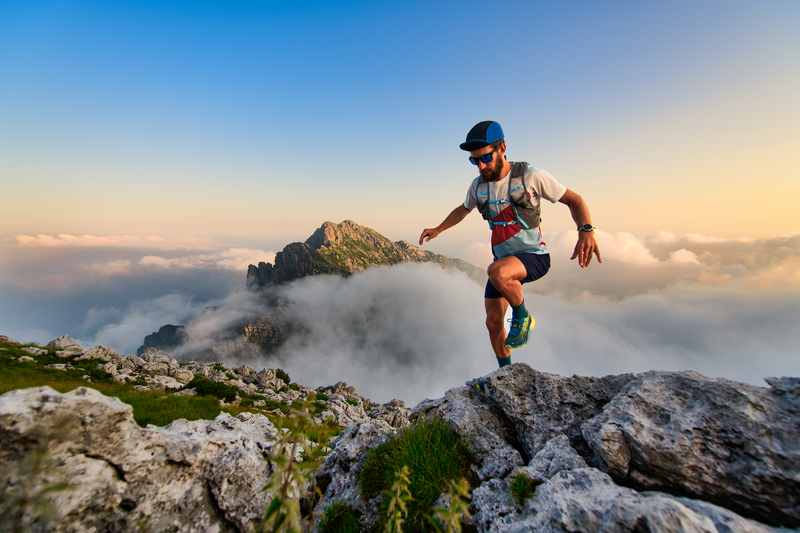
95% of researchers rate our articles as excellent or good
Learn more about the work of our research integrity team to safeguard the quality of each article we publish.
Find out more
REVIEW article
Front. Cell. Neurosci. , 02 April 2025
Sec. Cellular Neuropathology
Volume 19 - 2025 | https://doi.org/10.3389/fncel.2025.1548252
This article is part of the Research Topic Methods in cellular neurobiology research View all 3 articles
The dorsal horn of the spinal cord represents the first site in the central nervous system (CNS) where nociceptive signals are integrated. As a result, there has been a rapid growth in the number of studies investigating the ionic mechanisms regulating the excitability of dorsal horn neurons under normal and pathological conditions. We believe that it is time to look back and to critically examine what picture emerges from this wealth of studies. What are the actual types of neurons described in the literature based on electrophysiological criteria? Are these electrophysiologically-defined subpopulations strongly linked to specific morphological, functional, or molecular traits? Are these electrophysiological properties stable, or can they change during development or in response to peripheral injury? Here we provide an in-depth overview of both early and recent publications that explore the factors influencing dorsal horn neuronal excitability (including intrinsic membrane properties and synaptic transmission), how these factors vary across distinct subtypes of dorsal horn neurons, and how such factors are altered by peripheral nerve or tissue damage. The meta-research presented below leads to the conclusion that the dorsal horn is comprised of highly heterogeneous subpopulations in which the observed electrophysiological properties of a given neuron often fail to easily predict other properties such as biochemical phenotype or morphology. This highlights the need for future studies which can more fully interrogate the properties of dorsal horn neurons in a multi-modal manner.
The spinal cord dorsal horn (DH) serves as the initial integration site where somatosensory input is processed by the central nervous system. It is organized into distinct laminae, each associated with specific types of sensory information. Primary afferent fibers (PAFs) that transmit pain, touch, itch, and proprioceptive inputs are categorized into different types (Aα, Aβ, Aδ, and C), each conveying distinct sensory modalities (Figure 1A). In particular, myelinated Aβ and low threshold (LT) Aδ fibers, which mediate innocuous mechanical sensitivity, primarily project to lamina II inner, III, and IV. In contrast, high threshold (HT) myelinated Aδ and unmyelinated C fibers, which mediate pain, thermal sensations, and itch, mainly terminate in lamina I and II (for review see Todd, 2010; Merighi, 2018). Low-threshold mechanoreceptive fibers also include a specific group of C fibers that project to lamina II. These fibers transmit pleasant touch and modulate unpleasant mechanical pain sensations (Liu et al., 2007; Seal et al., 2009). Within DH laminae, PAFs synapse onto both excitatory and inhibitory local interneurons as well as projection neurons, that extend their axons to supraspinal centers. In response to sensory stimulation, DH neurons may generate action potentials (APs) with varying firing patterns. In addition to stimulus-evoked activity, some DH neurons exhibit spontaneous firing. DH neuron excitability is shaped not only by sensory input but also by intrinsic electrical properties.
Figure 1. Spontaneous activity exhibited by dorsal horn neurons. (A) Schematic representation of the spinal cord dorsal horn and the primary afferent projections to the different laminae. (B) Representative example of irregular spontaneous firing recorded in an adult mouse lamina I spinoparabrachial (projection) neuron in an ex vivo spinal cord preparation. (C) Extracellular recordings were obtained in an ex vivo slice from a neonatal mouse using a multielectrode array situated in the superficial laminae of the cord. Action potentials of each individual neuron are represented as vertical lines and include representative examples of 6 neurons with different patterns recorded simultaneously. The right panel shows the area indicated by the asterisk in an expanded time base. Firing patterns include both regular and irregular patterns, with spikes in isolation as well as grouped in burst (RSB, regular slow burst; RFB, regular fast burst; RS, regular simple; ISB, irregular slow bursts; IFB, irregular fast burst; IS, irregular simple firing).
In this review, we provide an overview of early and recent data related to the generation and modulation of DH neuronal activity. In particular, we will review the mechanisms underlying spontaneous and evoked AP firing in DH neurons. Additionally, we will explore the intrinsic membrane properties of specific DH neuron populations and discuss how chronic pain can lead to modifications in neuronal excitability within spinal nociceptive circuits. While dorsal horn neurons can be classified via a multitude of other properties such as their transcriptional profile and functional role in somatosensation, these valid and informative classification approaches nonetheless fall outside the scope of the present review. Instead, we selectively focus on the electrophysiological signatures of dorsal horn neurons, based on the belief that further improving our understanding of the mechanisms regulating DH neuron excitability is essential for comprehending how DH networks operate under normal and pathological conditions.
Several different technical approaches have been used to gather information on the electrical behavior of spinal cord neurons and their sensory coding capabilities. These include a number of different animal preparations, recording techniques and stimulation protocols.
In vivo preparations of cats and rats coupled to extracellular single electrode techniques were commonly used in the past century and produced very useful data regarding how neurons respond to natural stimulation. Single neurons were recorded, one at a time with glass micropipettes or metallic electrodes, and their receptive fields explored. These experiments served to define the main functional types of DH neurons and to explore their general response properties thoroughly. Other in vivo preparations included different methods of spinalization to enable studies on descending modulation and in some exceptional cases, recordings were obtained from awake animals to check the validity of results obtained with anesthetized animals (Herrero et al., 1993). In vivo recordings with intracellular electrodes have been scarcely used due to technical difficulties related to mechanical stability; however, some remarkable studies have been published (Woolf et al., 1988). At present a few laboratories continue to use in vivo preparations with different electrophysiological approaches such as matrixes of electrodes which allow recordings from several neurons simultaneously (Greenspon et al., 2019). This technique opens the door to the study of circuits rather than single neurons.
In vitro preparations using rats or mice started to become popular toward the end of the last century, to solve the stability problem and to facilitate intracellular recordings. Intracellular current and voltage recordings are massively used but other techniques like extracellular electrode matrixes and calcium imaging are commonly reported coupled to in vitro preparations. Meanwhile, technologies based on novel voltage-sensitive dyes and genetically-encoded voltage indicators are currently under development (Hiyoshi et al., 2021). In vitro preparations include cell cultures as well as several ex vivo preparations developed for specific purposes. The entire or hemisected spinal cord preparations enable the use of simple techniques like dorsal root-ventral root recordings that proved very useful for pharmacological studies (Vicente-Baz et al., 2016). In addition, different slicing procedures have also been used to facilitate the access of a variety of electrodes to different laminae of the spinal cord. Besides sharp intracellular electrodes, electrodes for whole cell clamp, patch clamp and perforated patch electrodes are commonly used to study ionic currents and ion channels underlying the electrical behavior of dorsal horn neurons. Specialized preparations like the spinal cord attached to the hindlimb, tail, skin and brainstem have also been reported (King and Lopez-Garcia, 1993; Hachisuka et al., 2016; Lever et al., 2003). Under these in vitro conditions, stimulation protocols often include electrical stimulation of primary afferent pathways, intracellular current injection and more recently, optogenetics. Electrical stimulation of dorsal roots tends to recruit different afferent types in an intensity-dependent fashion so that thick myelinated Aβ fibers are activated by low-intensity currents whereas activation of thin myelinated Aδ and unmyelinated C afferents require progressively stronger stimuli. This procedure is simple and extensively used but does not allow for true selective activation of afferent types. Recently developed optogenetics and chemogenetics open the possibility of stimulating specific primary afferents or specific populations of secondary neurons contributing decisively to clarify the role of specific populations at shaping behavioral responses to somatosensory stimuli (Sharif et al., 2020; Harding et al., 2020; Choi et al., 2020).
Historically, there has been an interest in relating a neuron’s electrical behavior with its morphology, its position within the spinal cord, and its relationship to the rest of the somatosensory system. Intracellular and whole-cell recordings allow for injection of markers like biocytin or lucifer yellow, thereby enabling investigation of cell morphology at the end of an electrophysiological study (Grudt and Perl, 2002). Similarly, the use of genetic engineering in mice allows for the expression of green fluorescent protein or other fluorophores in specific populations of neurons, thereby allowing targeted electrophysiological studies (Hantman et al., 2004). Other imaging techniques include the use of anterograde markers to trace the destination of projecting axons, or retrograde markers to find the somas of neurons projecting to a particular brain region. These techniques have enabled electrophysiological studies of specific projection neurons (Li et al., 2023), which adds tremendous value to experimental observations that historically were performed blind to the identity of the recorded neuron.
Dorsal horn neurons and the central terminals of primary afferents express a wide variety of ion channels representing all the main families reported in the CNS, which are briefly summarized in Table 1. Differences in the array of ion channels expressed by any individual neuron, and the relative balance between them, allow for an enormous variability in the electrical properties of a given neuron.
Table 1. Functional roles of the principal ionic currents expressed by dorsal horn neurons and their modulation in different animal pain models.
Neurons located in the DH express different functional types of voltage-gated sodium currents with a particular spatial distribution. The axon hillock concentrates inactivating sodium channels, with this structure being fundamental for AP generation (Safronov et al., 1997). Tetrodotoxin-sensitive channels containing the Nav1.2 and Nav1.3 isoforms predominate in superficial areas of the DH (Hildebrand et al., 2011). Persistent sodium currents (INa,P) have also been reported and may be important for the integration of synaptic inputs and the generation of spontaneous firing (Prescott and De Koninck, 2005; Li and Baccei, 2011; Lucas-Romero et al., 2018).
Dorsal horn neurons express several types of potassium channels belonging to all known functional classes. Among the voltage-dependent channels, both inactivating and delayed rectifier currents are present in DH neurons (Wolff et al., 1998; Rivera-Arconada and Lopez-Garcia, 2010). For the inactivating type, the contribution of Kv4.2 subunits may be of particular importance in pathological conditions (Hu et al., 2006). The sustained component may involve several different Kv subunits, including delayed rectifier and Kv7/M-currents that can regulate the resting potential and the excitability of DH neurons (Murase et al., 1986; Nowak and Macdonald, 1983; Rivera-Arconada and Lopez-Garcia, 2005). Inward rectifier currents, as well as two-pore domain K+ channels, are also expressed by DH neurons and contribute to leak conductance (Li et al., 2013; Sano et al., 2003). Calcium-activated potassium currents of the SK and BK families are also present in DH neurons and modulate afterhyperpolarization and repetitive firing (Li and Baccei, 2011; Ma et al., 2023; Yang, 2016).
Calcium currents expressed by DH neurons include transient and sustained types of voltage-gated calcium currents with different properties and pharmacology (Huang, 1989; Ryu and Randic, 1990), as well as persistent calcium currents (Prescott and De Koninck, 2005). For example, N-type calcium channels predominate in lamina I neurons, which also express L- and T-type currents (Heinke et al., 2004a). L-type currents are implicated in the acceleration and prolongation of firing upon depolarization in some DH neurons (Morisset and Nagy, 1998). T-type currents participates in burst firing and helps to adjust the firing after hyperpolarization (Sinha et al., 2021; Rivera-Arconada and Lopez-Garcia, 2015). Calcium entry is implicated in spontaneous firing of DH neurons and necessary for the regulation of calcium-dependent currents (Li and Baccei, 2011).
Finally, other ionic currents are present in DH neurons, including the hyperpolarization-activated current (Ih), which is responsible for the anomalous rectification in response to hyperpolarization and helps to set the resting membrane potential and adjust the timing of rebound firing (Rivera-Arconada et al., 2013; Rivera-Arconada and Lopez-Garcia, 2015; Yoshimura and Jessell, 1989a). The calcium-activated non-specific cationic conductance (ICAN) and the non-selective sodium leak channel (NALCN) mediate non-selective currents that are also expressed in DH neurons and may contribute to the regulation of neuronal excitability (Ford et al., 2018; Morisset and Nagy, 1999; Li and Baccei, 2011).
Spontaneous activity (SA) has been documented in both the superficial (Lucas-Romero et al., 2018; Li et al., 2013; Li and Baccei, 2011; Roza et al., 2016; Medrano et al., 2016) and deeper (Fernandes et al., 2018; McGaraughty et al., 2018; Quinn et al., 2010; Monteiro et al., 2006; Jiang et al., 1995) laminae of the DH, where it can be driven by the intrinsic membrane properties of the dorsal horn neurons and/or synaptic inputs activated in the absence of sensory stimulation. For example, 72% of neurons in the deep DH generated spontaneous action potentials triggered by suprathreshold EPSPs, resulting in a firing rate that ranged from 0.2 to 50 Hz (Woolf and King, 1989). While a high prevalence of SA was also observed in deep DH neurons receiving sensory input from the ankle and knee joints (Martindale et al., 2007), other studies reported that SA in the deeper laminae was rare in the absence of injury (Suzuki and Dickenson, 2006; Cata et al., 2006) and occurred at a relatively low (< 2 Hz) frequency (Palecek et al., 1992; Weng et al., 2003; Quinn et al., 2010; McGaraughty et al., 2018). A subset (4%) of deep DH neurons can reportedly undergo a rapid transition between low- and high-frequency modes of spontaneous discharge, which can be triggered by stimulation of their sensory receptive field (Monteiro et al., 2006). In vitro extracellular recordings from the mouse sacral spinal cord also revealed a relationship between the pattern of sensory input-evoked firing and the rate of SA (Thaweerattanasinp et al., 2016). Furthermore, the level of SA in the deep DH can be controlled in a cell type-dependent manner by the tonic release of neuromodulators, as suggested by the finding that blocking nitric oxide synthesis elevates SA in high-threshold mechanoreceptive neurons but not in the low-threshold mechanoreceptive population (Hoheisel et al., 2000).
While additional studies are needed to fully elucidate the degree to which the prevalence and rate of SA differ across distinct laminae of the spinal cord, available evidence suggests a similar frequency of SA between nociceptive neurons in lamina I and lamina V (Eckert et al., 2006). Nonetheless, prior in vivo extracellular recordings suggest that the presence of laminar differences may depend on the functional subtype of DH neuron. Notably, deep DH neurons that respond transiently to colorectal distension (CRD) exhibit a higher rate of spontaneous firing compared to their counterparts located in the superficial laminae, while those neurons that respond in a sustained manner to CRD (or are inhibited by CRD) display similar levels of SA across laminae (Ness and Gebhart, 1989). The use of multielectrode array (MEA) recordings in the rodent DH (Greenspon et al., 2019; Yu et al., 2017; Lucas-Romero et al., 2024) will undoubtedly facilitate a better understanding of the laminar differences in SA occurring within the DH network.
The observed patterns of SA have been variously described using terms which include irregular, regular, clock-like, rhythmic, bursting and pacemaker (Lucas-Romero et al., 2024) (see Figures 1B, C). Irregular fast-burst (IFB) neurons are characterized by brief, high-frequency bursts of action potentials (2–5 spikes at ∼100 Hz) that occur at irregular intervals (Roza et al., 2016). A particular case of the latter pattern is “double-spiking,” in which cells fire two action potentials (APs) with interspike intervals as low as 2 ms (Rojas-Piloni et al., 2007). Other DH neurons exhibit burst-firing at regular intervals and can be classified as either Regular Slow Burst (RSB) neurons, whose intraburst firing is either regular or irregular, or Regular Fast Burst (RFB) neurons which commonly display regular firing within the burst (Lucas-Romero et al., 2018). Pacemaker neurons previously described in the superficial DH (Li et al., 2013; Li and Baccei, 2011) likely correspond to the RSB subtype described above. Meanwhile, regular simple (RS) neurons, also known as clock-like (CL) neurons, discharge single APs at ∼6 Hz with regular interspike intervals (Roza et al., 2016; Luz et al., 2014).
Spontaneous firing can originate from synaptic activity or the intrinsic membrane properties of a given neuron. Notably, while antagonists of fast synaptic transmission (including blockers of AMPA and NMDA subtypes of glutamate receptors) abolish SA within irregular firing neurons, most neurons (∼82%) exhibiting a regular pattern of SA were found to be insensitive to these antagonists (Lucas-Romero et al., 2018; Luz et al., 2014). Similarly, the defining feature of pacemaker neurons is their intrinsic ability to generate rhythmic burst-firing, and therefore such bursting persists following the block of fast synaptic transmission both in the ex vivo spinal cord preparation (Li and Baccei, 2011) and in culture (Legendre et al., 1985). Pacemakers also exhibit a slow depolarization underlying the burst-firing which essentially endows the neuron with a bistable membrane potential (Li and Baccei, 2011) that is reminiscent of a subset of cells in the deep DH (Monteiro et al., 2006; Morisset and Nagy, 1998). Generally, DH neurons displaying SA exhibit a more depolarized resting membrane potential, a more hyperpolarized spike threshold and lower AP duration compared to neurons that lack SA (Lucas-Romero et al., 2018). Furthermore, pacemaker interneurons in the newborn superficial DH are characterized by a lower membrane capacitance and higher membrane resistance compared to adjacent, non-pacemakers in the same ex vivo spinal cord slices (Li and Baccei, 2011).
While SA has been documented in multiple cell types within the DH, including both excitatory and inhibitory interneurons residing in the superficial laminae (Li and Baccei, 2014), emerging evidence supports the existence of cell type-dependent variations in SA within the DH network. For example, within the neonatal spinal cord, SA is more common within lamina I projection neurons targeting the parabrachial nucleus (PB) compared to those innervating the periaqueductal gray (Li and Baccei, 2012), although in vivo recordings suggest that the level of SA within adult spinoparabrachial neurons is low (Bester et al., 2000). In addition, most pacemaker neurons in the newborn superficial DH correspond to glutamatergic interneurons located within lamina I, with an absence of intrinsic burst-firing within lamina II (Li and Baccei, 2011). This observation generally agrees with other work showing that rhythmic SA is more commonly found in local interneurons than spinal projection neurons (Sandkühler and Eblen-Zajjur, 1994; Fernandes et al., 2016). Nonetheless, recordings from lamina I projection neurons in an ex vivo intact spinal cord preparation revealed the existence of pacemaker activity in both the spino-PB and spino-PAG subpopulations (Li et al., 2015).
Interestingly, the ionic mechanisms underlying this intrinsic, rhythmic burst-firing also appear to vary across cell types. In contrast to lamina I interneurons (Li and Baccei, 2011), spinoparabrachial neurons showing pacemaker activity possessed higher membrane capacitance, lower membrane resistance, and a greater inward-rectifying K+ conductance compared to adjacent spinoparabrachial neurons that lacked intrinsic burst-firing (Li and Baccei, 2021). Age is also an important factor shaping the level of SA within the DH network, as the overall prevalence of SA and pacemaker activity is significantly downregulated between postnatal day (P)2 and P9 (Li and Baccei, 2011). Developmental alterations in passive membrane properties likely play a role, since superficial DH neurons show an age-dependent hyperpolarization of the resting potential and reduction in membrane resistance (Walsh et al., 2009). In addition, a developmental increase in the density of the rapid A-type voltage-gated K+ currents has been reported in the DH (Melnick, 2011). Finally, it should be noted that SA can also be synchronized across multiple subgroups of DH neurons, manifested as “population bursts” occurring at irregular intervals (Lucas-Romero et al., 2022), which can span several segmental levels in the lumbosacral spinal cord (Manjarrez et al., 2003).
While the above studies on SA in dorsal horn neurons were predominantly conducted using ex vivo electrophysiological recordings from spinal cord slices, the use of calcium imaging techniques has allowed investigators to document the existence of SA within the in vivo dorsal horn in both anesthetized (Sullivan and Sdrulla, 2022; Johannssen and Helmchen, 2010) and freely behaving (Sekiguchi et al., 2016; Shekhtmeyster et al., 2023) mice. The percentage of dorsal horn neurons exhibiting spontaneous Ca2+ transients in anesthetized mice in vivo is reportedly lower (5%) compared to prior estimates of SA obtained from spinal cord slices (62%; Doolen et al., 2012). The rate of spontaneous firing is significantly reduced by the use of anesthesia, with one study reporting a reduction from 0.52 to 0.08 Hz (Sekiguchi et al., 2016), which occurs in a dose-dependent manner (Sullivan and Sdrulla, 2022). Interestingly, there was no significant difference in the level SA between excitatory and inhibitory dorsal horn neurons regardless of the dose of anesthetic used (Sullivan and Sdrulla, 2022). While technical limitations have generally restricted such analysis to the superficial laminae of the spinal cord, recent methodological advances permitting the imaging of neuronal activity within deeper laminae (Shekhtmeyster et al., 2023) promise to further increase our understanding of the patterns of SA occurring within the complex dorsal horn network under both normal and pathological conditions.
Mounting evidence supports a key role for persistent, voltage-gated Na+ currents (INa,P) in the generation of SA within the DH (Lucas-Romero et al., 2018; Li and Baccei, 2011; Cho et al., 2015). Interestingly, INa,P currents could be facilitated by reductions in external Ca2+ levels during repetitive firing, as previously reported in the ventral horn (Brocard et al., 2013; Tazerart et al., 2008). It is also clear that the level of leak (i.e., voltage-independent) membrane conductance profoundly regulates the level of SA within the DH network. Indeed, a high ratio of INa,P to leak conductance represents a hallmark feature of pacemaker neurons in the DH at P2–P3 (Li and Baccei, 2011). A major contributor to leak membrane conductance is the family of classic inward-rectifying K+ (Kir2) channels (Hibino et al., 2010). Blocking Kir2 channels in the neonatal DH unmasks rhythmic burst firing in ∼42% of non-bursting lamina I neurons (Li et al., 2013) and robustly enhances the firing of multiple subpopulations of lamina I projection neurons (Ford and Baccei, 2016). Activity-dependent elevations in the concentrations of extracellular K+ could also contribute to a reduction in constitutive K+ efflux during rhythmic burst-firing (Brocard et al., 2013). In contrast, tonic Na+ influx through NALCN channels, which are responsible for the vast majority of leak Na+ conductance in CNS neurons (Lu et al., 2007; Shi et al., 2016), constitutively enhances the spontaneous firing of lamina I spinoparabrachial neurons (Ford et al., 2018). A low level of resting Cl– conductance could also facilitate SA in the DH (Keller et al., 2007), which can occur secondarily to weaker tonic synaptic inhibition mediated by GABAAR and glycine receptors (Takazawa and MacDermott, 2010). Furthermore, G protein-coupled inward-rectifying K+ (Kir3) channels (i.e., GIRK channels) are subject to tonic modulation by metabotropic glutamate (mGluR) and GABAB receptors which can modulate endogenous burst-firing in the deep DH (Derjean et al., 2003).
L-type and N-type voltage-gated calcium channels (VGCC) are known to contribute to intrinsic burst-firing in the immature DH (Li and Baccei, 2011). Pacemaker activity can also be facilitated by the Ca2+-activated non-selective cationic current (ICAN) (Li and Baccei, 2011), which does not inactivate in the presence of elevated [Ca2+]int (Partridge et al., 1994) and drives plateau potentials in other CNS neurons (Sheroziya et al., 2009). Meanwhile, although low-threshold T-type VGCC are dispensable for pacemaker activity in the neonatal DH (Li and Baccei, 2011), these channels are important for the generation of rebound depolarizations in deeper laminae (Rivera-Arconada and Lopez-Garcia, 2015). Hyperpolarization-activated cyclic nucleotide-gated (HCN) channels can cooperate with T-type VGCC to accelerate recovery from spike afterhyperpolarization and promote rebound firing (Li and Baccei, 2011; Rivera-Arconada and Lopez-Garcia, 2015) in addition to their contributions to leak conductance (Rivera-Arconada et al., 2013). Finally, multiple subtypes of voltage-gated K+ channels and Ca2+-activated K+ (KCa) channels shape the different components of spontaneous burst-firing, including delayed-rectifier voltage-gated K+ channels which control spike duration and small-conductance KCa (i.e., SK) channels that are involved in burst termination (Li and Baccei, 2011; Legendre et al., 1985).
It is well documented that SA contributes to neuronal survival as well as the formation and refinement of neuronal circuits throughout the CNS during early development (Shatz and Stryker, 1988; Tritsch et al., 2007). In the newborn superficial DH, it has been proposed that pacemaker activity could serve as a surrogate for noxious sensory experience by promoting the activity-dependent wiring of spinal nociceptive networks (Li and Baccei, 2011). The observation that pacemaker interneurons in the superficial DH connect to flexor and extensor motor pathways in the ventral horn (Li et al., 2015) raises the intriguing possibility that pacemakers might provide endogenous excitatory drive to developing sensorimotor networks that underlie nociceptive withdrawal reflexes. Meanwhile, the existence of intrinsic burst-firing in identified spinoparabrachial and spino-periaqueductal gray neurons (Li et al., 2015) suggests that pacemaker activity (or other forms of SA) could also provide endogenous glutamatergic drive to supraspinal pain circuits. Indeed, spontaneous firing in the DH has been linked to the generation of SA in the somatosensory cortex (Manjarrez et al., 2002), and spontaneous neuronal bursts have been observed in the human cortex during the neonatal period (Fabrizi et al., 2011).
Classical studies performed in cats and rats in vivo allowed the study of DH neuron responses to natural stimulation of the skin and deep tissues. An in-depth discussion of this issue can be found elsewhere (Willis and Coggeshall, 2004; Price and Dubner, 1977). Many functional classifications were proposed for DH neurons but perhaps the most popular includes three types based on the nature of the stimulus driving the neuron (Mendell, 1966; Handwerker et al., 1975; Cervero et al., 1976). Low-threshold or type 1 neurons respond to light touch (or the electrical activation of thick myelinated afferents of cutaneous origin) and are found mostly in deep laminae. Wide dynamic range (WDR) or type 2 neurons respond to both touch and pinch of the skin and again are mostly reported in deep laminae but also in superficial LI. Finally, nociceptive-specific or type 3 neurons respond to nociceptive stimuli only (or the activation of nociceptive afferents from the skin, muscle or viscera) and are most commonly found in LI. Low threshold neurons tend to show little spontaneous activity, respond with brief trains of action potentials to natural stimuli such as touch and hair movement and may respond to cooling in the non-nociceptive range (Collins, 1984). Nociceptive specific neurons show also little spontaneous activity responding to intense mechanical stimulation of the skin and a proportion of them to noxious heating with a certain capacity for intensity coding within the nociceptive range (Christensen and Perl, 1970). In contrast, many wide dynamic range neurons tend to show spontaneous activity, non- or slowly-adapting responses to natural stimuli, and their firing frequency encodes stimulus intensity very well (Medrano et al., 2016; Wall et al., 1979). In addition to mechanical sensitivity, many dorsal horn neurons are sensitive to thermal stimuli including cooling and warming as well as noxious cold and heat. Some of these neurons are nociceptive-specific whereas others are of the WDR type (Gieré et al., 2021). Yet another class of neurons reported mainly in LII shows spontaneous activity that was inhibited by natural stimuli (Cervero et al., 1979).
An interesting concept emerging from these studies is that of “convergence,” by which one single DH neuron receives information from many primary afferents. In fact, careful examination of neuronal responses to specific classes of peripheral receptors, has allowed for identification of up to 19 different response profiles in WDR neurons, each one with a specific range of sensitivities (for example hair movement and pinch, or hair movement and pressure) (Heavner and De Jong, 1973). Additionally, intracellular studies demonstrate that neurons may display subthreshold responses to peripheral stimuli, further complicating the basic functional classification built on extracellular data (Woolf et al., 1988). Another derivative of the concept of convergence is that dorsal horn neurons have “receptive fields” with different sizes and shapes, but always larger than those of single afferents. Studies focusing on the properties of the receptive fields of these neurons demonstrated a somatotopic organization as well as the crucial influence of descending modulation which dynamically shapes their boundaries (Wall, 1967).
Intracellular recordings allow for current injection through the electrode in the form of pulses, ramps or sinusoids, which can be used to transiently alter the membrane potential and elicit AP firing. This type of stimulation constitutes a widely used strategy in single neuron studies to test for basic electrophysiological properties (membrane resistance, capacitance, etc.) as well as the intrinsic excitability of neurons, understood as the ability to generate action potentials. Current injection enables the analysis of changes in electrophysiological properties of neurons due to certain treatments or to the application of drugs.
Spinal cord neurons exhibit a wide variety of firing patterns. The most studied region within the spinal cord is probably the superficial DH (laminae I and II) because of its involvement in nociceptive signal processing, and most, if not all, of the documented firing patterns have been reported in neurons located within this area (see, for example, Grudt and Perl, 2002; Ruscheweyh and Sandkühler, 2002; Prescott and De Koninck, 2002). The firing pattern of action potentials in response to depolarizing current pulses has been widely used as a classification system, with responses to hyperpolarizing pulses also considered on occasion (Lopez-Garcia and King, 1994; Graham et al., 2004). A major classifying criterion is firing adaptation during current injection pulses (see Figure 2).
Figure 2. Action potential firing patterns evoked by intracellular current injection. Representative examples of firing patterns recorded from postnatal mouse lamina II neurons in ex vivo slice preparations. Action potentials were evoked by injecting depolarizing current steps using the patch-clamp technique. Initial membrane potentials and current step amplitudes are indicated near each panel.
Tonic neurons are those that maintain the firing throughout the depolarization. Neurons that show firing adaptation with spikes restricted to the first part of the pulse have been referred to as phasic, initial bursting, transient or adapting firing neurons (Grudt and Perl, 2002; Ruscheweyh and Sandkühler, 2002; Prescott and De Koninck, 2002; Melnick et al., 2004a; but see Sinha et al., 2021). Perhaps an extreme case of adaptation could be the single spike pattern, where only one or two action potentials are evoked by depolarization. Temporary refractoriness to fire is the other basic feature for classification. Delayed firing neurons are those that show a delay in firing from pulse onset, gap firing neurons show periods of absence of firing after an initial discharge, and some neurons do not fire at all and are classified as reluctant firing neurons. In addition, some groups have extended the range of firing patterns (Grudt and Perl, 2002; Ruscheweyh et al., 2004) and defined special features associated to depolarization induced firing (e.g., plateaus; Morisset and Nagy, 1999). A more detailed study of firing patterns by analyzing firing latency allowed to Sinha et al. (2021) to separate delayed firing neurons into short and long-latency types. However, for some neurons the classification is not so straightforward. A mixture of firing characteristics can make the classification difficult. For example, some neurons may only fire a burst at the onset of low-amplitude pulses, and show sustained firing at large intensity pulses (e.g., bursting firing pattern from Ruscheweyh et al., 2004; initial bursting firing from Sinha et al., 2021). In addition, the expression of certain firing patterns is very dependent on the membrane potential at the initiation of the pulse (Ruscheweyh and Sandkühler, 2002).
Some studies have shown that excitatory neurons tend to show delayed firing, whereas tonic or initial burst firing is common in inhibitory neurons (Yasaka et al., 2010; Punnakkal et al., 2014; Heinke et al., 2004b). However other work has reported the opposite (Santos et al., 2007). Therefore, an accurate identification of neurotransmitter phenotype may require the analysis of additional features such as morphology (Grudt and Perl, 2002; Yasaka et al., 2010).
The expression of a particular firing pattern is dependent on the array of ionic currents expressed by a neuron (as summarized in Table 1). A small subset of defined ionic currents have been strongly related to the expression of specific firing patterns (Figure 3), but other additional channels are also important for finely shaping the output of a given neuron.
Figure 3. Regulation of the firing patterns by several voltage-dependent currents. Schematic drawing showing the influence of several subthreshold currents on the firing pattern of a model neuron. A tonic firing neuron may become adapting by the action of a slow subthreshold outward potassium current (Ik), and can regain the tonic pattern by persistent sodium currents (INa,p). The transient A-type potassium current (IA) is fundamental for the delayed firing pattern. The Ih may adjust the resting potential and determine the occurrence of pulse sag and rebound depolarization, but its role in defining the firing pattern is minor.
The A-type potassium current (IA) is the dominant conductance in the soma of delayed firing neurons (Melnick, 2011), whereas it is small or absent in tonic neurons (Ruscheweyh and Sandkühler, 2002; Melnick et al., 2004b). Blockade of IA with 4-AP abolishes the delay in firing (Melnick, 2011; Ruscheweyh and Sandkühler, 2002). The presence of IA with different kinetics can produce delayed firing with different latencies (Sinha et al., 2021). A slower IA may be involved in gap firing. In addition, the proportion of reluctant versus delayed firing neurons may change with temperature and this difference may be explained by the sensitivity of IA to the temperature (Graham et al., 2008).
Tonic firing neurons express slow-activating potassium currents of the delayed rectifier type (IKDR) (Melnick et al., 2004b). The Kv7 channel opener retigabine hyperpolarizes deep DH neurons and promotes spike frequency adaptation in tonic neurons (Rivera-Arconada and Lopez-Garcia, 2005). Similarly, the activation of GIRK channels by the μ-opioid receptor in tonic neurons changes the tonic firing pattern to adapting (Santos et al., 2004). Tonic neurons can maintain firing even with a low percentage of inactivating sodium channels available (Melnick et al., 2004b) and also express persistent sodium currents (Prescott and De Koninck, 2005). Blocking persistent sodium currents with riluzole abolishes tonic firing and promotes adaptation (Lucas-Romero et al., 2018). Calcium-dependent potassium channels may also regulate the firing of tonic neurons. Activation of SK channels changes the pattern from tonic to adaptive, whereas its blockade with apamin increases firing frequency (Ma et al., 2023; Yang, 2016; Melnick et al., 2004b; Kim et al., 2008).
Adapting firing neurons may express a lower density of both delayed rectifier potassium currents and inactivating sodium currents compared to tonic neurons, with the latter being a determinant of firing adaptation (Melnick et al., 2004a). T-type calcium currents have also been reported in a proportion of tonic neurons from lamina I and II, although their involvement in this firing pattern is not clear (Prescott and De Koninck, 2002; Wu et al., 2018; Candelas et al., 2019). In hamsters, neurons with strong adapting firing patterns, presumably single spike, showed large T-type currents (Ku and Schneider, 2011). Sinha et al. (2021) showed that initial bursting neurons consistently expressed T-type calcium currents. Hyperpolarization-activated currents (Ih) may also be important for regulating firing. This current is widely expressed in DH neurons and its block can increase input resistance and firing (Lucas-Romero et al., 2018; Rivera-Arconada et al., 2013). The Ih is not specific for any firing pattern, but it has been reported more often in tonic and initial bursting neurons than in neurons displaying firing patterns implying the expression of A-type potassium currents (Hu et al., 2016; Yasaka et al., 2010; Boyle et al., 2019; Hughes et al., 2012; Zhu et al., 2021).
Dorsal horn neurons located in the superficial and deep dorsal horn may also produce voltage-dependent plateau potentials, consisting of prolonged depolarizations observed after the termination of the depolarizing current pulse injection in both tonic firing and adapting neurons (Morisset and Nagy, 1998; Fernandes et al., 2016). Generation of plateau potentials has been primarily linked to the expression of L-type calcium channels (Morisset and Nagy, 1999; Fernandes et al., 2016), together with the ICAN current and Kir channels (Morisset and Nagy, 1999; Derjean et al., 2003). The intense discharges and long-lasting after-discharges associated with plateau potentials represent an important intrinsic mechanism of input-output amplification, involved in the generation of wind-up (Morisset and Nagy, 2000).
The proportion of firing patterns under in vitro and in vivo conditions, as well as across different stages of postnatal development, is highly stable (Walsh et al., 2009; Baccei and Fitzgerald, 2005). The temperature is a factor that affect the kinetic of the ionic channels (for an exhaustive analysis on the effects of temperature on voltage dependent potassium currents see Ranjan et al., 2019), and hence temperature constitutes a potential factor that may influence the expression of firing patterns. Some reports have shown a changes in the distribution of firing patterns at different recording temperatures (Graham et al., 2008; Smith et al., 2015), but changing the temperature during the recording does not seem to affect the firing pattern (Santos et al., 2007), suggesting that differences may be due to a bias toward recording from certain types of neurons. The same is true when considering different orientations of the slices used for ex vivo recordings (Smith et al., 2015).
The firing pattern constitute an important parameter to characterize the excitability of dorsal horn neurons using a simple and reliable method, it is also a useful system for neuronal classification when applied under adequate conditions and considering additional features.
Stimulation of PAFs, either by natural or electrical stimuli, results in the release of excitatory neurotransmitters, primarily glutamate. This, in turn, excites DH neurons, leading to the generation of excitatory postsynaptic potentials (EPSPs). Single or repetitive stimulation of glutamatergic synapses can produce suprathreshold mono- or polysynaptic responses, primarily mediated by AMPA and NMDA receptors. The firing pattern of APs in DH neurons depends on several factors, including the type and number of synaptic inputs, intrinsic properties of the neuron, recruitment of polysynaptic circuits, co-activation of inhibitory inputs, and ongoing neuronal activity.
In most interneurons located in lamina I and outer lamina II, single or repetitive electrical stimulation of nociceptive Aδ and C fibers in ex vivo preparations elicits suprathreshold EPSPs. Firing patterns can range from weak AP discharge to prolonged firing superimposed on slow depolarizations (Jeftinija, 1988; Yoshimura and Jessell, 1989b; Bardoni et al., 2000; see Figure 4). Lamina I projection neurons exhibit diverse responses to PAF stimulation. A study conducted on rat spinoparabrachial neurons identified three distinct groups of cells based on their responses to low-threshold fiber activation: (1) low-output neurons, typically responding with a single spike; (2) medium-output neurons (including both WDR and nociceptive-specific neurons), generating short AP bursts; (3) high-output neurons, firing prolonged AP discharges. Based on these properties, high output neurons, which represent only 20% of the spinoparabrachial neuron population, generate most of the output spiking activity (Agashkov et al., 2019).
Figure 4. Primary afferent-evoked firing in rodent superficial dorsal horn neurons. Examples of action potential discharge in the same projection neuron shown in Figure 1B, in response to increasing intensities of dorsal root stimulation sufficient to recruit low (top panel) and high threshold fibers (center and bottom panels).
Action potential firing can be induced in DH neurons not only by PAF stimulation, but also by activation of neighboring excitatory interneurons. Paired recording experiments in the rat isolated spinal cord have demonstrated that single stimuli delivered to lamina II excitatory interneurons evoke APs in approximately one-third of nearby postsynaptic neurons (Luz et al., 2010). In lamina I, excitatory inputs from neighboring neurons are sufficient to generate single or multiple spikes in about 50% of neurons projecting through the anterolateral system (Luz et al., 2010).
In the deep dorsal horn (laminae III-IV), most neurons receive low-threshold A fiber-mediated monosynaptic inputs, which elicit short bursts of APs. However, subpopulations of these neurons exhibit prolonged EPSPs with repetitive AP, likely due to the activation of polysynaptic circuits (King et al., 1988; Schneider, 2005). WDR neurons of the “antenna type,” located in lamina III and extending their dendrites to both superficial and deep DH, generate short AP bursts in response to low threshold inputs, while high-threshold C fibers evoke sustained firing (Fernandes et al., 2018). Similarly, WDR neurons in lamina V fire either a single spike or brief bursts in response to low threshold inputs, while nociceptive fibers tend to evoke long-lasting AP discharges that outlast the EPSP (Morisset and Nagy, 1998).
Gamma-aminobutyric acid (GABA)- and glycine-mediated inhibitory postsynaptic potentials (IPSPs) are commonly observed in superficial DH neurons following stimulation of PAFs. These IPSPs arise from the activation of polysynaptic circuits involving inhibitory interneurons. In both the rat and mouse, these interneurons make up about 25% of the neurons in laminae I-II and 40% in lamina III (Polgár et al., 2013; Hughes and Todd, 2020). Recent studies have shown that in laminae I-III, about one third of inhibitory interneurons are exclusively GABAergic, while the remaining two-thirds release both GABA and glycine, with GABA being the predominant neurotransmitter. In deeper laminae, most neurons (about 90%) are also both GABA- and glycinergic, with a strong dominance of glycine (Miranda et al., 2022; Miranda et al., 2023).
Depending on the postsynaptic receptor involved (glycine or GABAA), IPSPs can exhibit fast or slow kinetics, respectively. Additionally, their latency can be short or long, in relation to the length of the polysynaptic chain involved (Luz et al., 2014; Yoshimura and Nishi, 1995). PAF-induced IPSPs exert different effects on AP firing: fast and/or short latency IPSPs can shunt monosynaptic EPSPs and limit their ability to generate APs, thereby preventing spike initiation; slow and/or long latency inhibitory responses may effectively prevent long-lasting repetitive firing and/or after-discharges (Yoshimura and Nishi, 1995). In addition to their role in mediating fast synaptic inhibition, GABA and glycine also mediate tonic inhibitory currents in the spinal cord DH (Takahashi et al., 2006; Takazawa and MacDermott, 2010; Gradwell et al., 2017). These tonic inhibitory currents modulate neuronal excitability by altering neuronal input resistance, action potential threshold, firing pattern, and input/output gain (Semyanov et al., 2004). Indeed, blocking GABA and/or glycine receptors in inhibitory interneurons has been shown to alter neuronal excitability by enhancing action potential discharge (Takazawa and MacDermott, 2010; Gradwell et al., 2017).
The degree of inhibition onto the postsynaptic neuron plays a crucial role in determining the involvement of specific glutamatergic receptors in EPSPs and subsequent firing. Fast EPSPs, evoked by single stimuli, primarily rely on AMPA receptors, including both Ca2+-permeable and Ca2+-impermeable subtypes (Luz et al., 2010; Tong and MacDermott, 2006; Yoshimura and Jessell, 1990; Santos et al., 2009). NMDA receptors can be recruited during repetitive stimulation of presynaptic inputs and prolonged postsynaptic discharges (Bardoni et al., 2000; Agashkov et al., 2019). The removal of inhibition through GABAA and glycine receptor antagonists enhances the depolarization of lamina I and II neurons, leading to more robust activation of NMDA receptors. Consequently, this promotes the temporal summation of EPSPs, facilitates the generation of prolonged AP discharges, and activates reverberating polysynaptic circuits (Bardoni et al., 2000; Agashkov et al., 2019; Torsney and MacDermott, 2006).
In deep DH neurons, stimulation of low threshold PAFs results in inhibitory responses with variable latencies (Schneider, 2005). In a manner similar to what has been observed in the superficial DH, the removal of postsynaptic inhibition also increases neuronal excitability in laminae III-IV and promotes synaptic summation during repetitive stimulation (Betelli et al., 2015). In the same DH region, presynaptic GABAA receptors expressed on A fiber terminals regulate afferent-induced firing by decreasing the number of APs evoked during repetitive A fiber stimulation (Betelli et al., 2015).
Firing pattern analysis has been used as a simple method to infer how a given neuron might respond to a synaptic input, even though this stimulation does not reproduce the temporal characteristics of a real synaptic response (Graham et al., 2007). In an ex vivo preparation of the adult rat spinal cord, A-fiber electrical stimulation evoked APs only in tonic firing neurons (Thomson et al., 1989). In the hemisected cord-hindlimb preparation, WDR neurons often exhibited a tonic firing pattern in response to current injection, whereas neurons specific to tactile or nociceptive inputs displayed phasic patterns (Lopez-Garcia and King, 1994). In the whole spinal cord preparation, neurons firing with a phasic or single spike pattern responded to afferent stimulation with either a single spike or a short burst of APs. In contrast, tonic cells were more likely to generate prolonged EPSPs and sustained AP discharges (Fernandes et al., 2016; Agashkov et al., 2019; Dougherty and Chen, 2016). From a functional perspective, tonic-firing neurons that respond to PAF stimulation with prolonged EPSPs and firing could operate as integrators of synaptic inputs, faithfully transmitting sensory inputs in terms of duration and intensity. In contrast, rapidly adapting phasic neurons are optimally excited by short stimuli and do not rely on temporal summation for spike generation. Lastly, single spike neurons demonstrate the ability to follow high frequency trains, acting as precise coincidence detectors (Prescott and De Koninck, 2002).
Voltage-dependent ionic currents significantly influence the response to synaptic inputs in both excitatory and inhibitory interneurons. The A-type potassium current (IA) has been extensively studied in this context (reviewed in Clerc and Moqrich, 2022). Research on DH GAD67-GFP + (inhibitory) and GFP- (excitatory) interneurons in mouse spinal cord slices revealed that IA densities are higher in GFP- cells compared to GFP + cells (Yoo et al., 2021). In excitatory neurons, a large IA is necessary to reduce excitability under naïve conditions, whereas a small IA in inhibitory interneurons leads to high excitability, aligning with the tonic firing pattern prevalent in these neurons (as described in section “8 Firing properties of identified subpopulations of dorsal horn neurons” and Table 2). In subpopulations of nociceptive excitatory interneurons, IA prevents action potentials triggered by Aβ fiber stimulation in these neurons (Zhang et al., 2018). Blocking IA with intracellular 4-aminopyridine permits Aβ fiber-mediated firing in nociceptive interneurons, resulting in the condition of allodynia (Zhang et al., 2018).
Table 2. Electrophysiological profiles observed in subpopulations of dorsal horn interneurons as identified by molecular markers.
Finally, the processing of sensory transmission in the superficial DH can be significantly influenced by the ability of a subset of neurons to generate spontaneous intrinsic burst-firing. Recent work has demonstrated that adult mouse spinoparabrachial neurons displaying spontaneous burst-firing also exhibit higher levels of primary afferent-evoked AP discharge compared to those lacking such activity (Li et al., 2023). Additionally, the magnitude of afferent-evoked AP firing significantly correlates with the resting potential and membrane resistance, rather than the overall level of glutamatergic synaptic drive. This finding confirms the importance of the intrinsic membrane properties of DH neurons in shaping the input-output relationship at sensory synapses. In the deep DH, spontaneous bursting capability is associated with accelerated firing rates and enhanced spike afterdischarges (Morisset and Nagy, 1998; Russo and Hounsgaard, 1996). Nonetheless, the effect of spontaneous burst-firing on the gain of nociceptive signaling in the DH is likely to depend on the timing of the afferent input in relation to the phase of spontaneous activity, as pacemaker activity can filter out sensory inputs arriving during the generation of a spontaneous burst (Li and Baccei, 2011).
Repetitive low-frequency stimulation of C-fiber inputs induces short-term synaptic facilitation, resulting in a progressive increase of the number of APs evoked by each stimulus, which eventually results in a prolonged after-discharge. This phenomenon, termed wind-up, was originally observed in lamina IV spinocervical neurons in vivo (Mendell and Wall, 1965; Mendell, 1966). Since then, numerous studies, both in vitro and in vivo, have characterized wind-up in various spinal neurons, including those in the superficial DH, deep DH, and motoneurons (for reviews, see Baranauskas and Nistri, 1998; Herrero et al., 2000). Experiments performed ex vivo on rat spinal cord have demonstrated that low-frequency activation of C fibers (0.2–2 Hz) is essential for inducing wind-up in DH neurons (Zieglgänsberger and Sutor, 1983; Jeftinija and Urban, 1994; Morisset and Nagy, 2000). In lamina I spinoparabrachial neurons, a subpopulation of excitatory interneurons contributes to wind-up by promoting reverberating network activity (Hachisuka et al., 2018). Under physiological conditions, synaptic inhibition reduces the activity of these polysynaptic circuits.
The generation of plateau potentials has been linked to the expression of wind-up in adult rats in vivo (Reali et al., 2011). Accordingly, wind-up is suppressed by blockers of L-type voltage-dependent calcium channels and ICAN, the two main depolarizing currents underlying plateau potentials (Morisset and Nagy, 2000; Fossat et al., 2007). Wind-up also involves a synaptic component, including both NMDA (Jeftinija and Urban, 1994; Dickenson and Sullivan, 1987; Woolf and Thompson, 1991) and tackykinin receptors (Baranauskas et al., 1995).
The extensive heterogeneity of firing patterns observed in DH neurons poses an obstacle to the use of this parameter to rigorously identify the neurotransmitter phenotype (i.e., glutamatergic vs. GABAergic) of the sampled neuron. The strongest association demonstrated to date is that inhibitory DH neurons commonly display a tonic pattern of repetitive AP discharge in response to prolonged depolarization, with no delay in the onset of firing and little evidence of spike frequency adaption or the expression of hyperpolarization-activated cation currents reported in the rat DH (Yasaka et al., 2010; Maxwell et al., 2007). Similarly, studies recording from GABAergic neurons in the immature mouse DH (identified via GAD67-EGFP expression) consistently reported a predominance of tonic firing within this population, with delayed and phasic patterns of discharge also observed to a lower extent (Takazawa and MacDermott, 2010; Punnakkal et al., 2014; Daniele and MacDermott, 2009). Meanwhile, GAD67-EGFP neurons in the adult DH commonly exhibited an initial bursting pattern of repetitive firing with a lower prevalence of tonic and gap firing observed (Heinke et al., 2004b), suggesting the possibility that age-dependent changes in the intrinsic firing properties of spinal GABAergic neurons may occur during later stages of development.
The close relationship between GABAergic neurons and tonic firing also appears to extend to the deep DH (Punnakkal et al., 2014; Gassner et al., 2013; Cui et al., 2016). In addition, glycinergic neurons residing in both the superficial and deep laminae of the DH (identified via the GlyT2 promoter) mostly show tonic firing (∼80%) with phasic discharge also reported (Punnakkal et al., 2014; He et al., 2021). In contrast, glutamatergic neurons in the DH (often identified via the expression of VGLUT2) commonly display a delayed firing phenotype, with gap and reluctant firing patterns also reported in this population, along with strong spike frequency adaptation (Yasaka et al., 2010; Maxwell et al., 2007; Yasaka et al., 2014). The prevalence of reluctant firing neurons within the population of excitatory neurons is in general agreement with prior observations that glutamatergic neurons generally possess higher rheobase levels and lower repetitive firing frequencies compared to inhibitory neurons in the DH (Punnakkal et al., 2014).
Firing patterns have also been shown to vary across different morphological subtypes of DH neurons, identified according to the location, size of the soma, and dendritic and axonal arborizations (Grudt and Perl, 2002). Islet cells commonly display tonic firing in response to persistent depolarization, which might be expected since they are predominantly inhibitory in nature (Yasaka et al., 2010; Maxwell et al., 2007). The relatively smaller subsets of GABAergic neurons showing other morphologies (such as radial and vertical) reportedly exhibit more variable firing properties (Maxwell et al., 2007). Meanwhile, other studies have demonstrated an association between tonic firing and a fusiform dendritic morphology, while pyramidal neurons in the DH often display phasic discharge and multipolar neurons exhibit both delayed firing and single-spiking patterns of repetitive firing (Prescott and De Koninck, 2002). Finally, vertical neurons (which include both excitatory and inhibitory subpopulations) commonly show delayed firing patterns, while transient central cells in the DH have been suggested to fire in a phasic manner (Yasaka et al., 2010; Yasaka et al., 2014; Lu and Perl, 2005). Little is known regarding the degree to which the firing properties of a given morphological cell type varies depending on laminar location within the DH.
Glutamatergic neurons can be classified into distinct subpopulations based on their expression of biochemical markers such as neuropeptides (for review see Peirs et al., 2020), and the proliferation of available Cre mouse lines in recent years has afforded the opportunity to characterize the intrinsic firing properties of these discrete (albeit overlapping) subtypes of excitatory DH neurons (see Table 2 and Figure 5). For example, somatostatin-lineage neurons in the DH, which have been linked to the transmission of mechanical pain (Duan et al., 2014), predominantly show a delayed firing pattern (Yasaka et al., 2010) and discharge action potentials in response to Aβ-fiber or Aδ/C-fiber input depending on their dorsoventral location (Duan et al., 2014). Excitatory neurons expressing the Y1 receptor for neuropeptide Y (NPY), which have been implicated in neuropathic pain (Nelson et al., 2023), also predominantly show a delayed onset of firing in response to prolonged depolarization, which is accompanied by high expression of A-type voltage-gated K+ currents (Sinha et al., 2021). These cells also commonly exhibit rebound firing upon termination of membrane hyperpolarization (Sinha et al., 2021). Similarly, a high percentage (∼79%) of substance P-expressing DH neurons showed delayed firing and, as might be expected, expression of A-type K+ currents (Dickie et al., 2019). The calretinin-positive subpopulation of mouse DH neurons, which amplify nociceptive signaling in the DH and directly excite ascending projection neurons (Smith et al., 2019), also mostly showed a delayed firing pattern and large A-type K+ currents, with tonic and initial bursting patterns of repetitive firing also observed which may correspond to the small percentage (∼12%) of calretinin neurons that are predicted to be GABAergic (Smith et al., 2015). It should be noted that the calretinin population is not unique in being comprised of both excitatory and inhibitory interneurons, as this has also been observed in other neuronal populations including (but not limited to) those expressing nNOS, dynorphin and galanin (Sardella et al., 2011; Duan et al., 2014; Abraira et al., 2017). This complexity must be considered when attempting to associate biochemically- or genetically-identified subpopulations of DH neurons with a specific neurotransmitter phenotype.
Figure 5. Localization of specific classes of neurons in the dorsal horn. Schematic diagram illustrating the localization of various neuronal classes described in section “8 Firing properties of identified subpopulations of dorsal horn neurons” and in Table 2 (refer to this table for abbreviations and references). Arrows indicate the regions where different neurons have been detected. The same class has been reported twice if two distinct subpopulations have been identified for the same neuron type, based on the their morphology and functional properties.
Meanwhile, DH neurons expressing gastrin-releasing peptide (GRP), which have been implicated in spinal pruriceptive transmission, commonly display transient (∼50%) or single-spiking (∼33%) firing patterns (Dickie et al., 2019). Cells expressing the GRP receptor GRPR, which represent a subset of the postsynaptic targets of GRP-expressing neurons, also show a high prevalence of delayed firing (56%) with a sizeable number of cells displaying tonic (23%) and phasic (15%) firing also reported (Bardoni et al., 2019). GRPR+ neurons have also been shown to exhibit a high prevalence of single-spiking and correspond to vertical cells in terms of their morphology (Polgár et al., 2023). Another subpopulation of glutamatergic neurons implicated in itch signaling expresses the receptor for neuromedin B (NMBR) and features comparable numbers of delayed and phasic firing neurons (Wan et al., 2017). Finally, neurons expressing the tachykinin 2 (Tac2) gene (encoding neurokinin B) show a mix of delayed, tonic, initial burst and phasic firing within lamina II of the mouse DH, although Tac2+ cells in lamina III are nearly all phasic (Chen et al., 2020).
Like their glutamatergic counterparts, GABAergic neurons within the spinal DH can also be subdivided based on their expression of distinct biochemical markers, as summarized in Table 2 (for review see Peirs et al., 2020). Neurons derived from the prodynorphin (DYN) lineage, which suppress mechanical pain and itch (Duan et al., 2014; Kardon et al., 2014), predominantly exhibit tonic or delayed firing within the adult (P49-P63) superficial DH, while these cells show a high prevalence of phasic discharge during the neonatal period (P6-P7) (Brewer et al., 2020), thus highlighting the importance of considering age when attempting to relate the repetitive firing patterns to neurotransmitter phenotype. Meanwhile, parvalbumin-lineage neurons, which are important for the inhibition of mechanical sensitivity (Qiu et al., 2024), commonly exhibit islet or central morphology and reside in laminae IIinner – III, were found to be mostly tonic firing with some initial bursting also reported with a high incidence of Ih (Boyle et al., 2019; Gradwell et al., 2022a; Gradwell et al., 2022b). Interestingly, parvalbumin DH neurons can switch from tonic to phasic firing (with increased spike frequency adaptation) under neuropathic conditions, which likely contributes to the overall hyperexcitability of the spinal nociceptive network after peripheral nerve damage (Ma et al., 2023).
The subpopulations of neurons expressing nitric oxide synthase (nNOS) or galanin (identified via the expression of the prion promoter; PrP) predominantly showed tonic firing (∼72%) with initial burst-firing the next most prevalent pattern observed (Ganley et al., 2015). As expected from their GABAergic nature, cholinergic DH neurons (which partially overlap with the nNOS+ population) mostly exhibited tonic AP discharge with strong rebound firing (Mesnage et al., 2011). Similarly, genetic strategies that permitted the selective recording from the inhibitory subset of calretinin neurons in the DH showed these were overwhelmingly islet cells that fired in a tonic manner (Davis et al., 2023).
Despite their glutamatergic nature, extrapolating the above findings to the output neurons of the spinal nociceptive circuit is difficult given that projection neurons comprise less than 5% of the overall neuronal population within the spinal DH (Spike et al., 2003; Cameron et al., 2015) and thus are commonly identified via retrograde labeling from the brain, although the recent development of Phox2a-Cre mice allows for the genetic identification of a subset of ascending spinal projection neurons (Roome et al., 2020). Rat spinoparabrachial and spino-PAG neurons show almost exclusively tonic firing during early life (i.e., P2–P5) but phasic, delayed and bursting patterns of repetitive discharge become evident by adolescence (P30–P32) (Li and Baccei, 2012). Other studies conducted in the rat DH reported that the gap and bursting firing patterns were mostly restricted to projection neurons (as opposed to unidentified DH neurons) and were commonly found in the spinoparabrachial and spino-PAG populations, respectively (Ruscheweyh et al., 2004). The gap and bursting firing patterns were attributed to the expression of A-type K+ currents and T-type voltage-gated Ca2+ currents, respectively (Ruscheweyh et al., 2004). Meanwhile, adult mouse spino-PAG neurons show spontaneous burst-firing (as well as irregular discharge) and predominantly displayed tonic or initial burst patterns of firing in response to prolonged current injection, with spontaneously bursting cells also showing high levels of primary afferent-evoked firing (Li et al., 2023).
Other studies revealed that mouse spinoparabrachial neurons can be clustered into four distinct subpopulations based on their electrophysiological properties, and those neurons located in the deep DH (i.e., laminae III-V) exhibited higher repetitive firing frequencies compared to the lamina I spinoparabrachial population (Browne et al., 2021). In comparison to interneurons in the DH, projection neurons are reportedly more excitable compared to unidentified cells (> 95% of which are expected to correspond to interneurons) which showed mostly tonic firing (Graham et al., 2008).
There is mounting evidence indicating that peripheral lesions can change the electrical behavior of DH neurons. Peripheral lesions may affect the skin, muscles, joints or viscera leading to inflammation as well as lesions affecting sensory nerves that lead to neuropathy. Central changes in nociceptive processing are usually referred to as central sensitization (Woolf, 1983), a phenomenon different from (and dependent on) peripheral sensitization. Central sensitization occurs as a consequence of the arrival of a sustained barrage of action potentials to the spinal cord and the brain through nociceptive pathways.
Many observations using different preclinical models have established that nociceptive DH neurons, particularly wide dynamic range neurons, can increase their spontaneous firing, responses to natural stimuli and the size of their receptive fields under conditions of persistent pain. For example, a recent study using a model of chemotherapy-induced neuropathic pain shows that WDR neurons acquire spontaneous activity and a wider sensitivity to stimuli of different modalities (Meesawatsom et al., 2020). In addition, some of these neurons generate post-discharges to innocuous stimuli. Similarly, observations from a model of joint inflammation show that DH neurons enhance their preexisting responses, lower their response threshold, and expand their receptive fields, responding even to movement in the contralateral joint (Neugebauer and Schaible, 1990). The behavior of high-threshold neurons is also affected by peripheral lesions in similar ways (Hylden et al., 1989). It is interesting to note that different pain models tend to produce central changes with particular traits (Zain and Bonin, 2019). Different mechanisms are under discussion to explain changes in the DH that follow a peripheral injury. At the cellular level, these mechanisms can be reduced to synaptic plasticity and alterations in intrinsic membrane excitability (see Figure 6). Synaptic and intrinsic mechanisms are not mutually exclusive, and in fact may interact to generate the final hyperalgesic phenotype (Rivera-Arconada and Lopez-Garcia, 2010).
Figure 6. Modifications of synaptic transmission following peripheral injury. Cartoon depicting synaptic changes in dorsal horn occurring following peripheral injury. Role of descending system excluded for clarity. See main text for details. (1). Enhancement of presynaptic primary afferent depolarization and subsequent firing in orthodromic- and antidromic directions plus facilitation of conduction across branching points (arrows); (2). Presynaptic release of mediators by afferents following an NGF phenotipic change of primary afferents (Substance P, BDNF, ATP, CCL2, CSF1); (3). Enhancement of excitatory aminoacid receptor function (AMPAR and/or NMDAR following activation of other receptors (Neurokinin receptors and others). (4). Neuron-glia interactions: Microglia activation by inflammatory mediators (prostaglandins) and mediators released from primary afferents (ATP, CCL2, CSF1). (5). Modulators from glia and primary afferents (like BDNF) or byproducts of inflammation (Prostaglandins) act on second order dorsal horn neurons to depress or alter inhibitory transmission.
The synapses between primary afferents and DH neurons have been under intense scrutiny because they are the first relay of sensory information. These synapses can be regulated at the presynaptic level to modulate neurotransmitter release from primary afferents or at the postsynaptic level to modulate receptor sensitivity.
A presynaptic mechanism discussed at large in the literature is that of primary afferent depolarization (PAD). PAD was first described as a physiological means to produce presynaptic inhibition in muscle afferents (Eccles et al., 1962). PAD is produced by release of GABA and subsequent depolarization due to the outward movement of chloride from the afferent terminals which contain relatively high internal Cl– levels. Inflammatory stimuli can enhance PAD to reach firing threshold, thereby converting an inhibitory mechanism to an excitatory one (Pitcher and Cervero, 2010). Action potentials generated at central branches of the afferents are then transmitted antidromically to contribute to peripheral neurogenic inflammation and orthodromically to produce allodynia (Willis, 1999). Recent data suggest that PAD may also serve to facilitate AP conduction through branching points at central terminals of primary afferents (Hari et al., 2022). Thus, inflammation-induced enhancement of PAD could lead to increased excitation in the DH and enlargement of receptive fields.
Experimental evidence shows that synchronous firing of DH neurons associated with activity in primary afferents increases significantly after inflammation (Lucas-Romero et al., 2022). Interestingly, population bursts in the DH (i.e., the synchronous firing of multiple neurons) have been related to the generation of PAD and the backfiring of sensory neurons (Lucas-Romero et al., 2022), which are important for gating sensory inputs in the spinal cord (Boyle et al., 2019) as well as promoting the peripheral release of neuropeptides and subsequent neurogenic inflammation (Willis, 1999). Other presynaptic mechanisms include phenotypic changes in primary afferents. Substance P release may be increased under inflammatory conditions as a result of peripheral NGF-induced phenotypic modification of primary afferents (Woolf, 1996). Other phenotypic changes, associated mainly with neuropathy, have been reported to include the release of CCL2 and CSF1 by primary afferents (Gosselin et al., 2005).
Amino acid-mediated transmission has been intensively studied in connection with central sensitization. Changes in NMDA receptors on their own, or in combination with changes in subunit composition of AMPA receptors, may work as final effectors to increase excitability of DH neurons in inflamed animals by increasing postsynaptic glutamate-induced depolarizations and allowing calcium entry into neurons that promote further changes in excitability (Park et al., 2009). Many other molecules may modulate excitability of DH neurons through interaction with glutamate receptors. For example, dynorphin, substance P and CGRP released from nociceptive primary afferents may trigger intracellular signals that impinge on NMDA receptors to generate hyperexcitability of DH neurons (Dubner and Ruda, 1992; Bird et al., 2006).
Inhibitory synapses mediated by GABA and glycine are also subjected to plasticity during inflammatory and neuropathic conditions. Key mediators of inflammation like prostaglandin E2 have been shown to increase excitatory transmission and block inhibitory transmission mediated by glycine in DH neurons (Zeilhofer, 2008). A loss of inhibition through GABAergic synapses has been reported in models of neuropathic pain as well (Coull et al., 2003). This mechanism involves downregulation of KCC2 in superficial dorsal horn neurons which in turn causes a shift in the reversal potential of chloride ions such that GABA can have a depolarizing effect. A decrease of the spinal inhibitory tone, which frequently occurs following nerve injury, could enhance the activity of the excitatory network, giving rise to the abnormally elevated wind-up that is observed in some pathological conditions (Herrero et al., 2000).
Descending systems can release serotonin, noradrenaline and GABA from axons originating in brainstem nuclei. Monoamines interact with a variety of membrane receptors located at central terminals of primary afferents and/or DH neurons to produce either inhibitory or excitatory effects on nociceptive transmission via presynaptic and postsynaptic actions (Lopez-Garcia, 2006). Following peripheral inflammation, excitatory effects may be increased (Carr et al., 2014) and/or inhibitory effects may be decreased (Cervero et al., 1991) to cause an imbalance between excitation and inhibition.
Neuroinflammation and the intervention of glial cells constitutes a novel player in central sensitization related to both inflammatory and neuropathic pain as well as diffuse chronic pain syndromes similar to fibromyalgia. CCL2 and CSF1 together with ATP released from primary afferents activate microglia which in turn release proinflammatory interleukins and probably BDNF (Gosselin et al., 2005; Denk et al., 2016). BDNF released from microglia or primary afferent neurons has been shown to increase NMDA-mediated responses (Kerr et al., 1999), induce the anion shift in dorsal horn neurons (Coull et al., 2005), evoke micro-structural changes in synaptic elements (Bareyre et al., 2002) and promote other complex changes in neuronal metabolism (Pezet et al., 2002) which may contribute to the maintenance of hyperalgesia.
Prior studies comparing the basic electrophysiological properties of spinal neurons in naïve (or sham) vs. neuropathic animals reported no differences in resting potential, membrane resistance or in the proportion of neurons with different firing patterns (Gassner et al., 2013; Balasubramanyan et al., 2006; Schoffnegger et al., 2006). However, recent work reveals subtle changes in the excitability of inhibitory parvalbumin neurons in the DH. Tonic-firing parvalbumin neurons located ipsilateral to the lesion may require a higher current amplitude to maintain tonic firing and produce a lower firing frequency compared to neurons on the contralateral side in response to the same current injection (Boyle et al., 2019). Alternatively, these neurons may undergo a change in firing pattern from tonic to adaptive after nerve lesion (Ma et al., 2023). These differences in excitability could be relevant for the expression of allodynia after neuropathy. In this line, the neonatal treatment with the chemotherapeutic drug vincristine enhance the excitability of spinoparabrachial neurons located in lamina I (Schappacher et al., 2019). However, in a model of chronic spinal cord injury, inhibitory lamina I neurons with tonic and initial burst patterns show higher excitability as demonstrated by a depolarized membrane potential, lower rheobase and higher firing frequency. This mechanism may serve as a compensatory homeostatic response to the state of hyperexcitability in DH circuits (Dougherty and Hochman, 2008).
Changes suggestive of a reduced excitability of spinal neurons have also been reported in different animal models of pain (Li and Baccei, 2014; Farrell et al., 2017). Certain types of excitatory and inhibitory neurons show a lower membrane resistance and more hyperpolarized resting potentials, together with reduced firing frequency, in adult animals subjected to paw incision at 3 days after birth (Li and Baccei, 2014). In a model of colitis, more hyperpolarized membrane potentials and an alteration in AP firing adaptation have been described in certain DH neurons (Farrell et al., 2017). In a model of paw inflammation, an increase in excitability in the early stages of the inflammatory process was followed by a decrease in the longer term (Rivera-Arconada and Lopez-Garcia, 2010). In a recent report, the axon initial segment has been shown to shift distally away from the soma in inhibitory neurons after peripheral inflammation, which could be responsible for decreased excitability (Caspi et al., 2023).
Most of the studies highlighted above show that the excitability of spinal neurons may be altered in specific cell types during pathological processes. The mechanisms responsible for these changes are not fully understood, although changes in the expression of genes encoding sodium, calcium and potassium channels have been shown under pain states (Yang et al., 2004) (see Table 1). An increase in delayed rectifier currents could be responsible for a reduction in firing frequency during inflammation (Rivera-Arconada and Lopez-Garcia, 2010). Transient potassium currents (A-type current) may also be important in models of inflammation. Alterations in the voltage-dependent inactivation of this current may contribute to increased excitability in the early stages of an inflammatory process (Rivera-Arconada and Lopez-Garcia, 2010). Following capsaicin injection, this current may be reduced, allowing normally subthreshold inputs from Aβ fibers to reach firing threshold in neurons located in the superficial DH (Zhang et al., 2018). Interestingly, an enhancement of A-current may serve as a compensatory mechanism to reduce excitability once potentiation of synaptic strength has been established following the lesion (Tadros et al., 2018). Inward-rectifier potassium currents can also be altered after a neonatal insult thereby decreasing spinal neuronal excitability (Li and Baccei, 2014). Other potassium channels may also change their expression or modulation in models of neuropathy and inflammation (BK: Chen et al., 2009; Kir3: Ippolito et al., 2005), but in other studies no changes have been found (Mongan et al., 2005).
Regarding voltage-gated sodium channels, changes in the expression of alpha and beta subunits have been shown in models of neuropathy (Blackburn-Munro and Fleetwood-Walker, 1999; Hains et al., 2004; Lampert et al., 2006). Leak sodium channels are increased in both inflammatory and neuropathic models and are important regulators of membrane potential and excitability (Zhang et al., 2021; Li and Baccei, 2021). Meanwhile, increased activity of L- and N-type calcium channels has been reported in an animal model of streptozotocin-induced diabetes (Voitenko et al., 2000). In models of neuropathy caused by nerve injury, Cav1.2 and Cav1.3 channels (L-type) are up- and downregulated, respectively (Dobremez et al., 2005). These changes can be related to an increase in the expression of plateau potentials, but they may also be involved in both the reduction of wind-up in wide dynamic range neurons due to the decrease in Cav1.3 channels and the hyperexcitability of spinal neurons due to the increase in Cav1.2 (Reali et al., 2011; Radwani et al., 2016). Alterations in N-type channels may be involved in sensitization at the presynaptic level, with both increases and decreases described depending on the injury model (Cizkova et al., 2002; Rycroft et al., 2007). Changes in the expression of the α2/δ1 regulatory subunit have also been described and may contribute to increased calcium currents in models of peripheral neuropathy (Li et al., 2004; Alles et al., 2018) and chemotherapy-induced neuropathic pain (Chen et al., 2019; Xiao et al., 2007; Gauchan et al., 2009). Finally, peripheral lesion has been shown to regulate expression of several other channels. For example, the CNGA3 subunit of cyclic nucleotide-gated channels (Heine et al., 2011), HCN2 channels (Liu et al., 2018) and acid-sensing ion channels (Wu et al., 2004; Duan et al., 2007) are upregulated in DH neurons and their contribution to nociceptive processing is under investigation.
The modulation of ion channel expression provides a powerful mechanism to regulate the excitability of DH neurons by adjusting their input-output relationship. Changes directed to increase the excitability have been reported, but homeostatic compensation may also have a profound influence on the processing of nociceptive information.
In this review, we have described a wide range of activity patterns associated with both spontaneous and evoked action potential firing in the superficial and deep DH. This wide heterogeneity likely reflects the large variety of neuronal subtypes present in the DH.
A molecular characterization of the different DH populations has recently been provided by several studies employing RNA sequencing along with other molecular and functional approaches. By using the single-cell RNA sequencing technique, Häring et al. (2018) identified 15 excitatory and 15 inhibitory molecular subtypes located in the mouse DH. More recently, using single-nucleus RNA sequencing (snRNA-seq), five distinct populations of projection neurons belonging to the anterolateral system have been described (Bell et al., 2024). These neuronal clusters were found in specific locations within the mouse DH laminae and exhibited different functional roles in processing pain, itch, and thermal stimuli. Similarly, the use of snRNA-seq, spatial transcriptomics, and immunohistochemistry in the adult human spinal cord has enabled the identification of 64 different cell clusters (29 of glial cells and 35 of neurons), organized primarily by anatomical location (Yadav et al., 2023).
Hierarchical cluster analysis combining electrophysiological and morphological properties has revealed the presence of 5 relatively distinct clusters in the mouse superficial DH: two groups of excitatory interneurons and three groups of inhibitory interneurons (Browne et al., 2020). Interestingly, electrophysiological properties more effectively distinguished excitatory and inhibitory phenotypes than morphological-based clustering, showing that inhibitory interneurons are generally more excitable, tend to fire in a tonic pattern, and have a weak excitatory drive. Conversely, excitatory interneurons are typically less excitable, commonly exhibit delayed and phasic firing profiles, and are subject to a strong excitatory synaptic drive.
The molecular characterization of DH neurons, combined with the study of animal behavior, provide important insights for targeting specific neuronal populations and understanding their functional role in sensory processing. However, this classification alone is insufficient to differentiate the numerous subtypes of DH neurons. Given that electrophysiological, morphological, functional (excitatory vs inhibitory; interneuron vs. projection neuron), and molecular traits often do not cluster together, and that none of these single properties can predict the others within a single neuron, more complex analytic procedures should be employed. These procedures should include a comprehensive collection of electrophysiological characteristics, identification of morphological and molecular traits, analysis of excitatory and inhibitory inputs, assessment of neuronal connectivity, and evaluation of the functional role of the neuron in nociceptive behavior. The Patch-seq technique, which integrates electrophysiological, transcriptomic, and morphological characterization of individual neurons, could provide significant insights into understanding the functional properties of DH neurons. Moreover, to classify the subpopulations of DH neurons that play unique roles in somatosensation, the analysis should also consider other important variables, such as animal species, age, sex, and the specific pain models employed. Taking all these factors into account, it becomes clear that each DH neuron operates as an individual entity, adjusting and modulating its activity in response to the functional state of the DH network.
IR-A: Writing – original draft, Writing – review and editing. MB: Writing – original draft, Writing – review and editing. JL-G: Writing – original draft, Writing – review and editing. RB: Writing – original draft, Writing – review and editing.
The author(s) declare that financial support was received for the research and/or publication of this article. This work was funded by Spanish Ministry of Science and Innovation to IR-A and JL-G (grant PID2021-126330OB-I00 funded by MCIN/AEI and “ERDF A way of making Europe” by the European Union), by National Institutes of Health to MB (R01 NS080889 and R37 NS122141), and by grants from University of Modena and Reggio Emilia and from Banca Intesa-San Paolo to RB.
The authors declare that the research was conducted in the absence of any commercial or financial relationships that could be construed as a potential conflict of interest.
The authors declare that no Generative AI was used in the creation of this manuscript.
All claims expressed in this article are solely those of the authors and do not necessarily represent those of their affiliated organizations, or those of the publisher, the editors and the reviewers. Any product that may be evaluated in this article, or claim that may be made by its manufacturer, is not guaranteed or endorsed by the publisher.
Abraira, V. E., Kuehn, E. D., Chirila, A. M., Springel, M. W., Toliver, A. A., Zimmerman, A. L., et al. (2017). The cellular and synaptic architecture of the mechanosensory dorsal horn. Cell 168, 295–310.e19. doi: 10.1016/j.cell.2016.12.010
Agashkov, K., Krotov, V., Krasniakova, M., Shevchuk, D., Andrianov, Y., Zabenko, Y., et al. (2019). Distinct mechanisms of signal processing by lamina I spino-parabrachial neurons. Sci. Rep. 9:19231. doi: 10.1038/s41598-019-55462-7
Alba-Delgado, C., El Khoueiry, C., Peirs, C., Dallel, R., Artola, A., and Antri, M. (2015). Subpopulations of PKCγ interneurons within the medullary dorsal horn revealed by electrophysiologic and morphologic approach. Pain 156, 1714–1728. doi: 10.1097/j.pain.0000000000000221
Alles, S. R., Garcia, E., Balasubramanyan, S., Jones, K., Tyson, J. R., Joy, T., et al. (2018). Peripheral nerve injury increases contribution of L-type calcium channels to synaptic transmission in spinal lamina II: Role of α2δ-1 subunits. Mol. Pain 14:1744806918765806. doi: 10.1177/1744806918765806
Baccei, M. L., and Fitzgerald, M. (2005). Intrinsic firing properties of developing rat superficial dorsal horn neurons. Neuroreport 16, 1325–1328. doi: 10.1097/01.wnr.0000175612.08560.10
Balasubramanyan, S., Stemkowski, P. L., Stebbing, M. J., and Smith, P. A. (2006). Sciatic chronic constriction injury produces cell-type-specific changes in the electrophysiological properties of rat substantia gelatinosa neurons. J. Neurophysiol. 96, 579–590. doi: 10.1152/jn.00087.2006
Baranauskas, G., and Nistri, A. (1998). Sensitization of pain pathways in the spinal cord: cellular mechanisms. Prog. Neurobiol. 54, 349–365. doi: 10.1016/s0301-0082(97)00067-1
Baranauskas, G., Traversa, U., Rosati, A. M., and Nistri, A. (1995). An NK1 receptor-dependent component of the slow excitation recorded intracellularly from rat motoneurons following dorsal root stimulation. Eur. J. Neurosci. 7, 2409–2417. doi: 10.1111/j.1460-9568.1995.tb01039.x
Bardoni, R., Magherini, P. C., and MacDermott, A. B. (2000). Activation of NMDA receptors drives action potentials in superficial dorsal horn from neonatal rats. Neuroreport 11, 1721–1727. doi: 10.1097/00001756-200006050-00025
Bardoni, R., Shen, K.-F., Li, H., Jeffry, J., Barry, D. M., Comitato, A., et al. (2019). Pain inhibits GRPR Neurons via GABAergic signaling in the spinal cord. Sci. Rep. 9:15804. doi: 10.1038/s41598-019-52316-0
Bareyre, F. M., Haudenschild, B., and Schwab, M. E. (2002). Long-lasting sprouting and gene expression changes induced by the monoclonal antibody IN-1 in the adult spinal cord. J. Neurosci. 22, 7097–7110. doi: 10.1523/JNEUROSCI.22-16-07097.2002
Bell, A. M., Utting, C., Dickie, A. C., Kucharczyk, M. W., Quillet, R., Gutierrez-Mecinas, M., et al. (2024). Deep sequencing of Phox2a nuclei reveals five classes of anterolateral system neurons. Proc. Natl. Acad. Sci U S A. 121:e2314213121. doi: 10.1073/pnas.2314213121
Bester, H., Chapman, V., Besson, J. M., and Bernard, J. F. (2000). Physiological properties of the lamina I spinoparabrachial neurons in the rat. J. Neurophysiol. 83, 2239–2259. doi: 10.1152/jn.2000.83.4.2239
Betelli, C., MacDermott, A. B., and Bardoni, R. (2015). Transient, activity dependent inhibition of transmitter release from low threshold afferents mediated by GABAA receptors in spinal cord lamina III/IV. Mol. Pain 11:64. doi: 10.1186/s12990-015-0067-5
Bird, G. C., Han, J. S., Fu, Y., Adwanikar, H., Willis, W. D., and Neugebauer, V. (2006). Pain-related synaptic plasticity in spinal dorsal horn neurons: Role of CGRP. Mol. Pain 2:31. doi: 10.1186/1744-8069-2-31
Blackburn-Munro, G., and Fleetwood-Walker, S. M. (1999). The sodium channel auxiliary subunits beta1 and beta2 are differentially expressed in the spinal cord of neuropathic rats. Neuroscience 90, 153–164. doi: 10.1016/s0306-4522(98)00415-1
Boyle, K. A., Gradwell, M. A., Yasaka, T., Dickie, A. C., Polgár, E., Ganley, R. P., et al. (2019). Defining a spinal microcircuit that gates myelinated afferent input: implications for tactile allodynia. Cell. Rep. 28, 526–540.e6. doi: 10.1016/j.celrep.2019.06.040
Brewer, C. L., Styczynski, L. M., Serafin, E. K., and Baccei, M. L. (2020). Postnatal maturation of spinal dynorphin circuits and their role in somatosensation. Pain 161, 1906–1924. doi: 10.1097/j.pain.0000000000001884
Brocard, F., Shevtsova, N. A., Bouhadfane, M., Tazerart, S., Heinemann, U., Rybak, I. A., et al. (2013). Activity-dependent changes in extracellular Ca2+ and K+ reveal pacemakers in the spinal locomotor-related network. Neuron 77, 1047–1054. doi: 10.1016/j.neuron.2013.01.026
Browne, T. J., Gradwell, M. A., Iredale, J. A., Madden, J. F., Callister, R. J., Hughes, D. I., et al. (2020). Transgenic cross-referencing of inhibitory and excitatory interneuron populations to dissect neuronal heterogeneity in the dorsal horn. Front. Mol. Neurosci. 13:32. doi: 10.3389/fnmol.2020.00032
Browne, T. J., Smith, K. M., Gradwell, M. A., Iredale, J. A., Dayas, C. V., Callister, R. J., et al. (2021). Spinoparabrachial projection neurons form distinct classes in the mouse dorsal horn. Pain 162, 1977–1994. doi: 10.1097/j.pain.0000000000002194
Cameron, D., Polgár, E., Gutierrez-Mecinas, M., Gomez-Lima, M., Watanabe, M., and Todd, A. J. (2015). The organisation of spinoparabrachial neurons in the mouse. Pain 156, 2061–2071. doi: 10.1097/j.pain.0000000000000270
Candelas, M., Reynders, A., Arango-Lievano, M., Neumayer, C., Fruquière, A., Demes, E., et al. (2019). Cav3.2 T-type calcium channels shape electrical firing in mouse Lamina II neurons. Sci. Rep. 9:3112. doi: 10.1038/s41598-019-39703-3
Carr, F. B., Géranten, S. M., and Hunt, S. P. (2014). Descending controls modulate inflammatory joint pain and regulate CXC chemokine and iNOS expression in the dorsal horn. Mol. Pain 10:39. doi: 10.1186/1744-8069-10-39
Caspi, Y., Mazar, M., Kushnir, Y., Mazor, Y., Katz, B., Lev, S., et al. (2023). Structural plasticity of axon initial segment in spinal cord neurons underlies inflammatory pain. Pain 164, 1388–1401. doi: 10.1097/j.pain.0000000000002829
Cata, J. P., Weng, H.-R., Chen, J.-H., and Dougherty, P. M. (2006). Altered discharges of spinal wide dynamic range neurons and down-regulation of glutamate transporter expression in rats with paclitaxel-induced hyperalgesia. Neuroscience 138, 329–338. doi: 10.1016/j.neuroscience.2005.11.009
Cervero, F., Iggo, A., and Molony, V. (1979). An electrophysiological study of neurones in the substantia gelatinosa rolandi of the cat’s spinal cord. Q. J. Exp. Physiol. Cogn. Med. Sci. 64, 297–314. doi: 10.1113/expphysiol.1979.sp002484
Cervero, F., Iggo, A., and Ogawa, H. (1976). Nociceptor-driven dorsal horn neurones in the lumbar spinal cord of the cat. Pain 2, 5–24. doi: 10.1016/0304-3959(76)90042-7
Cervero, F., Schaible, H.-G., and Schmidt, R. F. (1991). Tonic descending inhibition of spinal cord neurones driven by joint afferents in normal cats and in cats with an inflamed knee joint. Exp. Brain Res. 83, 657–658. doi: 10.1007/BF00229846
Chen, S., Gao, X.-F., Zhou, Y., Liu, B.-L., Liu, X.-Y., Zhang, Y., et al. (2020). A spinal neural circuitry for converting touch to itch sensation. Nat. Commun. 11:5074. doi: 10.1038/s41467-020-18895-7
Chen, S.-R., Cai, Y.-Q., and Pan, H.-L. (2009). Plasticity and emerging role of BKCa channels in nociceptive control in neuropathic pain. J. Neurochem. 110, 352–362.
Chen, Y., Chen, S.-R., Chen, H., Zhang, J., and Pan, H.-L. (2019). Increased α2δ-1-NMDA receptor coupling potentiates glutamatergic input to spinal dorsal horn neurons in chemotherapy-induced neuropathic pain. J. Neurochem. 148, 252–274. doi: 10.1111/jnc.14627
Cho, J.-H., Choi, I.-S., Lee, S.-H., Lee, M.-G., and Jang, I.-S. (2015). Contribution of persistent sodium currents to the excitability of tonic firing substantia gelatinosa neurons of the rat. Neurosci. Lett. 591, 192–196. doi: 10.1016/j.neulet.2015.02.039
Choi, S., Hachisuka, J., Brett, M. A., Magee, A. R., Omori, Y., Iqbal, N.-U.-A., et al. (2020). Parallel ascending spinal pathways for affective touch and pain. Nature 587, 258–263. doi: 10.1038/s41586-020-2860-1
Christensen, B. N., and Perl, E. R. (1970). Spinal neurons specifically excited by noxious or thermal stimuli: Marginal zone of the dorsal horn. J. Neurophysiol. 33, 293–307. doi: 10.1152/jn.1970.33.2.293
Cizkova, D., Marsala, J., Lukacova, N., Marsala, M., Jergova, S., Orendacova, J., et al. (2002). Localization of N-type Ca2+ channels in the rat spinal cord following chronic constrictive nerve injury. Exp. Brain Res. 147, 456–463. doi: 10.1007/s00221-002-1217-3
Clerc, N., and Moqrich, A. (2022). Diverse roles and modulations of IA in spinal cord pain circuits. Cell. Rep. 38:110588. doi: 10.1016/j.celrep.2022.110588
Collins, J. G. (1984). Neuronal activity recorded from the spinal dorsal horn of physiologically intact, awake, drug-free, restrained cats: A preliminary report. Brain Res. 322, 301–304. doi: 10.1016/0006-8993(84)90121-5
Coull, J. A. M., Beggs, S., Boudreau, D., Boivin, D., Tsuda, M., Inoue, K., et al. (2005). BDNF from microglia causes the shift in neuronal anion gradient underlying neuropathic pain. Nature 438, 1017–1021. doi: 10.1038/nature04223
Coull, J. A. M., Boudreau, D., Bachand, K., Prescott, S. A., Nault, F., Sík, A., et al. (2003). Trans-synaptic shift in anion gradient in spinal lamina I neurons as a mechanism of neuropathic pain. Nature 424, 938–942. doi: 10.1038/nature01868
Cui, L., Miao, X., Liang, L., Abdus-Saboor, I., Olson, W., Fleming, M. S., et al. (2016). Identification of early RET+ deep dorsal spinal cord interneurons in gating pain. Neuron 91, 1137–1153. doi: 10.1016/j.neuron.2016.07.038
Daniele, C. A., and MacDermott, A. B. (2009). Low-threshold primary afferent drive onto GABAergic interneurons in the superficial dorsal horn of the mouse. J. Neurosci. 29, 686–695. doi: 10.1523/JNEUROSCI.5120-08.2009
Davis, O. C., Dickie, A. C., Mustapa, M. B., Boyle, K. A., Browne, T. J., Gradwell, M. A., et al. (2023). Calretinin-expressing islet cells are a source of pre- and post-synaptic inhibition of non-peptidergic nociceptor input to the mouse spinal cord. Sci. Rep. 13:11561. doi: 10.1038/s41598-023-38605-9
Denk, F., Crow, M., Didangelos, A., Lopes, D. M., and McMahon, S. B. (2016). Persistent alterations in microglial enhancers in a model of chronic pain. Cell Rep. 15, 1771–1781. doi: 10.1016/j.celrep.2016.04.063
Derjean, D., Bertrand, S., Le Masson, G., Landry, M., Morisset, V., and Nagy, F. (2003). Dynamic balance of metabotropic inputs causes dorsal horn neurons to switch functional states. Nat Neurosci 6, 274–281. doi: 10.1038/nn1016
Dickenson, A. H., and Sullivan, A. F. (1987). Evidence for a role of the NMDA receptor in the frequency dependent potentiation of deep rat dorsal horn nociceptive neurones following C fibre stimulation. Neuropharmacology 26, 1235–1238. doi: 10.1016/0028-3908(87)90275-9
Dickie, A. C., Bell, A. M., Iwagaki, N., Polgár, E., Gutierrez-Mecinas, M., Kelly, R., et al. (2019). Morphological and functional properties distinguish the substance P and gastrin-releasing peptide subsets of excitatory interneuron in the spinal cord dorsal horn. Pain 160, 442–462. doi: 10.1097/j.pain.0000000000001406
Dobremez, E., Bouali-Benazzouz, R., Fossat, P., Monteils, L., Dulluc, J., Nagy, F., et al. (2005). Distribution and regulation of L-type calcium channels in deep dorsal horn neurons after sciatic nerve injury in rats. Eur. J. Neurosci. 21, 3321–3333. doi: 10.1111/j.1460-9568.2005.04177.x
Doolen, S., Blake, C. B., Smith, B. N., and Taylor, B. K. (2012). Peripheral nerve injury increases glutamate-evoked calcium mobilization in adult spinal cord neurons. Mol. Pain 8:56. doi: 10.1186/1744-8069-8-56
Dougherty, K. J., and Hochman, S. (2008). Spinal cord injury causes plasticity in a subpopulation of lamina I GABAergic interneurons. J. Neurophysiol. 100, 212–223. doi: 10.1152/jn.01104.2007
Dougherty, P. M., and Chen, J. (2016). Relationship of membrane properties, spike burst responses, laminar location, and functional class of dorsal horn neurons recorded in vitro. J. Neurophysiol. 116, 1137–1151. doi: 10.1152/jn.00187.2016
Duan, B., Cheng, L., Bourane, S., Britz, O., Padilla, C., Garcia-Campmany, L., et al. (2014). Identification of spinal circuits transmitting and gating mechanical pain. Cell 159, 1417–1432. doi: 10.1016/j.cell.2014.11.003
Duan, B., Wu, L.-J., Yu, Y.-Q., Ding, Y., Jing, L., Xu, L., et al. (2007). Upregulation of acid-sensing ion channel ASIC1a in spinal dorsal horn neurons contributes to inflammatory pain hypersensitivity. J. Neurosci. 27, 11139–11148. doi: 10.1523/JNEUROSCI.3364-07.2007
Dubner, R., and Ruda, M. A. (1992). Activity-dependent neuronal plasticity following tissue injury and inflammation. Trends Neurosci. 15, 96–103. doi: 10.1016/0166-2236(92)90019-5
Eccles, J. C., Kostyuk, P. G., and Schmidt, R. F. (1962). Central pathways responsible for depolarization of primary afferent fibres. J. Physiol. 161, 237–257. doi: 10.1113/jphysiol.1962.sp006884
Eckert, W. A., Julius, D., and Basbaum, A. I. (2006). Differential contribution of TRPV1 to thermal responses and tissue injury-induced sensitization of dorsal horn neurons in laminae I and V in the mouse. Pain 126, 184–197. doi: 10.1016/j.pain.2006.06.032
Fabrizi, L., Slater, R., Worley, A., Meek, J., Boyd, S., Olhede, S., et al. (2011). A shift in sensory processing that enables the developing human brain to discriminate touch from pain. Curr. Biol. 21, 1552–1558. doi: 10.1016/j.cub.2011.08.010
Farrell, K. E., Keely, S., Walker, M. M., Brichta, A. M., Graham, B. A., and Callister, R. J. (2017). Altered intrinsic and synaptic properties of lumbosacral dorsal horn neurons in a mouse model of colitis. Neuroscience 362, 152–167. doi: 10.1016/j.neuroscience.2017.08.029
Fernandes, E. C., Luz, L. L., Mytakhir, O., Lukoyanov, N. V., Szucs, P., and Safronov, B. V. (2016). Diverse firing properties and Aβ-, Aδ-, and C-afferent inputs of small local circuit neurons in spinal lamina I. Pain 157, 475–487. doi: 10.1097/j.pain.0000000000000394
Fernandes, E. C., Santos, I. C., Kokai, E., Luz, L. L., Szucs, P., and Safronov, B. V. (2018). Low- and high-threshold primary afferent inputs to spinal lamina III antenna-type neurons. Pain 159, 2214–2222. doi: 10.1097/j.pain.0000000000001320
Ford, N. C., and Baccei, M. L. (2016). Inward-rectifying K+ (Kir2) leak conductance dampens the excitability of lamina I projection neurons in the neonatal rat. Neuroscience 339, 502–510. doi: 10.1016/j.neuroscience.2016.10.027
Ford, N. C., Ren, D., and Baccei, M. L. (2018). NALCN channels enhance the intrinsic excitability of spinal projection neurons. Pain 159, 1719–1730. doi: 10.1097/j.pain.0000000000001258
Fossat, P., Sibon, I., Le Masson, G., Landry, M., and Nagy, F. (2007). L-type calcium channels and NMDA receptors: A determinant duo for short-term nociceptive plasticity. Eur. J. Neurosci. 25, 127–135. doi: 10.1111/j.1460-9568.2006.05256.x
Ganley, R. P., Iwagaki, N., del Rio, P., Baseer, N., Dickie, A. C., Boyle, K. A., et al. (2015). Inhibitory interneurons that express GFP in the PrP-GFP mouse spinal cord are morphologically heterogeneous, innervated by several classes of primary afferent and include lamina I projection neurons among their postsynaptic targets. J. Neurosci. 35, 7626–7642. doi: 10.1523/JNEUROSCI.0406-15.2015
Gassner, M., Leitner, J., Gruber-Schoffnegger, D., Forsthuber, L., and Sandkühler, J. (2013). Properties of spinal lamina III GABAergic neurons in naïve and in neuropathic mice. Eur. J. Pain 17, 1168–1179. doi: 10.1002/j.1532-2149.2013.00294.x
Gauchan, P., Andoh, T., Ikeda, K., Fujita, M., Sasaki, A., Kato, A., et al. (2009). Mechanical allodynia induced by paclitaxel, oxaliplatin and vincristine: different effectiveness of gabapentin and different expression of voltage-dependent calcium channel alpha(2)delta-1 subunit. Biol. Pharm. Bull. 32, 732–734. doi: 10.1248/bpb.32.732
Gieré, C., Melchior, M., Dufour, A., and Poisbeau, P. (2021). Spinal integration of hot and cold nociceptive stimuli by wide-dynamic-range neurons in anesthetized adult rats. Pain Rep. 6:e983. doi: 10.1097/PR9.0000000000000983
Gosselin, R. D., Varela, C., Banisadr, G., Mechighel, P., Rostene, W., Kitabgi, P., et al. (2005). Constitutive expression of CCR2 chemokine receptor and inhibition by MCP-1/CCL2 of GABA-induced currents in spinal cord neurones. J. Neurochem. 95, 1023–1034. doi: 10.1111/j.1471-4159.2005.03431.x
Gradwell, M. A., Boyle, K. A., Browne, T. J., Bell, A. M., Leonardo, J., Peralta Reyes, F. S., et al. (2022a). Diversity of inhibitory and excitatory parvalbumin interneuron circuits in the dorsal horn. Pain 163, e432–e452. doi: 10.1097/j.pain.0000000000002422
Gradwell, M. A., Boyle, K. A., Callister, R. J., Hughes, D. I., and Graham, B. A. (2017). Heteromeric α/β glycine receptors regulate excitability in parvalbumin-expressing dorsal horn neurons through phasic and tonic glycinergic inhibition. J. Physiol. 595, 7185–7202. doi: 10.1113/JP274926
Gradwell, M. A., Smith, K. M., Dayas, C. V., Smith, D. W., Hughes, D. I., Callister, R. J., et al. (2022b). Altered intrinsic properties and inhibitory connectivity in aged parvalbumin-expressing dorsal horn neurons. Front. Neural Circuits 16:834173. doi: 10.3389/fncir.2022.834173
Graham, B. A., Brichta, A. M., and Callister, R. J. (2004). In vivo responses of mouse superficial dorsal horn neurones to both current injection and peripheral cutaneous stimulation. J. Physiol. 561, 749–763. doi: 10.1113/jphysiol.2004.072645
Graham, B. A., Brichta, A. M., and Callister, R. J. (2007). Pinch-current injection defines two discharge profiles in mouse superficial dorsal horn neurones, in vitro. J. Physiol. 578, 787–798. doi: 10.1113/jphysiol.2006.123349
Graham, B. A., Brichta, A. M., and Callister, R. J. (2008). Recording temperature affects the excitability of mouse superficial dorsal horn neurons. In Vitro. J. Neurophysiol. 99, 2048–2059. doi: 10.1152/jn.01176.2007
Greenspon, C. M., Battell, E. E., Devonshire, I. M., Donaldson, L. F., Chapman, V., and Hathway, G. J. (2019). Lamina-specific population encoding of cutaneous signals in the spinal dorsal horn using multi-electrode arrays. J. Physiol. 597, 377–397. doi: 10.1113/JP277036
Grudt, T. J., and Perl, E. R. (2002). Correlations between neuronal morphology and electrophysiological features in the rodent superficial dorsal horn. J. Physiol. 540, 189–207. doi: 10.1113/jphysiol.2001.012890
Hachisuka, J., Baumbauer, K. M., Omori, Y., Snyder, L. M., Koerber, H. R., and Ross, S. E. (2016). Semi-intact ex vivo approach to investigate spinal somatosensory circuits. Elife 5:e22866. doi: 10.7554/eLife.22866
Hachisuka, J., Omori, Y., Chiang, M. C., Gold, M. S., Koerber, H. R., and Ross, S. E. (2018). Wind-up in lamina I spinoparabrachial neurons: A role for reverberatory circuits. Pain 159, 1484–1493. doi: 10.1097/j.pain.0000000000001229
Hains, B. C., Saab, C. Y., Klein, J. P., Craner, M. J., and Waxman, S. G. (2004). Altered sodium channel expression in second-order spinal sensory neurons contributes to pain after peripheral nerve injury. J. Neurosci. 24, 4832–4839. doi: 10.1523/JNEUROSCI.0300-04.2004
Handwerker, H. O., Iggo, A., and Ogawa, H. (1975). Dorsal horn neurones responding to cutaneous afferent input. J. Physiol. 244, 1–2.
Hantman, A. W., van den Pol, A. N., and Perl, E. R. (2004). Morphological and physiological features of a set of spinal substantia gelatinosa neurons defined by green fluorescent protein expression. J. Neurosci. 24, 836–842. doi: 10.1523/jneurosci.4221-03.2004
Harding, E. K., Fung, S. W., and Bonin, R. P. (2020). Insights into spinal dorsal horn circuit function and dysfunction using optical approaches. Front. Neural Circuits 14:31. doi: 10.3389/fncir.2020.00031
Hari, K., Lucas-Osma, A. M., Metz, K., Lin, S., Pardell, N., Roszko, D. A., et al. (2022). GABA facilitates spike propagation through branch points of sensory axons in the spinal cord. Nat. Neurosci. 25, 1288–1299. doi: 10.1038/s41593-022-01162-x
Häring, M., Zeisel, A., Hochgerner, H., Rinwa, P., Jakobsson, J. E. T., Lönnerberg, P., et al. (2018). Neuronal atlas of the dorsal horn defines its architecture and links sensory input to transcriptional cell types. Nat. Neurosci. 21, 869–880. doi: 10.1038/s41593-018-0141-1
He, X., Liu, P., Zhang, X., Jiang, Z., Gu, N., Wang, Q., et al. (2021). Molecular and electrophysiological characterization of dorsal horn neurons in a GlyT2-iCre-tdTomato mouse line. J. Pain Res. 14, 907–921. doi: 10.2147/JPR.S296940
Heavner, J. E., and De Jong, R. H. (1973). Spinal cord neuron response to natural stimuli. A microelectrode study. Exp. Neurol. 39, 293–306. doi: 10.1016/0014-4886(73)90232-X
Heine, S., Michalakis, S., Kallenborn-Gerhardt, W., Lu, R., Lim, H.-Y., Weiland, J., et al. (2011). CNGA3: A target of spinal nitric oxide/cGMP signaling and modulator of inflammatory pain hypersensitivity. J. Neurosci. 31, 11184–11192. doi: 10.1523/JNEUROSCI.6159-10.2011
Heinke, B., Balzer, E., and Sandkühler, J. (2004a). Pre- and postsynaptic contributions of voltage-dependent Ca2+ channels to nociceptive transmission in rat spinal lamina I neurons. Eur. J. Neurosci. 19, 103–111. doi: 10.1046/j.1460-9568.2003.03083.x
Heinke, B., Ruscheweyh, R., Forsthuber, L., Wunderbaldinger, G., and Sandkühler, J. (2004b). Physiological, neurochemical and morphological properties of a subgroup of GABAergic spinal lamina II neurones identified by expression of green fluorescent protein in mice. J. Physiol. 560, 249–266. doi: 10.1113/jphysiol.2004.070540
Herrero, J. F., Coates, T. W., Higgins, M., Livingston, A., Waterman, A. E., and Headley, P. M. (1993). A technique for recording from spinal neurones in awake sheep. J. Neurosci. Methods 46, 225–232. doi: 10.1016/0165-0270(93)90071-x
Herrero, J. F., Laird, J. M., and López-García, J. A. (2000). Wind-up of spinal cord neurones and pain sensation: Much ado about something? Prog Neurobiol. 61, 169–203. doi: 10.1016/s0301-0082(99)00051-9
Hibino, H., Inanobe, A., Furutani, K., Murakami, S., Findlay, I., and Kurachi, Y. (2010). Inwardly rectifying potassium channels: their structure, function, and physiological roles. Physiol. Rev. 90, 291–366. doi: 10.1152/physrev.00021.2009
Hildebrand, M. E., Mezeyova, J., Smith, P. L., Salter, M. W., Tringham, E., and Snutch, T. P. (2011). Identification of sodium channel isoforms that mediate action potential firing in lamina I/II spinal cord neurons. Mol. Pain 7:67. doi: 10.1186/1744-8069-7-67
Hiyoshi, K., Shiraishi, A., Fukuda, N., and Tsuda, S. (2021). In vivo wide-field voltage imaging in zebrafish with voltage-sensitive dye and genetically encoded voltage indicator. Dev. Growth Differ. 63, 417–428. doi: 10.1111/dgd.12744
Hoheisel, U., Unger, T., and Mense, S. (2000). A block of spinal nitric oxide synthesis leads to increased background activity predominantly in nociceptive dorsal horn neurones in the rat. Pain 88, 249–257. doi: 10.1016/S0304-3959(00)00336-5
Hu, H.-J., Carrasquillo, Y., Karim, F., Jung, W. E., Nerbonne, J. M., Schwarz, T. L., et al. (2006). The kv4.2 potassium channel subunit is required for pain plasticity. Neuron 50, 89–100. doi: 10.1016/j.neuron.2006.03.010
Hu, T., Liu, N., Lv, M., Ma, L., Peng, H., Peng, S., et al. (2016). Lidocaine inhibits HCN currents in rat spinal substantia gelatinosa neurons. Anesth. Analg. 122, 1048–1059. doi: 10.1213/ANE.0000000000001140
Huang, L. Y. (1989). Calcium channels in isolated rat dorsal horn neurones, including labelled spinothalamic and trigeminothalamic cells. J. Physiol. 411, 161–177. doi: 10.1113/jphysiol.1989.sp017566
Hughes, D. I., and Todd, A. J. (2020). Central nervous system targets: Inhibitory interneurons in the spinal cord. Neurotherapeutics 17, 874–885. doi: 10.1007/s13311-020-00936-0
Hughes, D. I., Sikander, S., Kinnon, C. M., Boyle, K. A., Watanabe, M., Callister, R. J., et al. (2012). Morphological, neurochemical and electrophysiological features of parvalbumin-expressing cells: A likely source of axo-axonic inputs in the mouse spinal dorsal horn. J. Physiol. 590, 3927–3951. doi: 10.1113/jphysiol.2012.235655
Hylden, J. L. K., Nahin, R. L., Traub, R. J., and Dubner, R. (1989). Expansion of receptive fields of spinal lamina I projection neurons in rats with unilateral adjuvant-induced inflammation: The contribution of dorsal horn mechanisms. Pain 37, 229–243. doi: 10.1016/0304-3959(89)90135-8
Ippolito, D. L., Xu, M., Bruchas, M. R., Wickman, K., and Chavkin, C. (2005). Tyrosine phosphorylation of K(ir)3.1 in spinal cord is induced by acute inflammation, chronic neuropathic pain, and behavioral stress. J. Biol. Chem. 280, 41683–41693. doi: 10.1074/jbc.M507069200
Iwagaki, N., Ganley, R. P., Dickie, A. C., Polgár, E., Hughes, D. I., Del Rio, P., et al. (2016). A combined electrophysiological and morphological study of neuropeptide Y-expressing inhibitory interneurons in the spinal dorsal horn of the mouse. Pain 157, 598–612. doi: 10.1097/j.pain.0000000000000407
Jeftinija, S. (1988). Enkephalins modulate excitatory synaptic transmission in the superficial dorsal horn by acting at mu-opioid receptor sites. Brain Res. 460, 260–268. doi: 10.1016/0006-8993(88)90371-x
Jeftinija, S., and Urban, L. (1994). Repetitive stimulation induced potentiation of excitatory transmission in the rat dorsal horn: An in vitro study. J. Neurophysiol. 71, 216–228. doi: 10.1152/jn.1994.71.1.216
Jiang, M. C., Cleland, C. L., and Gebhart, G. F. (1995). Intrinsic properties of deep dorsal horn neurons in the L6-S1 spinal cord of the intact rat. J. Neurophysiol. 74, 1819–1827. doi: 10.1152/jn.1995.74.5.1819
Johannssen, H. C., and Helmchen, F. (2010). In vivo Ca2+ imaging of dorsal horn neuronal populations in mouse spinal cord. J. Physiol. 588, 3397–3402. doi: 10.1113/jphysiol.2010.191833
Kardon, A. P., Polgár, E., Hachisuka, J., Snyder, L. M., Cameron, D., Savage, S., et al. (2014). Dynorphin acts as a neuromodulator to inhibit itch in the dorsal horn of the spinal cord. Neuron 82, 573–586. doi: 10.1016/j.neuron.2014.02.046
Keller, A. F., Beggs, S., Salter, M. W., and De Koninck, Y. (2007). Transformation of the output of spinal lamina I neurons after nerve injury and microglia stimulation underlying neuropathic pain. Mol. Pain 3:27. doi: 10.1186/1744-8069-3-27
Kerr, B. J., Bradbury, E. J., Bennett, D. L. H., Trivedi, P. M., Dassan, P., French, J., et al. (1999). Brain-derived neurotrophic factor modulates nociceptive sensory inputs and NMDA-evoked responses in the rat spinal cord. J. Neurosci. 19, 5138–5148. doi: 10.1523/JNEUROSCI.19-12-05138.1999
Kim, D. K., Kwak, J., Kim, S. J., and Kim, J. (2008). Long-lasting enhancement in the intrinsic excitability of deep dorsal horn neurons. Pain 139, 181–189. doi: 10.1016/j.pain.2008.03.025
King, A. E., and Lopez-Garcia, J. A. (1993). Excitatory amino acid receptor-mediated neurotransmission from cutaneous afferents in rat dorsal horn in vitro. J. Physiol. 472, 443–457. doi: 10.1113/jphysiol.1993.sp019955
King, A. E., Thompson, S. W., Urban, L., and Woolf, C. J. (1988). The responses recorded in vitro of deep dorsal horn neurons to direct and orthodromic stimulation in the young rat spinal cord. Neuroscience 27, 231–242. doi: 10.1016/0306-4522(88)90233-3
Ku, W., and Schneider, S. P. (2011). Multiple T-type Ca2+ current subtypes in electrophysiologically characterized hamster dorsal horn neurons: Possible role in spinal sensory integration. J. Neurophysiol. 106, 2486–2498. doi: 10.1152/jn.01083.2010
Lampert, A., Hains, B. C., and Waxman, S. G. (2006). Upregulation of persistent and ramp sodium current in dorsal horn neurons after spinal cord injury. Exp. Brain Res. 174, 660–666. doi: 10.1007/s00221-006-0511-x
Legendre, P., McKenzie, J. S., Dupouy, B., and Vincent, J. D. (1985). Evidence for bursting pacemaker neurones in cultured spinal cord cells. Neuroscience 16, 753–767. doi: 10.1016/0306-4522(85)90092-2
Lever, I. J., Grant, A. D., Pezet, S., Gerard, N. P., Brain, S. D., and Malcangio, M. (2003). Basal and activity-induced release of substance P from primary afferent fibres in NK1 receptor knockout mice: Evidence for negative feedback. Neuropharmacology 45, 1101–1110. doi: 10.1016/s0028-3908(03)00298-3
Li, C.-Y., Song, Y.-H., Higuera, E. S., and Luo, Z. D. (2004). Spinal dorsal horn calcium channel alpha2delta-1 subunit upregulation contributes to peripheral nerve injury-induced tactile allodynia. J. Neurosci. 24, 8494–8499. doi: 10.1523/JNEUROSCI.2982-04.2004
Li, J., and Baccei, M. L. (2011). Pacemaker neurons within newborn spinal pain circuits. J. Neurosci. 31, 9010–9022. doi: 10.1523/JNEUROSCI.6555-10.2011
Li, J., and Baccei, M. L. (2012). Developmental regulation of membrane excitability in rat spinal lamina I projection neurons. J. Neurophysiol. 107, 2604–2614. doi: 10.1152/jn.00899.2011
Li, J., and Baccei, M. L. (2014). Neonatal tissue injury reduces the intrinsic excitability of adult mouse superficial dorsal horn neurons. Neuroscience 256, 392–402. doi: 10.1016/j.neuroscience.2013.10.057
Li, J., and Baccei, M. L. (2021). Intrinsic burst-firing in lamina I spinoparabrachial neurons during adolescence. Neurosci. Lett. 750:135794. doi: 10.1016/j.neulet.2021.135794
Li, J., Blankenship, M. L., and Baccei, M. L. (2013). Inward-rectifying potassium (Kir) channels regulate pacemaker activity in spinal nociceptive circuits during early life. J. Neurosci. 33, 3352–3362. doi: 10.1523/JNEUROSCI.4365-12.2013
Li, J., Kritzer, E., Ford, N. C., Arbabi, S., and Baccei, M. L. (2015). Connectivity of pacemaker neurons in the neonatal rat superficial dorsal horn. J. Comp. Neurol. 523, 1038–1053. doi: 10.1002/cne.23706
Li, J., Serafin, E. K., and Baccei, M. L. (2023). Intrinsic and synaptic properties of adult mouse spinoperiaqueductal gray neurons and the influence of neonatal tissue damage. Pain 164, 905–917. doi: 10.1097/j.pain.0000000000002787
Liu, Q., Vrontou, S., Rice, F. L., Zylka, M. J., Dong, X., and Anderson, D. J. (2007). Molecular genetic visualization of a rare subset of unmyelinated sensory neurons that may detect gentle touch. Nat. Neurosci. 10, 946–948. doi: 10.1038/nn1937
Liu, X., Zhang, L., Jin, L., Tan, Y., Li, W., and Tang, J. (2018). HCN2 contributes to oxaliplatin-induced neuropathic pain through activation of the CaMKII/CREB cascade in spinal neurons. Mol. Pain 14:1744806918778490. doi: 10.1177/1744806918778490
Lopez-Garcia, J. (2006). Serotonergic modulation of spinal sensory circuits. CTMC 6, 1987–1996. doi: 10.2174/156802606778522159
Lopez-Garcia, J. A., and King, A. E. (1994). Membrane properties of physiologically classified rat dorsal horn neurons in vitro: Correlation with cutaneous sensory afferent input. Eur. J. Neurosci. 6, 998–1007. doi: 10.1111/j.1460-9568.1994.tb00594.x
Lu, B., Su, Y., Das, S., Liu, J., Xia, J., and Ren, D. (2007). The neuronal channel NALCN contributes resting sodium permeability and is required for normal respiratory rhythm. Cell 129, 371–383. doi: 10.1016/j.cell.2007.02.041
Lu, Y., and Perl, E. R. (2005). Modular organization of excitatory circuits between neurons of the spinal superficial dorsal horn (laminae I and II). J. Neurosci. 25, 3900–3907. doi: 10.1523/JNEUROSCI.0102-05.2005
Lucas-Romero, J., Rivera-Arconada, I., and Lopez-Garcia, J. A. (2022). Synchronous firing of dorsal horn neurons at the origin of dorsal root reflexes in naïve and paw-inflamed mice. Front. Cell. Neurosci. 16:1004956. doi: 10.3389/fncel.2022.1004956
Lucas-Romero, J., Rivera-Arconada, I., and Lopez-Garcia, J. A. (2024). Noise or signal? Spontaneous activity of dorsal horn neurons: Patterns and function in health and disease. Pflugers Arch. 476, 1171–1186. doi: 10.1007/s00424-024-02971-8
Lucas-Romero, J., Rivera-Arconada, I., Roza, C., and Lopez-Garcia, J. A. (2018). Origin and classification of spontaneous discharges in mouse superficial dorsal horn neurons. Sci. Rep. 8:9735. doi: 10.1038/s41598-018-27993-y
Luz, L. L., Szucs, P., and Safronov, B. V. (2014). Peripherally driven low-threshold inhibitory inputs to lamina I local-circuit and projection neurones: A new circuit for gating pain responses. J. Physiol. 592, 1519–1534. doi: 10.1113/jphysiol.2013.269472
Luz, L. L., Szucs, P., Pinho, R., and Safronov, B. V. (2010). Monosynaptic excitatory inputs to spinal lamina I anterolateral-tract-projecting neurons from neighbouring lamina I neurons. J. Physiol. 588, 4489–4505. doi: 10.1113/jphysiol.2010.197012
Ma, X., Miraucourt, L. S., Qiu, H., Sharif-Naeini, R., and Khadra, A. (2023). Modulation of SK channels via calcium buffering tunes intrinsic excitability of parvalbumin interneurons in neuropathic pain: A computational and experimental investigation. J. Neurosci. 43, 5608–5622. doi: 10.1523/JNEUROSCI.0426-23.2023
Manjarrez, E., Jiménez, I., and Rudomin, P. (2003). Intersegmental synchronization of spontaneous activity of dorsal horn neurons in the cat spinal cord. Exp. Brain Res. 148, 401–413. doi: 10.1007/s00221-002-1303-6
Manjarrez, E., Rojas-Piloni, G., Vazquez, D., and Flores, A. (2002). Cortical neuronal ensembles driven by dorsal horn spinal neurones with spontaneous activity in the cat. Neurosci. Lett. 318, 145–148. doi: 10.1016/s0304-3940(01)02497-1
Martindale, J. C., Wilson, A. W., Reeve, A. J., Chessell, I. P., and Headley, P. M. (2007). Chronic secondary hypersensitivity of dorsal horn neurones following inflammation of the knee joint. Pain 133, 79–86. doi: 10.1016/j.pain.2007.03.006
Maxwell, D. J., Belle, M. D., Cheunsuang, O., Stewart, A., and Morris, R. (2007). Morphology of inhibitory and excitatory interneurons in superficial laminae of the rat dorsal horn. J. Physiol. 584, 521–533. doi: 10.1113/jphysiol.2007.140996
McGaraughty, S., Chu, K. L., and Xu, J. (2018). Characterization and pharmacological modulation of noci-responsive deep dorsal horn neurons across diverse rat models of pathological pain. J. Neurophysiol. 120, 1893–1905. doi: 10.1152/jn.00325.2018
Medrano, M. C., Dhanasobhon, D., Yalcin, I., Schlichter, R., and Cordero-Erausquin, M. (2016). Loss of inhibitory tone on spinal cord dorsal horn spontaneously and nonspontaneously active neurons in a mouse model of neuropathic pain. Pain 157, 1432–1442. doi: 10.1097/j.pain.0000000000000538
Meesawatsom, P., Hathway, G., Bennett, A., Constantin-Teodosiu, D., and Chapman, V. (2020). Spinal neuronal excitability and neuroinflammation in a model of chemotherapeutic neuropathic pain: Targeting the resolution pathways. J. Neuroinflammation 17, 316. doi: 10.1186/s12974-020-01997-w
Melnick, I. V. (2011). A-type K+ current dominates somatic excitability of delayed firing neurons in rat substantia gelatinosa. Synapse 65, 601–607. doi: 10.1002/syn.20879
Melnick, I. V., Santos, S. F. A., and Safronov, B. V. (2004a). Mechanism of spike frequency adaptation in substantia gelatinosa neurones of rat. J. Physiol. 559, 383–395. doi: 10.1113/jphysiol.2004.066415
Melnick, I. V., Santos, S. F. A., Szokol, K., Szûcs, P., and Safronov, B. V. (2004b). Ionic basis of tonic firing in spinal substantia gelatinosa neurons of rat. J. Neurophysiol. 91, 646–655. doi: 10.1152/jn.00883.2003
Mendell, L. M. (1966). Physiological properties of unmyelinated fiber projection to the spinal cord. Exp. Neurol. 16, 316–332. doi: 10.1016/0014-4886(66)90068-9
Mendell, L. M., and Wall, P. D. (1965). Responses of single dorsal cord cells to peripheral cutaneous unmyelinated fibres. Nature 206, 97–99. doi: 10.1038/206097a0
Merighi, A. (2018). The histology, physiology, neurochemistry and circuitry of the substantia gelatinosa Rolandi (lamina II) in mammalian spinal cord. Prog. Neurobiol. 169, 91–134. doi: 10.1016/j.pneurobio.2018.06.012
Mesnage, B., Gaillard, S., Godin, A. G., Rodeau, J.-L., Hammer, M., Von Engelhardt, J., et al. (2011). Morphological and functional characterization of cholinergic interneurons in the dorsal horn of the mouse spinal cord. J. Comp. Neurol. 519, 3139–3158. doi: 10.1002/cne.22668
Miranda, C. O., Hegedüs, K., Kis, G., and Antal, M. (2023). Synaptic targets of glycinergic neurons in laminae I-III of the spinal dorsal horn. Int. J. Mol. Sci. 24:6943. doi: 10.3390/ijms24086943
Miranda, C. O., Hegedüs, K., Wildner, H., Zeilhofer, H. U., and Antal, M. (2022). Morphological and neurochemical characterization of glycinergic neurons in laminae I-IV of the mouse spinal dorsal horn. J. Comp. Neurol. 530, 607–626. doi: 10.1002/cne.25232
Mongan, L. C., Hill, M. J., Chen, M. X., Tate, S. N., Collins, S. D., Buckby, L., et al. (2005). The distribution of small and intermediate conductance calcium-activated potassium channels in the rat sensory nervous system. Neuroscience 131, 161–175. doi: 10.1016/j.neuroscience.2004.09.062
Monteiro, C., Lima, D., and Galhardo, V. (2006). Switching-on and -off of bistable spontaneous discharges in rat spinal deep dorsal horn neurons. Neurosci. Lett. 398, 258–263. doi: 10.1016/j.neulet.2006.01.008
Morisset, V., and Nagy, F. (1998). Nociceptive integration in the rat spinal cord: Role of non-linear membrane properties of deep dorsal horn neurons. Eur. J. Neurosci. 10, 3642–3652. doi: 10.1046/j.1460-9568.1998.00370.x
Morisset, V., and Nagy, F. (1999). Ionic basis for plateau potentials in deep dorsal horn neurons of the rat spinal cord. J. Neurosci. 19, 7309–7316. doi: 10.1523/JNEUROSCI.19-17-07309.1999
Morisset, V., and Nagy, F. (2000). Plateau potential-dependent windup of the response to primary afferent stimuli in rat dorsal horn neurons. Eur. J. Neurosci. 12, 3087–3095. doi: 10.1046/j.1460-9568.2000.00188.x
Murase, K., Ryu, P. D., and Randić, M. (1986). Substance P augments a persistent slow inward calcium-sensitive current in voltage-clamped spinal dorsal horn neurons of the rat. Brain Res. 365, 369–376. doi: 10.1016/0006-8993(86)91652-5
Nelson, T. S., Allen, H. N., Basu, P., Prasoon, P., Nguyen, E., Arokiaraj, C. M., et al. (2023). Alleviation of neuropathic pain with neuropeptide Y requires spinal Npy1r interneurons that coexpress Grp. JCI Insight 8:e169554. doi: 10.1172/jci.insight.169554
Ness, T. J., and Gebhart, G. F. (1989). Characterization of superficial T13-L2 dorsal horn neurons encoding for colorectal distension in the rat: Comparison with neurons in deep laminae. Brain Res. 486, 301–309. doi: 10.1016/0006-8993(89)90516-7
Neugebauer, V., and Schaible, H. G. (1990). Evidence for a central component in the sensitization of spinal neurons with joint input during development of acute arthritis in cat’s knee. J. Neurophysiol. 64, 299–311. doi: 10.1152/jn.1990.64.1.299
Nowak, L. M., and Macdonald, R. L. (1983). Muscarine-sensitive voltage-dependent potassium current in cultured murine spinal cord neurons. Neurosci. Lett. 35, 85–91. doi: 10.1016/0304-3940(83)90531-1
Palecek, J., Palecková, V., Dougherty, P. M., Carlton, S. M., and Willis, W. D. (1992). Responses of spinothalamic tract cells to mechanical and thermal stimulation of skin in rats with experimental peripheral neuropathy. J. Neurophysiol. 67, 1562–1573. doi: 10.1152/jn.1992.67.6.1562
Park, J.-S., Voitenko, N., Petralia, R. S., Guan, X., Xu, J.-T., Steinberg, J. P., et al. (2009). Persistent inflammation induces GluR2 internalization via NMDA receptor-triggered PKC activation in dorsal horn neurons. J. Neurosci. 29, 3206–3219. doi: 10.1523/JNEUROSCI.4514-08.2009
Partridge, L. D., Müller, T. H., and Swandulla, D. (1994). Calcium-activated non-selective channels in the nervous system. Brain Res. Brain Res. Rev. 19, 319–325. doi: 10.1016/0165-0173(94)90017-5
Peirs, C., Dallel, R., and Todd, A. J. (2020). Recent advances in our understanding of the organization of dorsal horn neuron populations and their contribution to cutaneous mechanical allodynia. J. Neural Transm. 127, 505–525. doi: 10.1007/s00702-020-02159-1
Pezet, S., Malcangio, M., and McMahon, S. B. (2002). BDNF: A neuromodulator in nociceptive pathways? Brain Res. Rev. 40, 240–249. doi: 10.1016/S0165-0173(02)00206-0
Pitcher, M. H., and Cervero, F. (2010). Role of the NKCC1 co-transporter in sensitization of spinal nociceptive neurons. Pain 151, 756–762. doi: 10.1016/j.pain.2010.09.008
Polgár, E., Dickie, A. C., Gutierrez-Mecinas, M., Bell, A. M., Boyle, K. A., Quillet, R., et al. (2023). Grpr expression defines a population of superficial dorsal horn vertical cells that have a role in both itch and pain. Pain 164, 149–170. doi: 10.1097/j.pain.0000000000002677
Polgár, E., Durrieux, C., Hughes, D. I., and Todd, A. J. (2013). A quantitative study of inhibitory interneurons in laminae I-III of the mouse spinal dorsal horn. PLoS One 8:e78309. doi: 10.1371/journal.pone.0078309
Prescott, S. A., and De Koninck, Y. (2002). Four cell types with distinctive membrane properties and morphologies in lamina I of the spinal dorsal horn of the adult rat. J. Physiol. 539, 817–836. doi: 10.1113/jphysiol.2001.013437
Prescott, S. A., and De Koninck, Y. (2005). Integration time in a subset of spinal lamina I neurons is lengthened by sodium and calcium currents acting synergistically to prolong subthreshold depolarization. J. Neurosci. 25, 4743–4754. doi: 10.1523/JNEUROSCI.0356-05.2005
Price, D. D., and Dubner, R. (1977). Neurons that subserve the sensory-discriminative aspects of pain. Pain 3, 307–338. doi: 10.1016/0304-3959(77)90063-X
Punnakkal, P., von Schoultz, C., Haenraets, K., Wildner, H., and Zeilhofer, H. U. (2014). Morphological, biophysical and synaptic properties of glutamatergic neurons of the mouse spinal dorsal horn. J. Physiol. 592, 759–776. doi: 10.1113/jphysiol.2013.264937
Qiu, H., Miraucourt, L. S., Petitjean, H., Xu, M., Theriault, C., Davidova, A., et al. (2024). Parvalbumin gates chronic pain through the modulation of firing patterns in inhibitory neurons. Proc. Natl. Acad. Sci. U S A. 121:e2403777121. doi: 10.1073/pnas.2403777121
Quillet, R., Dickie, A. C., Polgár, E., Gutierrez-Mecinas, M., Bell, A. M., Goffin, L., et al. (2023). Characterisation of NPFF-expressing neurons in the superficial dorsal horn of the mouse spinal cord. Sci. Rep. 13:5891. doi: 10.1038/s41598-023-32720-3
Quinn, K. P., Dong, L., Golder, F. J., and Winkelstein, B. A. (2010). Neuronal hyperexcitability in the dorsal horn after painful facet joint injury. Pain 151, 414–421. doi: 10.1016/j.pain.2010.07.034
Radwani, H., Lopez-Gonzalez, M. J., Cattaert, D., Roca-Lapirot, O., Dobremez, E., Bouali-Benazzouz, R., et al. (2016). Cav1.2 and Cav1.3 L-type calcium channels independently control short- and long-term sensitization to pain. J. Physiol. 594, 6607–6626. doi: 10.1113/JP272725
Ranjan, R., Logette, E., Marani, M., Herzog, M., Tâche, V., Scantamburlo, E., et al. (2019). A kinetic map of the homomeric voltage-gated potassium channel (Kv) family. Front. Cell. Neurosci. 13:358. doi: 10.3389/fncel.2019.00358
Reali, C., Fossat, P., Landry, M., Russo, R. E., and Nagy, F. (2011). Intrinsic membrane properties of spinal dorsal horn neurones modulate nociceptive information processing in vivo. J. Physiol. 589, 2733–2743. doi: 10.1113/jphysiol.2011.207712
Rivera-Arconada, I., and Lopez-Garcia, J. A. (2005). Effects of M-current modulators on the excitability of immature rat spinal sensory and motor neurones. Eur. J. Neurosci. 22, 3091–3098. doi: 10.1111/j.1460-9568.2005.04507.x
Rivera-Arconada, I., and Lopez-Garcia, J. A. (2010). Changes in membrane excitability and potassium currents in sensitized dorsal horn neurons of mice pups. J. Neurosci. 30, 5376–5383. doi: 10.1523/JNEUROSCI.4359-09.2010
Rivera-Arconada, I., and Lopez-Garcia, J. A. (2015). Characterisation of rebound depolarisation in mice deep dorsal horn neurons in vitro. Pflugers Arch. 467, 1985–1996. doi: 10.1007/s00424-014-1623-y
Rivera-Arconada, I., Roza, C., and Lopez-Garcia, J. A. (2013). Characterization of hyperpolarization-activated currents in deep dorsal horn neurons of neonate mouse spinal cord in vitro. Neuropharmacology 70, 148–155. doi: 10.1016/j.neuropharm.2013.01.019
Rojas-Piloni, G., Dickenson, A. H., and Condés-Lara, M. (2007). Superficial dorsal horn neurons with double spike activity in the rat. Neurosci. Lett. 419, 147–152. doi: 10.1016/j.neulet.2007.04.032
Roome, R. B., Bourojeni, F. B., Mona, B., Rastegar-Pouyani, S., Blain, R., Dumouchel, A., et al. (2020). Phox2a defines a developmental origin of the anterolateral system in mice and humans. Cell Rep. 33:108425. doi: 10.1016/j.celrep.2020.108425
Roza, C., Mazo, I., Rivera-Arconada, I., Cisneros, E., Alayón, I., and López-García, J. A. (2016). Analysis of spontaneous activity of superficial dorsal horn neurons in vitro: Neuropathy-induced changes. Pflugers Arch. 468, 2017–2030. doi: 10.1007/s00424-016-1886-6
Ruscheweyh, R., and Sandkühler, J. (2002). Lamina-specific membrane and discharge properties of rat spinal dorsal horn neurones in vitro. J. Physiol. 541, 231–244. doi: 10.1113/jphysiol.2002.017756
Ruscheweyh, R., Ikeda, H., Heinke, B., and Sandkühler, J. (2004). Distinctive membrane and discharge properties of rat spinal lamina I projection neurones in vitro. J. Physiol. 555, 527–543. doi: 10.1113/jphysiol.2003.054049
Russo, R. E., and Hounsgaard, J. (1996). Plateau-generating neurones in the dorsal horn in an in vitro preparation of the turtle spinal cord. J. Physiol. 493, 39–54. doi: 10.1113/jphysiol.1996.sp021363
Rycroft, B. K., Vikman, K. S., and Christie, M. J. (2007). Inflammation reduces the contribution of N-type calcium channels to primary afferent synaptic transmission onto NK1 receptor-positive lamina I neurons in the rat dorsal horn. J. Physiol. 580, 883–894. doi: 10.1113/jphysiol.2006.125880
Ryu, P. D., and Randic, M. (1990). Low- and high-voltage-activated calcium currents in rat spinal dorsal horn neurons. J. Neurophysiol. 63, 273–285. doi: 10.1152/jn.1990.63.2.273
Safronov, B. V., Wolff, M., and Vogel, W. (1997). Functional distribution of three types of Na+ channel on soma and processes of dorsal horn neurones of rat spinal cord. J. Physiol. 503, 371–385. doi: 10.1111/j.1469-7793.1997.371bh.x
Sandkühler, J., and Eblen-Zajjur, A. A. (1994). Identification and characterization of rhythmic nociceptive and non-nociceptive spinal dorsal horn neurons in the rat. Neuroscience 61, 991–1006. doi: 10.1016/0306-4522(94)90419-7
Sano, Y., Inamura, K., Miyake, A., Mochizuki, S., Kitada, C., Yokoi, H., et al. (2003). A novel two-pore domain K+ channel, TRESK, is localized in the spinal cord. J. Biol. Chem. 278, 27406–27412. doi: 10.1074/jbc.M206810200
Santos, S. F. A., Luz, L. L., Szucs, P., Lima, D., Derkach, V. A., and Safronov, B. V. (2009). Transmission efficacy and plasticity in glutamatergic synapses formed by excitatory interneurons of the substantia gelatinosa in the rat spinal cord. PLoS One 4:e8047. doi: 10.1371/journal.pone.0008047
Santos, S. F. A., Melnick, I. V., and Safronov, B. V. (2004). Selective postsynaptic inhibition of tonic-firing neurons in substantia gelatinosa by μ-opioid agonist. Anesthesiology 101, 1177–1183. doi: 10.1097/00000542-200411000-00018
Santos, S. F. A., Rebelo, S., Derkach, V. A., and Safronov, B. V. (2007). Excitatory interneurons dominate sensory processing in the spinal substantia gelatinosa of rat. J. Physiol. 581, 241–254. doi: 10.1113/jphysiol.2006.126912
Sardella, T. C. P., Polgár, E., Watanabe, M., and Todd, A. J. (2011). A quantitative study of neuronal nitric oxide synthase expression in laminae I-III of the rat spinal dorsal horn. Neuroscience 192, 708–720. doi: 10.1016/j.neuroscience.2011.07.011
Schappacher, K. A., Xie, W., Zhang, J.-M., and Baccei, M. L. (2019). Neonatal vincristine administration modulates intrinsic neuronal excitability in the rat dorsal root ganglion and spinal dorsal horn during adolescence. Pain 160, 645–657. doi: 10.1097/j.pain.0000000000001444
Schneider, S. P. (2005). Mechanosensory afferent input and neuronal firing properties in rodent spinal laminae III-V: Re-examination of relationships with analysis of responses to static and time-varying stimuli. Brain Res. 1034, 71–89. doi: 10.1016/j.brainres.2004.11.046
Schoffnegger, D., Heinke, B., Sommer, C., and Sandkühler, J. (2006). Physiological properties of spinal lamina II GABAergic neurons in mice following peripheral nerve injury. J. Physiol. 577, 869–878. doi: 10.1113/jphysiol.2006.118034
Seal, R. P., Wang, X., Guan, Y., Raja, S. N., Woodbury, C. J., Basbaum, A. I., et al. (2009). Injury-induced mechanical hypersensitivity requires C-low threshold mechanoreceptors. Nature 462, 651–655. doi: 10.1038/nature08505
Sekiguchi, K. J., Shekhtmeyster, P., Merten, K., Arena, A., Cook, D., Hoffman, E., et al. (2016). Imaging large-scale cellular activity in spinal cord of freely behaving mice. Nat. Commun. 7:11450. doi: 10.1038/ncomms11450
Semyanov, A., Walker, M. C., Kullmann, D. M., and Silver, R. A. (2004). Tonically active GABA A receptors: modulating gain and maintaining the tone. Trends Neurosci. 27, 262–269. doi: 10.1016/j.tins.2004.03.005
Sharif, B., Ase, A. R., Ribeiro-da-Silva, A., and Séguéla, P. (2020). Differential Coding of Itch and Pain by a Subpopulation of Primary Afferent Neurons. Neuron 106, 940–951.e4. doi: 10.1016/j.neuron.2020.03.021
Shatz, C. J., and Stryker, M. P. (1988). Prenatal tetrodotoxin infusion blocks segregation of retinogeniculate afferents. Science 242, 87–89. doi: 10.1126/science.3175636
Shekhtmeyster, P., Carey, E. M., Duarte, D., Ngo, A., Gao, G., Nelson, N. A., et al. (2023). Multiplex translaminar imaging in the spinal cord of behaving mice. Nat. Commun. 14:1427. doi: 10.1038/s41467-023-36959-2
Sheroziya, M. G., von Bohlen, Und Halbach, O., Unsicker, K., and Egorov, A. V. (2009). Spontaneous bursting activity in the developing entorhinal cortex. J. Neurosci. 29, 12131–12144. doi: 10.1523/JNEUROSCI.1333-09.2009
Shi, Y., Abe, C., Holloway, B. B., Shu, S., Kumar, N. N., Weaver, J. L., et al. (2016). Nalcn is a “Leak” sodium channel that regulates excitability of brainstem chemosensory neurons and breathing. J. Neurosci. 36, 8174–8187. doi: 10.1523/JNEUROSCI.1096-16.2016
Sinha, G. P., Prasoon, P., Smith, B. N., and Taylor, B. K. (2021). Fast A-type currents shape a rapidly adapting form of delayed short latency firing of excitatory superficial dorsal horn neurons that express the neuropeptide Y Y1 receptor. J. Physiol. 599, 2723–2750. doi: 10.1113/JP281033
Smith, K. M., Boyle, K. A., Madden, J. F., Dickinson, S. A., Jobling, P., Callister, R. J., et al. (2015). Functional heterogeneity of calretinin-expressing neurons in the mouse superficial dorsal horn: Implications for spinal pain processing. J. Physiol. 593, 4319–4339. doi: 10.1113/JP270855
Smith, K. M., Browne, T. J., Davis, O. C., Coyle, A., Boyle, K. A., Watanabe, M., et al. (2019). Calretinin positive neurons form an excitatory amplifier network in the spinal cord dorsal horn. Elife 8:e49190. doi: 10.7554/eLife.49190
Spike, R. C., Puskár, Z., Andrew, D., and Todd, A. J. (2003). A quantitative and morphological study of projection neurons in lamina I of the rat lumbar spinal cord. Eur. J. Neurosci. 18, 2433–2448. doi: 10.1046/j.1460-9568.2003.02981.x
Sullivan, S. J., and Sdrulla, A. D. (2022). Excitatory and inhibitory neurons of the spinal cord superficial dorsal horn diverge in their somatosensory responses and plasticity in vivo. J. Neurosci. 42, 1958–1973. doi: 10.1523/JNEUROSCI.1860-21.2021
Suzuki, R., and Dickenson, A. H. (2006). Differential pharmacological modulation of the spontaneous stimulus-independent activity in the rat spinal cord following peripheral nerve injury. Exp. Neurol. 198, 72–80. doi: 10.1016/j.expneurol.2005.10.032
Tadros, M. A., Zouikr, I., Hodgson, D. M., and Callister, R. J. (2018). Excitability of rat superficial dorsal horn neurons following a neonatal immune challenge. Front. Neurol. 9:743. doi: 10.3389/fneur.2018.00743
Takahashi, A., Mashimo, T., and Uchida, I. (2006). GABAergic tonic inhibition of substantia gelatinosa neurons in mouse spinal cord. Neuroreport 17, 1331–1335. doi: 10.1097/01.wnr.0000230515.86090.bc
Takazawa, T., and MacDermott, A. B. (2010). Glycinergic and GABAergic tonic inhibition fine tune inhibitory control in regionally distinct subpopulations of dorsal horn neurons. J. Physiol. 588, 2571–2587. doi: 10.1113/jphysiol.2010.188292
Tazerart, S., Vinay, L., and Brocard, F. (2008). The persistent sodium current generates pacemaker activities in the central pattern generator for locomotion and regulates the locomotor rhythm. J. Neurosci. 28, 8577–8589. doi: 10.1523/JNEUROSCI.1437-08.2008
Thaweerattanasinp, T., Heckman, C. J., and Tysseling, V. M. (2016). Firing characteristics of deep dorsal horn neurons after acute spinal transection during administration of agonists for 5-HT1B/1D and NMDA receptors. J. Neurophysiol. 116, 1644–1653. doi: 10.1152/jn.00198.2016
Thomson, A. M., West, D. C., and Headley, P. M. (1989). Membrane characteristics and synaptic responsiveness of superficial dorsal horn neurons in a slice preparation of adult rat spinal cord. Eur. J. Neurosci. 1, 479–488. doi: 10.1111/j.1460-9568.1989.tb00354.x
Todd, A. J. (2010). Neuronal circuitry for pain processing in the dorsal horn. Nat. Rev. Neurosci. 11, 823–836. doi: 10.1038/nrn2947
Tong, C.-K., and MacDermott, A. B. (2006). Both Ca2+-permeable and -impermeable AMPA receptors contribute to primary synaptic drive onto rat dorsal horn neurons. J. Physiol. 575, 133–144. doi: 10.1113/jphysiol.2006.110072
Torsney, C., and MacDermott, A. B. (2006). Disinhibition opens the gate to pathological pain signaling in superficial neurokinin 1 receptor-expressing neurons in rat spinal cord. J. Neurosci. 26, 1833–1843. doi: 10.1523/JNEUROSCI.4584-05.2006
Tritsch, N. X., Yi, E., Gale, J. E., Glowatzki, E., and Bergles, D. E. (2007). The origin of spontaneous activity in the developing auditory system. Nature 450, 50–55. doi: 10.1038/nature06233
Vicente-Baz, J., Lopez-Garcia, J. A., and Rivera-Arconada, I. (2016). Effects of novel subtype selective M-current activators on spinal reflexes in vitro: Comparison with retigabine. Neuropharmacology 109, 131–138. doi: 10.1016/j.neuropharm.2016.05.025
Voitenko, N. V., Kruglikov, I. A., Kostyuk, E. P., and Kostyuk, P. G. (2000). Effect of streptozotocin-induced diabetes on the activity of calcium channels in rat dorsal horn neurons. Neuroscience 95, 519–524. doi: 10.1016/s0306-4522(99)00453-4
Wall, P. D. (1967). The laminar organization of dorsal horn and effects of descending impulses. J. Physiol. 188, 403–423. doi: 10.1113/jphysiol.1967.sp008146
Wall, P. D., Merrill, E. G., and Yaksh, T. L. (1979). Responses of single units in laminae 2 and 3 of cat spinal cord. Brain Res. 160, 245–260. doi: 10.1016/0006-8993(79)90422-0
Walsh, M. A., Graham, B. A., Brichta, A. M., and Callister, R. J. (2009). Evidence for a critical period in the development of excitability and potassium currents in mouse lumbar superficial dorsal horn neurons. J. Neurophysiol. 101, 1800–1812. doi: 10.1152/jn.90755.2008
Wan, L., Jin, H., Liu, X.-Y., Jeffry, J., Barry, D. M., Shen, K.-F., et al. (2017). Distinct roles of NMB and GRP in itch transmission. Sci. Rep. 7:15466. doi: 10.1038/s41598-017-15756-0
Weng, H.-R., Cordella, J. V., and Dougherty, P. M. (2003). Changes in sensory processing in the spinal dorsal horn accompany vincristine-induced hyperalgesia and allodynia. Pain 103, 131–138. doi: 10.1016/s0304-3959(02)00445-1
Willis, W. D. (1999). Dorsal root potentials and dorsal root reflexes: a double-edged sword. Exp. Brain Res. 124, 395–421. doi: 10.1007/s002210050637
Willis, W. D., and Coggeshall, R. E. (2004). Sensory Mechanisms of The Spinal Cord, 3rd Edn, Vol 1. Alphen aan den Rijn: Kluver Academic.
Wolff, M., Vogel, W., and Safronov, B. V. (1998). Uneven distribution of K+ channels in soma, axon and dendrites of rat spinal neurones: functional role of the soma in generation of action potentials. J. Physiol. 509, 767–776. doi: 10.1111/j.1469-7793.1998.767bm.x
Woolf, C. J. (1983). Evidence for a central component of post-injury pain hypersensitivity. Nature 306, 686–688. doi: 10.1038/306686a0
Woolf, C. J. (1996). Phenotypic modification of primary sensory neurons: The role of nerve growth factor in the production of persistent pain. Philos. Trans. R. Soc. Lond. B 351, 441–448. doi: 10.1098/rstb.1996.0040
Woolf, C. J., and King, A. E. (1989). Subthreshold components of the cutaneous mechanoreceptive fields of dorsal horn neurons in the rat lumbar spinal cord. J. Neurophysiol. 62, 907–916. doi: 10.1152/jn.1989.62.4.907
Woolf, C. J., and Thompson, S. W. N. (1991). The induction and maintenance of central sensitization is dependent on N-methyl-D-aspartic acid receptor activation; Implications for the treatment of post-injury pain hypersensitivity states. Pain 44, 293–299. doi: 10.1016/0304-3959(91)90100-C
Woolf, C. J., Thompson, S. W., and King, A. E. (1988). Prolonged primary afferent induced alterations in dorsal horn neurones, an intracellular analysis in vivo and in vitro. J. Physiol. 83, 255–266.
Wu, J., Peng, S., Xiao, L., Cheng, X., Kuang, H., Zhu, M., et al. (2018). Cell-type specific distribution of T-Type calcium currents in lamina II neurons of the rat spinal cord. Front. Cell. Neurosci. 12:370. doi: 10.3389/fncel.2018.00370
Wu, L.-J., Duan, B., Mei, Y.-D., Gao, J., Chen, J.-G., Zhuo, M., et al. (2004). Characterization of acid-sensing ion channels in dorsal horn neurons of rat spinal cord. J. Biol. Chem. 279, 43716–43724. doi: 10.1074/jbc.M403557200
Xiao, W., Boroujerdi, A., Bennett, G. J., and Luo, Z. D. (2007). Chemotherapy-evoked painful peripheral neuropathy: analgesic effects of gabapentin and effects on expression of the alpha-2-delta type-1 calcium channel subunit. Neuroscience 144, 714–720. doi: 10.1016/j.neuroscience.2006.09.044
Yadav, A., Matson, K. J. E., Li, L., Hua, I., Petrescu, J., Kang, K., et al. (2023). A cellular taxonomy of the adult human spinal cord. Neuron 111, 328–344.e7. doi: 10.1016/j.neuron.2023.01.007
Yang, K. (2016). Regulation of excitability in tonic firing substantia gelatinosa neurons of the spinal cord by small-conductance Ca(2+)-activated K(+) channels. Neuropharmacology 105, 15–24. doi: 10.1016/j.neuropharm.2016.01.001
Yang, L., Zhang, F.-X., Huang, F., Lu, Y.-J., Li, G.-D., Bao, L., et al. (2004). Peripheral nerve injury induces trans-synaptic modification of channels, receptors and signal pathways in rat dorsal spinal cord. Eur. J. Neurosci. 19, 871–883. doi: 10.1111/j.0953-816x.2004.03121.x
Yasaka, T., Tiong, S. Y. X., Hughes, D. I., Riddell, J. S., and Todd, A. J. (2010). Populations of inhibitory and excitatory interneurons in lamina II of the adult rat spinal dorsal horn revealed by a combined electrophysiological and anatomical approach. Pain 151, 475–488. doi: 10.1016/j.pain.2010.08.008
Yasaka, T., Tiong, S. Y., Polgár, E., Watanabe, M., Kumamoto, E., Riddell, J. S., et al. (2014). A putative relay circuit providing low-threshold mechanoreceptive input to lamina I projection neurons via vertical cells in lamina II of the rat dorsal horn. Mol. Pain 10:3. doi: 10.1186/1744-8069-10-3
Yoo, S., Santos, C., Reynders, A., Marics, I., Malapert, P., Gaillard, S., et al. (2021). TAFA4 relieves injury-induced mechanical hypersensitivity through LDL receptors and modulation of spinal A-type K+ current. Cell. Rep. 37:109884. doi: 10.1016/j.celrep.2021.109884
Yoshimura, M., and Jessell, T. (1990). Amino acid-mediated EPSPs at primary afferent synapses with substantia gelatinosa neurones in the rat spinal cord. J. Physiol. 430, 315–335. doi: 10.1113/jphysiol.1990.sp018293
Yoshimura, M., and Jessell, T. M. (1989a). Membrane properties of rat substantia gelatinosa neurons in vitro. J. Neurophysiol. 62, 109–118. doi: 10.1152/jn.1989.62.1.109
Yoshimura, M., and Jessell, T. M. (1989b). Primary afferent-evoked synaptic responses and slow potential generation in rat substantia gelatinosa neurons in vitro. J. Neurophysiol. 62, 96–108. doi: 10.1152/jn.1989.62.1.96
Yoshimura, M., and Nishi, S. (1995). Primary afferent-evoked glycine- and GABA-mediated IPSPs in substantia gelatinosa neurones in the rat spinal cord in vitro. J. Physiol. 482, 29–38. doi: 10.1113/jphysiol.1995.sp020497
Yu, F., Zhao, Z.-Y., He, T., Yu, Y.-Q., Li, Z., and Chen, J. (2017). Temporal and spatial dynamics of peripheral afferent-evoked activity in the dorsal horn recorded in rat spinal cord slices. Brain Res. Bull. 131, 183–191. doi: 10.1016/j.brainresbull.2017.04.012
Zain, M., and Bonin, R. P. (2019). Alterations in evoked and spontaneous activity of dorsal horn wide dynamic range neurons in pathological pain: A systematic review and analysis. Pain 160, 2199–2209. doi: 10.1097/j.pain.0000000000001632
Zeilhofer, H. U. (2008). Loss of glycinergic and GABAergic inhibition in chronic pain—contributions of inflammation and microglia. Int. Immunopharmacol. 8, 182–187. doi: 10.1016/j.intimp.2007.07.009
Zhang, D., Zhao, W., Liu, J., Ou, M., Liang, P., Li, J., et al. (2021). Sodium leak channel contributes to neuronal sensitization in neuropathic pain. Prog. Neurobiol. 202:102041. doi: 10.1016/j.pneurobio.2021.102041
Zhang, Y., Liu, S., Zhang, Y.-Q., Goulding, M., Wang, Y.-Q., and Ma, Q. (2018). Timing mechanisms underlying gate control by feedforward inhibition. Neuron 99, 941–955.e4. doi: 10.1016/j.neuron.2018.07.026
Zhi, Y.-R., Cao, F., Su, X.-J., Gao, S.-W., Zheng, H.-N., Jiang, J.-Y., et al. (2022). The T-type calcium channel Cav3.2 in somatostatin interneurons in spinal dorsal horn participates in mechanosensation and mechanical allodynia in mice. Front. Cell. Neurosci. 16:875726. doi: 10.3389/fncel.2022.875726
Zhu, M., Yan, Y., Cao, X., Zeng, F., Xu, G., Shen, W., et al. (2021). Electrophysiological and morphological features of rebound depolarization characterized interneurons in rat superficial spinal dorsal horn. Front. Cell Neurosci. 15:736879. doi: 10.3389/fncel.2021.736879
Keywords: spinal cord, nociception, electrophysiological techniques, ionic channels, action potentials, dorsal horn neurons, firing pattern
Citation: Rivera-Arconada I, Baccei ML, López-García JA and Bardoni R (2025) An electrophysiologist’s guide to dorsal horn excitability and pain. Front. Cell. Neurosci. 19:1548252. doi: 10.3389/fncel.2025.1548252
Received: 19 December 2024; Accepted: 13 March 2025;
Published: 02 April 2025.
Edited by:
Adalberto Merighi, University of Turin, ItalyReviewed by:
Erika S. Piedras-Renteria, Loyola University Chicago, United StatesCopyright © 2025 Rivera-Arconada, Baccei, López-García and Bardoni. This is an open-access article distributed under the terms of the Creative Commons Attribution License (CC BY). The use, distribution or reproduction in other forums is permitted, provided the original author(s) and the copyright owner(s) are credited and that the original publication in this journal is cited, in accordance with accepted academic practice. No use, distribution or reproduction is permitted which does not comply with these terms.
*Correspondence: Rita Bardoni, cml0YS5iYXJkb25pQHVuaW1vcmUuaXQ=
†These authors have contributed equally to this work
Disclaimer: All claims expressed in this article are solely those of the authors and do not necessarily represent those of their affiliated organizations, or those of the publisher, the editors and the reviewers. Any product that may be evaluated in this article or claim that may be made by its manufacturer is not guaranteed or endorsed by the publisher.
Research integrity at Frontiers
Learn more about the work of our research integrity team to safeguard the quality of each article we publish.