (2017). Assessment of the chemical stability of nanofiltration and reverse osmosis membranes employed in treatment of acid gold mining effluent. Sep. Purif. Technol. 174, 301–311. ISSN 1383-5866. doi:10.1016/j.seppur.2016.11.007
Rozaini, M. N. H., Semail, N.-F., Saad, B., Kamaruzaman, S., Abdullah, W. N., Rahim, N. A., et al. (2019). Molecularly imprinted silica gel incorporated with agarose polymer matrix as mixed matrix membrane for separation and preconcentration of sulfonamide antibiotics in water samples. Talanta 199, 522–531. ISSN 0039-9140. doi:10.1016/j.talanta.2019.02.096
Ruiz-García, A., Nuez, I., Carrascosa-Chisvert, M. D., and Santana, J. J. (2020). Simulations of BWRO systems under different feedwater characteristics. Analysis of operation windows and optimal operating points. Desalination 491, 114582. ISSN 0011-9164. doi:10.1016/j.desal.2020.114582
Saad, A. A.-J., and Omar, A. A. (2010). Comparative study on the use of reverse osmosis and adsorption process for heavy metals removal from wastewater in Saudi arabia. Res. J. Environ. Sci. 4, 400–406. doi:10.3923/rjes.2010.400.406
Sagle, A., and Freeman, B., Fundamentals of membranes for water treatment the future of desalination in Texas, 2 (363) (2004), p. 137
Şahin, F., Topuz, B., and Kalıpçılar, H. (2017). Synthesis of ZIF-7, ZIF-8, ZIF-67 and ZIF-L from recycled mother liquors. Microporous Mesoporous Mater. 261, 259–267. ISSN 1387-1811. doi:10.1016/j.micromeso.2017.11.020
Saleh, T. A., Mustaqeem, M., and Khaled, M. (2022). Water treatment technologies in removing heavy metal ions from wastewater: a review. Environ. Nanotechnol. Monit. Manag. 17, 100617. ISSN 2215-1532. doi:10.1016/j.enmm.2021.100617
Samaei, S. M., Gato-Trinidad, S., and Altaee, A. (2020). Performance evaluation of reverse osmosis process in the post-treatment of mining wastewaters: case study of Costerfield mining operations, Victoria, Australia. J. Water Process Eng. 34, 101116. doi:10.1016/j.jwpe.2019.101116
Sandu, T., Sarbu, A., Caprescu, S., Stoica, E.-B., Iordache, T.-V., and Chiriac, A.-L. (2022). Polymer membranes as innovative means of quality restoring for wastewater bearing heavy metals. Membranes 12, 1179. doi:10.3390/membranes12121179
Senán-Salinas, J., Blanco, A., García-Pacheco, R., Landaburu-Aguirre, J., and García-Calvo, E. (2021). Prospective Life Cycle Assessment and economic analysis of direct recycling of end-of-life reverse osmosis membranes based on Geographic Information Systems. doi:10.1016/j.jclepro.2020.124400
Shannon, M., Bohn, P., Elimelech, M., Georgiadis, J. G., Marinas, B. J., and Mayes, A. M. (2008). Science and technology for water purification in the coming decades. Nature 452, 301–310. doi:10.1038/nature06599
Sheng, P. X., Ting, Y. P., Chen, J. P., and Hong, L. (2004). Sorption of lead, copper, cadmium, zinc, and nickel by marine algal biomass: characterization of biosorptive capacity and investigation of mechanisms. J. Colloid Interface Sci. 275 (1), 131–141. ISSN 0021-9797. doi:10.1016/j.jcis.2004.01.036
Singhidi, I., Anqi, A. E., Elkhaleefa, A., Mohamed, A., Ali, I. H., and Brima, E. I. (2021). Temperature impact on reverse osmosis permeate flux in the remediation of hexavalent chromium. Water 14 (1), 44. doi:10.3390/w14010044
Song, X., Gan, B., Yang, Z., Tang, C. Y., and Gao, C. (2019a). Confined nanobubbles shape the surface roughness structures of thin film composite polyamide desalination membranes. J. Membr. Sci. 582, 342–349. ISSN 0376-7388. doi:10.1016/j.memsci.2019.04.027
Song, X., Smith, J. W., Kim, J., Zaluzec, N. J., Chen, W., An, H., et al. (2019b). Unraveling the morphology–function relationships of polyamide membranes using quantitative electron tomography. ACS Appl. Mat. Interfaces 11, 8517–8526. doi:10.1021/acsami.8b20826
Speth, T., Gusses, A., and Summers, R. (2000). Evaluation of nanofiltration pretreatments for flux loss control. Desalination 130, 31–44. ISSN 0011-9164. doi:10.1016/S0011-9164(00)00072-2
Sunil, K., Karunakaran, G., Yadav, S., Padaki, M., Zadorozhnyy, V., and Krishna, P. R. (2018). Al-Ti2O6 a mixed metal oxide based composite membrane: a unique membrane for removal of heavy metals. Chem. Eng. J. 348, 678–684. ISSN 1385-8947. doi:10.1016/j.cej.2018.05.017
Thaçi, B. S., and Gashi, S. T. (2019). Reverse osmosis removal of heavy metals from wastewater effluents using biowaste materials pretreatment. Pol. J. Environ. Stud. 28 (1), 337–341. doi:10.15244/pjoes/81268
Ujang, Z., and Anderson, G. K. (2000). Effect of the operating parameters on the separation of metal chelates using low pressure reverse osmosis membrane (LPROM). Water Sci. Technol. 41 (10-11), 135–142. doi:10.2166/wst.2000.0626
Upadhyay, U., Sreedhar, I., Singh, S. A., Patel, C. M., and Anitha, K. L. (2021). Recent advances in heavy metal removal by chitosan based adsorbents. Carbohydr. Polym. 251, 117000. ISSN 0144-8617. doi:10.1016/j.carbpol.2020.117000
Valdés, H., Saavedra, A., Flores, M., Vera-Puerto, I., Aviña, H., and Belmonte, M. (2021). Reverse osmosis concentrate: physicochemical characteristics, environmental impact, and technologies. Membr. (Basel) 11 (10), 753. doi:10.3390/membranes11100753
Van der Bruggen, B., Lejon, L., and Vandecasteele, C. (2003). Reuse, treatment, and discharge of the concentrate of pressure-driven membrane processes. Environ. Sci. Technol. 37 (17), 3733–3738. doi:10.1021/es0201754
Vrijenhoek, E., Hong, S., and Elimelech, M. (2001). Influence of membrane surface properties on initial rate of colloidal fouling of reverse osmosis and nanofiltration membranes. J. Membr. Sci. 188, 115–128. ISSN 0376-7388. doi:10.1016/S0376-7388(01)00376-3
Wang, J., and Chen, C. (2009). Biosorbents for heavy metals removal and their future. Biotechnol. Adv. 27 (2), 195–226. ISSN 0734-9750. doi:10.1016/j.biotechadv.2008.11.002
Wang, Y., Dai, X., Zhan, Y., Ding, X., Wang, M., and Wang, X. (2019). In situ growth of ZIF-8 nanoparticles on chitosan to form the hybrid nanocomposites for high-efficiency removal of Congo Red. Int. J. Biol. Macromol. 137, 77–86. ISSN 0141-8130. doi:10.1016/j.ijbiomac.2019.06.195
White, C., Sayer, J. A., and Gadd, G. M. (1997). Microbial solubilization and immobilization of toxic metals: key bi-ogeochemical processes for treatment of contamination. doi:10.1111/j.1574-6976.1997.tb00333.x
WHO (2017). Guidelines for drinking-water quality: fourth edition incorporating the first addendum. Geneva: World Health Organization. PMID: 28759192.
Wilson, D. I., Christie, G., Fryer, P. J., Hall, M. I., Landel, J. R., and Whitehead, K. A. (2022). Lessons to learn from roadmapping in cleaning and decontamination. Food Bioprod. Process. 135 (156-164), 156. ISSN 0960-3085. doi:10.1016/j.fbp.2022.07.011
Wu, B., Wang, S., Wang, J., Song, X., Zhou, Y., and Gao, C. (2020). Facile fabrication of high-performance thin film nanocomposite desalination membranes imbedded with alkyl group-capped silica nanoparticles. Polymers 12 (6), 1415. doi:10.3390/polym12061415
Xiang, H., Min, X., Tang, C. J., Sillanpää, M., and Zhao, F. (2022). Recent advances in membrane filtration for heavy metal removal from wastewater: a mini review. J. Water Process Eng. 49, 103023. ISSN 2214-7144. doi:10.1016/j.jwpe.2022.103023
Xu, Y., Gao, X., Wang, X., Wang, Q., Ji, Z., Wang, X., et al. (2016). Highly and stably water permeable thin film nanocomposite membranes doped with MIL-101 (Cr) nanoparticles for reverse osmosis application. Materials 9, 870. doi:10.3390/ma9110870
Yan, W., Wang, Z., Zhao, S., Wang, J., Zhang, P., and Cao, X. (2019). Combining co-solvent-optimized interfacial polymerization and protective coating-controlled chlorination for highly permeable reverse osmosis membranes with high rejection. J. Membr. Sci. 572, 61–72. doi:10.1016/j.memsci.2018.10.084
Yang, R., Li, H., Huang, M., Yang, H., and Li, A. (2016). A review on chitosan-based flocculants and their applications in water treatment. Water Res. 95, 59–89. doi:10.1016/j.watres.2016.02.068
Yang, Z., Zhou, Y., Feng, Z., Rui, X., Zhang, T., and Zhang, Z. A. (2019). A review on reverse osmosis and nanofiltration membranes for water purification. Polymers 11 (8), 1252. doi:10.3390/polym11081252
Yu, C., Li, H., Zhang, X., Lü, Z., Yu, S., Liu, M., et al. (2018). Polyamide thin-film composite membrane fabricated through interfacial polymerization coupled with surface amidation for improved reverse osmosis performance. J. Membr. Sci. 566, 87–95. 0376 - 7388. doi:10.1016/j.memsci.2018.09.012
Zakmout, A., Sadi, F., Portugal, C. A. M., Crespo, J. G., and Velizarov, S. (2020). Tannery effluent treatment by nanofiltration, reverse osmosis and chitosan modified membranes. Membranes 10, 378. doi:10.3390/membranes10120378
Zhang, C. C., Sui, H. Y., Feng, G. L., You, M., Shi, W. X., and Meng, J. Q. (2023). Molecular design of hydrophilized polyethersulfone to enhance water/salt selectivity. doi:10.1021/acs.macromol.2c02285
Zhang, L., Wu, Y., Qu, X., Li, Z., and Ni, J. (2009). Mechanism of combination membrane and electro-winning process on treatment and remediation of Cu2+ polluted water body. J. Environ. Sci. 21, 764–769. ISSN 1001-0742. doi:10.1016/S1001-0742(08)62338-4
Zhang, M., Hu, X., Peng, L., Zhou, S., Zhou, Y., Xie, S., et al. (2022). The intrinsic parameters of the polyamide nanofilm in thin-film composite reverse osmosis (TFC-RO) membranes: the impact of monomer concentration. Membranes 12 (4), 417. doi:10.3390/membranes12040417
Zheng, J., Li, M., Yu, K., Hu, J., Zhang, X., and Wang, L. (2017). Sulfonated multiwall carbon nanotubes assisted thin-film nanocomposite membrane with enhanced water flux and anti-fouling property. J. Membr. Sci. 524, 344–353. ISSN 0376-7388. doi:10.1016/j.memsci.2016.11.032
Keywords: reverse osmosis, heavy metal, thin-film composite, thin-film nanocomposite, wastewater
Citation: Kapepula VL and Luis P (2024) Removal of heavy metals from wastewater using reverse osmosis. Front. Chem. Eng. 6:1334816. doi: 10.3389/fceng.2024.1334816
Received: 07 November 2023; Accepted: 08 January 2024;
Published: 20 February 2024.
Edited by:
Chuan-Yu Wu, University of Surrey, United KingdomReviewed by:
Sadiye Velioglu, Gebze Technical University, TürkiyeCopyright © 2024 Kapepula and Luis. This is an open-access article distributed under the terms of the Creative Commons Attribution License (CC BY). The use, distribution or reproduction in other forums is permitted, provided the original author(s) and the copyright owner(s) are credited and that the original publication in this journal is cited, in accordance with accepted academic practice. No use, distribution or reproduction is permitted which does not comply with these terms.
*Correspondence: Vercus Lumami Kapepula, bHVtYW1pa2FwZXB1bGFAZ21haWwuY29t; Patricia Luis, cGF0cmljaWEubHVpc0B1Y2xvdXZhaW4uYmU=
Disclaimer: All claims expressed in this article are solely those of the authors and do not necessarily represent those of their affiliated organizations, or those of the publisher, the editors and the reviewers. Any product that may be evaluated in this article or claim that may be made by its manufacturer is not guaranteed or endorsed by the publisher.
Research integrity at Frontiers
Learn more about the work of our research integrity team to safeguard the quality of each article we publish.