- 1Biodigital Innovation Lab, Translational Bioengineering Department, Exact Sciences and Engineering University Center, Universidad de Guadalajara, Guadalajara, Mexico
- 2Translational Bioengineering Department, Exact Sciences and Engineering University Center, Universidad de Guadalajara, Guadalajara, Mexico
Introduction
Non-static Neuron identity emerges from the complementary synaptic transcriptional architecture shaped by neuron signaling and surrounding non-neuronal cells. When signals from a series of neurons are combined, they form circuits through which information flows, and brain functioning occurs as a superposition of these neural circuits. To ensure proper communication and function, neurons develop complementary phenotypes of axonal projections and electrophysiological behaviors, creating gradients that define brain regions and allocate neurochemical functions (Vogel et al., 2024).
In the mature nervous system, axonal projections extend over long distances and communicate with different well-established regions (Pal et al., 2024) in both peripheral nervous systems (PNS) and central nervous systems (CNS). Information flows inside each axon despite the great distance, resulting in immediate responses.
The mechanism involved in such responses has been described as a soma-centric notion, where the neuronal soma provides total molecular information through the axon (Dalla Costa et al., 2021). However, Nijssen et al. (2018) showed evidence of differences between the axonal transcriptome and the soma transcriptome from Spinal Motor Neurons (MNs).
Several authors suggest that, given these differences, the soma alone may not provide all transcriptomic information. Instead, surrounding glial cells accompanying the axon along its pathway may supply some transcriptomic information. These glial cells play an essential role in proper brain function (Dalla Costa et al., 2021; Giuditta et al., 2008).
The described glia-to-axon relationship becomes particularly significant in the CNS, where different brain regions have distinct molecular, cellular, and functional characteristics (Vogel et al., 2024; Siletti et al., 2023). As axons extend through various brain areas, their transcriptome profiles differ from their soma's (Nijssen et al., 2018). This molecular variation suggests that axons adapt their transcriptomic identity to match their local cellular environment as they traverse different brain regions. The local glial cells provide specific transcriptional resources to the axon in each area. We term this space-dependent, dynamic identity adaptation of neuronal axons the “neuronal continuum.”
Axonal identity differ from neuronal soma
Modern neuroscience has produced high-resolution cell classifications of the brain based on expression profiles from single-cell sequencing techniques such as Single-Nucleus RNA sequencing (snRNA-seq) (Siletti et al., 2023). This technique focuses on the transcriptional cell identity from the cell nuclei (Hodge et al., 2019). These atlases provide us with CNS cellular cartography for most brain regions of some species (Siletti et al., 2023; Yao et al., 2023; Chen et al., 2023), and they typically report two main groups of neurons—glutamatergic and GABAergic—together with a third non-neuronal cell lineage known as glia, which plays critical regulatory roles throughout the brain (Liu et al., 2023).
Different cognitive functions are region-associated and require specific configurations of axon-dendrite junctions (Dalla Costa et al., 2021), which determine how information flows. These physical pathways, where dendrite and axon morphology can change, are crucial for neural communication. Recent research has shown that changes in these junctions often accompany regulation in local gene expression (Gao et al., 2023).
Nijssen et al. (2018), developed a method for sequencing the transcriptome from a single axon, similar to snRNA-seq, called Axon-seq. They sequenced axons from MNs, finding that the axonal transcriptomic profile differs from its soma in a single neuron. More interestingly, a unique transcription factor signature was found in distal axons that was not found in any of the soma reported, leading us to some questions: Who is responsible for this transcriptomic identity switch, and why does this happen?
Glia-to-axon communication
Glial cells constitute half of the cell population in the mammalian nervous system. The glia-to-neuron ratio varies across brain structures and species (Liu et al., 2023). They are classified into different types: astrocytes, microglia, synantocytes (Tizabi et al., 2024), oligodendrocytes, and Schwann cells, the last two being the myelin cells of the nervous system. Similar scenarios exist in PNS and the CNS, where glial cells and neurons maintain a close relationship. Glial cells residing at neuronal junctions or within axonal segments play crucial roles in providing fundamental communication support for neuronal survival (Liu et al., 2023).
Recent findings have highlighted the fundamental contribution of glial cells to brain function. Glial cells show shifting characteristics in the myelin sheaths (Xin and Chan, 2020). Glial cells are also very diverse, with varying and specific subtypes and proportions of cellular neighborhoods along brain regions; each neighborhood has its own types of neurons and glia (Siletti et al., 2023).
Giuditta et al. (2008) described glia-to-axon mechanisms from direct evidence with the squid giant axon, showing that beyond simple communication between the glia surrounding the soma/axon, the glia provides the molecular content to the PNS axon. Studies have also reported glia-to-axon communication with Schwann cells that interact with axons to support and deliver genetic material and machinery (Das et al., 2021). Court et al. (2008) and later, Cada and Mizuno (2024), demonstrated the flow of ribosomes from the glia into the axon. Recently, other authors (Krämer-Albers and Werner, 2023) have explained the glia-to-axon communication mechanism between oligodendrocytes and axons, describing the mechanism of exosome (cargo with molecular information) exchange oligodendrocyte-to-axon.
Neuron and glia transcription
Besides PNS neurons, where axons can reach up to a meter in humans (Twiss and Fainzilber, 2009), CNS axons can travel long distances through the layers and regions of the brain. The frontotemporal arcuate fasciculus (AF) is a well-studied pathway that connects different brain regions. This white matter bundle involved in language processing is 4–5 cm long (Basile et al., 2024).
Despite the long distance, a soma-centric delivery was the primary proposed mechanism for mRNA transport (Dalla Costa et al., 2021). However, Twiss and Fainzilber (2009) reported an anterograde mRNA transport rate of only 16 mm/h, suggesting that relying solely on the nuclear transcription mechanism or any other mechanisms from nuclei only as mRNA synthesis is insufficient due to anterograde and retrograde pathway that information needed to travel. These observations highlight the limitations of single transcriptional machinery in the nucleus to support long axons.
Gradients and scales
Gene expression gradients were initially described in a rostral-caudal direction (Fornito et al., 2019). Lau et al. (2021) shows a gradual decay of gene expression according to physical distance in the mouse cortex. Furthermore, Vogel et al. (2024), described three principal axes of gene expression gradients aligned with the brain's anatomical architecture.
In addition, snRNA-seq studies showed continuity for major neuronal populations in the adult human and mouse brains (Siletti et al., 2023; Hodge et al., 2019), describing gradients of local cell type identity within each brain region.
Current neuroscience explores the brain across multiple scales (micro-meso-macro scales), each defined by its informational unit. For example, at the mesoscale, the unit would be a set of neighboring cells with locally similar gene expression. From this shared expression, other characteristics emerge, i.e., morphology, function, and interconnectivity (Poulin et al., 2016). Each cellular neighborhood is fuzzily self-defined in a physical space in the brain, and neighborhood interconnectivity will require those boundaries to shift dynamically their gene expression in microgradients. Individual axons whose segments cross different neighborhoods will require multiple local transcriptional sources to thrive.
Neuronal continuum
Since continuity has been reported across different scales, it is plausible that this phenomenon also occurs within individual neurons. Each brain region is molecularly, cellularly, and functionally distinct and has gene expression patterns necessary for proper cerebral function.
While information flows along the axons that travel long distances, they visit different brain regions and change their morphology and gene expression. Each axonal segment acquires a distinct transcriptional identity from the soma, combining the nuclear molecular content with the one supplied by the surrounding glial cells in their neighborhood (Farias et al., 2020). Such neuronal continuum means neurons do not show a single identity but a continuous molecular profile collectively established by axonal segments and the soma, dependent on the cellular neighborhood (Figure 1).
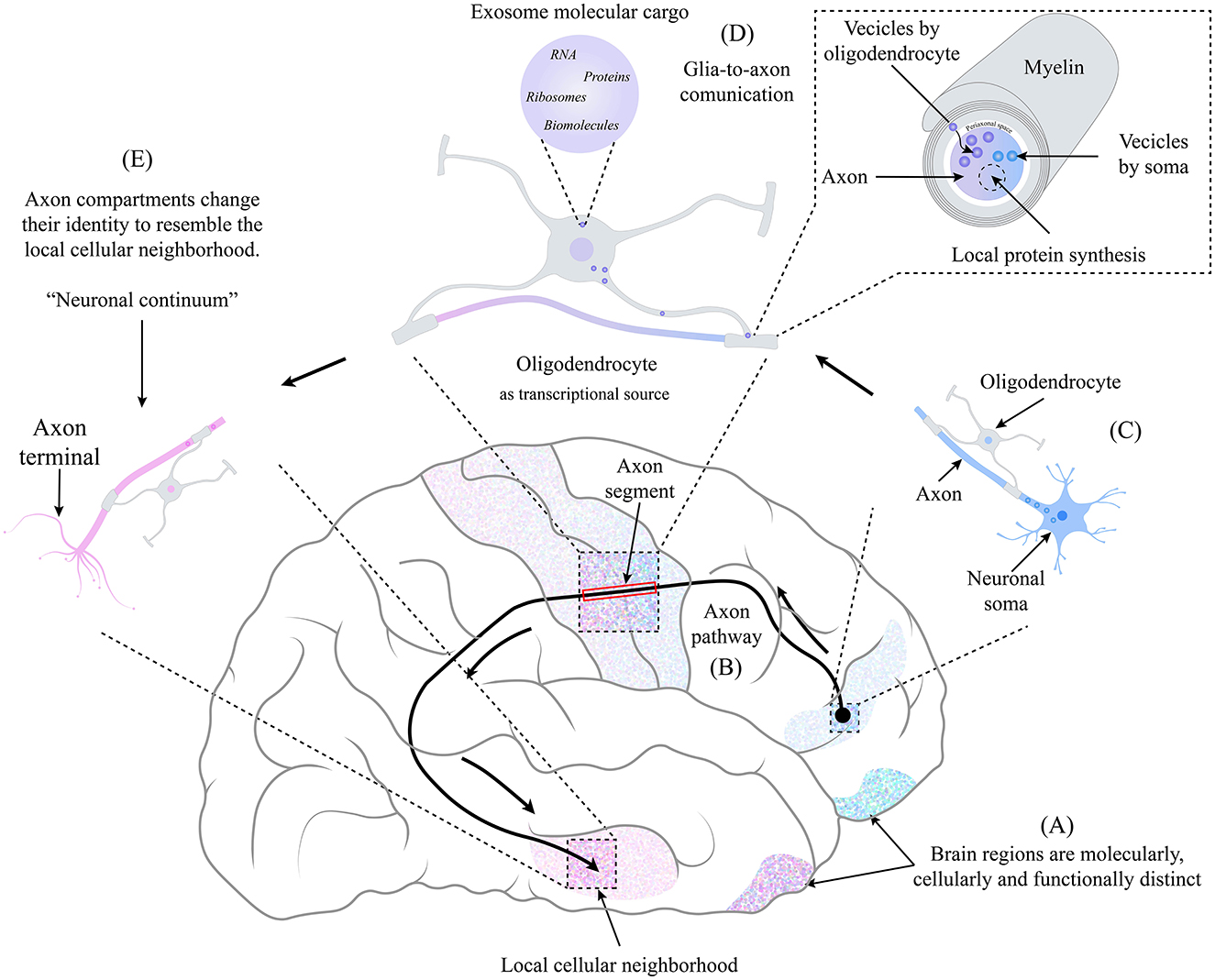
Figure 1. The identity of an axonal compartment switches in the function of the physical location through which it moves, resembling the identity of the local cellular neighborhood. (A) Each brain region is molecularly, cellularly, and functionally distinct. (B) Axonal pathways may be longer. The frontotemporal arcuate fasciculus is a well-studied pathway connecting different brain regions. (C) At the beginning of the axonal path, the neuronal body is in a specific brain region as its axon begins its pathway. On the way, the axon is accompanied by oligodendrocytes (and other glial cells), and a very close relationship is maintained. (D) Due to their relationship, a glia-to-axon communication occurs, where, through the mechanism of exosome transfer (Krämer-Albers and Werner, 2023), the oligodendrocyte provides the necessary information and molecular machinery to the axonal segment, which depends on the cellular neighborhood in which it is located. Giving rise to the local synthesis of proteins that, combined with the molecular content coming from the soma (Dalla Costa et al., 2021), produces a switch of identity resembling the cellular neighborhood. (E) at the end of the axonal pathway, each axonal compartment has an identity distinct from the neuronal soma and other axonal compartments, creating a non-static identity space-dependent that we term “Neuronal Continuum.”
We propose that oligodendrocytes, and secondarily other glial cells, serve as multiple transcriptional sources glia-to-axon, providing molecular machinery and content to axonal compartments in the CNS, similar to the role of Schwann cells in the PNS. Recent findings demonstrate that oligodendrocytes play a leading role in regulating neural synapse development, synaptic transmission, and plasticity (Liu et al., 2023; Xin and Chan, 2020).
Discussion
The neuronal continuum impacts myelin-associated diseases and other neuronal disorders. Nijssen et al. (2018) compared the axonal transcriptomic profile from healthy and amyotrophic lateral sclerosis MNs, demonstrating a differential expression of 121 mRNAs necessary for the property neuron function. Also, oligodendrocyte heterogeneity is implicated in conditions such as Multiple Sclerosis. In this typical demyelination disease, it was shown that differences in oligodendrocyte subtypes between control and patients could contribute to inflammation (Jäkel et al., 2019), possibly due to a disruption of molecular supply at certain axon segments derived from missing oligodendrocyte subtypes. Recent studies have also reported that myelin-related cells impact conditions commonly associated with neuronal disorders (Murdock and Tsai, 2023). By acknowledging the essential role of glia-to-axon for spatially dependent molecular resources, we can better understand the complex interplay between neurons and glia in health and disease.
The traditional view of neurons possessing a fixed identity defined solely by their intrinsic properties is being challenged by emerging evidence of a neuronal continuum. Recognizing that neuron identity is not static but spatially dynamic, influenced by surrounding glial cells within a cellular neighborhood, offers a new understanding of how neuronal identity is established and maintained. By exploring the neuronal continuum, we can improve our understanding of various conditions, such as axon-related diseases, to design novel interventions for neurodegenerative diseases. It also opens new avenues for research into neural development, regeneration, and therapeutic strategies targeting glial-neuronal interactions.
Author contributions
GG: Conceptualization, Investigation, Supervision, Writing – original draft, Writing – review & editing. OP: Conceptualization, Writing – original draft, Writing – review & editing. RR-V: Conceptualization, Supervision, Writing – review & editing. HV-P: Conceptualization, Supervision, Writing – review & editing. JAM: Conceptualization, Supervision, Writing – review & editing.
Funding
The author(s) declare financial support was received for the research, authorship, and/or publication of this article. GG is the receptor of a master degree fellowship from CONAHCyT (CVU 1184258).
Acknowledgments
The authors express immense gratitude for the Biodigital Innovation Lab (BIL)'s innovative visualizations, simulations, and modeling that significantly aided their research endeavors. They jovially liken BIL's futuristic and mind-bending digital depictions of biological systems to jacking into the bio-matrix and accessing cheat codes. GG wants to thank the unconditional support of Sorpresa. Gracias Gatito.
Conflict of interest
The authors declare that the research was conducted in the absence of any commercial or financial relationships that could be construed as a potential conflict of interest.
Generative AI statement
The author(s) declare that Gen AI was used in the creation of this manuscript. During the preparation of this work, the author(s) used Grammarly in order to review grammar and writing, ChatGPT was used to improve text and coherence writing, as well as DeepL and DeepL Write were used to translate and improve academic redaction respectively. After using For Review Only these tools, the author(s) reviewed and edited the content as needed and took full responsibility for the content of the publication.
Publisher's note
All claims expressed in this article are solely those of the authors and do not necessarily represent those of their affiliated organizations, or those of the publisher, the editors and the reviewers. Any product that may be evaluated in this article, or claim that may be made by its manufacturer, is not guaranteed or endorsed by the publisher.
References
Basile, G. A., Nozais, V., Quartarone, A., Giustiniani, A., Ielo, A., Cerasa, A., et al. (2024). Functional anatomy and topographical organization of the frontotemporal arcuate fasciculus. Commun. Biol. 7:1655. doi: 10.1038/s42003-024-07274-3
Cada, A. K., and Mizuno, N. (2024). Molecular cartography within axons. Curr. Opin. Cell Biol. 88:102358. doi: 10.1016/j.ceb.2024.102358
Chen, A., Sun, Y., Lei, Y., Li, C., Liao, S., Meng, J., et al. (2023). Single-cell spatial transcriptome reveals cell-type organization in the macaque cortex. Cell 186, 3726–3743.e24. doi: 10.1016/j.cell.2023.06.009
Court, F. A., Hendriks, W. T. J., MacGillavry, H. D., Alvarez, J., and Van Minnen, J. (2008). Schwann cell to axon transfer of ribosomes: toward a novel understanding of the role of glia in the nervous system. J. Neurosci. 28, 11024–11029. doi: 10.1523/JNEUROSCI.2429-08.2008
Dalla Costa, I., Buchanan, C. N., Zdradzinski, M. D., Sahoo, P. K., Smith, T. P., Thames, E., et al. (2021). The functional organization of axonal mRNA transport and translation. Nat. Rev. Neurosci. 22, 77–91. doi: 10.1038/s41583-020-00407-7
Das, S., Vera, M., Gandin, V., Singer, R. H., and Tutucci, E. (2021). Intracellular mRNA transport and localized translation. Nat. Rev. Mol. Cell Biol. 22, 483–504. doi: 10.1038/s41580-021-00356-8
Farias, J., Holt, C. E., Sotelo, J. R., and Sotelo-Silveira, J. R. (2020). Axon microdissection and transcriptome profiling reveals the in vivo RNA content of fully differentiated myelinated motor axons. RNA 26, 595–612. doi: 10.1261/rna.073700.119
Fornito, A., Arnatkevičiutė, A., and Fulcher, B. D. (2019). Bridging the gap between connectome and transcriptome. Trends Cogn. Sci. 23, 34–50. doi: 10.1016/j.tics.2018.10.005
Gao, L., Liu, S., Wang, Y., Wu, Q., Gou, L., and Yan, J. (2023). Single-neuron analysis of dendrites and axons reveals the network organization in mouse prefrontal cortex. Nat. Neurosci. 26, 1111–1126. doi: 10.1038/s41593-023-01339-y
Giuditta, A., Tai Chun, J., Eyman, M., Cefaliello, C., Bruno, A. P., and Crispino, M. (2008). Local gene expression in axons and nerve endings: the glia-neuron unit. Physiol. Rev. 88, 515–555. doi: 10.1152/physrev.00051.2006
Hodge, R. D., Bakken, T. E., Miller, J. A., Smith, K. A., Barkan, E. R., Graybuck, L. T., et al. (2019). Conserved cell types with divergent features in human versus mouse cortex. Nature 573, 61–68. doi: 10.1038/s41586-019-1506-7
Jäkel, S., Agirre, E., Mendanha Falcão, A., Van Bruggen, D., Lee, K. W., Knuesel, I., et al. (2019). Altered human oligodendrocyte heterogeneity in multiple sclerosis. Nature 566, 543–547. doi: 10.1038/s41586-019-0903-2
Krämer-Albers, E.-M., and Werner, H. B. (2023). Mechanisms of axonal support by oligodendrocyte-derived extracellular vesicles. Nat. Rev. Neurosci. 24, 474–486. doi: 10.1038/s41583-023-00711-y
Lau, H. Y. G., Fornito, A., and Fulcher, B. D. (2021). Scaling of gene transcriptional gradients with brain size across mouse development. Neuroimage 224:117395. doi: 10.1016/j.neuroimage.2020.117395
Liu, Y., Shen, X., Zhang, Y., Zheng, X., Cepeda, C., Wang, Y., et al. (2023). Interactions of glial cells with neuronal synapses, from astrocytes to microglia and oligodendrocyte lineage cells. Glia 71, 1383–1401. doi: 10.1002/glia.24343
Murdock, M. H., and Tsai, L.-H. (2023). Insights into Alzheimer's disease from single-cell genomic approaches. Nat. Neurosci. 26, 181–195. doi: 10.1038/s41593-022-01222-2
Nijssen, J., Aguila, J., Hoogstraaten, R., Kee, N., and Hedlund, E. (2018). Axon-seq decodes the motor axon transcriptome and its modulation in response to ALS. Stem Cell Reports 11, 1565–1578. doi: 10.1016/j.stemcr.2018.11.005
Pal, S., Lim, J. W. C., and Richards, L. J. (2024). Diverse axonal morphologies of individual callosal projection neurons reveal new insights into brain connectivity. Curr. Opin. Neurobiol. 84:102837. doi: 10.1016/j.conb.2023.102837
Poulin, J.-F., Tasic, B., Hjerling-Leffler, J., Trimarchi, J. M., and Awatramani, R. (2016). Disentangling neural cell diversity using single-cell transcriptomics. Nat. Neurosci. 19, 1131–1141. doi: 10.1038/nn.4366
Siletti, K., Hodge, R., Mossi Albiach, A., Lee, K. W., Ding, S.-L., Hu, L., et al. (2023). Transcriptomic diversity of cell types across the adult human brain. Science 382:eadd7046. doi: 10.1126/science.add7046
Tizabi, Y., Getachew, B., Hauser, S. R., Tsytsarev, V., Manhães, A. C., and Da Silva, V. D. A. (2024). Role of glial cells in neuronal function, mood disorders, and drug addiction. Brain Sci. 14:558. doi: 10.3390/brainsci14060558
Twiss, J. L., and Fainzilber, M. (2009). Ribosomes in axons – scrounging from the neighbors? Trends Cell Biol. 19, 236–243. doi: 10.1016/j.tcb.2009.02.007
Vogel, J. W., Alexander-Bloch, A. F., Wagstyl, K., Bertolero, M. A., Markello, R. D., Pines, A., et al. (2024). Deciphering the functional specialization of whole-brain spatiomolecular gradients in the adult brain. Proc. Natl. Acad. Sci. U.S.A. 121:e2219137121. doi: 10.1073/pnas.2219137121
Xin, W., and Chan, J. R. (2020). Myelin plasticity: sculpting circuits in learning and memory. Nat. Rev. Neurosci. 21, 682–694. doi: 10.1038/s41583-020-00379-8
Keywords: neuronal continuum, cell identity, glia cells, neurons, axon compartments, glia-to-axon, cellular neighborhoods
Citation: Guzmán G, Paredes O, Romo-Vázquez R, Vélez-Pérez H and Morales JA (2025) Neuron identity switches in response to the gradient gene expression pathway. Front. Cell. Neurosci. 19:1536444. doi: 10.3389/fncel.2025.1536444
Received: 28 November 2024; Accepted: 27 January 2025;
Published: 19 February 2025.
Edited by:
Hyong Kyu Kim, Chungbuk National University, Republic of KoreaReviewed by:
Hiroyuki Arakawa, University of Michigan, United StatesJin-A Lee, Hannam University, Republic of Korea
Copyright © 2025 Guzmán, Paredes, Romo-Vázquez, Vélez-Pérez and Morales. This is an open-access article distributed under the terms of the Creative Commons Attribution License (CC BY). The use, distribution or reproduction in other forums is permitted, provided the original author(s) and the copyright owner(s) are credited and that the original publication in this journal is cited, in accordance with accepted academic practice. No use, distribution or reproduction is permitted which does not comply with these terms.
*Correspondence: J. Alejandro Morales, amFsZWphbmRyby5tb3JhbGVzQGFjYWRlbWljb3MudWRnLm14