- 1Brain Science Institute, Korea Institute of Science and Technology (KIST), Seoul, Republic of Korea
- 2Division of Bio-Medical Science and Technology, KIST School, Korea University of Science and Technology (UST), Seoul, Republic of Korea
- 3Department of Integrated Biomedical and Life Sciences, Korea University, Seoul, Republic of Korea
- 4Department of Life Science, Korea University, Seoul, Republic of Korea
The prevailing belief has been that the fundamental structures of cerebellar neuronal circuits, consisting of a few major neuron types, are simple and well understood. Given that the cerebellum has long been known to be crucial for motor behaviors, these simple yet organized circuit structures seemed beneficial for theoretical studies proposing neural mechanisms underlying cerebellar motor functions and learning. On the other hand, experimental studies using advanced techniques have revealed numerous structural properties that were not traditionally defined. These include subdivided neuronal types and their circuit structures, feedback pathways from output Purkinje cells, and the multidimensional organization of neuronal interactions. With the recent recognition of the cerebellar involvement in non-motor functions, it is possible that these newly identified structural properties, which are potentially capable of generating greater complexity than previously recognized, are associated with increased information capacity. This, in turn, could contribute to the wide range of cerebellar functions. However, it remains largely unknown how such structural properties contribute to cerebellar neural computations through the regulation of neuronal activity or synaptic transmissions. To promote further research into cerebellar circuit structures and their functional significance, we aim to summarize the newly identified structural properties of the cerebellar cortex and discuss future research directions concerning cerebellar circuit structures and their potential functions.
1 Introduction
Our understanding of the cerebellar circuit structures has gradually evolved, as numerous studies have clarified previously unknown synaptic connections, organization of circuit formation, or cell types. These structural properties appear to make cerebellar circuits more complex than previously understood, potentially serving as effective components for the expansion of neuronal signals transmitted through the cerebellum. In this article, we summarize these structural updates and discuss their possible contributions to neural computations. The structural updates introduced here are obtained from studies mainly in rodents unless otherwise stated. Although our focus is on structural properties, we also describe functionally identified properties related to the updated structures. Furthermore, based on our revised understanding, we consider the future direction of research related to new structural properties of cerebellar circuits.
Before discussing recent updates, it is important to summarize the fundamental structures of the cerebellum. At the gross morphology level, the cerebellar cortex comprises three tightly folded layers: the granular layer (GL), Purkinje cell layer (PCL), and molecular layer (ML) from the inside. The basic circuit structures within the cerebellum and the morphology of individual types of neurons have long been known (Eccles et al., 1967; Ito, 2006). There are two major afferent pathways into the cerebellum, climbing fibers (CFs) and mossy fibers (MFs). While CFs directly innervate Purkinje cells (PCs), MFs indirectly innervate PCs via granule cells (GCs), which have somas and short dendrites in the GL and their parallel fiber (PF) axons in the ML. PCs provide the sole output from the cerebellar cortex, projecting mainly to the cerebellar nuclei (CN) and vestibular nucleus. PC somas are aligned in the PCL, and their highly elaborate dendritic trees are located in the ML, arranged in a single sagittal plane. This morphology of PC dendrites is beneficial for receiving inputs from many PFs, which run parallel to the layer structures and intersect vertically with PC dendrites. Conversely, a single PC receives synaptic inputs from only one CF, although hundreds of synapses form between them. Molecular layer interneurons (MLIs) receive inputs from PFs and inhibit PCs. Another type of inhibitory interneurons, Golgi cells (GoCs), receive inputs from both PFs and MFs, and inhibit GCs. These typical cerebellar circuit structures are strikingly conserved throughout the cerebellum and are believed to be crucial for cerebellar neural computations.
2 Update of the structural organization of GCs
Cerebellar GCs are the most abundant neurons in the brain, consisting of more than 50% of all brain neurons (Herculano-Houzel et al., 2015). GCs have a unique morphology, characterized by a very small soma with only 3–4 short dendrites, and an ascending axon extending into the ML, where bifurcated PFs are formed. Each of the 3–4 dendrites of a GC makes a synapse with a single MF terminal in the glomerulus. Although these numerous small GCs have simply been considered homogeneous, their heterogeneous properties have also been discovered in terms of molecular expression (Lein et al., 2007; Kozareva et al., 2021), morphology (Houston et al., 2017), and physiological properties (D'Angelo et al., 1998; Gandolfi et al., 2014; Chabrol et al., 2015; Dorgans et al., 2019; Masoli et al., 2020; Straub et al., 2020; Rhee et al., 2021; Shuster et al., 2021). A well-known anatomical heterogeneity is the position of individual PFs within the ML, resulting in the formation of characteristic laminar structures by all PFs (Figure 1A). PF heterogeneity is evident not only in their position but also in their varying diameters, i.e., they are thinner in the outer ML and thicker in the inner ML (Sultan, 2000; Wyatt et al., 2005; Straub et al., 2020). Consistent with the general correlation between axon diameter and action potential propagation velocity (Schmidt and Knösche, 2019), the velocity is higher in the inner layer (Figure 1B; Straub et al., 2020). Differences also exist in the firing properties of GCs within the inner and outer GL (Figure 1C; Straub et al., 2020), suggesting that these distinct firing properties might be transmitted to spatially correlated PFs in the ML. However, several studies have demonstrated no spatial correlation between GC somas in the GL and PFs in the ML (Zong et al., 2005; Wilms and Häusser, 2015; Markwalter et al., 2019; Rhee et al., 2021; Kim et al., 2023b), indicating that the properties of GCs detected in the GL cannot be directly related to the locations of PFs in the ML. Advanced techniques linking specific GCs to their PF locations are required to characterize GCs in the GL according to PF locations. One study used adeno-associated virus (AAV)-mediated labeling of a group of GCs with a bundle of PFs in the ML and demonstrated MF stimulation-mediated, nonuniform calcium responses in GCs specific to their PF locations (Rhee et al., 2021). Additionally, GCs with PFs in different ML sublayers exhibit moderately, yet significantly, different connectivity with MFs of varying origins (Figure 1D; Shuster et al., 2021; Kim et al., 2023b). Thus, PF location-dependent variability in GC properties, namely, action potential propagation velocity in PFs, functional properties in GCs, and connectivity with MFs, has gradually been revealed.
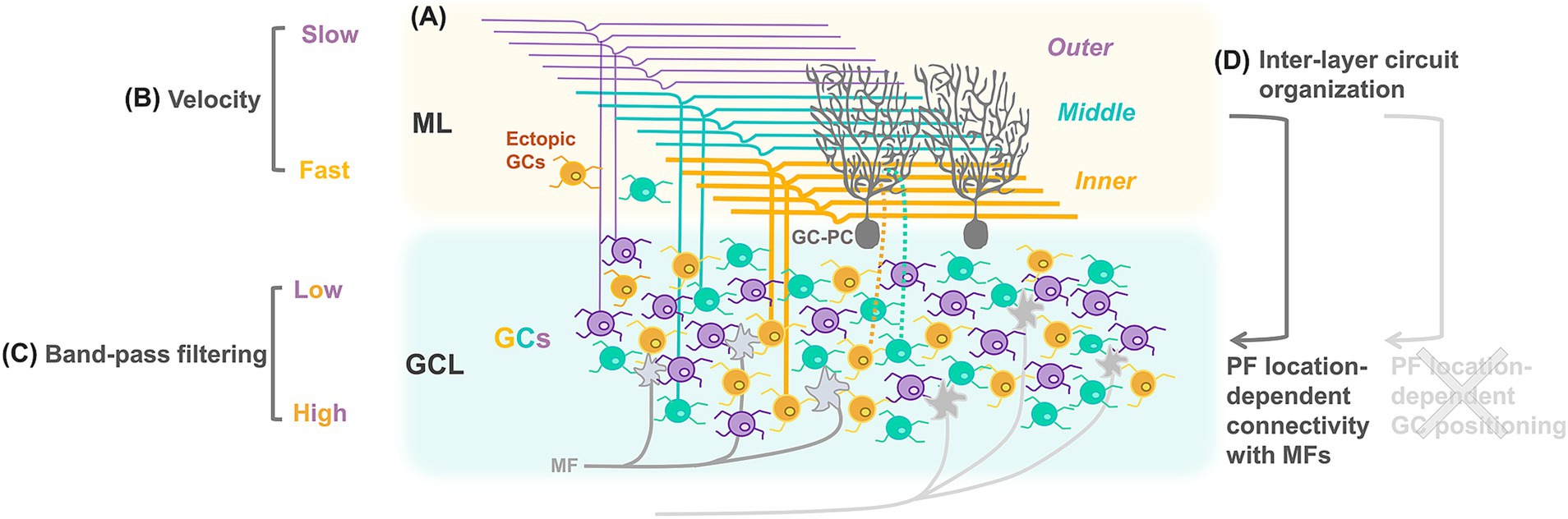
Figure 1. Schematic diagram showing heterogeneous properties of cerebellar GCs. PF diameters (A) and the velocity of action potential propagation (B) are varied according to the location of PFs in the ML. Firing properties of GCs are varied according to their own locations in the GL (C). Whereas distributions of GCs and PFs appear not to be spatially correlated, connectivity of GCs with MFs in the GL is organized according to the location of their PFs in the ML (D).
It would be interesting to clarify the role of these GC properties according to PF locations. In general, a conceptual role of GCs is considered to be separation of contextual information (Cayco-Gajic and Silver, 2019). Characteristic GC circuit properties, such as large expansion and sparse synaptic connectivity from MFs to GCs, and broad feedback inhibition mediated by GoCs, are advantageous for pattern separation (Billings et al., 2014; Cayco-Gajic et al., 2017; Litwin-Kumar et al., 2017). One traditional theory for pattern separation is sparse coding, which posits that only sparse populations of GCs are activated to convey specific types of information. This theory can explain the associative learning that occurs at synapses with PC dendrites (Marr, 1969; James, 1971). However, in vivo calcium imaging studies suggested that activities in denser-than-expected GC populations represent specific type of contextual information (Giovannucci et al., 2017; Knogler et al., 2017; Wagner et al., 2017). In contrast, isolated sensory stimuli were recently reported to trigger relatively sparse GC population responses via local synaptic inhibition (Fleming et al., 2024). Computational studies suggested that the sparse synaptic connections between MFs and GCs may contribute to the pattern separation of contextual information, regardless of whether GC population activity is sparse or dense (Cayco-Gajic et al., 2017). It is not entirely clear whether GCs enhance pattern separation via sparse coding or other computational mechanisms. In any case, an increased representation of neuronal signals appears beneficial for pattern separation, which may be achieved through the combination of PF location-dependent GC properties described in the previous paragraph.
In addition to the PF location-dependent organization of GCs, other GC-related structural updates have been reported. The analysis of large-scale electron microscopy data revealed structured connectivity not only from MFs to GCs but also from GCs to PCs (Nguyen et al., 2023). Compared with randomized connections, pairs of GCs tended to share MF inputs, and pairs of PCs exhibited similar PF input patterns. A computational model predicted that such structured connectivity increased resilience to noise without significantly affecting pattern separation capacity. In another study, ectopic GCs located in the ML, long thought to have simply ceased migration during development, were found to be more abundant in the posterior cerebellum than previously expected—comprising approximately 40% of the population relative to MLIs—and exhibited firing properties similar to those of GCs located in the GL (Dey et al., 2022). This raises the possibility that ectopic GCs may have a unique functional role.
3 Update of the circuit surrounding MLIs
MLIs, a major type of inhibitory interneuron in the cerebellum, provide feedforward inhibition to PCs by receiving excitatory synaptic inputs from PFs. They also receive inputs from neighboring MLIs through chemical and/or electrical synapses (Häusser and Clark, 1997; Mittmann et al., 2005; Rieubland et al., 2014). MLIs are traditionally classified into two cell types: basket cells (BCs) and stellate cells (SCs), based on several anatomical characteristics (Eccles et al., 1967; Jörntell et al., 2010). BCs, with relatively straight dendrites, are mainly located in the inner one-third of the ML, while SCs, with dendrites and axons within a more limited area, are mainly located in the outer two-thirds of the ML (Schilling, 2013; Sotelo, 2015; Sergaki et al., 2017; Kim and Augustine, 2021). Notably, BCs innervate the area around the PC somas, and form pericellular baskets on the PC somas and dense plexuses called pinceau on the PC axon initial segment (AIS), providing strong inhibitory control of PC activity (King et al., 1993; Sultan and Bower, 1998; Bower, 2010; Sotelo, 2015). In contrast, SCs send inhibitory inputs to PC dendrites (Sotelo, 2015; Sergaki et al., 2017). Beyond these basic anatomical properties, higher-order circuit mechanisms also contribute to MLI functions. As the characteristics of MLIs have been comprehensively summarized in other review articles (e.g., Jörntell et al., 2010; Sotelo, 2015; Kim and Augustine, 2021), we focus on new information associated with MLI circuits and discuss their possible organization.
3.1 Cell types of MLIs
Even though BCs and SCs are the two stereotyped MLIs, the classification of MLIs is still under debate. There is a contrasting idea to the traditional classification of BCs and SCs; all MLIs originate from a single type of interneurons with continuous variation in morphology and different target locations on PCs (Rakic, 1972; Sultan and Bower, 1998; Bower, 2010). A transcriptome study identified two distinct types of MLIs, MLI1s and MLI2s, characterized by different molecular expressions, such as Sorcs3 in MLI1s and Nxph1 in MLI2s (Figure 2A; Kozareva et al., 2021). Because both MLI1s and MLI2s showed similar continuum in morphological properties, particularly an SC-like morphology in the outer one-third of the ML, they do not align with the traditional BC and SC classification. However, further analyses revealed at least two distinct morphological aspects between MLI1s and MLI2s (Lackey et al., 2024). First, MLI1 somas are spiny, while MLI2 somas are smooth. Second, MLI1s in the inner two-third of the ML contribute to pinceau formations, whereas all MLI2s, regardless of soma location, retain classical SC morphologies without contributing to pinceau. Supporting this second observation, another study identified two discrete morphological types of MLIs, canonical BCs and SCs with extensive heterogeneity, which are distinguished by the morphological features of axons rather than their soma positions in the ML (Wang and Lefebvre, 2022). This study confirmed the dissociations between morphological and transcriptomic types, but showed some relationships between them. Sorcs3-positive MLIs, namely MLI1s, are mostly BCs and SCs with long axons, further distinguished by the combined expression levels of Grm8 and Cacna1e. Nxph1-positive MLIs, namely MLI2s, are largely SCs with short axons and partly SCs with long axons located in the superficial layer.
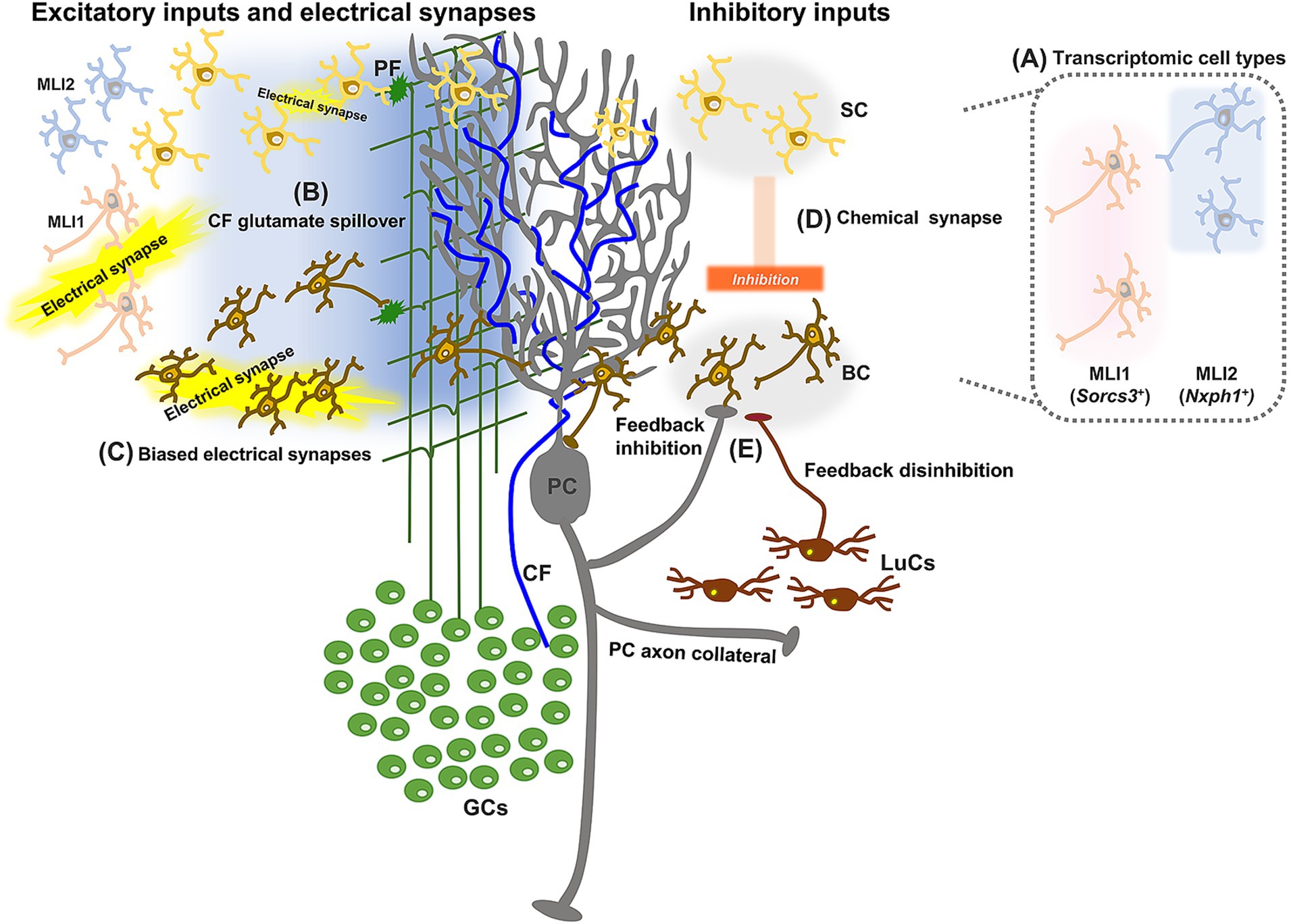
Figure 2. Schematic diagram showing update of the neuronal circuits surrounding MLIs. In addition to well-established circuit structures, studies revealed two distinct types of MLIs based on molecular expression (A), spillover-mediated CF-MLI connections (B), organized electrical coupling between MLIs (C), MLI-MLI synaptic connections biased from the outer to the inner ML (D), and inhibitory inputs from PCs to MLIs (E).
In general, neuronal cell-type classification considers not only transcriptional and morphological properties, but also electrophysiological and connectional properties (Zeng and Sanes, 2017). MLI2s were found to be more excitable than MLI1s, and the expression of Gjd2, the gene encoding the dominant gap junction protein, connexin 36, was detected in MLI1s, but not in MLI2s (Kozareva et al., 2021), indicating electrical differences in the two transcriptionally identified cell types. In terms of connectional properties, a subpopulation of MLIs located in the inner one-third of the ML was found to receive inhibitory inputs from PCs (Witter et al., 2016; Halverson et al., 2022), which may contribute to the disinhibition of other PCs. Further studies are required to clarify whether these MLIs have other characteristic properties and can be categorized into a specific cell type based on the functional uniqueness.
The anatomical organization of MLIs and their circuits, which affect cell type classification, appears to rely on process occurring during the postnatal developmental period. MLIs are gradually generated from precursors in the white matter (WM), which originate in the ventricular zone (Yamanaka et al., 2004). Immature postmitotic MLIs radially migrate toward the ML, where they find their final positions through a complex migratory route involving radial and tangential migration (Simat et al., 2007a; Cameron et al., 2009; Park et al., 2021). This complex migration is regulated by several pathways, such as excitatory and inhibitory synaptic transmission (Wefers et al., 2017). Specifically, the blockade of synaptic transmission from PF bundles resulted in abnormal distributions of MLIs (Park et al., 2019), indicating that PF inputs likely regulate the migration that determines the final positions of MLIs. The final position of MLIs also depends on their timing of birth; early-and late-born MLIs are positioned in the inner and outer parts of the ML, respectively, meaning that BCs are generally born earlier than SCs (Yamanaka et al., 2004; Weisheit et al., 2006; Leto et al., 2008; Schilling et al., 2008; Sudarov et al., 2011; Consalez and Hawkes, 2012; Sotelo, 2015), although their positions are not strictly defined. Despite differences in morphology, transcriptional and electrophysiological properties, or connectivity in mature MLIs, MLI precursors before leaving the WM are indistinguishable in terms of their genetic markers (Maricich and Herrup, 1999; Leto et al., 2006; Carter et al., 2018). This suggests that each MLI acquires its characteristic phenotypes after migration begins. It has been proposed that an additional tangential migration, supported by premigratory GCs in the EGL, is required for the synaptic differentiation of SCs (Cadilhac et al., 2021). Additionally, a study linking morphological and transcriptomic types demonstrated that BC and SC morphological identities diverged during early phases of migration, prior to the expression of transcriptomic markers (Wang and Lefebvre, 2022). Thus, the diverse characteristics of MLIs may arise from the impact of their migration process itself or the environmental situations encountered during migration.
3.2 Excitatory inputs to MLIs
As described above, PFs are well-known excitatory inputs to MLIs. With the revelation of the heterogeneous properties of GCs and PFs, different patterns of short-term synaptic plasticity (STP) were observed in PF synapses of the two types of MLIs, namely, BCs and SCs. The PF-SC synapses demonstrated persistent facilitation, whereas PF-BC synapses showed depression following initial paired-pulse facilitation (Bao et al., 2010). This implies that PF inputs trigger firing in BCs first, followed by delayed firing in SCs. Given their target locations on PCs, PF inputs may sequentially hyperpolarize PCs from soma to dendrites (Bao et al., 2010; Blackman et al., 2013). A recent study further categorized STP in unitary PF-MLI synapses into four types, attributing this diversity to the heterogeneous expression of the key STP molecule, synapsin II, in GCs, but found no clear MLI type-dependent STP patterns (Dorgans et al., 2019). Although it is unclear how to reconcile these conflicting results in terms of cell types, the diversity of PF-MLI synaptic functions may contribute to increased coding patterns, potentially allowing efficient separation of different contexts in the cerebellum.
In contrast to the clear excitatory synaptic connections from PFs to MLIs, excitatory inputs from CFs to MLIs have long remained controversial. Although anatomical studies of labeled CFs suggested possible synaptic connections between CFs and MLIs (Palay and Chan-Palay, 1974; Sugihara et al., 1999), no conventional synaptic architecture was found between them in electron microscopy studies (Hámori and Szentágothai, 1980; Kollo et al., 2006). Nevertheless, functional effects of CFs were detected in MLIs (Eccles et al., 1966; Jörntell and Ekerot, 2003). It became clear that CF-MLI connections were mediated uniquely, through glutamate spillover (Figure 2B; Szapiro and Barbour, 2007; Mathews et al., 2012; Coddington et al., 2013). CF synapses to PCs have a very high release probability and typically show multivesicular release (Wadiche and Jahr, 2001; Foster et al., 2002; Harrison and Jahr, 2003), so that CF activation may reasonably lead to glutamate spillover. The involvement of N-methyl-D aspartate (NMDA) receptors in the spillover-mediated CF-MLI connections is also plausible, considering that MLIs have NMDA receptors on their dendrites (Carter and Regehr, 2000; Clark and Cull-Candy, 2002). Thus, it is now generally accepted that MLIs receive CF inputs via glutamate spillover. This spillover-mediated, CF-dependent regulation of MLIs has been shown to be involved in sensory processing in vivo (Arlt and Häusser, 2020).
3.3 Electrical synapses between MLIs
As commonly seen in inhibitory interneurons of the mammalian brain (Galarreta and Hestrin, 2001; Pereda, 2014; Coulon and Landisman, 2017), MLIs are also connected with each other via electrical synapses (Mann-Metzer and Yarom, 1999). The electrical synapses between MLIs rely on the gap junction protein connexin 36, which is differentially expressed according to their locations in the ML (Alcami and Marty, 2013). MLIs in the inner ML, presumably BCs, have higher levels of connexin 36 than those in the outer ML. Consistently, electrophysiological recordings have demonstrated that electrical connections are more frequent and stronger in BCs than in SCs (Figure 2C; Alcami and Marty, 2013). The difference in electrical connections is clear between MLI1s and MLI2s, as the expression of Gjd2 and electrical coupling were found only in MLI1s, but not ML2s (Kozareva et al., 2021; Lackey et al., 2024). Given that BCs are likely part of MLI1s (Wang and Lefebvre, 2022; Lackey et al., 2024), these results regarding cell type-dependent electrical connections appear consistent. Furthermore, studies using multiple patch-clamp recordings and optogenetic mapping identified that electrical coupling occurs in the sagittal plane (Kim et al., 2014; Rieubland et al., 2014). Such structured organization of electrical connections between MLIs contributes to the regulation of feedforward inhibition of PCs, by generating spatiotemporally controlled synchronization of MLI activity (Hoehne et al., 2020).
3.4 Inhibitory inputs to MLIs
MLIs are well known to receive GABAergic inhibitory synaptic inputs from neighboring MLIs (Llano and Gerschenfeld, 1993; Häusser and Clark, 1997; Mittmann et al., 2005), and this inhibitory connection is presumably important for fine-tuning the temporal precision of spiking in PCs (Mittmann et al., 2005). Similar to the electrical connections between MLIs, their inhibitory synaptic connections were also found to be oriented in the sagittal plane, although not as strongly biased as electrical coupling (Rieubland et al., 2014). Additionally, transitive patterns of MLI-MLI synaptic connections were demonstrated to be organized from the outer to the inner part of the ML (Figure 2D; Arlt and Häusser, 2020). This raises the possibility that such MLI microcircuit organization, together with the location of activated PFs, may be utilized for the regulation of cerebellar information processing – e.g., additional activation of PFs in the outer ML may lead to greater excitation of PCs through the reduction of MLI-dependent inhibition of PCs. Cell type-dependent innervation patterns have recently been demonstrated: MLI1s mainly innervate PCs to inhibit them, while MLI2s mainly innervate MLI1s to disinhibit PCs (Lackey et al., 2024). In other words, many MLI1s would receive relatively strong inhibitory inputs from MLI2s, whereas MLI2s may have little or no inhibitory inputs from other MLIs.
In addition to the inhibitory synaptic inputs from MLIs, early anatomical studies have suggested other possible inputs from PCs and Lugaro cells (LuCs), the latter of which are a type of inhibitory interneuron in the GL (Hámori and Szentágothai, 1968; Larramendi and Lemkey-Johnston, 1970; O’Donoghue et al., 1989; Lainé and Axelrad, 1996, 1998). While functional synaptic connections from LuCs to MLIs are not yet clarified, functional inhibitory inputs from PCs to MLIs were identified by recording synaptic currents evoked by the optogenetic stimulation of PCs (Figure 2E; Witter et al., 2016; Halverson et al., 2022). Optogenetically evoked synaptic currents were detected in approximately 16 to 30% of MLIs, indicating these are not extremely rare synaptic connections. Reciprocally connected pairs of PCs and MLIs have not been found so far, thus MLIs receiving inputs from PCs are considered to send inhibitory inputs to other PCs, suggesting that this pathway from PCs to MLIs contributes to synchronization of PCs through disinhibition of MLIs (Halverson et al., 2022). Given that inhibitory LuCs also receive inputs from PCs (Witter et al., 2016), PC population activity may rely on the organization of connections among PCs, LuCs, and MLIs, if LuCs functionally inhibit MLIs. Direct connections from PCs to MLIs are considered to mediate synchronization of neighboring PCs (Witter et al., 2016; Halverson et al., 2022), while indirect connections from PCs to MLIs through LuCs may contribute to desynchronization of other PC populations, as seen between aldolase C/zebrin II-positive and-negative PC populations (Tsutsumi et al., 2015). In addition, whereas glutamate spillover from CFs primarily exerts excitatory influence to MLIs, as described above, other MLIs located outside the glutamate diffusion limit have been shown to be inhibited upon CF activation via disynaptic inhibition (Coddington et al., 2013). Thus, CF-mediated functional segregation of MLIs may also contribute to the distinctive patterns of activity of different PC populations. Alternatively, segregation of MLIs could be along the perpendicular direction to the layer, as CF inputs were reported to activate many MLIs but inhibit MLIs in the deep ML that have strong inhibitory impacts onto PCs (Arlt and Häusser, 2020).
4 Newly identified circuit properties in PCs
PCs are a unique type of neuron specific to the cerebellar cortex and have been extensively studied from various perspectives, including development, dendritic arborization, synapse formation, electrophysiological properties, synaptic regulation, molecular expression, and neuronal circuits (Yuzaki, 2004; Sotelo and Dusart, 2009; De Zeeuw et al., 2011; Cerminara et al., 2015; Leto et al., 2016; Nedelescu et al., 2018; De Zeeuw, 2021). PCs provide the sole output of the cerebellar cortex and form GABAergic inhibitory synapses with neurons in target regions outside the cerebellar cortex, mainly in the CN. Despite the wealth of knowledge about PCs, properties of PC axon collaterals and ephaptic coupling around PCs had not been adequately analyzed, leaving their importance in cerebellar computations and functions unclear. However, in the past decade, several studies have provided more detailed characterizations of these aspects. Additionally, the morphological diversity and dopaminergic signaling of PCs have also been demonstrated.
4.1 PC axon collaterals
While PC axons project outside the cerebellar cortex, PC axon collaterals enable the modulation of information processing within the cerebellar cortex by sending feedback signals. Early studies already described PC axon collaterals as forming synapses onto other PCs and neurons of the ML (Eccles et al., 1967; Larramendi and Lemkey-Johnston, 1970; Chan-Palay and Palay, 1971). Although they were anatomically observed in both young and adult rodents (Larramendi and Lemkey-Johnston, 1970; Chan-Palay and Palay, 1971; Bishop, 1982; Hawkes and Leclerc, 1989; Bernard et al., 1993), their functional synaptic transmission was first confirmed only in young animals (Orduz and Llano, 2007; Watt et al., 2009; Hirono et al., 2012). These studies demonstrated that PC axon collaterals form functional synapses with other PCs and globular cells, small inhibitory interneurons located in the GL. A computational model and experimental confirmation demonstrated that PC axon collaterals generated waves of PC activity traveling along chains of connected PCs in the developing cerebellum, whereas these waves were not observed in the mature cerebellum (Watt et al., 2009). This led to an idea that PC axon collaterals play a crucial role only in cerebellar circuit formation during postnatal development. In contrast, studies using optogenetic stimulation with patch-clamp recording identified functional synaptic transmissions from PC axon collaterals in adult animals (Figure 3A; Guo et al., 2016; Witter et al., 2016), although these synaptic connections were restricted to the parasagittal plane and were present only near the PCs sending the axon collaterals. GABAergic inhibitory synaptic transmissions were recorded from PCs, MLIs, LuCs, and candelabrum cells (neurons situated in the PCL), but not from GoCs, upon optogenetic stimulation of PCs or spontaneous PC firing (Witter et al., 2016; Halverson et al., 2022; Osorno et al., 2022). While there were variabilities depending on the lobule, PC axon collaterals also projected to the GL, and inhibited GCs and a subset of unipolar brush cells (UBCs) (Guo et al., 2016; Guo et al., 2021). These studies suggest that PC axon collaterals may have additional roles in the mature cerebellum. It would be interesting to differentiate properties of collateral axons in the cerebellar cortex from axons projecting to the CN, as this may lead to techniques to selectively manipulate collateral axons.
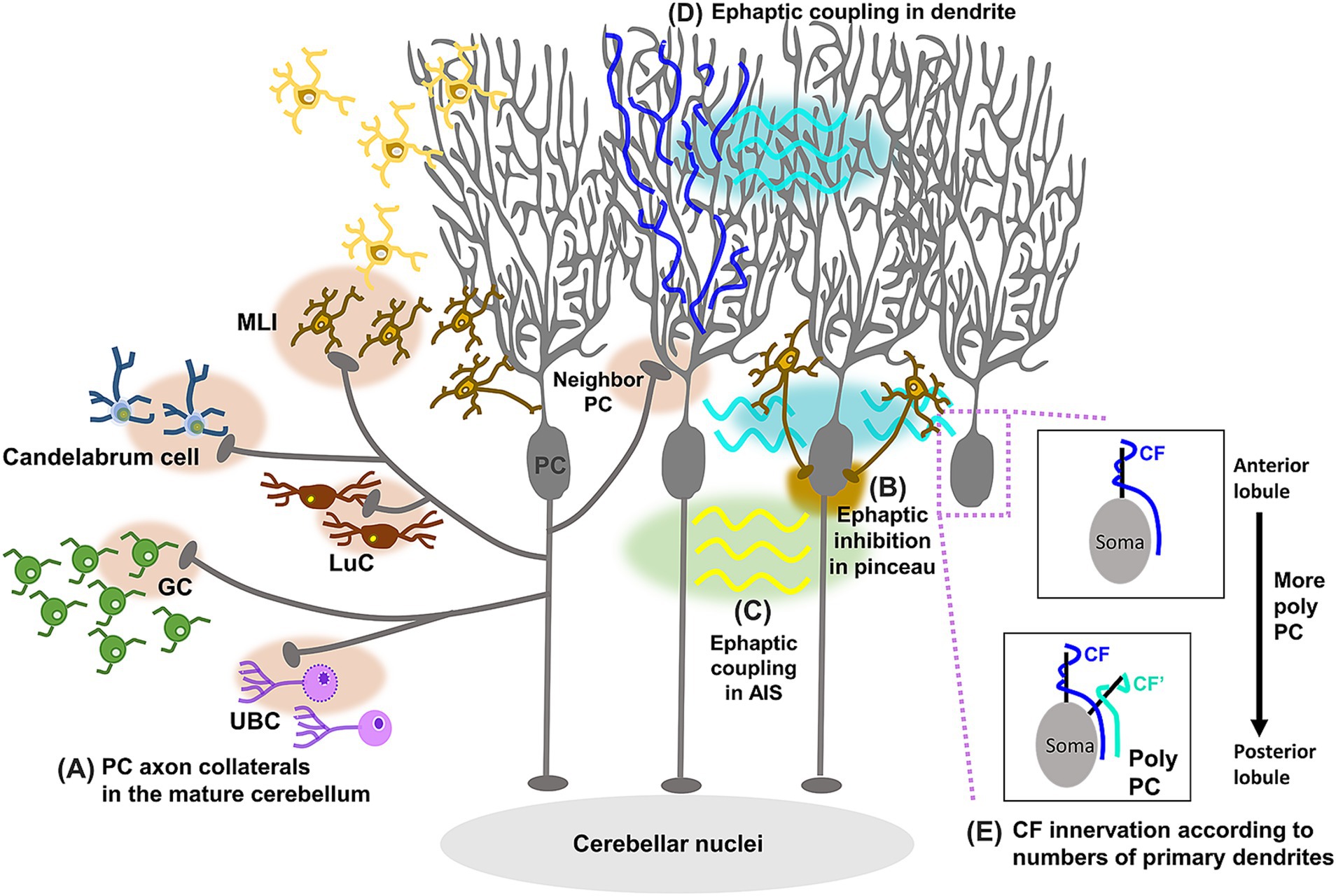
Figure 3. Schematic diagram showing update of PC circuit structures. PC axon collaterals form functional synaptic connections (A) to other PCs, MLIs, LuCs, candelabrum cells, GCs, and UBCs in the mature cerebellum. In addition, studies have demonstrated ephaptic coupling between the pinceau in BCs and the AIS in PCs, triggering rapid inhibition of PCs (B), between PCs in the AIS, leading to the synchronization of spontaneous PC firing (C), and between PC dendrites, causing the inhibition of many neighboring PCs upon excitation by CF inputs (D). PCs with two primary dendrites have been found more frequently in posterior lobules, receiving two discrete CF inputs on each of the two primary dendrites (E).
4.2 Ephaptic coupling around PCs
Signal processing and transmission in the nervous system involve the flow of current across neuronal cell membranes, which alters the electrical potential in extracellular regions. Through these alterations in extracellular potential, neurons can interact with adjacent neurons without forming the specific cell-to-cell contacts required for chemical and electrical synaptic transmission. These electrical interactions, described as early as the 1940s (Katz and Schmitt, 1940; Arvanitaki, 1942), are now called ephaptic transmission or coupling (Anastassiou and Koch, 2015; Faber and Pereda, 2018). In the well-established circuit structures where PFs provide direct excitatory inputs and disynaptic inhibitory inputs through BCs or SCs onto PCs, recordings from PCs during PF volleys were expected to show a sequence of excitation followed by inhibition. However, an initial study revealed that a brief inhibition occurred before this expected sequence (Korn and Axelrad, 1980). Due to its short latency, this brief inhibition was proposed to be electrically generated by nearby BCs. BCs form pinceau that wrap around and terminate on the AIS of PCs (Sotelo, 2015). While BC axons make GABAergic synaptic contacts onto PC somas, the pinceau themselves are largely devoid of chemical and electrical synapses (Ichikawa et al., 2011), raising a question about their functions. The dense plexuses of pinceau appear to be well-suited for generating local electric fields, and indeed, one study demonstrated that the pinceau could induce rapid ephaptic inhibition of PC axons (Figure 3B; Blot and Barbour, 2014). This study proposed that pinceau-dependent ephaptic inhibition allows GCs to instantaneously inhibit PCs. Given the variability in pinceau size (Zhou et al., 2020), this inhibitory regulation may differ depending on PC zones identified by specific molecular markers, such as aldolase C/zebrin II (Apps and Hawkes, 2009; Cerminara et al., 2015).
In addition to the ephaptic coupling between the pinceau in BCs and the AIS in PCs, two other types of ephaptic coupling between PCs have been identified (Han et al., 2018; Han et al., 2020). One type of ephaptic coupling observed in the AIS of PCs led to the synchronization of spontaneous PC firing (Figure 3C; Han et al., 2018). Another type of coupling, occurring between PC dendrites, resulted in the inhibition of multiple neighboring PCs when synaptically connected PCs were activated by CF inputs (Figure 3D; Han et al., 2020). This study proposed that this type of ephaptic coupling allows CF inputs to efficiently activate CN neurons through the simultaneous inhibition of many PCs. Although these two types of ephaptic coupling between PCs may seem contradictory—one causing excitation and the other inhibition of neighboring PCs—they likely contribute to the spatially distinct responses of neighboring PCs upon CF inputs.
4.3 PC morphological diversity
The diversity of PCs has been well characterized by different expression levels of marker molecules, such as aldolase C/zebrin II (Cerminara et al., 2015). The expression patterns of these molecules form longitudinally striped compartments known as microzones. PCs in different microzones (e.g., AldoC+ and AldoC-microzones) exhibit distinct firing patterns and project to specific sets of CN or vestibular nuclei, linking each microzone to distinct functions (De Zeeuw, 2021). For instance, fast and slow eye movements are mediated by distinct PC subpopulations in the flocculus, known as 9- and 9+ PCs, respectively (Blot et al., 2023). Apart from these modules visualized by molecular markers, the diversity of PC morphology has been gradually recognized. PCs typically have a characteristic morphology with a large, planar, highly branched dendritic tree, and the diversity is observed in the dendritic shapes (Nedelescu and Abdelhack, 2013; Nedelescu et al., 2018; Chang et al., 2020; Busch and Hansel, 2023; Magnus et al., 2023). In zebrafish, four types of PCs with different morphological and firing properties were classified, and they were active at different phases during swimming, suggesting that different types of PCs have different functions (Chang et al., 2020). In mice, morphological types were specifically considered based on the number of primary dendrites (Nedelescu and Abdelhack, 2013; Nedelescu et al., 2018; Busch and Hansel, 2023). Most PCs in the anterior and central lobules have single primary dendrites, but some PCs, particularly those in the posterior lobules, have two primary dendrites. Furthermore, a recent study (Busch and Hansel, 2023) demonstrated that PCs with two or multiple primary dendrites, called as poly PCs, are a near-universal morphological feature in humans and are functionally different from normative PCs in terms of the number of CF innervations. While PCs usually receive inputs from a single CF, each of the two primary dendrites of poly PCs receives discrete CF inputs (Figure 3E), suggesting that poly PCs could integrate different information from independent CFs. Since poly PCs are more prevalent in the posterior lobules, this information integration would be more beneficial for functions involving the posterior lobules, such as fear-evoked freezing behavior (Koutsikou et al., 2014).
Updates have also been made to our understanding of PC axonal morphology. Axonal swellings, known as torpedoes, were historically associated with pathological conditions (Louis et al., 2009; Babij et al., 2013; Louis et al., 2014; Redondo et al., 2015). However, axonal swellings have also been observed in healthy young animals and are thought to serve a different function from those associated with disease (Ljungberg et al., 2016). In fact, axonal swellings in healthy animals have been shown to enhance action potential fidelity and are linked to cerebellum-dependent motor leaning (Lang-Ouellette et al., 2021). Thus, these swellings appear to represent a form of axonal structural plasticity.
4.4 Dopaminergic signaling related to PCs
In addition to influences from traditional synaptic inputs, axon collaterals of other PCs, and ephaptic coupling, PCs have been reported to be affected by dopamine through D2 receptors (Cutando et al., 2022). D2 receptor-mediated dopaminergic signaling appears to play a role in regulating social behaviors. Dopamine release may originate from dopaminergic projection neurons outside the cerebellum, as early studies showed sparse dopaminergic innervation in the cerebellum (Panagopoulos et al., 1991; Ikai et al., 1992; Nelson et al., 1997). Another potential source could be a subset of PCs that express tyrosine hydroxylase, the rate-limiting enzyme in the classical dopamine synthesis pathway (Takada et al., 1993; Abbott et al., 1996). However, a recent study demonstrated that dopamine is synthesized in PCs via an alternative pathway involving a member of the cytochrome P450 superfamily, CYP2Ds (Li et al., 2023). This study also revealed a role for D1 receptors in Bergmann glial cells, contributing to motor and social behaviors. Understanding how dopamine released from PCs acts in a coordinated manner on D2 receptors in PCs and D1 receptors in Bergmann glial cells would be an intriguing avenue for future research.
5 Update of the GoC circuits
Even though GoCs are the most abundant type of inhibitory interneuron in the GL, they are sparse, existing at a ratio of one to several hundred or thousand GCs (D'Angelo and Casali, 2012). These few GoCs are heterogeneous, in terms of their molecular markers, morphology, and electrophysiological properties (Dieudonné, 1998; Geurts et al., 2001; Simat et al., 2007b; Barmack and Yakhnitsa, 2008; D'Angelo and Casali, 2012). For instance, transgenic mice expressing GFP under the glycine transporter 2 (GlyT2) promoter are used to identify GoCs, yet GFP-positive neurons in these mice include approximately 85% of GoCs and other types of neurons, but exclude 15% of GoCs that are purely GABAergic (Simat et al., 2007b). However, all GoCs have two classes of dendrites, basal and apical, and a widely ramified axon (Barmack and Yakhnitsa, 2008). Basic circuit structures also appear to be conserved among the GoCs, which provide feedforward and feedback inhibition onto GCs (Figure 4A-a) through excitatory synaptic connections from MFs to basal dendrites in the GL (Figure 4A-b) and from PFs to apical dendrites in the ML (Figure 4A-c), respectively. The regulation mediated by GoCs appears to be critical for fine-tuning GC activity (Crowley et al., 2009; Fleming et al., 2024).
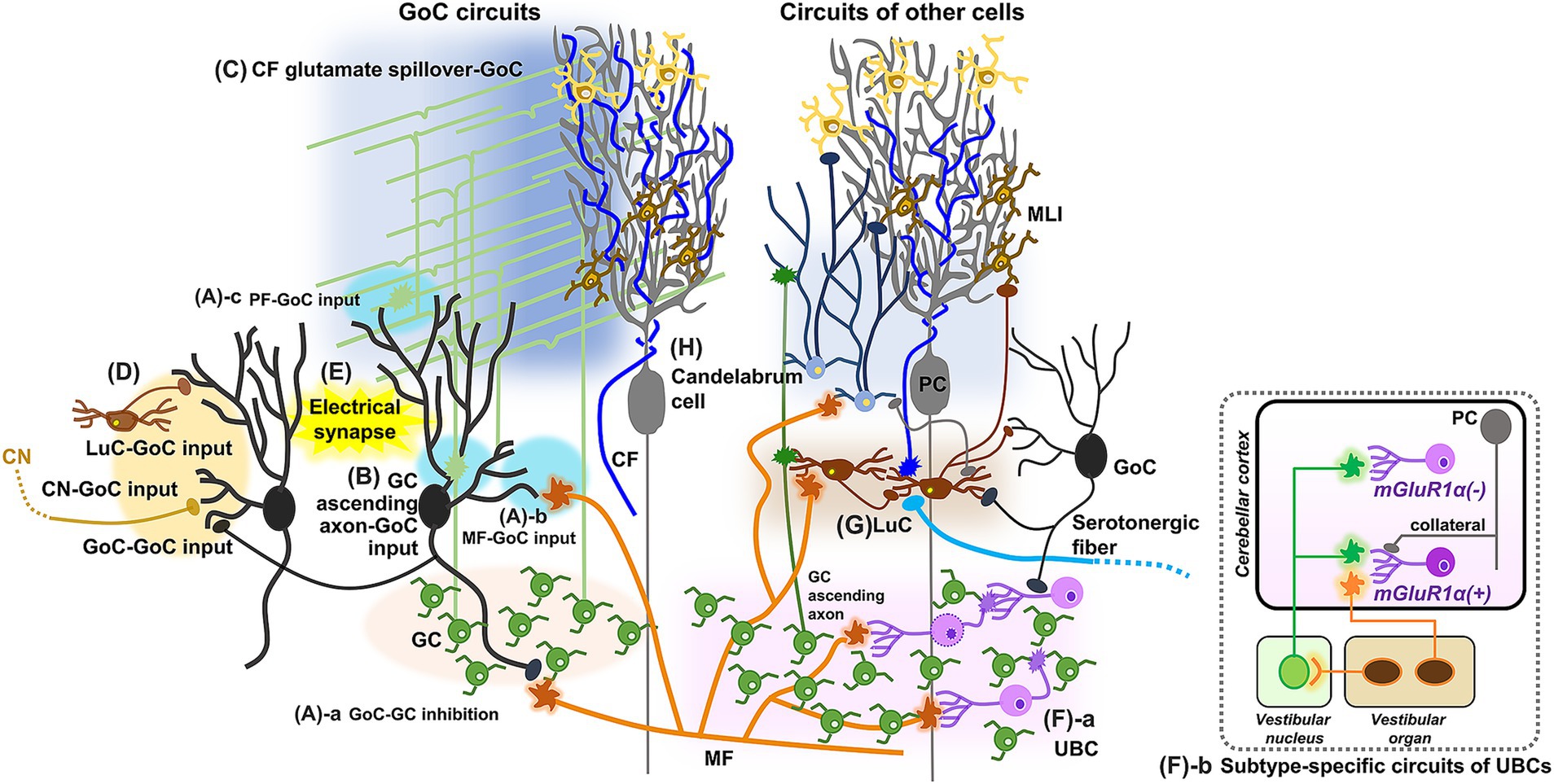
Figure 4. Schematic diagram showing update of GoCs and other cell types. In addition to well-established circuit structures (A), GoCs receive inputs from GC ascending axons (B) and spillover-mediated excitatory inputs from the CFs (C), may receive inhibitory inputs from LuCs, other GoCs and CN neurons (D), and have electrical coupling between GoCs (E). UBCs receive excitatory input from a single MF and inhibitory inputs from GoCs, and make synapses onto GCs or other UBCs (F-a). Cell-type specific circuit structures of UBCs are also revealed (F-b). LuCs, projecting to MLIs and probably to GoCs, have diverse inputs from MFs, CFs, GCs, other LuCs, GoCs, PCs, and serotonergic fibers (G). Candelabrum cells receive excitatory inputs from MFs and GCs, and innervate MLIs (H).
In addition to these basic circuit structures, several studies have demonstrated additional inputs onto GoCs. While excitatory inputs from GCs are predominantly made through PFs in the ML, it has also been shown that ascending axons of GCs form functional synaptic contacts onto the proximal dendrites of GoCs in the GL (Figure 4B; Cesana et al., 2013). Although early studies suggested synaptic contacts from the CFs to GoCs (Scheibel and Scheibel, 1954; Marr, 1969), subsequent research did not find such contacts (Galliano et al., 2013). Another study demonstrated that CFs have excitatory effects on GoCs through spillover-mediated transmission, similar to the case with MLIs (Figure 4C; Nietz et al., 2017). In contrast to the clear excitatory synaptic inputs from MFs and PFs onto GoCs, the source of inhibitory synaptic inputs remained unidentified for a long time and is still not completely clear (Figure 4D). It was suggested that LuCs provide inhibitory inputs via the release of glycine and GABA in the presence of serotonin, and MLIs also provide GABAergic inhibitory inputs (Dumoulin et al., 2001). However, studies using paired patch-clamp recordings or anatomical analyses demonstrated that GoCs do not receive inhibitory inputs from MLIs (Hull and Regehr, 2012; Eyre and Nusser, 2016). Instead, functional synaptic responses between neighboring GoCs were detected in 20% of all connections tested (Hull and Regehr, 2012). Considering the anatomical observation that a small fraction of GABAergic synaptic inputs to GoC dendrites contain GlyT2, a marker of glycinergic terminals (Eyre and Nusser, 2016), it is suggested that LuCs, which release both GABA and glycine, may actually innervate GoCs. Alternatively, some CN neurons, which also release a mixture of GABA and glycine, have been shown to innervate GoCs (Ankri et al., 2015).
GoCs can be subdivided based on their heterogeneous properties, which may have biological implications. Studies have attempted to classify GoC subtypes based on biochemical and neurochemical properties (Geurts et al., 2001; Simat et al., 2007b), such as the expression of Rat-303, metabotropic glutamate receptor (mGluR) 2, somatostatin, and neurogranin in glycinergic and/or GABAergic neurons. Although the direct association between the biochemical subtypes of GoCs and their electrophysiological properties has not yet been clarified, synaptic connections that are selective or preferable for specific GoC subtypes have been reported (Ankri et al., 2015; Eyre and Nusser, 2016). GlyT2-positive inhibitory axons preferentially make synapses onto neurogranin-positive and GlyT2-negative GoCs rather than neurogranin-negative and GlyT2-positive GoCs (Eyre and Nusser, 2016). Consistently, CN neurons releasing both GABA and glycine selectively, but not exclusively, innervate purely GABAergic GoCs (Ankri et al., 2015), which are neurogranin-positive and GlyT2-negative (Simat et al., 2007b). Thus, there may be GoC subtype-dependent circuit organizations, suggesting functional differences among the biochemical and neurochemical subtypes of GoCs.
Similar to the MLIs, GoCs are also electrically coupled via gap junctions (Figure 4E; Dugué et al., 2009; Vervaeke et al., 2010; Hull and Regehr, 2012). Both depolarization and hyperpolarization can be transmitted through these gap junctions, but the inhibition of neighboring GoCs by the transmission of spike afterhyperpolarization appears to be a major effect of their electrical coupling (Dugué et al., 2009; Vervaeke et al., 2010). Computational models suggest that the electrical coupling of GoCs with excitatory inputs from MFs regulates the synchronization and desynchronization of GoC population activity (Dugué et al., 2009; Vervaeke et al., 2010). This dynamic regulation of population activity in local GoC circuits was indeed observed in awake animals (Gurnani and Silver, 2021). These findings raise the possibility that electrical coupling and excitatory synaptic inputs may be the major regulators of GoCs, while relatively minor inhibitory synaptic inputs may modulate specific pathways.
6 Circuits of traditionally minor neuron types
In addition to the major neurons described above, at least four other types of neurons have been reported in the cerebellar cortex (Schilling et al., 2008). Although the cerebellum is a well-studied brain region, these other neuron types are less well understood. Presumably owing to the very small numbers of them within the cerebellum, which is densely packed with GCs and PCs, investigating these minor neurons has been difficult. The lack of clearly distinguishable molecular markers also poses a challenge (Tam et al., 2021). Consequently, there is no unified categorization of these neuron types (Geurts et al., 2001; Kozareva et al., 2021; Tam et al., 2021). Nevertheless, the basic anatomical properties of these relatively minor neuron types are gradually being clarified, and their functions have been proposed based on their anatomical properties.
6.1 UBCs
UBCs are the only excitatory interneurons among them and are located in the GL of the cerebellar vermis. Specifically, UBCs are abundant in cerebellar regions associated with vestibular functions, such as lobules IX and X in the vermis, but are relatively rare in other lobules (Diño et al., 1999). They have a distinctive morphology, characterized by a single short dendrite with a brush-like end. UBCs receive an excitatory input from a single MF and inhibitory inputs from GoCs (Figure 4F-a; Harris et al., 1993; Floris et al., 1994; Kalinichenko and Okhotin, 2005; Mugnaini et al., 2011; McDonough et al., 2020). Their axons branch out locally and form MF terminal-like structures in the GL, which make synapses onto GCs or other UBCs (Mugnaini et al., 2011; van Dorp and De Zeeuw, 2015). UBCs have been thought to temporally amplify short-lived signals arising from a single MF by utilizing their unique circuit structures (Kinney et al., 1997; Mugnaini et al., 2011; van Dorp and De Zeeuw, 2014). However, several studies suggested that UBCs have more complex circuits and functions. Two biochemical subtypes of UBCs have been identified: mGluR1α-positive UBCs, which lack calretinin, and mGluR1α-negative UBCs, which express calretinin (Nunzi et al., 2002; Diño and Mugnaini, 2008). Functionally distinct subtypes appear to be associated with these biochemical differences: MF inputs excite mGluR1α-positive UBCs, but suppress firing in mGluR1α-negative UBCs (Russo et al., 2008; Borges-Merjane and Trussell, 2015; Zampini et al., 2016). Subtype-specific circuit structures have also been reported (Figure 4F-b). Primary sensory afferents from the vestibular organ selectively innervate mGluR1α-positive UBCs, while secondary sensory afferents from the vestibular nucleus innervate both UBC subtypes (Balmer and Trussell, 2019). Additionally, inhibitory connections from PC collateral axons specifically innervate mGluR1α-positive UBCs (Guo et al., 2021). Given these specific circuit structures, UBCs may mediate bidirectional activity patterns in distinct GC pathways by amplifying excitatory signals in one pathway while suppressing firing in another.
6.2 LuCs
LuCs are a type of inhibitory interneuron in the GL, characterized by fusiform somas located beneath PC somas, and are intermediate in size between GoCs and globular cells, which are two other inhibitory interneurons in the GL. Anatomical studies demonstrated that LuCs extend poorly ramified horizontal and bipolar dendrites beneath the PC somas, where they receive inhibitory inputs from PC collateral axons, and project their axons to the ML, where they innervate MLIs (Lainé and Axelrad, 1996, 1998; Simat et al., 2007b). As mentioned above, the functional synaptic connections from PCs to LuCs were confirmed by electrophysiological recordings upon optogenetic activation of PCs (Witter et al., 2016). Another recent study presented a wider range of anatomical connections, revealing inputs from CFs, MFs, GC ascending axons, GoCs, other LuCs, and serotonergic fibers, and axonal projections to GoC dendrites (Figure 4G), but not to PC dendrites (Miyazaki et al., 2021). This raises an intriguing possibility that LuCs integrate various inputs and then coordinate two types of inhibitory circuits, though further functional studies are necessary (Miyazaki et al., 2021).
6.3 Globular cells
Globular cells have often been considered a subtype of LuCs (Lainé and Axelrad, 2002; Schilling et al., 2008; Prestori et al., 2019). Although globular cells exhibit different morphological properties, such as having a small and round soma with radiating dendrites, they share circuit properties with LuCs, in terms of inputs from PC collateral axons and projections to the ML (Lainé and Axelrad, 2002). One functional difference reported was that globular cells responded to both serotonin and noradrenaline, whereas small LuCs responded only to serotonin (Hirono et al., 2012). In addition, studies using single-cell transcriptomic analysis suggested three types of inhibitory interneurons around the PCL, presumably LuCs, globular cells, and candelabrum cells (Kozareva et al., 2021; Osorno et al., 2022). Although the functional relevance of distinguishing LuCs and globular cells is still unclear, the possibility is gradually emerging that they exhibit unique functions due to their distinct molecular expressions.
6.4 Candelabrum cells
Candelabrum cells were identified more than two decades ago as neurons with a small soma situated in the PCL, with a few dendrites extending toward the surface of the ML and axons winding through or above the PCL (Lainé and Axelrad, 1994). They have long been the most enigmatic cell type in the cerebellum. Interestingly, a recent study established a mouse line showing fluorescent labeling in candelabrum cells, sufficiently distinguishing them from other cell types (Osorno et al., 2022). The study found that GABAergic candelabrum cells receive excitatory inputs from MFs and GCs, and strong inhibitory inputs from PC collateral axons, while they inhibited MLIs (Figure 4H). This mouse line may thus facilitate in unraveling the long-standing mystery of candelabrum cells.
7 Discussion: Structural properties that need further clarification
As we have described throughout this article, new structural properties have been demonstrated in the cerebellar cortex. Although the cerebellum has long been thought to consist of an orderly repetition of simple structures, recent findings have revealed that it is actually more complex than previously expected. However, the more details we learn, the more questions arise. Since it is now generally accepted that the cerebellum is involved in cognitive and affective functions in addition to motor functions, there will likely be more studies addressing how the cerebellum processes multiple types of information. For such studies, it is crucial to further understand cerebellar circuit properties. Here, we discuss circuit structures that should be elucidated by considering newly identified structural properties.
7.1 Feedforward and feedback microcircuits
Feedforward and feedback microcircuits are fundamental components of neural computations, widely distributed across various brain regions (D'Souza and Burkhalter, 2017; Park et al., 2023). Among these regions, the cerebellum stands out: GCs receive both feedforward and feedback inhibitions from GoCs, and PCs receive feedforward inhibition from MLIs (D'Angelo et al., 2013; Kim and Augustine, 2021). Furthermore, studies have revealed additional microcircuits within the cerebellar cortex, including feedback inhibitions on various neuron types from PCs and feedforward excitation on GCs mediated by UBCs (Kinney et al., 1997; Mugnaini et al., 2011; van Dorp and De Zeeuw, 2015; Guo et al., 2016; Witter et al., 2016; Guo et al., 2021; Osorno et al., 2022; Hariani et al., 2024). Although the specific arrangements of these microcircuits have been gradually elucidated (Balmer and Trussell, 2019; Guo et al., 2021; Halverson et al., 2022), a comprehensive understanding remains elusive. For example, PC collateral axons send feedback inhibitions to GCs, UBCs, LuCs, candelabrum cells, and MLIs, some of which connect each other, yet their circuit arrangements at the individual neuron level are not clear. There might be organized connections among them, similar to the connections between MLIs and PCs: MLIs appear to receive feedback inhibition from PCs that are distinct from the PCs innervated by those particular MLIs (Halverson et al., 2022). Since the resulting neuronal activity and potential regulations can differ according to the circuit arrangements (Figure 5A), further analyses utilizing advanced techniques, such as a combination of sparse labeling and anterograde transsynaptic labeling, are expected.
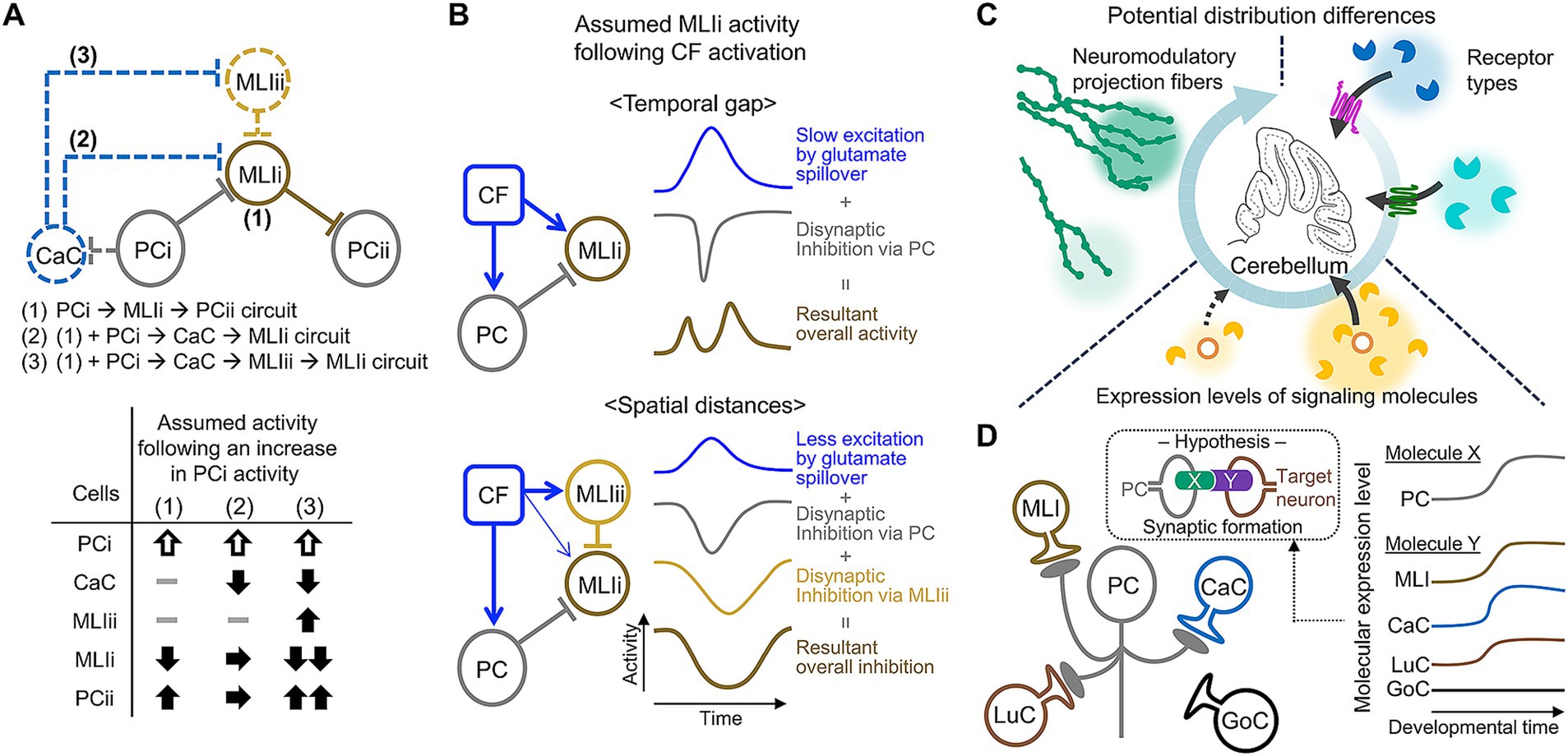
Figure 5. Examples of structural properties requiring further clarification. (A) Three possible circuit arrangements among PCs, MLIs, and candelabrum cells (CaCs) at the individual neuron level (top). The activities of neurons in these circuits may differ in response to an increase in PCi activity (bottom). (B) Two examples of circuit structures involving MLIi, which receives conflicting inputs: excitation from glutamate spillover originating from CF and PC-dependent inhibition. The time course of MLIi activity following CF activation may vary depending on whether the excitatory and inhibitory inputs are temporally distinct (top) or spatially organized (bottom). (C) Potential mechanisms of other factors (e.g., neuromodulators or circulating molecules) exhibiting spatial selectivity. (D) A diagram illustrating the use of developmental time-and cell type-dependent transcriptome analysis data in the research on mechanisms of specific synaptic formation of PC axon collaterals. If the expression of hypothetical molecules X and Y increases in PCs and target neurons, respectively, during developmental periods, these molecules may play a role in synaptic formation. Note that the Roman numbers following PC or MLI in this figure (e.g., PCi or MLIi) are used to differentiate between distinct PCs or MLIs.
7.2 Superficially conflicting functions among new structural organization
A large amount of effort has been made over the past decade to advance our understanding of cerebellar circuits. We assume that the additional organization of cerebellar circuits increases the options of regulating PC activity, consequently leading to pattern separations of signals provided by the cerebellum. However, it is still difficult to picture exactly how such circuit organization collaboratively controls PC activity. Superficially contradicting aspects make it even more challenging. For example, in MLI circuits, MLIs in the inner ML or MLI1s likely have strong inhibitory effects on PCs due to their high efficiency of individual inhibitory synaptic inputs (Arlt and Häusser, 2020) and strong electrical coupling (Alcami and Marty, 2013; Lackey et al., 2024). However, they are inhibited by MLIs in the outer ML or MLI2s (Rieubland et al., 2014; Lackey et al., 2024). This raises concerns that the strong inhibitory effects from the inner MLIs might be underutilized or wasted. Additionally, glutamate spillover from CFs (Coddington et al., 2013; Arlt and Häusser, 2020) and feedback signals from PCs (Witter et al., 2016) could have both excitatory and inhibitory effects on MLIs, raising the possibility that they might functionally cancel each other out. These concerns might be alleviated by obtaining more precise information about the spatiotemporal aspects of MLI circuits, such as temporal gaps or the distinct spatial distances between excitatory and inhibitory effects (Figure 5B). A study provided a clue about the first concern by recording the activity of MLI1s, MLI2s, and PCs during licking, which periodically modulates PC firing (Lackey et al., 2024). Changes in PC firing rates were inversely correlated with MLI1s and correlated with MLI2s, suggesting the organization of bidirectional control of PC firing by these two groups of MLIs. Alternatively, it would be interesting to computationally predict the tuning of PC activities by including all known components of the complex MLI circuit organizations.
7.3 Other factors involved in the cerebellar circuit structures
While this article focuses on neurons and excitatory inputs in the cerebellar cortex, many other factors also contribute to cerebellar circuit structures as components or regulators. These include non-neuronal cells, neuromodulators, and molecules arriving from circulation (Schweighofer et al., 2004; Koibuchi and Ikeda, 2013; Araujo et al., 2019; Guedes et al., 2022; Gruol, 2023; Li et al., 2024). To comprehensively understand cerebellar circuit structures and their functions, these factors must also be taken into account. Indeed, information on their contributions has gradually been updated. For example, immunohistochemical analyses have investigated layer-or lobule-dependent distributions and orientations of neuromodulatory projection fibers (Carlson et al., 2021; Longley et al., 2021). Other studies have reported novel interactions between PCs and Bergmann glial cells through dopamine released from PCs (Li et al., 2023), and cerebellar circuit formation through cytokine-dependent regulation of microglial functions (Kana et al., 2019; Guedes et al., 2023). Regulation by these factors appears less selective than synaptic transmission-mediated regulation, as evidenced by volume transmission of neuromodulators (Özçete et al., 2024) and delivery of cytokines through circulation (Wu et al., 2023). Nevertheless, recent studies have shown their involvement in specific cerebellar functions or disfunctions (Yamamoto et al., 2019; Kim et al., 2021; Cutando et al., 2022; Snell et al., 2022; Guedes et al., 2023; Li et al., 2023; Stanley et al., 2023; Dewa et al., 2024). As the broad range of cerebellar functions appear to be achieved through computations in different functional modules/domains, as shown in human studies (Guell et al., 2018; Diedrichsen et al., 2019; King et al., 2019), it would be intriguing to determine how the factors described in this paragraph exhibit a certain extent of spatial selectivity within the cerebellum (Figure 5C).
7.4 Circuit organization during postnatal development
Well-organized circuit structures of the cerebellum gradually form during postnatal development through dynamic processes regulated by both cell-autonomous mechanisms and interactions between developing neurons in the cerebellum (Leto et al., 2016; Park et al., 2021; van der Heijden and Sillitoe, 2021; Kim et al., 2023a). While extensive research has focused on these processes for traditionally known circuit structures, less is understood about the developmental mechanisms of newly identified structures. The updated structures are relatively minor or complex, complicating the investigation of their developmental processes. In general, a useful approach to understand developmental processes may be to examine the effects of molecular deficiencies during specific developmental windows. Developmental time-and cell type-dependent transcriptome analysis data could help to identify relevant molecules (Figure 5D). This approach indeed led us to discover developmental mechanisms that maintain the PC soma monolayer after its formation. Specifically, multiple epidermal growth factor-like domains protein 11, which substantially increases during the late stage of GC development (Rosenberg et al., 2018), plays a role in this maintenance (Jun et al., 2023). Furthermore, integrating transcriptomic data with known information about circuit formation could be beneficial for hypothesizing the developmental mechanisms of the updated structures. For example, neuropilin-1 (Nrp1) expressed in BCs is crucial for the formation of pinceau synapses in the AIS of PCs (Telley et al., 2016). Whereas candelabrum cells also express high levels of Nrp1, they do not innervate PCs (Osorno et al., 2022). Two potential mechanisms are proposed to explain this discrepancy (Schilling, 2024): the timing of expression of Nrp1 and its partner proteins in PCs, MLIs, or candelabrum cells might be regulated, or different splice variants of Nrp1 may contribute differently to their synaptic connections. Thus, hypotheses based on existing data would help advance our understanding of how cerebellar circuits, including newly identified structures, are coordinately organized during postnatal development.
8 Conclusion and perspectives
This article highlights recent advances in our understanding of cerebellar cortex circuit structures, including greater heterogeneity among neuron types, previously unidentified synaptic connections, and more complex circuit organizations. In general, characteristic circuit structures within the cerebellum are believed essential for neural computations and their roles in behavior (D'Angelo et al., 2011; Billings et al., 2014). In line with this belief, existing theories propose functions for some newly identified circuit structures, such as aspects of MLI organization that may contribute to the functional microdomains of PCs (Coddington et al., 2013; Witter et al., 2016; Hoehne et al., 2020; Halverson et al., 2022), or the two types of UBCs that may convey different vestibular information bidirectionally to distinct GC pathways (Russo et al., 2008; Borges-Merjane and Trussell, 2015; Zampini et al., 2016; Balmer and Trussell, 2019). Ideally, our understanding of the structural-functional relationships in cerebellar circuits will be enhanced through the proposal and validation of further theories. Constructing theoretical models based on experimental observations would be valuable for comprehensively predicting the cooperative or antagonistic effects of various circuit structures. The development of techniques for targeted manipulations, such as inhibiting specific connections, collateral axons, or cell types, would facilitate experimental validation. Transcriptomic data could be instrumental in this technical development. It is crucial to investigate through these analyses how the unique circuit structures and neuronal morphologies in the cerebellar cortex contribute to neural computation, extending beyond the structural studies discussed in the previous section (6.1). This would advance our understanding of cerebellar functions and the broader mechanisms underlying brain operation.
Author contributions
SJ: Writing – original draft, Writing – review & editing. HP: Writing – original draft. MK: Writing – original draft. SK: Writing – original draft. TK: Writing – original draft. DK: Writing – original draft. YY: Conceptualization, Funding acquisition, Resources, Supervision, Visualization, Writing – original draft, Writing – review & editing. KT-Y: Conceptualization, Funding acquisition, Resources, Supervision, Validation, Visualization, Writing – original draft, Writing – review & editing.
Funding
The author(s) declare that financial support was received for the research, authorship, and/or publication of this article. This work was supported by the KIST Institutional Program (project no.: 2E32901), the National Research Foundation of Korea (NRF) grant funded by the Korean Ministry of Science and ICT (NRF grant nos.: 2021R1C1C2007843, 2022R1A2C2006857, and RS-2024-00338607), and the National R&D Program through the NRF funded by MSIT (grant no. 2021M3F3A2A01037811).
Acknowledgments
We thank Taegon Kim for valuable discussions during constructing and writing the manuscript.
Conflict of interest
The authors declare that the research was conducted in the absence of any commercial or financial relationships that could be construed as a potential conflict of interest.
Publisher’s note
All claims expressed in this article are solely those of the authors and do not necessarily represent those of their affiliated organizations, or those of the publisher, the editors and the reviewers. Any product that may be evaluated in this article, or claim that may be made by its manufacturer, is not guaranteed or endorsed by the publisher.
References
Abbott, L. C., Isaacs, K. R., and Heckroth, J. A. (1996). Co-localization of tyrosine hydroxylase and zebrin II immunoreactivities in Purkinje cells of the mutant mice, tottering and tottering/leaner. Neuroscience 71, 461–475. doi: 10.1016/0306-4522(95)00444-0
Alcami, P., and Marty, A. (2013). Estimating functional connectivity in an electrically coupled interneuron network. Proc. Natl. Acad. Sci. USA 110, E4798–E4807. doi: 10.1073/pnas.1310983110
Anastassiou, C. A., and Koch, C. (2015). Ephaptic coupling to endogenous electric field activity: why bother? Curr. Opin. Neurobiol. 31, 95–103. doi: 10.1016/j.conb.2014.09.002
Ankri, L., Husson, Z., Pietrajtis, K., Proville, R., Léna, C., Yarom, Y., et al. (2015). A novel inhibitory nucleo-cortical circuit controls cerebellar Golgi cell activity. eLife 4:e06262. doi: 10.7554/eLife.06262
Apps, R., and Hawkes, R. (2009). Cerebellar cortical organization: a one-map hypothesis. Nat. Rev. Neurosci. 10, 670–681. doi: 10.1038/nrn2698
Araujo, A. P. B., Carpi-Santos, R., and Gomes, F. C. A. (2019). The role of astrocytes in the development of the cerebellum. Cerebellum 18, 1017–1035. doi: 10.1007/s12311-019-01046-0
Arlt, C., and Häusser, M. (2020). Microcircuit rules governing impact of single interneurons on Purkinje cell output in vivo. Cell Rep. 30, 3020–3035 e 3023. doi: 10.1016/j.celrep.2020.02.009
Arvanitaki, A. (1942). Effects evoked in an axon by the activity of a contiguous one. J. Neurophysiol. 5, 89–108. doi: 10.1152/jn.1942.5.2.89
Babij, R., Lee, M., Cortés, E., Vonsattel, J. P., Faust, P. L., and Louis, E. D. (2013). Purkinje cell axonal anatomy: quantifying morphometric changes in essential tremor versus control brains. Brain 136, 3051–3061. doi: 10.1093/brain/awt238
Balmer, T. S., and Trussell, L. O. (2019). Selective targeting of unipolar brush cell subtypes by cerebellar mossy fibers. eLife 8:e44964. doi: 10.7554/eLife.44964
Bao, J., Reim, K., and Sakaba, T. (2010). Target-dependent feedforward inhibition mediated by short-term synaptic plasticity in the cerebellum. J. Neurosci. 30, 8171–8179. doi: 10.1523/JNEUROSCI.0276-10.2010
Barmack, N. H., and Yakhnitsa, V. (2008). Functions of interneurons in mouse cerebellum. J. Neurosci. 28, 1140–1152. doi: 10.1523/JNEUROSCI.3942-07.2008
Bernard, C., Axelrad, H., and Giraud, B. G. (1993). Effects of collateral inhibition in a model of the immature rat cerebellar cortex-multineuron correlations. Cogn. Brain Res. 1, 100–122. doi: 10.1016/0926-6410(93)90016-X
Billings, G., Piasini, E., Lőrincz, A., Nusser, Z., and Silver, R. A. (2014). Network structure within the cerebellar input layer enables lossless sparse encoding. Neuron 83, 960–974. doi: 10.1016/j.neuron.2014.07.020
Bishop, G. A. (1982). The pattern of distribution of the local axonal collaterals of Purkinje cells in the intermediate cortex of the anterior lobe and paramedian lobule of the cat cerebellum. J. Comp. Neurol. 210, 1–9. doi: 10.1002/cne.902100102
Blackman, A. V., Abrahamsson, T., Costa, R. P., Lalanne, T., and Sjöström, P. J. (2013). Target-cell-specific short-term plasticity in local circuits. Front. Synaptic Neurosci. 5:11. doi: 10.3389/fnsyn.2013.00011
Blot, A., and Barbour, B. (2014). Ultra-rapid axon-axon ephaptic inhibition of cerebellar Purkinje cells by the pinceau. Nat. Neurosci. 17, 289–295. doi: 10.1038/nn.3624
Blot, F. G. C., White, J. J., van Hattem, A., Scotti, L., Balaji, V., Adolfs, Y., et al. (2023). Purkinje cell microzones mediate distinct kinematics of a single movement. Nat. Commun. 14:4358. doi: 10.1038/s41467-023-40111-5
Borges-Merjane, C., and Trussell, L. O. (2015). ON and OFF unipolar brush cells transform multisensory inputs to the auditory system. Neuron 85, 1029–1042. doi: 10.1016/j.neuron.2015.02.009
Bower, J. M. (2010). Model-founded explorations of the roles of molecular layer inhibition in regulating purkinje cell responses in cerebellar cortex: more trouble for the beam hypothesis. Front. Cell. Neurosci. 4:27. doi: 10.3389/fncel.2010.00027
Busch, S. E., and Hansel, C. (2023). Climbing fiber multi-innervation of mouse Purkinje dendrites with arborization common to human. Science 381, 420–427. doi: 10.1126/science.adi1024
Cadilhac, C., Bachy, I., Forget, A., Hodson, D. J., Jahannault-Talignani, C., Furley, A. J., et al. (2021). Excitatory granule neuron precursors orchestrate laminar localization and differentiation of cerebellar inhibitory interneuron subtypes. Cell Rep. 34:108904. doi: 10.1016/j.celrep.2021.108904
Cameron, D. B., Kasai, K., Jiang, Y., Hu, T., Saeki, Y., and Komuro, H. (2009). Four distinct phases of basket/stellate cell migration after entering their final destination (the molecular layer) in the developing cerebellum. Dev. Biol. 332, 309–324. doi: 10.1016/j.ydbio.2009.05.575
Carlson, E. S., Hunker, A. C., Sandberg, S. G., Locke, T. M., Geller, J. M., Schindler, A. G., et al. (2021). Catecholaminergic innervation of the lateral nucleus of the cerebellum modulates cognitive behaviors. J. Neurosci. 41, 3512–3530. doi: 10.1523/jneurosci.2406-20.2021
Carter, R. A., Bihannic, L., Rosencrance, C., Hadley, J. L., Tong, Y., Phoenix, T. N., et al. (2018). A single-cell transcriptional atlas of the developing murine cerebellum. Curr. Biol. 28, 2910–2920.e2. doi: 10.1016/j.cub.2018.07.062
Carter, A. G., and Regehr, W. G. (2000). Prolonged synaptic currents and glutamate spillover at the parallel Fiber to stellate cell synapse. J. Neurosci. 20, 4423–4434. doi: 10.1523/JNEUROSCI.20-12-04423.2000
Cayco-Gajic, N. A., Clopath, C., and Silver, R. A. (2017). Sparse synaptic connectivity is required for decorrelation and pattern separation in feedforward networks. Nat. Commun. 8:1116. doi: 10.1038/s41467-017-01109-y
Cayco-Gajic, N. A., and Silver, R. A. (2019). Re-evaluating circuit mechanisms underlying pattern separation. Neuron 101, 584–602. doi: 10.1016/j.neuron.2019.01.044
Cerminara, N. L., Lang, E. J., Sillitoe, R. V., and Apps, R. (2015). Redefining the cerebellar cortex as an assembly of non-uniform Purkinje cell microcircuits. Nat. Rev. Neurosci. 16, 79–93. doi: 10.1038/nrn3886
Cesana, E., Pietrajtis, K., Bidoret, C., Isope, P., D'Angelo, E., Dieudonné, S., et al. (2013). Granule cell ascending axon excitatory synapses onto Golgi cells implement a potent feedback circuit in the cerebellar granular layer. J. Neurosci. 33, 12430–12446. doi: 10.1523/JNEUROSCI.4897-11.2013
Chabrol, F. P., Arenz, A., Wiechert, M. T., Margrie, T. W., and DiGregorio, D. A. (2015). Synaptic diversity enables temporal coding of coincident multisensory inputs in single neurons. Nat. Neurosci. 18, 718–727. doi: 10.1038/nn.3974
Chang, W., Pedroni, A., Hohendorf, V., Giacomello, S., Hibi, M., Köster, R. W., et al. (2020). Functionally distinct Purkinje cell types show temporal precision in encoding locomotion. Proc. Natl. Acad. Sci. USA 117, 17330–17337. doi: 10.1073/pnas.2005633117
Chan-Palay, V., and Palay, S. L. (1971). The synapse en marron between Golgi II neurons and mossy fibers in the Rat's cerebellar cortex. Z. Anat. Entwicklungsgesch. 133, 274–287. doi: 10.1007/BF00519303
Clark, B. A., and Cull-Candy, S. G. (2002). Activity-dependent recruitment of extrasynaptic NMDA receptor activation at an AMPA receptor-only synapse. J. Neurosci. 22, 4428–4436. doi: 10.1523/jneurosci.22-11-04428.2002
Coddington, L. T., Rudolph, S., Vande Lune, P., Overstreet-Wadiche, L., and Wadiche, J. I. (2013). Spillover-mediated feedforward inhibition functionally segregates interneuron activity. Neuron 78, 1050–1062. doi: 10.1016/j.neuron.2013.04.019
Consalez, G. G., and Hawkes, R. (2012). The compartmental restriction of cerebellar interneurons. Front. Neural. Circuits 6:123. doi: 10.3389/fncir.2012.00123
Coulon, P., and Landisman, C. E. (2017). The potential role of gap junctional plasticity in the regulation of state. Neuron 93, 1275–1295. doi: 10.1016/j.neuron.2017.02.041
Crowley, J. J., Fioravante, D., and Regehr, W. G. (2009). Dynamics of fast and slow inhibition from cerebellar golgi cells allow flexible control of synaptic integration. Neuron 63, 843–853. doi: 10.1016/j.neuron.2009.09.004
Cutando, L., Puighermanal, E., Castell, L., Tarot, P., Belle, M., Bertaso, F., et al. (2022). Cerebellar dopamine D2 receptors regulate social behaviors. Nat. Neurosci. 25, 900–911. doi: 10.1038/s41593-022-01092-8
D'Angelo, E., and Casali, S. (2012). Seeking a unified framework for cerebellar function and dysfunction: from circuit operations to cognition. Front. Neural Circuits 6:116. doi: 10.3389/fncir.2012.00116
D'Angelo, E., Filippi, G. D., Rossi, P., and Taglietti, V. (1998). Ionic mechanism of Electroresponsiveness in cerebellar granule cells implicates the action of a persistent sodium current. J. Neurophysiol. 80, 493–503. doi: 10.1152/jn.1998.80.2.493
D'Angelo, E., Mazzarello, P., Prestori, F., Mapelli, J., Solinas, S., Lombardo, P., et al. (2011). The cerebellar network: from structure to function and dynamics. Brain Res. Rev. 66, 5–15. doi: 10.1016/j.brainresrev.2010.10.002
D'Angelo, E., Solinas, S., Mapelli, J., Gandolfi, D., Mapelli, L., and Prestori, F. (2013). The cerebellar Golgi cell and spatiotemporal organization of granular layer activity. Front. Neural. Circuits 7:93. doi: 10.3389/fncir.2013.00093
De Zeeuw, C. I. (2021). Bidirectional learning in upbound and downbound microzones of the cerebellum. Nat. Rev. Neurosci. 22, 92–110. doi: 10.1038/s41583-020-00392-x
De Zeeuw, C. I., Hoebeek, F. E., Bosman, L. W., Schonewille, M., Witter, L., and Koekkoek, S. K. (2011). Spatiotemporal firing patterns in the cerebellum. Nat. Rev. Neurosci. 12, 327–344. doi: 10.1038/nrn3011
Dewa, K. I., Arimura, N., Kakegawa, W., Itoh, M., Adachi, T., Miyashita, S., et al. (2024). Neuronal DSCAM regulates the peri-synaptic localization of GLAST in Bergmann glia for functional synapse formation. Nat. Commun. 15:458. doi: 10.1038/s41467-023-44579-z
Dey, M. R., Reddy, K., Yoshida, H., Nishiyama, N., Zemelman, B. V., and Nishiyama, H. (2022). Granule cells constitute one of the major neuronal subtypes in the molecular layer of the posterior cerebellum. eNeuro 9, ENEURO.0289–ENEU21.2022. doi: 10.1523/eneuro.0289-21.2022
Diedrichsen, J., King, M., Hernandez-Castillo, C., Sereno, M., and Ivry, R. B. (2019). Universal transform or multiple functionality? Understanding the contribution of the human cerebellum across task domains. Neuron 102, 918–928. doi: 10.1016/j.neuron.2019.04.021
Dieudonné, S. (1998). Submillisecond kinetics and low efficacy of parallel fibre—Golgi cell synaptic currents in the rat cerebellum. J. Physiol. 510, 845–866. doi: 10.1111/j.1469-7793.1998.845bj.x
Diño, M. R., and Mugnaini, E. (2008). Distribution and phenotypes of unipolar brush cells in relation to the granule cell system of the rat cochlear nucleus. Neuroscience 154, 29–50. doi: 10.1016/j.neuroscience.2008.01.035
Diño, M. R., Willard, F. H., and Mugnaini, E. (1999). Distribution of unipolar brush cells and other calretinin immunoreactive components in the mammalian cerebellar cortex. J. Neurocytol. 28, 99–123. doi: 10.1023/a:1007072105919
Dorgans, K., Demais, V., Bailly, Y., Poulain, B., Isope, P., and Doussau, F. (2019). Short-term plasticity at cerebellar granule cell to molecular layer interneuron synapses expands information processing. eLife 8:e41586. doi: 10.7554/eLife.41586
D'Souza, R. D., and Burkhalter, A. (2017). A laminar organization for selective Cortico-cortical communication. Front. Neuroanat. 11:71. doi: 10.3389/fnana.2017.00071
Dugué, G. P., Brunel, N., Hakim, V., Schwartz, E., Chat, M., Lévesque, M., et al. (2009). Electrical coupling mediates tunable low-frequency oscillations and resonance in the cerebellar Golgi cell network. Neuron 61, 126–139. doi: 10.1016/j.neuron.2008.11.028
Dumoulin, A., Triller, A., and Dieudonné, S. (2001). IPSC kinetics at identified GABAergic and mixed GABAergic and glycinergic synapses onto cerebellar Golgi cells. J. Neurosci. 21, 6045–6057. doi: 10.1523/JNEUROSCI.21-16-06045.2001
Eccles, J. C., Ito, M., and Szentágothai, J. (1967). The cerebellum as a neuronal machine. Berlin, Heidelberg: Springer.
Eccles, J. C., Llinás, R., and Sasaki, K. (1966). Parallel fibre stimulation and the responses induced thereby in the Purkinje cells of the cerebellum. Exp. Brain Res. 1, 17–39. doi: 10.1007/bf00235207
Eyre, M. D., and Nusser, Z. (2016). Only a minority of the inhibitory inputs to cerebellar Golgi cells originates from local GABAergic cells. eNeuro 3, ENEURO.0055–ENEU16.2016. doi: 10.1523/ENEURO.0055-16.2016
Faber, D. S., and Pereda, A. E. (2018). Two forms of electrical transmission between neurons. Front. Mol. Neurosci. 11:427. doi: 10.3389/fnmol.2018.00427
Fleming, E. A., Field, G. D., Tadross, M. R., and Hull, C. (2024). Local synaptic inhibition mediates cerebellar granule cell pattern separation and enables learned sensorimotor associations. Nat. Neurosci. 27, 689–701. doi: 10.1038/s41593-023-01565-4
Floris, A., Diño, M., Jacobowitz, D. M., and Muganaini, E. (1994). The unipolar brush cells of the rat cerebellar cortex and cochlear nucleus are calretinin-positive-a study by light and electron microscopic immunocytochemistry. Anat. Embryol. 189, 495–520. doi: 10.1007/BF00186824
Foster, K. A., Kreitzer, A. C., and Regehr, W. G. (2002). Interaction of postsynaptic receptor saturation with presynaptic mechanisms produces a reliable synapse. Neuron 36, 1115–1126. doi: 10.1016/S0896-6273(02)01106-6
Galarreta, M., and Hestrin, S. (2001). Electrical synapses between GABA-releasing interneurons. Nat. Rev. Neurosci. 2, 425–433. doi: 10.1038/35077566
Galliano, E., Baratella, M., Sgritta, M., Ruigrok, T. J., Haasdijk, E. D., Hoebeek, F. E., et al. (2013). Anatomical investigation of potential contacts between climbing fibers and cerebellar Golgi cells in the mouse. Front. Neural. Circuits 7:59. doi: 10.3389/fncir.2013.00059
Gandolfi, D., Pozzi, P., Tognolina, M., Chirico, G., Mapelli, J., and D'Angelo, E. (2014). The spatiotemporal organization of cerebellar network activity resolved by two-photon imaging of multiple single neurons. Front. Cell. Neurosci. 8:92. doi: 10.3389/fncel.2014.00092
Geurts, F. J., Timmermans, J., Shigemoto, R., and Schutter, E. D. (2001). Morphological and neurochemical differentiation of large granular layer interneurons in the adult rat cerebellum. Neuroscience 104, 499–512. doi: 10.1016/S0306-4522(01)00058-6
Giovannucci, A., Badura, A., Deverett, B., Najafi, F., Pereira, T. D., Gao, Z., et al. (2017). Cerebellar granule cells acquire a widespread predictive feedback signal during motor learning. Nat. Neurosci. 20, 727–734. doi: 10.1038/nn.4531
Gruol, D. L. (2023). The neuroimmune system and the cerebellum. Cerebellum. doi: 10.1007/s12311-023-01624-3
Guedes, J. R., Ferreira, P. A., Costa, J. M., Cardoso, A. L., and Peça, J. (2022). Microglia-dependent remodeling of neuronal circuits. J. Neurochem. 163, 74–93. doi: 10.1111/jnc.15689
Guedes, J. R., Ferreira, P. A., Costa, J., Laranjo, M., Pinto, M. J., Reis, T., et al. (2023). IL-4 shapes microglia-dependent pruning of the cerebellum during postnatal development. Neuron 111, 3435–3449.e8. doi: 10.1016/j.neuron.2023.09.031
Guell, X., Schmahmann, J. D., Gabrieli, J., and Ghosh, S. S. (2018). Functional gradients of the cerebellum. eLife 7:e36652. doi: 10.7554/eLife.36652
Guo, C., Rudolph, S., Neuwirth, M. E., and Regehr, W. G. (2021). Purkinje cell outputs selectively inhibit a subset of unipolar brush cells in the input layer of the cerebellar cortex. eLife 10:e68802. doi: 10.7554/eLife.68802
Guo, C., Witter, L., Rudolph, S., Elliott, H. L., Ennis, K. A., and Regehr, W. G. (2016). Purkinje cells directly inhibit granule cells in specialized regions of the cerebellar cortex. Neuron 91, 1330–1341. doi: 10.1016/j.neuron.2016.08.011
Gurnani, H., and Silver, R. A. (2021). Multidimensional population activity in an electrically coupled inhibitory circuit in the cerebellar cortex. Neuron 109, 1739–1753.e8. doi: 10.1016/j.neuron.2021.03.027
Halverson, H. E., Kim, J., Khilkevich, A., Mauk, M. D., and Augustine, G. J. (2022). Feedback inhibition underlies new computational functions of cerebellar interneurons. eLife 11:e77603. doi: 10.7554/eLife.77603
Hámori, J., and Szentágothai, J. (1968). Identification of synapses formed in the cerebellar cortex by Purkinie axon collaterals-an Electron microscope study. Exp. Brain Res. 5, 118–128. doi: 10.1007/BF00238701
Hámori, J., and Szentágothai, J. (1980). Lack of evidence of synaptic contacts by climbing fibre collaterals to basket and stellate cells in developing rat cerebellar cortex. Brain Res. 186, 454–457. doi: 10.1016/0006-8993(80)90990-7
Han, K. S., Chen, C. H., Khan, M. M., Guo, C., and Regehr, W. G. (2020). Climbing fiber synapses rapidly and transiently inhibit neighboring Purkinje cells via ephaptic coupling. Nat. Neurosci. 23, 1399–1409. doi: 10.1038/s41593-020-0701-z
Han, K. S., Guo, C., Chen, C. H., Witter, L., Osorno, T., and Regehr, W. G. (2018). Ephaptic coupling promotes synchronous firing of cerebellar Purkinje cells. Neuron 100, 564–578.e3. doi: 10.1016/j.neuron.2018.09.018
Hariani, H. N., Algstam, A. B., Candler, C. T., Witteveen, I. F., Sidhu, J. K., and Balmer, T. S. (2024). A system of feed-forward cerebellar circuits that extend and diversify sensory signaling. eLife 12:RP88321. doi: 10.7554/eLife.88321
Harris, J., Moreno, S., Shaw, G., and Mugnaini, E. (1993). Unusual neurofilament composition in cerebellar unipolar brush neurons. J. Neurocytol. 22, 1039–1059. doi: 10.1007/BF01235748
Harrison, J., and Jahr, C. E. (2003). Receptor occupancy limits synaptic depression at climbing Fiber synapses. J. Neurosci. 23, 377–383. doi: 10.1523/JNEUROSCI.23-02-00377.2003
Häusser, M., and Clark, B. A. (1997). Tonic synaptic inhibition modulates neuronal output pattern and spatiotemporal synaptic integration. Neuron 19, 665–678. doi: 10.1016/S0896-6273(00)80379-7
Hawkes, R., and Leclerc, N. (1989). Purkinje cell axon collateral distributions reflect the chemical compartmentation of the rat cerebellar cortex. Brain Res. 476, 279–290. doi: 10.1016/0006-8993(89)91248-1
Herculano-Houzel, S., Catania, K., Manger, P. R., and Kaas, J. H. (2015). Mammalian brains are made of these: a dataset of the numbers and densities of neuronal and nonneuronal cells in the brain of Glires, Primates, Scandentia, Eulipotyphlans, Afrotherians and artiodactyls, and their relationship with body mass. Brain Behav. Evol. 86, 145–163. doi: 10.1159/000437413
Hirono, M., Saitow, F., Kudo, M., Suzuki, H., Yanagawa, Y., Yamada, M., et al. (2012). Cerebellar globular cells receive monoaminergic excitation and monosynaptic inhibition from Purkinje cells. PLoS One 7:e29663. doi: 10.1371/journal.pone.0029663
Hoehne, A., McFadden, M. H., and DiGregorio, D. A. (2020). Feed-forward recruitment of electrical synapses enhances synchronous spiking in the mouse cerebellar cortex. eLife 9:e57344. doi: 10.7554/eLife.57344
Houston, C. M., Diamanti, E., Diamantaki, M., Kutsarova, E., Cook, A., Sultan, F., et al. (2017). Exploring the significance of morphological diversity for cerebellar granule cell excitability. Sci. Rep. 7:46147. doi: 10.1038/srep46147
Hull, C., and Regehr, W. G. (2012). Identification of an inhibitory circuit that regulates cerebellar Golgi cell activity. Neuron 73, 149–158. doi: 10.1016/j.neuron.2011.10.030
Ichikawa, R., Yamasaki, M., Miyazaki, T., Konno, K., Hashimoto, K., Tatsumi, H., et al. (2011). Developmental switching of perisomatic innervation from climbing fibers to basket cell fibers in cerebellar Purkinje cells. J. Neurosci. 31, 16916–16927. doi: 10.1523/JNEUROSCI.2396-11.2011
Ikai, Y., Takada, M., Shinonaga, Y., and Mizuno, N. (1992). Dopaminergic and non-dopaminergic neurons in the ventral tegmental area of the rat project, respectively, to the cerebellar cortex and deep cerebellar nuclei. Neuroscience 51, 719–728. doi: 10.1016/0306-4522(92)90310-x
Ito, M. (2006). Cerebellar circuitry as a neuronal machine. Prog. Neurobiol. 78, 272–303. doi: 10.1016/j.pneurobio.2006.02.006
James, S. A. (1971). A theory of cerebellar function. Math. Biosci. 10, 25–61. doi: 10.1016/0025-5564(71)90051-4
Jörntell, H., Bengtsson, F., Schonewille, M., and De Zeeuw, C. I. (2010). Cerebellar molecular layer interneurons - computational properties and roles in learning. Trends Neurosci. 33, 524–532. doi: 10.1016/j.tins.2010.08.004
Jörntell, H., and Ekerot, C.-F. (2003). Receptive Field plasticity profoundly alters the cutaneous parallel Fiber synaptic input to cerebellar interneurons in vivo. J. Neurosci. 23, 9620–9631. doi: 10.1523/JNEUROSCI.23-29-09620.2003
Jun, S., Kim, M., Park, H., Hwang, E., Yamamoto, Y., and Tanaka-Yamamoto, K. (2023). Organization of Purkinje cell development by neuronal MEGF11 in cerebellar granule cells. Cell Rep. 42:113137. doi: 10.1016/j.celrep.2023.113137
Kalinichenko, S. G., and Okhotin, V. E. (2005). Unipolar brush cells--a new type of excitatory interneuron in the cerebellar cortex and cochlear nuclei of the brainstem. Neurosci. Behav. Physiol. 35, 21–36. doi: 10.1023/b:neab.0000049648.20702.ad
Kana, V., Desland, F. A., Casanova-Acebes, M., Ayata, P., Badimon, A., Nabel, E., et al. (2019). CSF-1 controls cerebellar microglia and is required for motor function and social interaction. J. Exp. Med. 216, 2265–2281. doi: 10.1084/jem.20182037
Katz, B., and Schmitt, O. H. (1940). Electric interaction between two adjacent nerve fibres. J. Physiol. 97, 471–488. doi: 10.1113/jphysiol.1940.sp003823
Kim, J., and Augustine, G. J. (2021). Molecular layer interneurons: key elements of cerebellar network computation and behavior. Neuroscience 462, 22–35. doi: 10.1016/j.neuroscience.2020.10.008
Kim, J. E., Chae, S., Kim, S., Jung, Y. J., Kang, M. G., Heo, W., et al. (2021). Cerebellar 5HT-2A receptor mediates stress-induced onset of dystonia. Sci. Adv. 7:eabb5735. doi: 10.1126/sciadv.abb5735
Kim, M., Jun, S., Park, H., Tanaka-Yamamoto, K., and Yamamoto, Y. (2023a). Regulation of cerebellar network development by granule cells and their molecules. Front. Mol. Neurosci. 16:1236015. doi: 10.3389/fnmol.2023.1236015
Kim, J., Lee, S., Tsuda, S., Zhang, X., Asrican, B., Gloss, B., et al. (2014). Optogenetic mapping of cerebellar inhibitory circuitry reveals spatially biased coordination of interneurons via electrical synapses. Cell Rep. 7, 1601–1613. doi: 10.1016/j.celrep.2014.04.047
Kim, T., Park, H., Tanaka-Yamamoto, K., and Yamamoto, Y. (2023b). Developmental timing-dependent organization of synaptic connections between mossy fibers and granule cells in the cerebellum. Commun Biol. 6:446. doi: 10.1038/s42003-023-04825-y
King, J. S., Chen, Y. F., and Bishop, G. A. (1993). An analysis of HRP-filled basket cell axons in the cat’s cerebellum II. Axonal distribution. Anat Embryol 188, 299–305. doi: 10.1007/BF00188220
King, M., Hernandez-Castillo, C. R., Poldrack, R. A., Ivry, R. B., and Diedrichsen, J. (2019). Functional boundaries in the human cerebellum revealed by a multi-domain task battery. Nat. Neurosci. 22, 1371–1378. doi: 10.1038/s41593-019-0436-x
Kinney, G. A., Overstreet, L. S., and Slater, N. T. (1997). Prolonged physiological entrapment of glutamate in the synaptic cleft of cerebellar unipolar brush cells. J. Neurophysiol. 78, 1320–1333. doi: 10.1152/jn.1997.78.3.1320
Knogler, L. D., Markov, D. A., Dragomir, E. I., Štih, V., and Portugues, R. (2017). Sensorimotor representations in cerebellar granule cells in larval zebrafish are dense, spatially organized, and non-temporally patterned. Curr. Biol. 27, 1288–1302. doi: 10.1016/j.cub.2017.03.029
Koibuchi, N., and Ikeda, Y. (2013). Hormones and cerebellar development. eds. M. Manto, J. D. Schmahmann, F. Rossi, and D. L. Gruol (Dordrecht: Springer Netherlands).
Kollo, M., Holderith, N. B., and Nusser, Z. (2006). Novel subcellular distribution pattern of A-type K+ channels on neuronal surface. J. Neurosci. 26, 2684–2691. doi: 10.1523/JNEUROSCI.5257-05.2006
Korn, H., and Axelrad, H. (1980). Electrical inhibition of Purkinje cells in the cerebellum of the rat. Proc. Natl. Acad. Sci. USA 77, 6244–6247. doi: 10.1073/pnas.77.10.6244
Koutsikou, S., Crook, J. J., Earl, E. V., Leith, J. L., Watson, T. C., Lumb, B. M., et al. (2014). Neural substrates underlying fear-evoked freezing: the periaqueductal grey-cerebellar link. J. Physiol. 592, 2197–2213. doi: 10.1113/jphysiol.2013.268714
Kozareva, V., Martin, C., Osorno, T., Rudolph, S., Guo, C., Vanderburg, C., et al. (2021). A transcriptomic atlas of mouse cerebellar cortex comprehensively defines cell types. Nature 598, 214–219. doi: 10.1038/s41586-021-03220-z
Lackey, E. P., Moreira, L., Norton, A., Hemelt, M. E., Osorno, T., Nguyen, T. M., et al. (2024). Specialized connectivity of molecular layer interneuron subtypes leads to disinhibition and synchronous inhibition of cerebellar Purkinje cells. Neuron 112, 2333–2348.e6. doi: 10.1016/j.neuron.2024.04.010
Lainé, J., and Axelrad, H. (1994). The candelabrum cell: a new interneuron in the cerebellar cortex. J. Comp. Neurol. 339, 159–173. doi: 10.1002/cne.903390202
Lainé, J., and Axelrad, H. (1996). Morphology of the Golgi-impregnated Lugaro cell in the rat cerebellar cortex: a reappraisal with a description of its axon. J. Comp. Neurol. 375, 618–640. doi: 10.1002/(sici)1096-9861(19961125)375:4<618::Aid-cne5>3.0.Co;2-4
Lainé, J., and Axelrad, H. (1998). Lugaro cells target basket and stellate cells in the cerebellar cortex. Neuroreport 9, 2399–2403. doi: 10.1097/00001756-199807130-00045
Lainé, J., and Axelrad, H. (2002). Extending the cerebellar Lugaro cell class. Neuroscience 115, 363–374. doi: 10.1016/s0306-4522(02)00421-9
Lang-Ouellette, D., Gruver, K. M., Smith-Dijak, A., Blot, F. G. C., Stewart, C. A., de Blavous, P., et al. (2021). Purkinje cell axonal swellings enhance action potential fidelity and cerebellar function. Nat. Commun. 12:4129. doi: 10.1038/s41467-021-24390-4
Larramendi, L. M., and Lemkey-Johnston, N. (1970). The distribution of recurrent Purkinje collateral synapses in the mouse cerebellar cortex: an electron microscopic study. J. Comp. Neurol. 138, 451–482. doi: 10.1002/cne.901380405
Lein, E. S., Hawrylycz, M. J., Ao, N., Ayres, M., Bensinger, A., Bernard, A., et al. (2007). Genome-wide atlas of gene expression in the adult mouse brain. Nature 445, 168–176. doi: 10.1038/nature05453
Leto, K., Arancillo, M., Becker, E. B., Buffo, A., Chiang, C., Ding, B., et al. (2016). Consensus Paper: Cerebellar Development. Cerebellum 15, 789–828. doi: 10.1007/s12311-015-0724-2
Leto, K., Bartolini, A., and Rossi, F. (2008). Development of cerebellar GABAergic interneurons: origin and shaping of the "minibrain" local connections. Cerebellum 7, 523–529. doi: 10.1007/s12311-008-0079-z
Leto, K., Carletti, B., Williams, I. M., Magrassi, L., and Rossi, F. (2006). Different types of cerebellar GABAergic interneurons originate from a common pool of multipotent progenitor cells. J. Neurosci. 26, 11682–11694. doi: 10.1523/jneurosci.3656-06.2006
Li, Z. H., Li, B., Zhang, X. Y., and Zhu, J. N. (2024). Neuropeptides and their roles in the cerebellum. Int. J. Mol. Sci. 25:2332. doi: 10.3390/ijms25042332
Li, C., Saliba, N. B., Martin, H., Losurdo, N. A., Kolahdouzan, K., Siddiqui, R., et al. (2023). Purkinje cell dopaminergic inputs to astrocytes regulate cerebellar-dependent behavior. Nat. Commun. 14:1613. doi: 10.1038/s41467-023-37319-w
Litwin-Kumar, A., Harris, K. D., Axel, R., Sompolinsky, H., and Abbott, L. F. (2017). Optimal degrees of synaptic connectivity. Neuron 93, 1153–1164.e 1157. doi: 10.1016/j.neuron.2017.01.030
Ljungberg, L., Lang-Ouellette, D., Yang, A., Jayabal, S., Quilez, S., and Watt, A. J. (2016). Transient developmental Purkinje cell axonal torpedoes in healthy and ataxic mouse cerebellum. Front. Cell. Neurosci. 10:248. doi: 10.3389/fncel.2016.00248
Llano, I., and Gerschenfeld, H. M. (1993). Beta-adrenergic enhancement of inhibitory synaptic activity in rat cerebellar stellate and Purkinje cells. J. Physiol. 468, 201–224. doi: 10.1113/jphysiol.1993.sp019767
Longley, M., Ballard, J., Andres-Alonso, M., Varatharajah, R. C., Cuthbert, H., and Yeo, C. H. (2021). A patterned architecture of monoaminergic afferents in the cerebellar cortex: noradrenergic and serotonergic fibre distributions within lobules and parasagittal zones. Neuroscience 462, 106–121. doi: 10.1016/j.neuroscience.2020.09.001
Louis, E. D., Faust, P. L., Vonsattel, J. P., Honig, L. S., Rajput, A., Rajput, A., et al. (2009). Torpedoes in Parkinson's disease, Alzheimer's disease, essential tremor, and control brains. Mov. Disord. 24, 1600–1605. doi: 10.1002/mds.22567
Louis, E. D., Kuo, S. H., Vonsattel, J. P., and Faust, P. L. (2014). Torpedo formation and Purkinje cell loss: modeling their relationship in cerebellar disease. Cerebellum 13, 433–439. doi: 10.1007/s12311-014-0556-5
Magnus, G., Xing, J., Zhang, Y., and Han, V. Z. (2023). Diversity of cellular physiology and morphology of Purkinje cells in the adult zebrafish cerebellum. J. Comp. Neurol. 531, 461–485. doi: 10.1002/cne.25435
Mann-Metzer, P., and Yarom, Y. (1999). Electrotonic coupling interacts with intrinsic properties to generate synchronized activity in cerebellar networks of inhibitory interneurons. J. Neurosci. 19, 3298–3306. doi: 10.1523/JNEUROSCI.19-09-03298.1999
Maricich, S. M., and Herrup, K. (1999). Pax-2 expression defines a subset of GABAergic interneurons and their precursors in the developing murine cerebellum. J. Neurobiol. 5, 281–294.
Markwalter, K. H., Yang, Y., Holy, T. E., and Bonni, A. (2019). Sensorimotor coding of Vermal granule neurons in the developing mammalian cerebellum. J. Neurosci. 39, 6626–6643. doi: 10.1523/JNEUROSCI.0086-19.2019
Marr, D. (1969). A theory of cerebellar cortex. J. Physiol. 202, 437–470. doi: 10.1113/jphysiol.1969.sp008820
Masoli, S., Tognolina, M., Laforenza, U., Moccia, F., and D'Angelo, E. (2020). Parameter tuning differentiates granule cell subtypes enriching transmission properties at the cerebellum input stage. Commun Biol 3:222. doi: 10.1038/s42003-020-0953-x
Mathews, P. J., Lee, K. H., Peng, Z., Houser, C. R., and Otis, T. S. (2012). Effects of climbing fiber driven inhibition on Purkinje neuron spiking. J. Neurosci. 32, 17988–17997. doi: 10.1523/JNEUROSCI.3916-12.2012
McDonough, A., Elsen, G. E., Daza, R. M., Bachleda, A. R., Pizzo, D., Delle Torri, O. M., et al. (2020). Unipolar (dendritic) brush cells are morphologically complex and require Tbr2 for differentiation and migration. Front. Neurosci. 14:598548. doi: 10.3389/fnins.2020.598548
Mittmann, W., Koch, U., and Häusser, M. (2005). Feed-forward inhibition shapes the spike output of cerebellar Purkinje cells. J. Physiol. 563, 369–378. doi: 10.1113/jphysiol.2004.075028
Miyazaki, T., Yamasaki, M., Tanaka, K. F., and Watanabe, M. (2021). Compartmentalized input-output Organization of Lugaro Cells in the cerebellar cortex. Neuroscience 462, 89–105. doi: 10.1016/j.neuroscience.2020.05.026
Mugnaini, E., Sekerková, G., and Martina, M. (2011). The unipolar brush cell: a remarkable neuron finally receiving deserved attention. Brain Res. Rev. 66, 220–245. doi: 10.1016/j.brainresrev.2010.10.001
Nedelescu, H., and Abdelhack, M. (2013). Comparative morphology of dendritic arbors in populations of Purkinje cells in mouse sulcus and apex. Neural Plast. 2013:948587. doi: 10.1155/2013/948587
Nedelescu, H., Abdelhack, M., and Pritchard, A. T. (2018). Regional differences in Purkinje cell morphology in the cerebellar vermis of male mice. J. Neurosci. Res. 96, 1476–1489. doi: 10.1002/jnr.24206
Nelson, T. E., King, J. S., and Bishop, G. A. (1997). Distribution of tyrosine hydroxylase-immunoreactive afferents to the cerebellum differs between species. J. Comp. Neurol. 379, 443–454. doi: 10.1002/(sici)1096-9861(19970317)379:3<443::aid-cne9>3.0.co;2-3
Nguyen, T. M., Thomas, L. A., Rhoades, J. L., Ricchi, I., Yuan, X. C., Sheridan, A., et al. (2023). Structured cerebellar connectivity supports resilient pattern separation. Nature 613, 543–549. doi: 10.1038/s41586-022-05471-w
Nietz, A. K., Vaden, J. H., Coddington, L. T., Overstreet-Wadiche, L., and Wadiche, J. I. (2017). Non-synaptic signaling from cerebellar climbing fibers modulates Golgi cell activity. eLife 6:e29215. doi: 10.7554/eLife.29215
Nunzi, M. G., Shigemoto, R., and Mugnaini, E. (2002). Differential expression of calretinin and metabotropic glutamate receptor mGluR1alpha defines subsets of unipolar brush cells in mouse cerebellum. J. Comp. Neurol. 451, 189–199. doi: 10.1002/cne.10344
O’Donoghue, D. L., King, J. S., and Bishop, G. A. (1989). Physiological and anatomical studies of the interactions between Purkinje cells and basket cells in the cat's cerebellar cortex: evidence for a unitary relationship. J. Neurosci. 9, 2141–2150. doi: 10.1523/JNEUROSCI.09-06-02141.1989
Orduz, D., and Llano, I. (2007). Recurrent axon collaterals underlie facilitating synapses between cerebellar Purkinje cells. Proc. Natl. Acad. Sci. USA 104, 17831–17836. doi: 10.1073/pnas.0707489104
Osorno, T., Rudolph, S., Nguyen, T., Kozareva, V., Nadaf, N. M., Norton, A., et al. (2022). Candelabrum cells are ubiquitous cerebellar cortex interneurons with specialized circuit properties. Nat. Neurosci. 25, 702–713. doi: 10.1038/s41593-022-01057-x
Özçete, Ö., Banerjee, A., and Kaeser, P. S. (2024). Mechanisms of neuromodulatory volume transmission. Mol. Psychiatry. doi: 10.1038/s41380-024-02608-3
Palay, S. L., and Chan-Palay, V. (1974). Cerebellar cortex cytology and organization. Heidelberg: Springer-Verlag.
Panagopoulos, N. T., Papadopoulos, G. C., and Matsokis, N. A. (1991). Dopaminergic innervation and binding in the rat cerebellum. Neurosci. Lett. 130, 208–212. doi: 10.1016/0304-3940(91)90398-d
Park, T. J., Deng, S., Manna, S., Islam, A., Yu, H., Yuan, Y., et al. (2023). Complex oxides for brain-inspired computing: a review. Adv. Mater. 35:e2203352. doi: 10.1002/adma.202203352
Park, H., Kim, T., Kim, J., Yamamoto, Y., and Tanaka-Yamamoto, K. (2019). Inputs from sequentially developed parallel fibers are required for cerebellar organization. Cell Rep. 28, 2939–2954 e 2935. doi: 10.1016/j.celrep.2019.08.010
Park, H., Yamamoto, Y., and Tanaka-Yamamoto, K. (2021). Refinement of cerebellar network organization by extracellular signaling during development. Neuroscience 462, 44–55. doi: 10.1016/j.neuroscience.2020.05.036
Pereda, A. E. (2014). Electrical synapses and their functional interactions with chemical synapses. Nat. Rev. Neurosci. 15, 250–263. doi: 10.1038/nrn3708
Prestori, F., Mapelli, L., and D'Angelo, E. (2019). Diverse neuron properties and complex network dynamics in the cerebellar cortical inhibitory circuit. Front. Mol. Neurosci. 12:267. doi: 10.3389/fnmol.2019.00267
Rakic, P. (1972). Mode of cell migration to the superficial layers of fetal monkey neocortex. J. Comp. Neurol. 145, 61–83. doi: 10.1002/cne.901450105
Redondo, J., Kemp, K., Hares, K., Rice, C., Scolding, N., and Wilkins, A. (2015). Purkinje cell pathology and loss in multiple sclerosis cerebellum. Brain Pathol. 25, 692–700. doi: 10.1111/bpa.12230
Rhee, J. K., Park, H., Kim, T., Yamamoto, Y., and Tanaka-Yamamoto, K. (2021). Projection-dependent heterogeneity of cerebellar granule cell calcium responses. Mol. Brain 14:63. doi: 10.1186/s13041-021-00773-y
Rieubland, S., Roth, A., and Häusser, M. (2014). Structured connectivity in cerebellar inhibitory networks. Neuron 81, 913–929. doi: 10.1016/j.neuron.2013.12.029
Rosenberg, A. B., Roco, C. M., Muscat, R. A., Kuchina, A., Sample, P., Yao, Z., et al. (2018). Single-cell profiling of the developing mouse brain and spinal cord with split-pool barcoding. Science 360, 176–182. doi: 10.1126/science.aam8999
Russo, M. J., Yau, H. J., Nunzi, M. G., Mugnaini, E., and Martina, M. (2008). Dynamic metabotropic control of intrinsic firing in cerebellar unipolar brush cells. J. Neurophysiol. 100, 3351–3360. doi: 10.1152/jn.90533.2008
Scheibel, M. E., and Scheibel, A. B. (1954). Observations on the intracortical relations of the climbing fibers of the cerebellum; a Golgi study. J. Comp. Neurol. 101, 733–763. doi: 10.1002/cne.901010305
Schilling, K. (2013). “Specification and development of GABAergic interneurons” in Handbook of the cerebellum and cerebellar disorders. eds. M. Manto, D. Gruol, D. Schmahmann, N. Koibuchi, and R. Sillitoe (Cham: Springer), 207–235.
Schilling, K. (2024). Revisiting the development of cerebellar inhibitory interneurons in the light of single-cell genetic analyses. Histochem. Cell Biol. 161, 5–27. doi: 10.1007/s00418-023-02251-z
Schilling, K., Oberdick, J., Rossi, F., and Baader, S. L. (2008). Besides Purkinje cells and granule neurons: an appraisal of the cell biology of the interneurons of the cerebellar cortex. Histochem. Cell Biol. 130, 601–615. doi: 10.1007/s00418-008-0483-y
Schmidt, H., and Knösche, T. R. (2019). Action potential propagation and synchronisation in myelinated axons. PLoS Comput. Biol. 15:e1007004. doi: 10.1371/journal.pcbi.1007004
Schweighofer, N., Doya, K., and Kuroda, S. (2004). Cerebellar aminergic neuromodulation: towards a functional understanding. Brain Res. Brain Res. Rev. 44, 103–116. doi: 10.1016/j.brainresrev.2003.10.004
Sergaki, M. C., López-Ramos, J. C., Stagkourakis, S., Gruart, A., Broberger, C., Delgado-García, J. M., et al. (2017). Compromised survival of cerebellar molecular layer interneurons lacking GDNF receptors GFRalpha1 or RET impairs Normal cerebellar motor learning. Cell Rep. 19, 1977–1986. doi: 10.1016/j.celrep.2017.05.030
Shuster, S. A., Wagner, M. J., Pan-Doh, N., Ren, J., Grutzner, S. M., Beier, K. T., et al. (2021). The relationship between birth timing, circuit wiring, and physiological response properties of cerebellar granule cells. Proc. Natl. Acad. Sci. USA 118:e2101826118. doi: 10.1073/pnas.2101826118
Simat, M., Ambrosetti, L., Lardi-Studler, B., and Fritschy, J. M. (2007a). GABAergic synaptogenesis marks the onset of differentiation of basket and stellate cells in mouse cerebellum. Eur. J. Neurosci. 26, 2239–2256. doi: 10.1111/j.1460-9568.2007.05846.x
Simat, M., Parpan, F., and Fritschy, J. M. (2007b). Heterogeneity of glycinergic and gabaergic interneurons in the granule cell layer of mouse cerebellum. J. Comp. Neurol. 500, 71–83. doi: 10.1002/cne.21142
Snell, H. D., Vitenzon, A., Tara, E., Chen, C., Tindi, J., Jordan, B. A., et al. (2022). Mechanism of stress-induced attacks in an episodic neurologic disorder. Sci. Adv. 8:eabh2675. doi: 10.1126/sciadv.abh2675
Sotelo, C. (2015). Molecular layer interneurons of the cerebellum: developmental and morphological aspects. Cerebellum 14, 534–556. doi: 10.1007/s12311-015-0648-x
Sotelo, C., and Dusart, I. (2009). Intrinsic versus extrinsic determinants during the development of Purkinje cell dendrites. Neuroscience 162, 589–600. doi: 10.1016/j.neuroscience.2008.12.035
Stanley, A. T., Post, M. R., Lacefield, C., Sulzer, D., and Miniaci, M. C. (2023). Norepinephrine release in the cerebellum contributes to aversive learning. Nat. Commun. 14:4852. doi: 10.1038/s41467-023-40548-8
Straub, I., Witter, L., Eshra, A., Hoidis, M., Byczkowicz, N., Maas, S., et al. (2020). Gradients in the mammalian cerebellar cortex enable Fourier-like transformation and improve storing capacity. eLife 9:e51771. doi: 10.7554/eLife.51771
Sudarov, A., Turnbull, R. K., Kim, E. J., Lebel-Potter, M., Guillemot, F., and Joyner, A. L. (2011). Ascl1 genetics reveals insights into cerebellum local circuit assembly. J. Neurosci. 31, 11055–11069. doi: 10.1523/JNEUROSCI.0479-11.2011
Sugihara, I., Wu, H.-S., and Shinoda, Y. (1999). Morphology of single Olivocerebellar axons labeled with biotinylated dextran amine in the rat. J Comp Neurobiol 414, 131–148. doi: 10.1002/(SICI)1096-9861(19991115)414:2<131::AID-CNE1>3.0.CO;2-F
Sultan, F. (2000). Exploring a critical parameter of timing in the mouse cerebellar microcircuitry-the parallel fiber diameter. Neurosci. Lett. 280, 41–44. doi: 10.1016/S0304-3940(99)00984-2
Sultan, F., and Bower, J. M. (1998). Quantitative Golgi study of the rat cerebellar molecular layer interneurons using principal component analysis. J Comp Neurobiol 393, 353–373. doi: 10.1002/(SICI)1096-9861(19980413)393:3<353::AID-CNE7>3.0.CO;2-0
Szapiro, G., and Barbour, B. (2007). Multiple climbing fibers signal to molecular layer interneurons exclusively via glutamate spillover. Nat. Neurosci. 10, 735–742. doi: 10.1038/nn1907
Takada, M., Sugimoto, T., and Hattori, T. (1993). Tyrosine hydroxylase immunoreactivity in cerebellar Purkinje cells of the rat. Neurosci. Lett. 150, 61–64. doi: 10.1016/0304-3940(93)90108-w
Tam, W. Y., Wang, X., Cheng, A. S. K., and Cheung, K. K. (2021). In search of molecular markers for cerebellar neurons. Int. J. Mol. Sci. 22:1850. doi: 10.3390/ijms22041850
Telley, L., Cadilhac, C., Cioni, J. M., Saywell, V., Jahannault-Talignani, C., Huettl, R. E., et al. (2016). Dual function of NRP1 in axon guidance and subcellular target recognition in cerebellum. Neuron 91, 1276–1291. doi: 10.1016/j.neuron.2016.08.015
Tsutsumi, S., Yamazaki, M., Miyazaki, T., Watanabe, M., Sakimura, K., Kano, M., et al. (2015). Structure-function relationships between aldolase C/zebrin II expression and complex spike synchrony in the cerebellum. J. Neurosci. 35, 843–852. doi: 10.1523/JNEUROSCI.2170-14.2015
van der Heijden, M. E., and Sillitoe, R. V. (2021). Interactions between Purkinje cells and granule cells coordinate the development of functional cerebellar circuits. Neuroscience 462, 4–21. doi: 10.1016/j.neuroscience.2020.06.010
van Dorp, S., and De Zeeuw, C. I. (2014). Variable timing of synaptic transmission in cerebellar unipolar brush cells. Proc. Natl. Acad. Sci. USA 111, 5403–5408. doi: 10.1073/pnas.1314219111
van Dorp, S., and De Zeeuw, C. I. (2015). Forward signaling by unipolar brush cells in the mouse cerebellum. Cerebellum 14, 528–533. doi: 10.1007/s12311-015-0693-5
Vervaeke, K., Lorincz, A., Gleeson, P., Farinella, M., Nusser, Z., and Silver, R. A. (2010). Rapid desynchronization of an electrically coupled interneuron network with sparse excitatory synaptic input. Neuron 67, 435–451. doi: 10.1016/j.neuron.2010.06.028
Wadiche, J. I., and Jahr, C. E. (2001). Multivesicular release at climbing Fiber-Purkinje cell synapses. Neuron 32, 301–313. doi: 10.1016/S0896-6273(01)00488-3
Wagner, M. J., Kim, T. H., Savall, J., Schnitzer, M. J., and Luo, L. (2017). Cerebellar granule cells encode the expectation of reward. Nature 544, 96–100. doi: 10.1038/nature21726
Wang, W. X., and Lefebvre, J. L. (2022). Morphological pseudotime ordering and fate mapping reveal diversification of cerebellar inhibitory interneurons. Nat. Commun. 13:3433. doi: 10.1038/s41467-022-30977-2
Watt, A. J., Cuntz, H., Mori, M., Nusser, Z., Sjöström, P. J., and Häusser, M. (2009). Traveling waves in developing cerebellar cortex mediated by asymmetrical Purkinje cell connectivity. Nat. Neurosci. 12, 463–473. doi: 10.1038/nn.2285
Wefers, A. K., Haberlandt, C., Tekin, N. B., Fedorov, D. A., Timmermann, A., van der Want, J. J. L., et al. (2017). Synaptic input as a directional cue for migrating interneuron precursors. Development 144, 4125–4136. doi: 10.1242/dev.154096
Weisheit, G., Gliem, M., Endl, E., Pfeffer, P. L., Busslinger, M., and Schilling, K. (2006). Postnatal development of the murine cerebellar cortex: formation and early dispersal of basket, stellate and Golgi neurons. Eur. J. Neurosci. 24, 466–478. doi: 10.1111/j.1460-9568.2006.04915.x
Wilms, C. D., and Häusser, M. (2015). Reading out a spatiotemporal population code by imaging neighbouring parallel fibre axons in vivo. Nat. Commun. 6:6464. doi: 10.1038/ncomms7464
Witter, L., Rudolph, S., Pressler, R. T., Lahlaf, S. I., and Regehr, W. G. (2016). Purkinje cell collaterals enable output signals from the cerebellar cortex to feed Back to Purkinje cells and interneurons. Neuron 91, 312–319. doi: 10.1016/j.neuron.2016.05.037
Wu, D., Chen, Q., Chen, X., Han, F., Chen, Z., and Wang, Y. (2023). The blood-brain barrier: structure, regulation, and drug delivery. Signal Transduct. Target. Ther. 8:217. doi: 10.1038/s41392-023-01481-w
Wyatt, K. D., Tanapat, P., and Wang, S. S. (2005). Speed limits in the cerebellum: constraints from myelinated and unmyelinated parallel fibers. Eur. J. Neurosci. 21, 2285–2290. doi: 10.1111/j.1460-9568.2005.04053.x
Yamamoto, M., Kim, M., Imai, H., Itakura, Y., and Ohtsuki, G. (2019). Microglia-triggered plasticity of intrinsic excitability modulates psychomotor behaviors in acute cerebellar inflammation. Cell Rep. 28, 2923–2938.e8. doi: 10.1016/j.celrep.2019.07.078
Yamanaka, H., Yanagawa, Y., and Obata, K. (2004). Development of stellate and basket cells and their apoptosis in mouse cerebellar cortex. Neurosci. Res. 50, 13–22. doi: 10.1016/j.neures.2004.06.008
Yuzaki, M. (2004). The delta2 glutamate receptor: a key molecule controlling synaptic plasticity and structure in Purkinje cells. Cerebellum 3, 89–93. doi: 10.1080/14734220410028921
Zampini, V., Liu, J. K., Diana, M. A., Maldonado, P. P., Brunel, N., and Dieudonné, S. (2016). Mechanisms and functional roles of glutamatergic synapse diversity in a cerebellar circuit. eLife 5:e15872. doi: 10.7554/eLife.15872
Zeng, H., and Sanes, J. R. (2017). Neuronal cell-type classification: challenges, opportunities and the path forward. Nat. Rev. Neurosci. 18, 530–546. doi: 10.1038/nrn.2017.85
Zhou, J., Brown, A. M., Lackey, E. P., Arancillo, M., Lin, T., and Sillitoe, R. V. (2020). Purkinje cell neurotransmission patterns cerebellar basket cells into zonal modules defined by distinct pinceau sizes. eLife 9:e55569. doi: 10.7554/eLife.55569
Keywords: cerebellar cortex, neuronal circuits, heterogeneity, Purkinje cells, granule cells, molecular layer interneurons
Citation: Jun S, Park H, Kim M, Kang S, Kim T, Kim D, Yamamoto Y and Tanaka-Yamamoto K (2024) Increased understanding of complex neuronal circuits in the cerebellar cortex. Front. Cell. Neurosci. 18:1487362. doi: 10.3389/fncel.2024.1487362
Edited by:
Marylka Yoe Uusisaari, Okinawa Institute of Science and Technology Graduate University, JapanReviewed by:
Manish Shukla, Penn State Milton S. Hershey Medical Center, United StatesAlessandro Leparulo, University of Padua, Italy
Meike E. van der Heijden, Virginia Tech Carilion, United States
Copyright © 2024 Jun, Park, Kim, Kang, Kim, Kim, Yamamoto and Tanaka-Yamamoto. This is an open-access article distributed under the terms of the Creative Commons Attribution License (CC BY). The use, distribution or reproduction in other forums is permitted, provided the original author(s) and the copyright owner(s) are credited and that the original publication in this journal is cited, in accordance with accepted academic practice. No use, distribution or reproduction is permitted which does not comply with these terms.
*Correspondence: Yukio Yamamoto, eXVraW8ua2lzdEBnbWFpbC5jb20=; Keiko Tanaka-Yamamoto, a2Vpa295YW1hdEBnbWFpbC5jb20=