- 1Department of Chemical and Biomolecular Engineering, University of Nebraska, Lincoln, NE, United States
- 2Department of Biomedical Engineering, Pennsylvania State University, University Park, PA, United States
- 3Huck Institutes of the Life Sciences, Pennsylvania State University, University Park, PA, United States
Human pluripotent stem cells (hPSCs) are ideal “raw materials” for making various human cell types for regenerative medicine and are needed in large numbers. 3D suspension culturing (e.g., stirred-tank bioreactor or STR), which suspends and cultures cells in an agitated medium, has been extensively studied to scale up hPSC production. However, a significant problem with 3D suspension is the uncontrolled spheroid agglomeration. It leads to cell growth arrest, cell apoptosis, and inhomogeneity in cell purity and quality. We propose that i) inhibiting the spheroid adhesion can prevent spheroid agglomeration and ii) the inhibition can be achieved via coating spheroids with biocompatible anti-adhesion molecules. We used PEG-lipids as model anti-adhesion molecules to successfully demonstrate the concept. PEG-lipids anchor to the spheroid surface through the interactions between their lipid chains and the cell membrane lipids. The flexible and hydrophilic PEG chains act as a dynamic barrier to prevent spheroid adhesion. We showed that the coating eliminated spheroid agglomeration, leading to homogenous spheroid size distribution and significant improvements in cell growth rate and volumetric yield. This novel approach is expected to impact large-scale hPSC production significantly. Furthermore, the approach can be generalized for culturing other human cell types.
Introduction
Eighty percent of healthcare costs are used to treat late-stage illnesses, which could be treated or managed early through regenerative medicine (Denning et al., 2016). Human pluripotent stem cells (hPSCs) including human embryonic stem cells (hESCs) (Thomson et al., 1998) and induced pluripotent stem cells (iPSCs), have unlimited proliferation capability. They can be expanded to generate large numbers of cells. hPSCs also have the potential to differentiate into all somatic cells (Tabar and Studer, 2014; Kimbrel and Lanza, 2015; Trounson and Mcdonald, 2015). Thus they are ideal “raw materials” for making various human cell types for regenerative medicine. Since their first report in 1998, hPSCs have been successfully differentiated into various cell types such as cardiomyocytes, beta cells, endothelial cells, vascular smooth muscle cells, muscle cells, neural stem cells, neurons, macrophages, T cells, natural killer cells, etc. (Yamanaka, 2020). hPSC-derived cells have been widely used to model genetic and environmental diseases (Brennand et al., 2011; Devine et al., 2011; Israel et al., 2012; Ambasudhan et al., 2013; Kondo et al., 2013; Reinhardt et al., 2013; Woodruff et al., 2013; Young et al., 2015; Soldner et al., 2016). They have been applied for screening new drugs (Höing et al., 2012; Naryshkin et al., 2014; Bright et al., 2015; Kumari et al., 2015; McNeish et al., 2015), testing drug toxicity (Matsa et al., 2016a; Matsa et al., 2016b; Burridge et al., 2016; Kodo et al., 2016; Sayed et al., 2016; Sharma et al., 2017; Magdy et al., 2018), engineering tissues, and developing cell-based therapies (Barker et al., 2017; Kikuchi et al., 2017; Mandai et al., 2017). Several clinical trials using hPSC derivatives are ongoing (Mandai et al., 2017; Deinsberger et al., 2020).
However, the widespread use of hPSCs and their derivatives is limited by the difficulty of producing them on large scales at high quality (Jenkins and Farid, 2015; Mount et al., 2015; Silva et al., 2015; Denning et al., 2016; Hartman et al., 2016; Kempf et al., 2016; National science and technology council, 2016). Regenerative medicine needs large numbers of cells (Lei and Schaffer, 2013; Denning et al., 2016; Kempf et al., 2016). For instance, ∼1 × 1010 cardiomyocytes or beta cells for treating a myocardial infarction or diabetic patient (Amour et al., 2006; Serra et al., 2012; Chong et al., 2014), 1 × 1011 platelets for a platelet transfusion (Amour et al., 2006; Serra et al., 2012; Chong et al., 2014), ∼1 × 1010 cardiomyocytes for making a human heart (Badylak et al., 2011), and ∼1 × 1010 cells for screening one million compounds (Desbordes and Studer, 2012). Regenerative medicine also requires cells to have high quality, such as normal genetics, epigenetics, phenotypes, and high in vivo safety and functions (Lei and Schaffer, 2013; Denning et al., 2016; Kempf et al., 2016). hPSC-derived cells can be prepared as universal cells and used as off-shelf products (i.e., allogeneic cells). This approach requires the capability to culture massive numbers of cells per batch (Denning et al., 2016; Hartman et al., 2016; Kempf et al., 2016). Alternatively, patient-specific cells can be prepared for personalized regenerative medicine (i.e., autologous cells). This approach requires a technology to produce cells for thousands of patients in parallel (Trainor et al., 2014). To date, the production of high-quality allogeneic or autologous cells at large scales has not been achieved due to the low efficiency of current cell culture methods (Baum et al., 2013; National Cell Manufacturing Consortium, 2016; National science and technology council, 2016).
hPSCs are typically cultured on a 2D surface. However, 2D culturing is labor- and space-consuming and is only suitable for preparing small numbers of cells (Kropp et al., 2017). Due to its success in culturing Chinese Hamster Ovary (CHO) cells for producing protein therapeutics, 3D suspension culturing (e.g., stirred-tank bioreactor or STR), which suspends and cultures cells in an agitated medium, has been extensively studied to scale up hPSC production (Polak and Mantalaris, 2008; Serra et al., 2012). However, a significant problem with 3D suspension is the uncontrolled cellular aggregation. hPSCs have strong cell-cell interactions (Chen et al., 2014a; Chen et al., 2014b) that make them aggregate to form large cell agglomerates in suspension (Kinney et al., 2011; Fridley et al., 2012; Kropp et al., 2017). The mass transport in agglomerates >500 µm in diameter becomes insufficient, leading to cell growth arrest, apoptosis, and uncontrolled differentiation (Hajdu et al., 2010; Kropp et al., 2017). Additionally, uncontrolled cellular aggregation leads to inhomogeneity in hPSC aggregate or spheroid size. Since the spheroid size significantly influences the hPSC differentiation efficiency (Jara-avaca et al., 2014; Kropp et al., 2017), the inhomogeneity in aggregate size results in heterogeneity in product purity (Jara-avaca et al., 2014; Kropp et al., 2017).
In short, uncontrolled cellular aggregation should be mitigated to improve the hPSC culture efficiency and product homogeneity in 3D suspension culture. This report proposes to coat hPSC spheroids with biocompatible anti-adhesion molecules to reduce spheroid agglomeration. To our best knowledge, this is the first report to propose and successfully demonstrate using a molecular coating to reduce spheroid agglomeration to enhance hPSC culture efficiency. We believe this novel approach will have a significant impact on large-scale hPSC production.
Materials and methods
Materials
E8 medium (E8), accutase and Live/Dead cell viability staining kit: Life Technologies; Y-27632: Selleckchem; Matrigel: BD Biosciences; Calcein AM viability dye (#50-169-52, eBiosicence); Ethidium homodimer I (#40010, Biotium); Antibodies for Nanog, Oct4, SSEA-4 and Alkaline phosphatase (R&D system, 10 μg/ml); Nestin antibody (BioLegend, 1:200); α-SMA antibody (Santa Cruz, 1:200); FOXA2 antibody (Santa Cruz, 1:200); Polyethylene Glycol conjugated with 1,2-Dipalmitoryl-sn-Glycero-3-Phosphoethanolamine (PEG-DPPE, Mw5000, #PG1-DP-5k, Nanocs); Polyethylene Glycol conjugated with 1,2-Distearoyl-sn-Glycero-3-Phosphoethanolamine (PEG-DSPE, Mw5000, #PG1-DS-5k, Nanocs); Fluorescein labeled DSPE-PEG (DSPE-PEG-FITC, Mw 5,000, PG2-DSFC-5k, Nanocs); Fluorescein labeled DPPE-PEG (DPPE-PEG-FITC, Mw5000, PG2-DPFC-5k, Nanocs).
Culturing hPSCs in 2D
Fib-iPSCs and MSC-iPSCs (iPSCs reprogrammed from human dermal fibroblasts and human mesenchymal stem cells, respectively) were obtained from George Q. Daley laboratory (Children’s Hospital Boston, Boston) (Park et al., 2008). hPSCs were maintained in a 6-well plate coated with Matrigel (BD Biosciences) in E8 medium as described in our previous publications (Lei and Schaffer, 2013; Li et al., 2018). Cells were passaged every 4 days with 0.5 mM EDTA (Invitrogen), and the medium was changed daily. Cells were routinely checked for the expression of pluripotency markers, Oct4 and Nanog, their capability to form teratomas, their karyotypes, and bacterial contamination.
hPSC spheroids formation and fusion experiments
Single hPSCs (1,000–6,000 cells) in 200 µL E8 medium were placed in one V-shape non-adhesive well of a 96-well plate for 48 h to form one hPSC spheroid. For the spheroid fusion experiments, two spheroids were placed in one V-shape well of the 96-well plate. The fusion process was then imaged with a microscope.
Surface modification of hPSC spheroids with PEG-lipids and 3D suspension culture
PEG-lipids in PBS was added to the spheroid suspension and then incubated for 30 min with gentle agitation at room temperature. These spheroids were washed once and then cultured in the incubator at 37°C, 5% CO2. For 3D suspension culture, single cells were suspended in E8 media in a low attachment 6-well plate and shaken at around 57 rpm for 24 h to form small clusters. After that, cell spheroids were treated with 250 μg/ml PEG-DPPE for 30 min in PBS before culturing in fresh E8 medium daily.
Scanning electron microscopy
Cell spheroids were prepared for scanning electron microscopy (SEM) to observe the cell spheroid morphology with or without PEG-DPPE coating. Briefly, cell spheroids were fixed in 2.5% glutaraldehyde in 100 mM phosphate buffer, PH 7.0 for 2–3 h at room temperature, then 4°C for 1–2 h. Then the samples were fixed in 1% osmium tetroxide (OsO4) in 100 mM phosphate buffer, PH 7.0 for 1 h at room temperature, followed by at least three washes in 100 mM phosphate buffer. Samples were subjected to dehydration through an alcohol series (30%, 50%, 70%, 2 × 95%, 2 × 100%) before HMDS coating (2:1 to 1:2 ethanol:HMDS; 100% HMDS) and then left the samples in 100% HMDS to air-dry in the hood. Keep in a low-vacuumed desiccator and transport to the core for sputter coating before image collection using SEM.
Staining, flow cytometry, and imaging. Cells cultured on 2D surfaces were fixed with 4% paraformaldehyde (PFA) at room temperature for 15 min, permeabilized with 0.25% Triton X-100 for 15 min, and blocked with 5% donkey serum for 1 h. Cells were then incubated with primary antibodies at 4°C overnight. After extensive washing, secondary antibodies and 4′,6-Diamidino-2-Phenylindole, Dihydrochloride (DAPI) were added and incubated for another 1 h at room temperature. Finally, cells were washed with PBS 3 times before imaging with a Zeiss Axio Observer Fluorescent Microscopy. LIVE/DEAD® Cell Viability staining was used to assess live and dead cells, according to the product manual.
EB differentiation
hPSC spheroids were cultured for five passages with PEG-DPPE and then suspended in DMEM +20% FBS +10 µM β-mercaptoethanol in a low adhesion plate for 6 days. The cell mass was then transferred onto plates coated with 0.1% gelatin and cultured in the same medium for another 6 days, followed by fixation and staining as above.
Statistical analysis
The data are presented as the mean ± SD. We used an unpaired t-test to compare two groups and one-way ANOVA to compare more than two groups. p < 0.05 was considered statistically significant.
Results
Using molecular coating to avoid spheroid agglomeration
3D suspension cultures are being widely studied for culturing hPSCs due to their simplicity, easiness for scaling up, and automation (New Brunswick Scientific et al., 2009; Serra et al., 2012). Ideally, inoculated single hPSCs expand through two phases: single cells first associate to form small cell clusters (i.e., initial clustering) that subsequently expand as spheroids (i.e., cell or spheroid expansion) (Figure 1A). Unfortunately, neighboring spheroids frequently adhere to each other and fuse to form large agglomerates (>500 μm, i.e., spheroid agglomeration) (Figure 1A). The initial clustering, spheroid expansion, and spheroid agglomeration were clearly demonstrated in a simple experiment. Two vials of hPSCs, stained with DIO and DID dyes, respectively, were mixed at 1:1 and cultured in suspension. The lipophilic DIO and DID dyes stain cells to appear green and red, respectively, under fluorescent microscopy. Single cells associated to form small clusters in 24 h that subsequently expanded as spheroids. Spheroid agglomeration was frequently found on day 4 (Figure 1B).
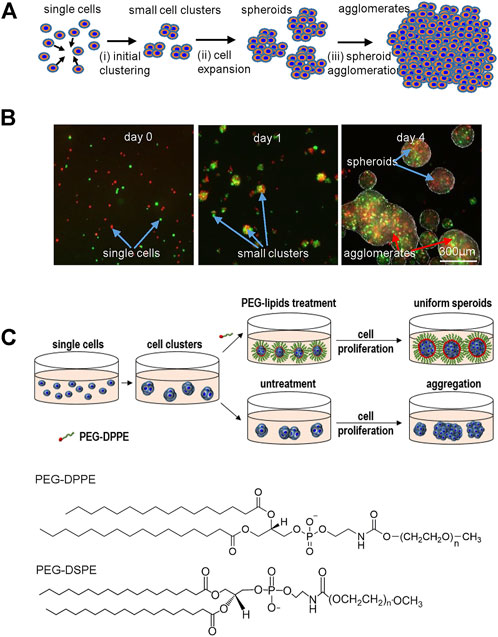
FIGURE 1. (A) The two phases in suspension cultures: single cells associate to form small clusters (i.e., initial clustering) that subsequently expand as spheroids (i.e., cell expansion). However, spheroids frequently aggregate into large agglomerates, leading to slow cell growth, death and uncontrolled differentiation. (B) Two vials of hPSCs, stained with DIO (green) and DID (red) dyes respectively, were mixed at 1:1 and cultured in suspension. Small clusters were seen at 24 h. Both small cell spheroids and large cell agglomerates were seen on day 4. (C) Schematic illustration of preventing spheroids agglomeration via surface coating with anti-adhesion molecules. PEG-lipids were added after small cluster were formed. The lipid segment was insert into the membrane of cells on the spheroid surface. The flexible PEG chains act as a dynamic barrier to prevent the spheroid adhesion. PEG-DPPE and PEG-DSPE are used in this study.
It is well known that the transport of nutrients, oxygen, and growth factors to cells located at the center of agglomerates with diameters larger than 500 µm becomes insufficient (Hajdu et al., 2010; Kropp et al., 2017). Therefore, the large cell spheroids exhibit a layer-like structure, including a necrotic core and an outer layer of live cells (Alvarez-Pérez et al., 2005; Lin and Chang, 2008). This was confirmed in our experiment. We prepared hPSC spheroids with diameters of 200 μm, 400 μm, 500 μm, 700 μm, and 800 µm and cultured them for 3 days (Figure 2). Live/dead staining showed very few dead cells in spheroids <400 µm and significant dead cells in spheroids ≥500 µm. Large necrotic cores appeared when the spheroid’s diameter exceeded 700 μm (Figures 2C, D). Therefore, uncontrolled cell aggregation (>500 µm) is a critical problem for 3D suspension culture (Lei and Schaffer, 2013; Lei et al., 2014; Li et al., 2018). The above mechanistic studies clearly show that if hPSCs could follow the initial clustering-spheroid expansion path without spheroid agglomeration, the resultant spheroids would have uniform size, and the cell growth rate, viability, yield, and quality could be maximized.
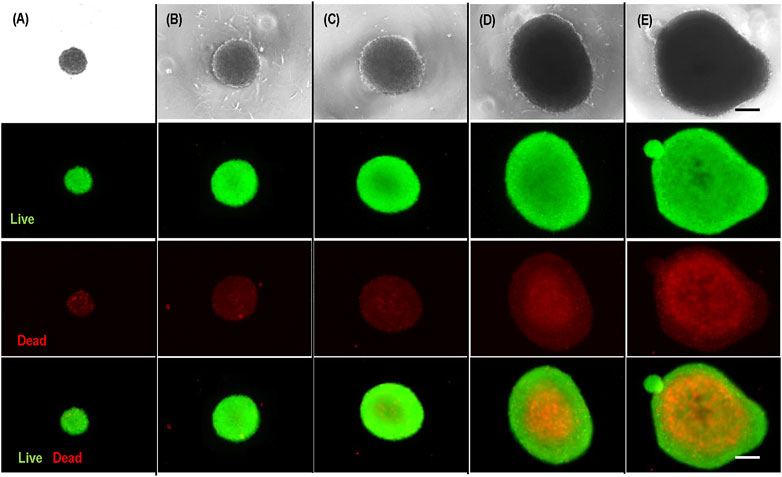
FIGURE 2. Live/dead staining of hPSC spheroids with diameter of 200 µm (A), 400 µm (B), 500 µm (C), 700 µm (D), 800 µm (E). Scale bar: 200 µm.
To find a solution to prevent spheroid agglomeration, we must understand the molecular mechanisms under cell aggregation and spheroid agglomeration. On the plasma membranes of single cells or cells on the spheroid surface, many cell-cell adhesion molecules (e.g., cadherins) and receptors for ECM proteins (e.g., integrins) are not bound by their ligands (Foty and Steinberg, 2005). These molecules drive cells to aggregate to maximize their interactions with their ligands (Ivascu and Kubbies, 2006; Pérez-Pomares and Foty, 2006; Ivascu and Kubbies, 2007). Cells can aggregate through, individually or combined, cell-cell adhesions and cell-matrix interactions. Through these mechanisms, single hPSCs associate to form small clusters. During the cell expansion in suspension cultures, and when two spheroids contact, cells on one spheroid can adhere to cells or ECMs of the other spheroid through cell-cell, cell-matrix interactions, leading to spheroid adhesion and agglomeration.
We propose that i) inhibiting the spheroid adhesion can prevent spheroid agglomeration and ii) the inhibition can be achieved via coating the spheroids with a layer of biocompatible anti-adhesion molecules (Figure 1C). This study will use Polyethylene glycol conjugated with lipids (PEG-lipids) as model anti-adhesion molecules to demonstrate the concept. The PEG-lipids can anchor to the spheroid surface through the interactions between their lipid chains and the cell membrane lipids (Tatsumi et al., 2012). The flexible and hydrophilic PEG chains act as a dynamic barrier to prevent spheroid adhesion.
Spheroid fusion assay
We developed a spheroid fusion assay for quantitatively studying spheroid agglomeration. To generate hPSC spheroids, single cells were suspended in V-bottom low attachment 96-well plates. Within hours, single cells settled at the well bottom and formed a cell cluster. For the fusion assay, two spheroids were placed into one low attachment V-shape well (Figure 3A). The fusion process was imaged at 0, 2, 8, and 28 h. We used the (πr2)/(πR2), wherein r and R are the radius of the interface of the two spheroids and the radius of the spheroids, respectively, to quantitatively measure the percentage of the spheroid fusion (Figure 3C). And t1/2 is referred to the time when 50% of the fusion is completed or (πr2)/(πR2) = 0.5 (Figure 3D). We found that t1/2 is less than 5 h. We measured the fusion rates of spheroids with different diameters and found that the spheroid size did not significantly affect the fusion rate (Figures 3B–D).
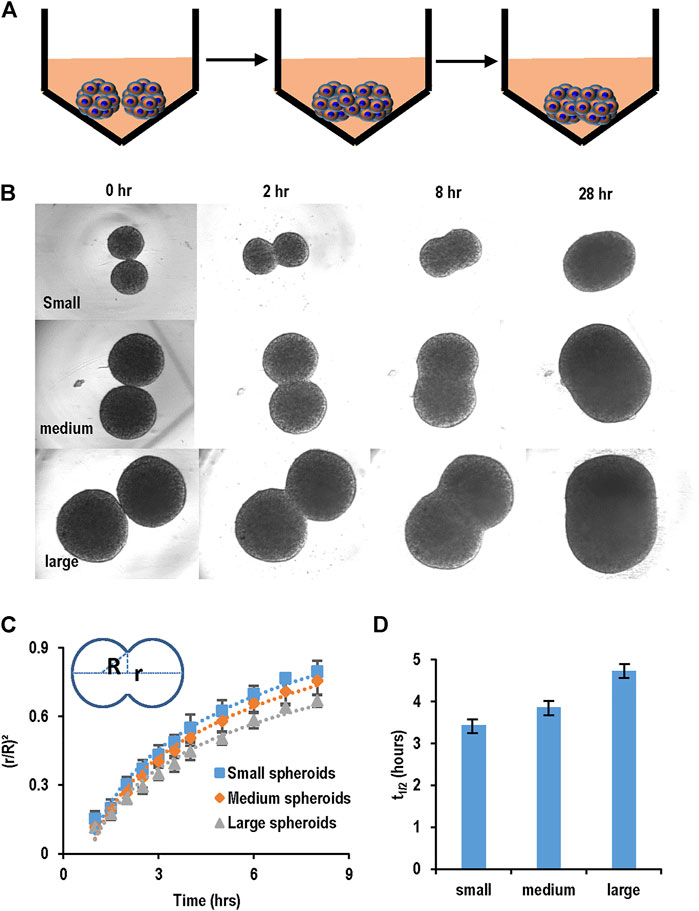
FIGURE 3. Spheroid fusion assay. (A,B) hPSC spheroids with different sizes (small, medium and large) were prepared in V-bottom low attachment 96-well plates, and then two spheroids were placed in contact and the fusion process was imaged using microscopy at 1, 2, 4, 8, and 28 h. (C) The spheroid fusion rates were quantified using (r/R)2, where r and R were the radius of the interface of the two spheroids and the radius of the spheroids, respectively. (D) t1/2, the time to complete 50% fusion or (r/R)2 = 1/2. Small, medium and large spheroids had similar fusion rates.
PEG-lipids coating on hPSC spheroids
PEG-lipids molecules have been reported for surface modification of cells to modulate immunogenicity (Teramura and Iwata, 2010a; Teramura and Iwata, 2010b; Inui et al., 2010; Takemoto et al., 2011; Tatsumi et al., 2012). We first studied if PEG-lipids could coat hPSC spheroids. Spheroids were prepared and treated with PEG-DPPE at varied concentrations for 30 min. Without PEG-DPPE coating, the spheroid surface was smooth, and the cell-to-cell boundaries were not clearly seen (Figures 4A, B). The spheroid surface became rough, and cell-to-cell boundaries became apparent after PEG-DPPE coating. The morphology change indicates that PEG-DPPE coating decreased the spheroid surface tension. The coating did not cause cell death. When treated with 100 μg/ml, 250 μg/ml, 500 μg/ml, very few dead cells were detected (Figure 4C).
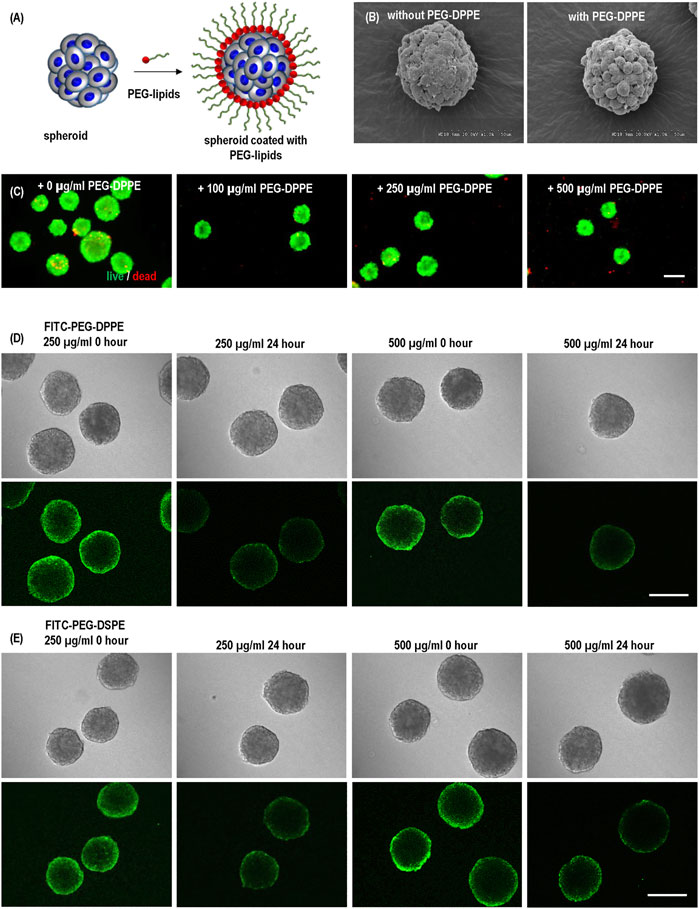
FIGURE 4. (A) hPSC spheroids were coated with PEG-lipids. (B) Scanning electron microscopy images of uncoated and coated spheroids. (C) Live dead staining of spheroids coated with 0, 100, 250, and 500 μg/ml PEG-DPPE. (D,E) Confocal scanning microscopic images of hPSC spheroids coated with 250 and 500 μg/ml FITC-PEG-DPPE (D) or FITC-PEG-DSPE (E). Free PEG-lipids were removed from the medium after coating. Images were taken immediately (0 h) and 24 h after coating. Scale bar: 200 µm.
To confirm the coating and investigate its stability, we coated spheroids with FITC labeled PEG-DPPE for 30 min and imaged them with a confocal microscope immediately and 24 h after the coating. Fluorescence was clearly observed at the spheroid surface, showing successful coating. However, 24 h later, the fluorescence intensity decreased to about 50%, indicating the half-life time of PEG-DPPE on the spheroid surface was about 24 h (Figure 4D). We treated the spheroids with both 250 μg/ml and 500 μg/ml PEG-DPPE and found the coating efficiency and half-life time were similar, indicating 250 μg/ml was sufficient for coating (Figure 4D). To assess if the lipid length affects the coating stability, we also coated spheroids with FITC labeled PEG-DSPE. The fatty acid DPPE and DSPE have 16 and 18 carbons, respectively. No significant difference was observed between FITC-PEG-DPPE and FITC-PEG-DSPE in terms of the fluorescent intensity immediately and 24 h after coating (Figure 4E).
PEG-lipids coating reduced spheroid agglomeration
We evaluated the effect of PEG-DPPE coating on the spheroid fusion process (Figure 5). Spheroids were generated and treated with 0, 100, 250, and 500 μg/ml PEG-DPPE for 30 min. Two spheroids were placed in one V-shape, and the fusion process was recorded (Figures 5A, B). We found that the fusion rate decreased as the PEG-DPPE concentration increased (Figure 5C). The fusion became extremely slow when treated with 250 or 500 μg/ml PEG-DPPE. The t1/2 for spheroids coated with 0, 100, 250, and 500 μg/ml PEG-DPPE was 3.7, 9.2, 26.8, and 69 h, respectively (Figure 5D). We observed that spheroids without coating adhered around 5 min after contacting, and PEG-DPPE coating extended this time to ∼20 min. Similar results were obtained when PEG-DSPE was used. Thus molecular coating could effectively prevent spheroid adhesion and agglomeration.
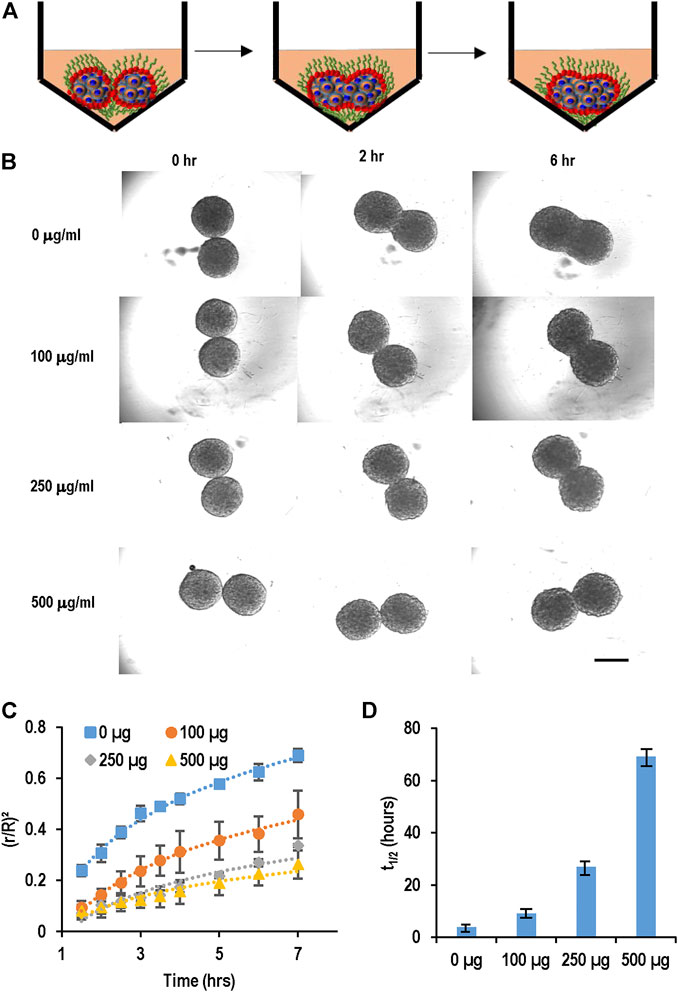
FIGURE 5. PEG-lipids coating slowed down spheroid fusion. (A,B) hPSC spheroids were coated with 0, 100, 250, 500 μg/ml PEG-DPPE, and two spheroids were placed in one well. Their fusion process was recorded with microscopy. (C) The fusion rates and (D) t1/2. Scale bar: 200 µm.
PEG-lipids coating prevented spheroid agglomeration in 3D suspension culture
Next, we investigated the effect of PEG-lipids coating in 3D suspension culture. Single cells were suspended in the medium in a low attachment-well plate with shaking at 57 rpm for 24 h to form small cell clusters. The clusters were treated with PEG-DPPE daily, and cultures without PEG-DPPE coating were used as the control. Two initial seeding densities (5 × 105 cells/ml and 1 × 106 cells/ml) were tested to evaluate the effect of seeding density. Two hPSC lines, induced pluripotent stem cells generated from human mesenchymal stem cell cells (MSC-iPSC) and skin fibroblasts (Fib-iPSC), were studied to assess if the coating method could be cell-line dependent. Cells were harvested daily to analyze the spheroid size, cell number, and cell viability.
For MSC-iPSCs, severe spheroid agglomerations were seen on day 3 and day 4, especially for the high seeding density (Figures 6A, B; Table 1). With the PEG-DPPE coating, uniform cell spheroids were formed at both seeding densities from day 1 to day 4 (Figures 6A, B). We also quantified the size distribution (Figures 6C, D). At low seeding density and without PEG-DPPE coating, the size distribution was 50–121 μm, 65–180 μm, and 85–379 μm, and the median was 90, 121, and 183 µm on day 1, 2, and 3, respectively. With PEG-DPPE coating, the size distribution became much narrower, e.g., 39–103 μm, 51–124 μm, and 74–178 µm on day 1, 2, and 3, respectively (Figures 6C, D). Moreover, the median became significantly smaller, for instance, 71, 84, and 117 µm on day 1, 2, and 3, respectively (Figures 6C, D). The reductions in mean spheroid size and size distribution by PEG-DPPE coating were also seen at the high seeding density. Without coating, the median was 93, 149, and 189 μm, and the distribution was 51–173 μm, 73–377 μm, and 92–510 µm on day 1, 2, and 3, respectively. With PEG-DPPE coating, the median was 72, 98, and 126 μm, and the size distribution was 41–108 μm, 59–148 μm, and 76–181 µm on day 1, 2, and 3, respectively. (Figures 6C, D). Large agglomerates were not seen with PEG-DPPE coating.
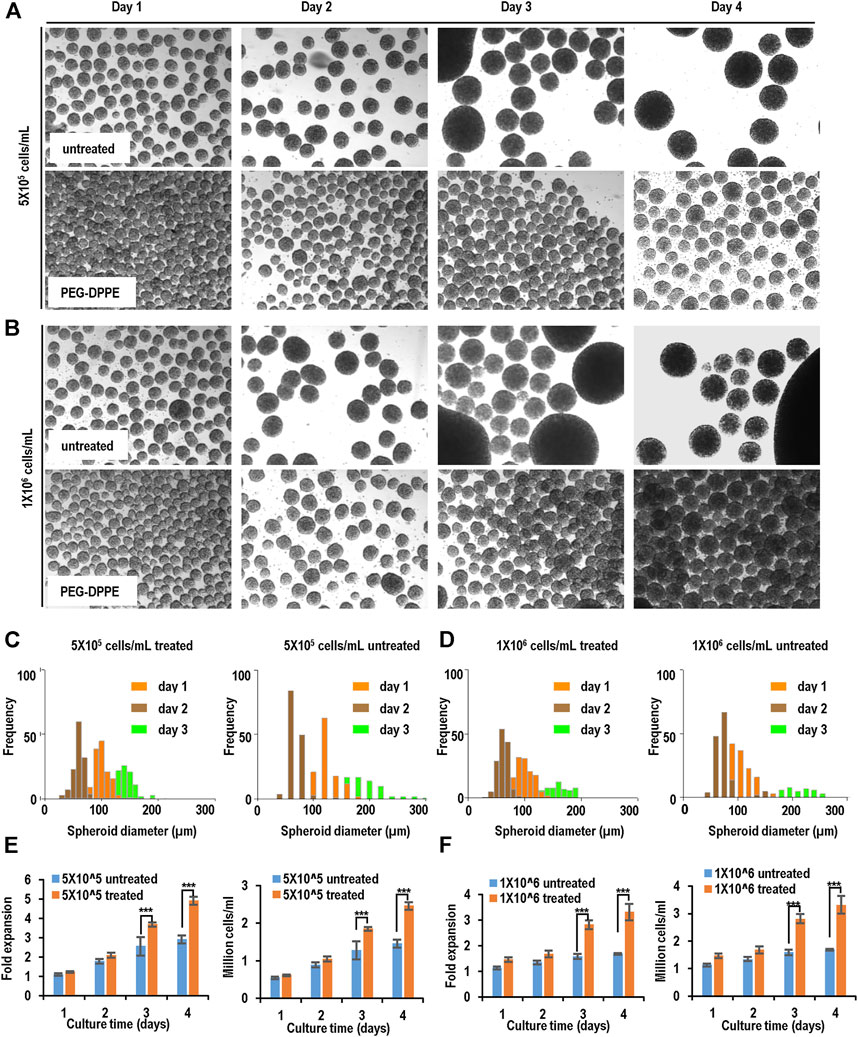
FIGURE 6. Suspension culture of MSC-iPSCs. (A,B) Phase images of spheroids on days 1–4 without and with PEG-DPPE coating at two seeding densities. (C,D) Size distribution of spheroids on days 1–3. (E,F) Fold of expansion and volumetric yield of uncoated and coated cells. ***: p < 0.001.
For both seeding densities without PEG-DPPE coating, the cell number exhibited a slow increase in the first 3 days, and no apparent increase was seen on day 4, resulting in about 2.9- and 1.7-fold expansion after 4 days with a final cell density at 1.5 × 106 cells/ml and 1.7 × 106 cells/ml for 5 × 105 cells/ml and 1 × 106 cells/ml seeding density, respectively, (Figures 6E, F). With PEG-DPPE coating, cells grew in all 4 days, resulting in about 4.9 - and 3.3-fold expansion with a final cell density at 2.5 × 106 cells/ml and 3.3 × 106 cells/ml for 5 × 105 cells/ml and 1 × 106 cells/ml, respectively (Figures 6E, F). Thus the cell expansion and yield were significantly improved by PEG-DPPE coating.
Similar results were achieved when Fib-iPSCs were used (Figure 7, Table 1). PEG-DPPE coating eliminated spheroid agglomeration. When seeded at 5 × 105 cells/ml and without coating, the median was 68, 118, and 183 μm, and the size distribution was 42–136 μm, 53–176 μm, and 76–356 µm on day 1, 2, and 3, respectively, with PEG-DPPE coating, the median was 61, 97, and 139 μm, and the size distributions were 27–85 μm, 48–146 μm, and 71–192 µm on day 1, 2, and 3, respectively. When seeded at 1 × 106 cells/ml without PEG-DPPE coating, the median was 71, 133, and 205 μm, and the size distribution was 51–158 μm, 67–322 μm, and 102–456 µm on day 1, 2, and 3, respectively. With PEG-DPPE coating, the median was 62, 98, and 140 μm, and the size distribution was 32–89 μm, 60–169 μm, and 80–195 µm on day 1, 2, and 3, respectively (Figures 7C, D). Without coating, about 3.2- and 1.7-fold expansion with a final cell density at 1.6 × 106 cells/ml and 1.7 × 106 cells/ml were obtained for 5 × 105 cells/ml and 1 × 106 cells/ml seeding density, respectively. With PEG-DPPE coating, about 5.5 - and 3.4-fold expansion with a final cell density at 2.8 × 106 cells/ml and 3.4 × 106 cells/ml were achieved for 5 × 105 cells/ml and 1 × 106 cells/ml seeding density, respectively (Figures 7E, F). In summary, PEG-DPPE coating leads to uniform spheroid formation in 3D suspension culture with significantly improved expansion fold and cell yield.
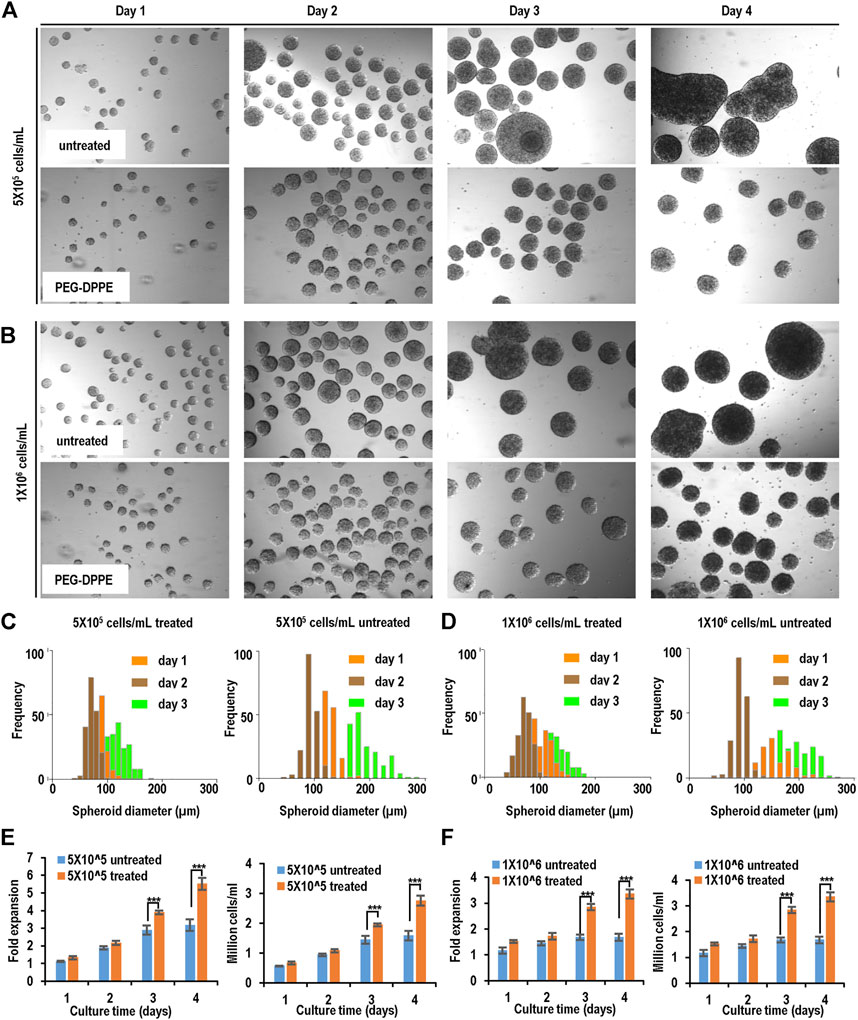
FIGURE 7. Suspension culture of Fib-iPSCs. (A,B) Phase images of spheroids on days 1–4 without and with PEG-DPPE coating at two seeding densities. (C,D) Size distribution of spheroids on days 1–3. (E,F) Fold of expansion and volumetric yield of uncoated and coated cells. ***: p < 0.001.
hPSCs retained pluripotency after long-term culture with PEG-lipids coating
We cultured hPSCs in suspension with PEG-DPPE coating for 10 passages and evaluated their pluripotency. The PEG-DPPE coating did not sacrifice the pluripotency. Confocal microscope image and flow cytometry analyses showed a majority of cells expressed pluripotency markers after 5 and 10 passages (Figures 8A–C). In addition, in vitro embryoid body (EB) differentiation was performed. hPSCs could be differentiated into the Nestin + ectodermal cells, α-SMA + mesodermal cells, and FOXA2+ endodermal cells in the EB assay (Figure 8D). All these results indicate that the PEG-lipids coating support hPSC culture without altering the pluripotency.
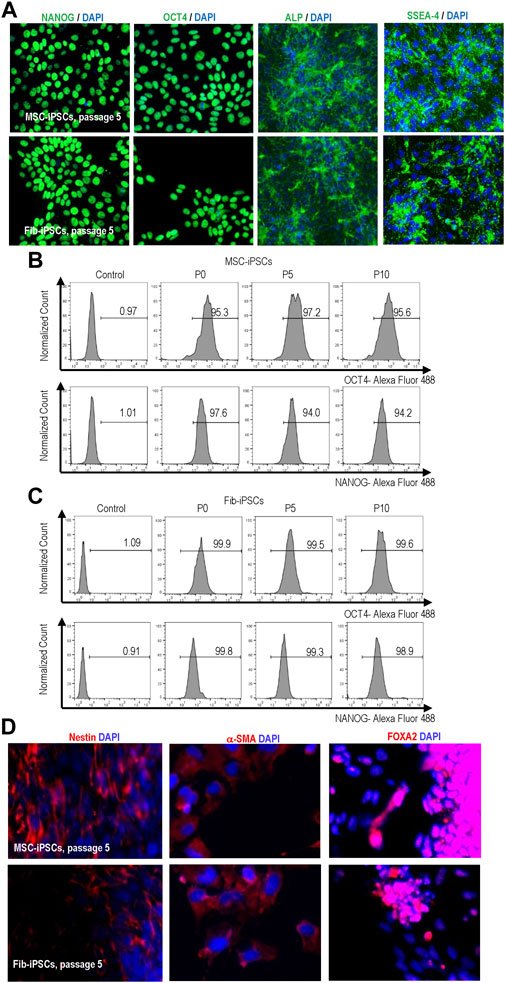
FIGURE 8. Expression of pluripotency markers in MSC-iPSCs and Fib-iPSCs at passage 0, 5 and 10 via imaging (A) and flow cytometry (B, C) analyses. These cells could be differentiated into the Nestin + ectodermal, SMA + mesodermal and FOXA2+ endodermal cells in the EB assay (D).
Discussion
hPSCs are an ideal cell source for producing various human cell types for regenerative medicine, cell therapy, drug discovery, and disease modeling (Yamanaka, 2020). Large numbers of cells are needed for these applications. 3D suspension is a promising approach to scale up the production (Lei and Schaffer, 2013; Lei et al., 2014; Lin et al., 2017a; Lin et al., 2018a; Lin et al., 2018b; Ekerdt et al., 2018; Li et al., 2018; Lin et al., 2019a; Lin et al., 2019b; Lin et al., 2019d; Wang and Lei, 2019; Wang et al., 2021). However, uncontrolled spheroid agglomeration is a significant problem that negatively affects cell viability, growth rate, yield, and quality in suspension culture. Thus methods to mitigate spheroid agglomeration are valuable and should be developed.
Researchers have tried to optimize the bioprocess parameters, such as the stirring speed, to reduce agglomeration (Kempf et al., 2016). In general, a higher stirring speed reduces spheroids’ settlement and agglomeration. However, a high stirring speed results in shear stress, negatively influencing cell viability. In short, this approach can mitigate spheroid agglomeration to a certain level but cannot wholly abolish it (Kempf et al., 2016). We and others have cultured hPSCs in hydrogels with the intention of using hydrogels as physical barriers to eliminate cell agglomeration (Gerecht et al., 2007; Chayosumrit et al., 2010; Serra et al., 2011; Stenberg et al., 2011), but hPSCs have slow growth in most hydrogels. Significantly, we found that a very soft thermoreversible hydrogel enabled the long-term, serial expansion of hPSCs with excellent cell viability, growth rate (20-fold/5 days), and yield (∼2.0 × 107 cells/ml) (Lei and Schaffer, 2013; Lei et al., 2014; Li et al., 2016; Lin et al., 2017a; Lin et al., 2017b). However, this hydrogel is unstable for long-term and large-scale cell culture. Additionally, the material is expensive and variable between batches.
Our lab also explored using hydrogel tube microbioreactors to prevent agglomeration (Lei and Schaffer, 2013; Lei et al., 2014; Li et al., 2016; Lin et al., 2017a; Lin et al., 2017b). In this method, cells are cultured in microscale hollow hydrogel tubes suspended in a bioreactor. The hydrogel tubes can confine the radial diameter of cell masses within the diffusion limit to ensure efficient mass transport. We showed that hPSCs could grow in hydrogel tubes with high viability (>95%), growth rate (1,000-fold expansion/9 days), pluripotency, and yield (5.0 × 108 cells/ml). However, the hydrogel tubes are incompatible with the existing stirred tank bioreactors since the stirring can break the soft hydrogel tubes. Thus special bioreactors should be developed to contain these hydrogel tubes.
In this study, we proposed to use molecular coating to prevent spheroid agglomeration. Compared to the hydrogel tubes, molecular coating is simple and compatible with existing bioreactors. The biocompatible anti-adhesion molecules can be added to the cell culture media as additives. The concept was successfully demonstrated using PEG-lipids. Spheroid agglomeration was eliminated, resulting in spheroids with uniform size. Consequently, the cell growth rate and volumetric yield were significantly improved. In the future, we can design molecules with better stability and anti-adhesion capabilities to further improve the bioprocess outcome. Although we used lipids to anchor the anti-adhesion molecules in this study, other methods, such as covalent conjugation, ionic binding, and the ligand or antibody-based affinity binding, can also be used to coat the anti-adhesion molecules. In this study, we used PEG as anti-adhesion molecules. In principle, any biocompatible molecules with anti-adhesion functions can be used. In this study, we focused on culturing hPSCs. In principle, the molecular coating method can be applied to any cell type in suspension culture.
Recent research shows that spheroids can be used as building blocks to bioprint tissues or organs (Mironov et al., 2003; Mironov et al., 2009; Mironov et al., 2011; Laschke and Menger, 2016). Uniform spheroids are important as building blocks. Current methods to prepare these building blocks include hanging drops, microfluidics, micro-molds, spinner suspension culture, and rotating wall vessels (Mironov et al., 2009; Mironov et al., 2011; Olsen and Alexis, 2014; Laschke and Menger, 2016). With the hanging drop method, small volumes of cell suspensions are placed on a lid that is subsequently inverted to form hanging drops. Cells in each hanging drop aggregate to form one spheroid. With the microfluidic and micro-mold methods, cells are placed in micro-wells to form one spheroid per well. With the spinner culture and rotating wall vessel methods, large numbers of cells are cultured in suspension in the spinner flasks or rotating wall vessels to simultaneously generate many spheroids. The microfluidics, hanging drop, and micro-molds cannot produce spheroids at a large scale (Olsen and Alexis, 2014). 3D suspension is the scalable method to produce spheroids but suffers from spheroid agglomeration. We believe the molecular coating method described in this study can be used to address the problem. (Takahashi et al., 2007), (Shi et al., 2017)
Data availability statement
The original contributions presented in the study are included in the article/supplementary material further inquiries can be directed to the corresponding author.
Author contributions
Author YL: Conceived and designed the analysis. Draft the paper. Author Ql: Collected the data. Performed the analysis. Author YP: Collected the data. Author LH: Performed the analysis and revised manuscript. Author YY and XW: Collected the data.
Funding
Research reported in this publication was supported by the National Heart, Lung, and Blood Institute of the National Institutes of Health under Award Number R33HL163711; and the National Cancer Institute under Award Number R33CA235326.
Conflict of interest
The authors declare that the research was conducted in the absence of any commercial or financial relationships that could be construed as a potential conflict of interest.
Publisher’s note
All claims expressed in this article are solely those of the authors and do not necessarily represent those of their affiliated organizations, or those of the publisher, the editors and the reviewers. Any product that may be evaluated in this article, or claim that may be made by its manufacturer, is not guaranteed or endorsed by the publisher.
References
Alvarez-Pérez, J., Ballesteros, P., and Cerdán, S. (2005). Microscopic images of intraspheroidal pH by 1H magnetic resonance chemical shift imaging of pH sensitive indicators. Magn. Reson Mater Phys. Biol. Med. 18 (6), 293–301. doi:10.1007/s10334-005-0013-z
Ambasudhan, R., Ryan, S. D., Dolatabadi, N., Chan, S. F., Zhang, X., Akhtar, M. W., et al. (2013). Isogenic human iPSC Parkinson’s model shows nitrosative stress-induced dysfunction in MEF2-pgc1α transcription. Cell 155 (6), 1351–1364. doi:10.1016/j.cell.2013.11.009
Amour, K. A. D., Bang, A. G., Eliazer, S., Kelly, O. G., Agulnick, A. D., Smart, N. G., et al. (2006). Production of pancreatic hormone – expressing endocrine cells from human embryonic stem cells. Nat. Biotechnol. 24 (11), 1392–1401. doi:10.1038/nbt1259
Badylak, S. F., Taylor, D., and Uygun, K. (2011). Whole-organ tissue engineering: Decellularization and recellularization of three-dimensional matrix scaffolds. Annu. Rev. Biomed. Eng. 13, 27–53. doi:10.1146/annurev-bioeng-071910-124743
Barker, R. A., Parmar, M., Studer, L., and Takahashi, J. (2017). Human trials of stem cell-derived dopamine neurons for Parkinson's disease: Dawn of a new era. Cell Stem Cell 21 (5), 569–573. doi:10.1016/j.stem.2017.09.014
Baum, E., Littman, N., Ruffin, M., Ward, S., and Aschheim, K. (2013). Key tools and technology hurdles in advancing stem-cell therapies. AvaliableAt: https://www.cirm.ca.gov/sites/default/files/files/.
Brennand, K. J., Simone, A., Jou, J., Gelboin-Burkhart, C., Tran, N., Sangar, S., et al. (2011). Modelling schizophrenia using human induced pluripotent stem cells. Nature 473 (7346), 221–225. doi:10.1038/nature09915
Bright, J., Hussain, S., Dang, V., Wright, S., Cooper, B., Byun, T., et al. (2015). Human secreted tau increases amyloid-beta production. Neurobiol. Aging 36 (2), 693–709. doi:10.1016/j.neurobiolaging.2014.09.007
Burridge, P. W., Li, Y. F., Matsa, E., Wu, H., Ong, S. G., Sharma, A., et al. (2016). Human induced pluripotent stem cell-derived cardiomyocytes recapitulate the predilection of breast cancer patients to doxorubicin-induced cardiotoxicity. Nat. Med. 22 (5), 547–556. doi:10.1038/nm.4087
Chayosumrit, M., Tuch, B., and Sidhu, K. (2010). Alginate microcapsule for propagation and directed differentiation of hESCs to definitive endoderm. Biomaterials 31 (3), 505–514. doi:10.1016/j.biomaterials.2009.09.071
Chen, K. G., Mallon, B. S., Johnson, K. R., Hamilton, R. S., Mckay, R. D. G., and Robey, P. G. (2014). Developmental insights from early mammalian embryos and core signaling pathways that influence human pluripotent cell growth and differentiation. Stem Cell Res. 12 (3), 610–621. doi:10.1016/j.scr.2014.02.002
Chen, K. G., Mallon, B. S., McKay, R. D. G., and Robey, P. G. (2014). Human pluripotent stem cell culture: Considerations for maintenance, expansion, and therapeutics. Cell Stem Cell, 14 (1), 13–26. doi:10.1016/j.stem.2013.12.005
Chong, J. J. H., Yang, X., Don, C. W., Minami, E., Liu, Y-W., Weyers, J. J., et al. (2014). Human embryonic-stem-cell-derived cardiomyocytes regenerate non-human primate hearts. Nature 510 (7504), 273–7. doi:10.1038/nature13233
Deinsberger, J., Reisinger, D., and Weber, B. (2020). Global trends in clinical trials involving pluripotent stem cells: A systematic multi-database analysis. npj Regen. Med. 5 (1), 15–13. doi:10.1038/s41536-020-00100-4
Denning, C., Borgdorff, V., Crutchley, J., Firth, K. S. A., George, V., Kalra, S., et al. (2016). Cardiomyocytes from human pluripotent stem cells: From laboratory curiosity to industrial biomedical platform. Biochimica Biophysica Acta - Mol. Cell Res. 1863 (7), 1728–1748. doi:10.1016/j.bbamcr.2015.10.014
Desbordes, S. C., and Studer, L. (2012). Adapting human pluripotent stem cells to high-throughput and high-content screening. Nat. Protoc. 8 (1), 111–130. doi:10.1038/nprot.2012.139
Devine, M. J., Ryten, M., Vodicka, P., Thomson, A. J., Burdon, T., Houlden, H., et al. (2011). Parkinson's disease induced pluripotent stem cells with triplication of the α-synuclein locus. Nat. Commun. 2 (1), 440–40. doi:10.1038/ncomms1453
Ekerdt, B. L., Fuentes, C. M., Lei, Y., Adil, M. M., Ramasubramanian, A., Segalman, R. A., et al. (2018). Thermoreversible hyaluronic acid-PNIPAAm hydrogel systems for 3D stem cell culture. Adv. Healthc. Mat. 7, e1800225. doi:10.1002/adhm.201800225
Foty, R. a., and Steinberg, M. S. (2005). The differential adhesion hypothesis: A direct evaluation. Dev Biol 278 (1), 255–63. doi:10.1016/j.ydbio.2004.11.012
Fridley, K. M., Kinney, M. A., and McDevitt, T. C. (2012). Hydrodynamic modulation of pluripotent stem cells. Stem Cell Res. Ther. 3 (6), 45. doi:10.1186/scrt136
Gerecht, S., Burdick, J. a., Ferreira, L. S., Townsend, S. a., Langer, R., and Vunjak-Novakovic, G. (2007). Hyaluronic acid hydrogel for controlled self-renewal and differentiation of human embryonic stem cells. Proc. Natl. Acad. Sci. U. S. A. 104 (27), 11298–11303. doi:10.1073/pnas.0703723104
Hajdu, Z., Mironov, V., Mehesz, A. N., Norris, R. A., Markwald, R. R., and Visconti, R. P. (2010). Tissue spheroid fusion-based in vitro screening assays for analysis of tissue maturation. J. Tissue Eng. Regen. Med. 4, 659–664. doi:10.1002/term.291
Hartman, M. E., Dai, D., and La, M. A. (2016). Human pluripotent stem cells : Prospects and challenges as a source of cardiomyocytes for in vitro modeling and cell-based cardiac repair. Adv. Drug Deliv. Rev. 96, 3–17. doi:10.1016/j.addr.2015.05.004
Höing, S., Rudhard, Y., Reinhardt, P., Glatza, M., Stehling, M., Wu, G., et al. (2012). Discovery of inhibitors of microglial neurotoxicity acting through multiple mechanisms using a stem-cell-based phenotypic assay. Cell Stem Cell 11 (5), 620–632. doi:10.1016/j.stem.2012.07.005
Inui, O., Teramura, Y., and Iwata, H. (2010). Retention dynamics of amphiphilic polymers PEG-lipids and PVA-Alkyl on the cell surface. ACS Appl. Mat. Interfaces 2 (5), 1514–1520. doi:10.1021/am100134v
Israel, M. A., Yuan, S. H., Bardy, C., Reyna, S. M., Mu, Y., Herrera, C., et al. (2012). Probing sporadic and familial Alzheimer's disease using induced pluripotent stem cells. Nature 482 (7384), 216–220. doi:10.1038/nature10821
Ivascu, A., and Kubbies, M. (2007). Diversity of cell-mediated adhesions in breast cancer spheroids. Int. J. Oncol. 11, 1403–1413. doi:10.3892/ijo.31.6.1403
Ivascu, a., and Kubbies, M. (2006). Rapid generation of single-tumor spheroids for high-throughput cell function and toxicity analysis. SLAS Discov. 11 (8), 922–932. doi:10.1177/1087057106292763
Jara-avaca, M., Kempf, H., Olmer, R., Kropp, C., Ru, M., Robles-diaz, D., et al. (2014). Controlling expansion and cardiomyogenic differentiation of human pluripotent stem cells in scalable suspension culture. Stem Cell Rep. 3, 1132–1146. doi:10.1016/j.stemcr.2014.09.017
Jenkins, M. J., and Farid, S. S. (2015). Human pluripotent stem cell-derived products: Advances towards robust, scalable and cost-effective manufacturing strategies. Biotechnol. J. 10, 83–95. doi:10.1002/biot.201400348
Kempf, H., Andree, B., and Zweigerdt, R. (2016). Large-scale production of human pluripotent stem cell derived cardiomyocytes. Adv. Drug Deliv. Rev. 96, 18–30. doi:10.1016/j.addr.2015.11.016
Kikuchi, T., Morizane, A., Doi, D., Magotani, H., Onoe, H., Hayashi, T., et al. (2017). Human iPS cell-derived dopaminergic neurons function in a primate Parkinson's disease model. Nature 548 (7669), 592–596. doi:10.1038/nature23664
Kimbrel, E. A., and Lanza, R. (2015). Current status of pluripotent stem cells: Moving the first therapies to the clinic. Nat. Rev. Drug Discov. 14 (10), 681–692. doi:10.1038/nrd4738
Kinney, M. A., Sargent, C. Y., and Mcdevitt, T. C. (2011). The multiparametric effects of hydrodynamic environments on stem cell culture. Tissue Eng. Part B Rev. 17, 249–262. doi:10.1089/ten.teb.2011.0040
Kodo, K., Ong, S. G., Jahanbani, F., Termglinchan, V., Hirono, K., Inanloorahatloo, K., et al. (2016). iPSC-derived cardiomyocytes reveal abnormal TGF-β signalling in left ventricular non-compaction cardiomyopathy. Nat. Cell Biol. 18 (10), 1031–1042. doi:10.1038/ncb3411
Kondo, T., Asai, M., Tsukita, K., Kutoku, Y., Ohsawa, Y., Sunada, Y., et al. (2013). Modeling Alzheimer's disease with iPSCs reveals stress phenotypes associated with intracellular Aβ and differential drug responsiveness. Cell Stem Cell 12 (4), 487–496. doi:10.1016/j.stem.2013.01.009
Kropp, C., Massai, D., and Zweigerdt, R. (2017). Progress and challenges in large-scale expansion of human pluripotent stem cells. Process Biochem. 59, 244–254. doi:10.1016/j.procbio.2016.09.032
Kumari, D., Swaroop, M., Southall, N., Huang, W., Zheng, W., and Usdin, K. (2015). High-throughput screening to identify compounds that increase fragile X mental retardation protein expression in neural stem cells differentiated from fragile X syndrome patient-derived induced pluripotent stem cells. Stem Cells Transl. Med. 4 (7), 800–808. doi:10.5966/sctm.2014-0278
Laschke, M. W., and Menger, M. D. (2016). Life is 3D : Boosting spheroid function for tissue engineering. Trends Biotechnol. 35, 133–144. doi:10.1016/j.tibtech.2016.08.004
Lei, Y., Jeong, D., Xiao, J., and Schaffer, D. V. (2014). Developing defined and scalable 3D culture systems for culturing human pluripotent stem cells at high densities. Cell. Mol. Bioeng. 7 (2), 172–183. doi:10.1007/s12195-014-0333-z
Lei, Y., and Schaffer, D. V. (2013). A fully defined and scalable 3D culture system for human pluripotent stem cell expansion and differentiation. Proc. Natl. Acad. Sci. U. S. A. 110, E5039–E5048. doi:10.1073/pnas.1309408110
Li, Q., Lin, H., Du, Q., Liu, K., Wang, O., Evans, C., et al. (2018). Scalable and physiologically relevant microenvironments for human pluripotent stem cell expansion and differentiation. Biofabrication 10, 25006. doi:10.1088/1758-5090/aaa6b5
Li, Q., Lin, H., Wang, O., Qiu, X., Kidambi, S., Deleyrolle, L. P., et al. (2016). Scalable production of glioblastoma tumor-initiating cells in 3 dimension thermoreversible hydrogels. Sci. Rep. 6, 31915. doi:10.1038/srep31915
Lin, H., Du, Q., Li, Q., Wang, O., Wang, Z., Elowsky, C., et al. (2019). Manufacturing human pluripotent stem cell derived endothelial cells in scalable and cell-friendly microenvironments, Biomater Sci, 7, 373–388. doi:10.1039/c8bm01095a
Lin, H., Du, Q., Li, Q., Wang, O., Wang, Z., Liu, K., et al. (2018). Hydrogel-based bioprocess for scalable manufacturing of human pluripotent stem cell-derived neural stem cells. ACS Appl. Mat. Interfaces 10, 29238–29250. doi:10.1021/acsami.8b05780
Lin, H., Du, Q., Li, Q., Wang, O., Wang, Z., Sahu, N., et al. (2018). A scalable and efficient bioprocess for manufacturing human pluripotent stem cell-derived endothelial cells. Stem Cell Rep. 11 (2), 454–469. doi:10.1016/j.stemcr.2018.07.001
Lin, H., Li, Q., Du, Q., Wang, O., Wang, Z., Akert, L., et al. (2019). Integrated generation of induced pluripotent stem cells in a low-cost device, Biomaterials, 189, 23–36. doi:10.1016/j.biomaterials.2018.10.027
Lin, H., Li, Q., and Lei, Y. (2017). An integrated miniature bioprocessing for personalized human induced pluripotent stem cell expansion and differentiation into neural stem cells. Sci. Rep. 7, 40191. doi:10.1038/srep40191
Lin, H., Li, Q., and Lei, Y. (2017). Three-dimensional tissues using human pluripotent stem cell spheroids as biofabrication building blocks. Biofabrication 9, 25007. doi:10.1088/1758-5090/aa663b
Lin, H., Qiu, X., Du, Q., Li, Q., Wang, O., Akert, L., et al. (2019). Engineered Microenvironment for Manufacturing Human Pluripotent Stem Cell-Derived Vascular Smooth Muscle Cells, Stem Cell Reports, 8, 84–97. doi:10.1016/j.stemcr.2018.11.009
Lin, R. Z., and Chang, H. Y. (2008). Recent advances in three-dimensional multicellular spheroid culture for biomedical research. Biotechnol. J. 3 (9–10), 1285–1384. doi:10.1002/biot.1285
Magdy, T., Schuldt, A. J. T., Wu, J. C., Bernstein, D., and Burridge, P. W. (2018). Human induced pluripotent stem cell (hiPSC)-derived cells to assess drug cardiotoxicity: Opportunities and problems. Annu. Rev. Pharmacol. Toxicol. 58, 83–103. doi:10.1146/annurev-pharmtox-010617-053110
Mandai, M., Watanabe, A., Kurimoto, Y., Hirami, Y., Morinaga, C., Daimon, T., et al. (2017). Autologous induced stem-cell–derived retinal cells for macular degeneration. N. Engl. J. Med. Overseas. Ed. 376 (11), 1038–1046. doi:10.1056/NEJMoa1608368
Matsa, E., Ahrens, J. H., and Wu, J. C. (2016). Human induced pluripotent stem cells as a platform for personalized and precision cardiovascular medicine. Physiol. Rev. 96 (3), 1093–1126. doi:10.1152/physrev.00036.2015
Matsa, E., Burridge, P. W., Yu, K. H., Ahrens, J. H., Termglinchan, V., Wu, H., et al. (2016). Transcriptome profiling of patient-specific human iPSC-cardiomyocytes predicts individual drug safety and efficacy responses in vitro. Cell Stem Cell 19 (3), 311–325. doi:10.1016/j.stem.2016.07.006
McNeish, J., Gardner, J. P., Wainger, B. J., Woolf, C. J., and Eggan, K. (2015). From dish to bedside: Lessons learned while translating findings from a stem cell model of disease to a clinical trial. Cell Stem Cell 17 (1), 8–10. doi:10.1016/j.stem.2015.06.013
Mironov, V., Boland, T., Trusk, T., Forgacs, G., and Markwald, R. R. (2003). Organ printing: Computer-aided jet-based 3D tissue engineering. Trends Biotechnol. 21 (4), 157–161. doi:10.1016/s0167-7799(03)00033-7
Mironov, V., Kasyanov, V., and Markwald, R. R. (2011). Organ printing: From bioprinter to organ biofabrication line. Curr. Opin. Biotechnol. 22 (5), 667–673. doi:10.1016/j.copbio.2011.02.006
Mironov, V., Visconti, R. P., Kasyanov, V., Forgacs, G., Drake, C. J., and Markwald, R. R. (2009). Organ printing: Tissue spheroids as building blocks. Biomaterials 30 (12), 2164–2174. doi:10.1016/j.biomaterials.2008.12.084
Mount, N. M., Ward, S. J., Kefalas, P., and Hyllner, J. (2015). Cell-based therapy technology classifications and translational challenges. Phil. Trans. R. Soc. B 370 (1680), 20150017. doi:10.1098/rstb.2015.0017
Naryshkin, N. a., Weetall, M., Dakka, A., Narasimhan, J., Zhao, X., Feng, Z., et al. (2014). SMN2 splicing modifiers improve motor function and longevity in mice with spinal muscular atrophy. Science 345 (6197), 688–693. doi:10.1126/science.1250127
National Cell Manufacturing Consortium (2016). Achieving large-scale, cost-effective, reproducible manufacturing of high-quality cells. Available from: http://cellmanufacturingusa.org/sites/default/files/NCMC_Roadmap_021816_high_res-2.pdf.
National science and technology council (2016). Advanced Manufacturing: A Snapshot of Priority Technology Areas Across the Federal Government. Available from: https://www.whitehouse.gov/sites/whitehouse.gov/files/images/Blog/NSTC SAM technology areas snapshot.pdf.
New Brunswick Scientific Gouzheng, W., Zhang, W., Rich, M., and Gossain, V. (2009). Growing CHO cells in a CelliGen ® BLU benchtop , stirred-tank bioreactor using single-use vessels. Biotechnol. Prog. 1, 4. doi:10.1038/nmeth.f.277
Olsen, T. R., and Alexis, F. (2014). Bioprocess. Tissues using Cell. Spheroids 4 (2), 2–5. doi:10.4172/2155-9821.1000e112
Park, I-H., Zhao, R., West, J. a., Yabuuchi, A., Huo, H., Ince, T. a., et al. (2008). Reprogramming of human somatic cells to pluripotency with defined factors. Nature 451, 141–146. doi:10.1038/nature06534
Pérez-Pomares, J. M., and Foty, R. A. (2006). Tissue fusion and cell sorting in embryonic development and disease: Biomedical implications. Bioessays, 28(8):809–21. doi:10.1002/bies.20442
Polak, J. M., and Mantalaris, S. (2008). Stem cells bioprocessing : An important milestone to move regenerative medicine research into the clinical arena. Pediatr. Res. 63, 461–466. doi:10.1203/pdr.0b013e31816a8c1c
Reinhardt, P., Schmid, B., Burbulla, L. F., Schöndorf, D. C., Wagner, L., Glatza, M., et al. (2013). Genetic correction of a lrrk2 mutation in human iPSCs links parkinsonian neurodegeneration to ERK-dependent changes in gene expression. Cell Stem Cell 12 (3), 354–367. doi:10.1016/j.stem.2013.01.008
Sayed, N., Liu, C., and Wu, J. C. (2016). Translation of human-induced Pluripotent Stem cells. J. Am. Coll. Cardiol. 67 (18), 2161–2176. doi:10.1016/j.jacc.2016.01.083
Serra, M., Brito, C., Correia, C., and Alves, P. M. (2012). Process engineering of human pluripotent stem cells for clinical application. Trends Biotechnol. 30 (6), 350–359. doi:10.1016/j.tibtech.2012.03.003
Serra, M., Correia, C., Malpique, R., Brito, C., Jensen, J., Bjorquist, P., et al. (2011). Microencapsulation technology: A powerful tool for integrating expansion and cryopreservation of human embryonic stem cells. PLoS One 6 (8), e23212. doi:10.1371/journal.pone.0023212
Sharma, A., Burridge, P. W., Mckeithan, W. L., Serrano, R., Shukla, P., Sayed, N., et al. (2017). High-throughput screening of tyrosine kinase inhibitor cardiotoxicity with human induced pluripotent stem cells. Sci. Transl. Med. 9 (377), eaaf2584. doi:10.1126/scitranslmed.aaf2584
Shi, Y., Inoue, H., Wu, J. C., and Yamanaka, S. (2017). Induced pluripotent stem cell technology: A decade of progress. Nat. Rev. Drug Discov. 16 (2), 115–130. doi:10.1038/nrd.2016.245
Silva, M., Daheron, L., Hurley, H., Bure, K., Barker, R., Carr, A. J., et al. (2015). Generating iPSCs: Translating cell reprogramming science into scalable and robust biomanufacturing strategies. Cell Stem Cell 16 (1), 13–17. doi:10.1016/j.stem.2014.12.013
Soldner, F., Stelzer, Y., Shivalila, C. S., Abraham, B. J., Latourelle, J. C., Barrasa, M. I., et al. (2016). Parkinson-associated risk variant in distal enhancer of α-synuclein modulates target gene expression. Nature 533 (7601), 95–99. doi:10.1038/nature17939
Stenberg, J., Elovsson, M., Strehl, R., Kilmare, E., Hyllner, J., and Lindahl, A. (2011). Sustained embryoid body formation and culture in a non-laborious three dimensional culture system for human embryonic stem cells. Cytotechnology 63 (3), 227–237. doi:10.1007/s10616-011-9344-y
Tabar, V., and Studer, L. (2014). Pluripotent stem cells in regenerative medicine: Challenges and recent progress. Nat. Rev. Genet. 15 (2), 82–92. doi:10.1038/nrg3563
Takahashi, K., Tanabe, K., Ohnuki, M., Narita, M., Ichisaka, T., Tomoda, K., et al. (2007). Induction of pluripotent stem cells from adult human fibroblasts by defined factors. Cell 131 (5), 861–872. doi:10.1016/j.cell.2007.11.019
Takemoto, N., Teramura, Y., and Iwata, H. (2011). Islet surface modification with urokinase through DNA hybridization. Bioconjug. Chem. 22 (4), 673–678. doi:10.1021/bc100453r
Tatsumi, K., Ohashi, K., Teramura, Y., Utoh, R., Kanegae, K., Watanabe, N., et al. (2012). The non-invasive cell surface modification of hepatocytes with PEG-lipid derivatives. Biomaterials 33 (3), 821–828. doi:10.1016/j.biomaterials.2011.10.016
Teramura, Y., and Iwata, H. (2010). Bioartificial pancreas. Adv. Drug Deliv. Rev. 62 (7–8), 827–840. doi:10.1016/j.addr.2010.01.005
Teramura, Y., and Iwata, H. (2010). Cell surface modification with polymers for biomedical studies. Soft Matter. 6 (6), 1081. doi:10.1039/b913621e
Thomson, J. A., Itskovitz-Eldor, J., Shapiro, S. S., Waknitz, M. A., Swiergiel, J. J., Marshall, V. S., et al. (1998). Embryonic stem cell lines derived from human blastocysts. Science 282, 1145–1147. doi:10.1126/science.282.5391.1145
Trainor, N., Pietak, A., and Smith, T. (2014). Rethinking clinical delivery of adult stem cell therapies. Nat. Biotechnol. 32 (8), 729–735. doi:10.1038/nbt.2970
Trounson, A., and Mcdonald, C. (2015). Stem cell therapies in clinical trials: Progress and challenges. Cell Stem Cell 17 (1), 11–22. doi:10.1016/j.stem.2015.06.007
Wang, O., and Lei, Y. (2019). Creating a cell-friendly microenvironment to enhance cell culture efficiency. Cell Gene Ther. Insights 5 (3), 341–350. doi:10.18609/cgti.2019.038
Wang, Z., Zuo, F., Liu, Q., Wu, X., Du, Q., Lei, Y., et al. (2021). Comparative study of human pluripotent stem cell-derived endothelial cells in hydrogel-based culture systems. ACS Omega 6 (10), 6942–6952. doi:10.1021/acsomega.0c06187
Woodruff, G., Young, J. E., Martinez, F. J., Buen, F., Gore, A., Kinaga, J., et al. (2013). The Presenilin-1 δE9 mutation results in reduced γ-secretase activity, but not total loss of PS1 function, in isogenic human stem cells. Cell Rep. 5 (4), 974–985. doi:10.1016/j.celrep.2013.10.018
Yamanaka, S. (2020). Pluripotent stem cell-based cell therapy—promise and challenges. Cell Stem Cell 27 (4), 523–531. doi:10.1016/j.stem.2020.09.014
Young, J. E., Boulanger-Weill, J., Williams, D. A., Woodruff, G., Buen, F., Revilla, A. C., et al. (2015). Elucidating molecular phenotypes caused by the SORL1 Alzheimer's disease genetic risk factor using human induced pluripotent stem cells. Cell Stem Cell 16 (4), 373–385. doi:10.1016/j.stem.2015.02.004
Keywords: human pluripotent stem cells, three-dimensional culture, surface coating, surfactant, poly (ethylene glycol)
Citation: Li Q, Pan Y, Han L, Yang Y, Wu X and Lei Y (2022) Improving three-dimensional human pluripotent cell culture efficiency via surface molecule coating. Front. Chem. Eng. 4:1031395. doi: 10.3389/fceng.2022.1031395
Received: 30 August 2022; Accepted: 04 October 2022;
Published: 20 October 2022.
Edited by:
Yan Li, Florida State University, United StatesReviewed by:
Xiaoping Bao, Purdue University, United StatesYong Yang, University of North Texas, United States
Copyright © 2022 Li, Pan, Han, Yang, Wu and Lei. This is an open-access article distributed under the terms of the Creative Commons Attribution License (CC BY). The use, distribution or reproduction in other forums is permitted, provided the original author(s) and the copyright owner(s) are credited and that the original publication in this journal is cited, in accordance with accepted academic practice. No use, distribution or reproduction is permitted which does not comply with these terms.
*Correspondence: Yuguo Lei, eXhsNjAzNEBwc3UuZWR1
†These authors have contributed equally to this work