- 1Institute of Molecular Medicine, National Tsing Hua University, Hsinchu, Taiwan
- 2Department of Biomedical Engineering and Environmental Sciences, National Tsing Hua University, Hsinchu, Taiwan
- 3Department of Medical Science, National Tsing Hua University, Hsinchu, Taiwan
Sonogenetics is an emerging approach that harnesses ultrasound for the manipulation of genetically modified cells. The great penetrability of ultrasound waves enables the non-invasive application of external stimuli to deep tissues, particularly advantageous for brain stimulation. Genetically encoded ultrasound mediators, a set of proteins that respond to ultrasound-induced bio-effects, play a critical role in determining the effectiveness and applications of sonogenetics. In this context, we will provide an overview of these ultrasound-responsive mediators, delve into the molecular mechanisms governing their response to ultrasound stimulation, and summarize their applications in neuromodulation.
Introduction
The development of techniques for manipulating the activities of target neurons is crucial for understanding neuronal circuits in the brain and offering potential therapeutic applications for brain-related disorders. Optogenetics is a well-established approach that employs light to control target cells with artificially expressed photosensitive proteins (Pastrana, 2011). It has provided precise control over neuronal cells in vitro and in vivo, advancing the field of neuroscience and offering novel strategies for treating various diseases (Mazzitelli et al., 2022; Mirzayi et al., 2022; Kim et al., 2023). However, this powerful tool faces limitations due to the poor penetration of light, which restricts its applications in deep tissues. Significant efforts have been dedicated to achieving non-invasive neuromodulation. For example, thermogenetics, magnetogenetics, and sonogenetics combine various external stimuli with genetic techniques to non-invasively stimulate cells buried in deep tissues (Bernstein et al., 2012; Azadeh et al., 2021; Del Sol-Fernández et al., 2022). Among these stimuli, ultrasound (US) has received approval from the Food and Drug Administration for clinical applications in various human diseases, including Parkinson's diseases, dyskinesia, essential tremor, and tremor-dominant Parkinson's disease. Furthermore, the US has exhibited significant potential in the control of pain (Petterson et al., 2020), histotripsy (Vidal-Jove et al., 2022), and thermoablation (Jung et al., 2015). Due to its substantial clinical promise, sonogenetics has garnered growing attention. This review will focus on sonogenetics and provide an overview of genetically encoded mediators that sensitize cells to ultrasound (US) stimulation.
Ultrasound and sonogenetics
US are acoustic waves with frequencies above the upper limit of human hearing. US waves efficiently propagate several centimeters deep at a speed of approximately 1.5 km/sec within soft tissues. An annular array of multiple transducers can deliver US waves to defined small tissue volumes and is therefore referred to as focused US (FUS). Due to its exceptional penetrability and spatiotemporal resolution, FUS has been widely employed in diagnostic imaging. The penetrability and spatial resolution of FUS are determined by its frequency. Higher frequency US provides better resolution but limited penetrability, while lower frequency US offers relatively poor resolution but excellent penetrability (Boissenot et al., 2016). This trade-off explains why high-frequency and low-frequency FUS are typically used for superficial and deep tissue imaging, respectively. In addition to imaging, accumulating reports have shown that low-intensity FUS (averaging < 100 W/cm2 acoustic pressure over the pulse train) can induce a wide range of bio-effects, including heating, mechanical forces, and cavitation (Figure 1) (Collins and Mesce, 2022). While the thermal effect is known to be triggered by continuous FUS at high frequencies (Pinton et al., 2010; Rossmanna and Haemmerich, 2014), the parameters of FUS that specifically cause other bio-effects are not fully understood (Chu et al., 2022). This unpredictability may result from variations in acoustic properties or the expression profiles of endogenous US-sensing proteins in different tissues. Consequently, opposite effects on neuromodulation in distinct tissues can be triggered by similar US stimulation parameters. For example, low-intensity US (<17 W/cm2) has been found to produce contrasting neuromodulation effects in different brain regions (details summarized in Wang et al., 2020). One strategy to address this challenge is the overexpression of heterogeneous US-sensing proteins that are expected to be responsive to US-induced bio-effects in target cells, thereby ensuring the desired neuromodulation via FUS stimulation. Furthermore, genetic tools enable the precise expression of US-sensing proteins in specific cell types, providing target cells with enhanced US sensitivity compared to naïve cells. This opens up the possibility of using FUS to intendedly activate genetically modified target cells but not naïve cells within FUS-illuminated regions. This review will provide an overview of several genetically encoded US-sensing proteins that are responsive to different US-indued bio-effects and describe their applications in neuromodulation.
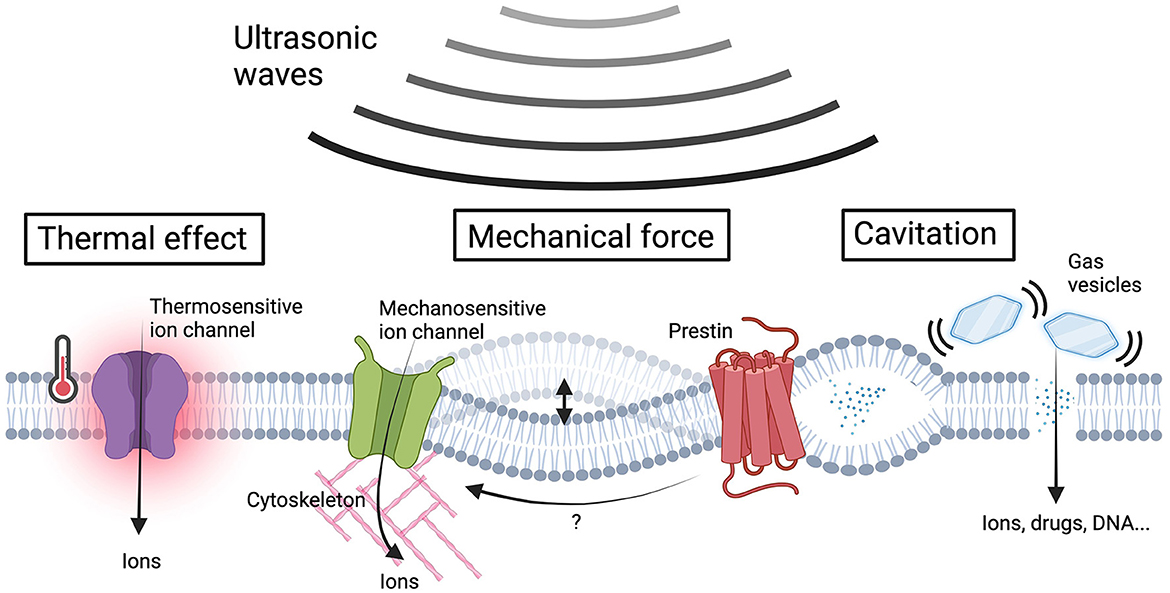
Figure 1. US-induced bio-effects and sensing proteins in sonogenetics. Ultrasonic waves give rise to a variety of bio-effects including thermal effects, membrane cavitation, and the generation of mechanical forces to cellular components. The US-induced heating activates thermosensitive ion channels, resulting in ion influx. The US energy could covert to mechanical forces that induce membrane deflection or intramembrane cavitation and subsequently activate mechanosensitive ion channels to facilitate ion influx. The increase in membrane tension induces transmembrane voltage fluctuations, which can be sensed by prestin, triggering downstream calcium influx through its electromobility. Gas vesicles vibrate in response to acoustic pressures, improving membrane permeability for the influx of ions and membrane impermeable molecules. The US-induced mechanical force effects can be further intensified by gas vesicles, promoting ion influx through mechanosensitive ion channels. The ion influx resulting from the aforementioned mechanisms alters the membrane potential, ultimately leading to neuromodulation (Figure created by BioRender.com).
Thermal effect-based sonogenetics
High-frequency US encounters significant absorption and scattering in tissues during propagation, resulting in the conversion of acoustic energy into heat. High-intensity FUS can rapidly elevate temperatures to the range of 55~80°C within the focal zone, leading to deleterious thermal effects for ablating diseased tissues (Bystritsky and Korb, 2015). By carefully tuning the US parameters, low-intensity FUS can induce localized noxious heat at approximately 42°C. Neuronal cells detect such temperature elevation through thermosensitive transient receptor potential (TRP) cationic channels (Zhu et al., 2019). Among the TRP family members, TRPV1 (Transient Receptor Potential Vanilloid 1) is a nonselective ligand-gated cation channel highly expressed in peripheral neurons and various brain regions (Fernandes et al., 2012; Pecze et al., 2016; Roet et al., 2019). TRPV1 can be activated by warm stimuli (~42°C) and regulate neuronal synaptic activities. Through viral overexpression of exogenous TRPV1 in the mouse cortex, several studies achieved successful activation of target neurons with genetic modification and modulation of animal locomotor behavior via FUS-induced thermal effects (Yang et al., 2021; Xu et al., 2023). It is noteworthy that optimized FUS stimulation (0.7 MPa) is necessary for specific modulation in genetically defined neurons, as naive mice are responsive to stronger FUS stimulation (1.1 MPa), possibly due to the contribution of endogenous thermosensors (Xu et al., 2023). The safety of this thermal modulation treatment can be secured by carefully tuning FUS parameters (Yang et al., 2021; Xu et al., 2023).
Mechanical force-based sonogenetics
Acoustic waves generate physical momentum when they encounter obstacles along their path, thus converting sound waves into mechanical forces that affect the given tissues. These obstacles can be attributed to biomolecules or materials with acoustic properties. The effects of mechanical forces on cells and tissues have been extensively studied, as they influence various biocomponents such as cell membranes (Vasan et al., 2022), cytoskeletons (Clark et al., 2008; Liang et al., 2013; Chuang and Chen, 2022), and the extracellular matrix (Chuang and Chen, 2022), subsequently altering the properties of connecting mechanosensors. The identification of numerous mechanosensitive ion channels has provided insights into the molecular mechanisms of cell mechanosensing (Lim et al., 2018). The recent research using high-speed imaging has provided direct evidence that US induces cell membrane deflection, resulting in membrane depolarization and neuron excitation (Vasan et al., 2022). Furthermore, Sorum et al. demonstrated that US activates a mechanosensitive channel known as TRAAK (TWIK-related arachidonic acid-activated K+ channel) (Sorum et al., 2021), and this activation depends on membrane structures. These studies support the hypothesis that US mechanically affects cell components, leading to the activation of mechanosensitive ion channels and downstream signaling pathways. Based on this working mechanism, numerous studies have identified several mechanosensitive ion channels that are involved in US sensing (Table 1).
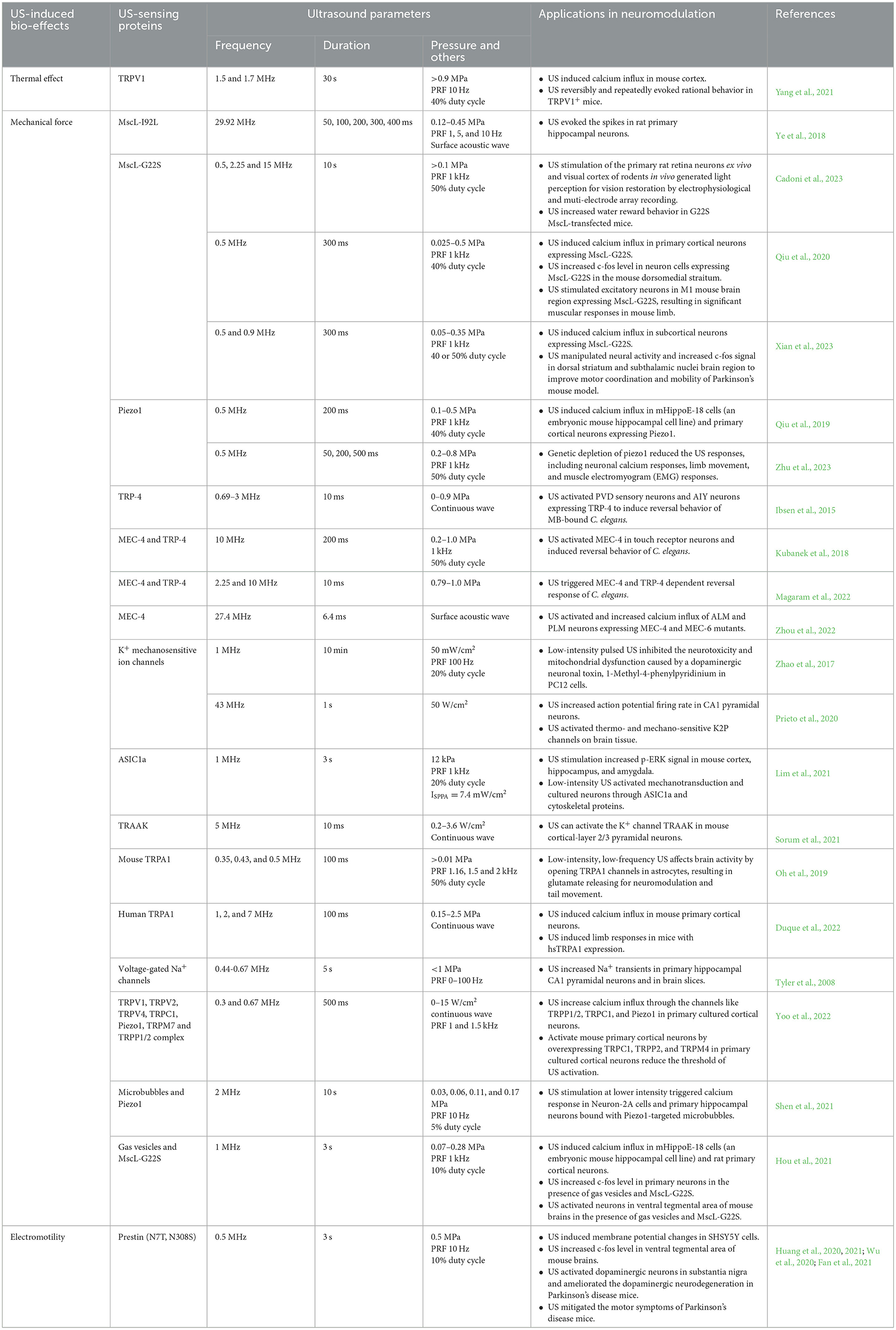
Table 1. Summary of genetically encoded US sensing proteins and their applications in neuromodulation.
US-sensitive mechanosensors have also shown their value in neuromodulation. The expression of TRP-4 in sensory neurons of C. elegans allows them to respond to US stimulation, resulting in reversed motor behavior in nematodes (Ibsen et al., 2015; Magaram et al., 2022). Yoo et al. confirmed that the overexpression of TRPC1, TRPP2, and TRPM4 in primary cultured cortical neurons makes them sensitive to US stimulation (Yoo et al., 2022). Oh et al. demonstrated that low-intensity and low-frequency US activates endogenous TRPA1 in mouse astrocytes, leading to the release of glutamate that activates NMDA receptors in neighboring neurons for neuromodulation (Oh et al., 2019). In a separate study, Duque et al. screened 191 TRP ion channels and found that human TRPA1 outperforms other candidates in response to pulsed high-frequency and high-intensity US (100 ms duration, 7 MHz, 2.5 MPa). Ectopically expressing human TRPA1 in mouse layer V motor cortical neurons allows them to respond to transcranial US stimulation, inducing limb responses in mice (Duque et al., 2022). The significant roles of endogenous Piezo1 in US sensing have been demonstrated through genetic depletion assays in mice (Li et al., 2019; Zhu et al., 2023). However, it remains unclear whether specific neuromodulation can be triggered in Piezo1-overexpressing neurons in vivo due to challenges associated with viral delivery of the Piezo1 gene. Ye et al. and Qiu et al. found that MscL-I92L and MscL-G22S, two MscL variants, exhibit greater mechanosensitivity compared to the wild-type, conferring US sensitivity to genetically modified neurons (Ye et al., 2018; Qiu et al., 2020). MscL-G22S-based sonogenetics enables specific activation in the dorsal striatum and inducible mouse locomotion (Xian et al., 2023). Stimulation of MscL-G22S-transfected neurons in the subthalamus by US successfully alleviates movement symptoms in parkinsonian mice (Xian et al., 2023). The majority of these studies activate mechanosensitive ion channels with US at frequencies lower than 3 MHz and peak pressures <1 MPa, suggesting that low-intensity and low-frequency US is sufficient for activating mechanosensitive ion channels. However, the activation of TRPA1 (Duque et al., 2022), TRAAK (Sorum et al., 2021), and Piezo1 (Prieto et al., 2018; Liao et al., 2021) with high-intensity (>1 MPa) and high-frequency (4.78~43 MHz) US stimulation still offers the flexibility of neuromodulation with better spatial resolution in superficial tissues. For example, MscL-G22S has been used to activate retinal and cortical neurons (Cadoni et al., 2023). By optimizing the parameters of high-frequency US (15 MHz), the study achieves excellent spatiotemporal resolution for proper visual restoration in superficial sites (Cadoni et al., 2023). In mechanical force-based sonogenetics, many efforts have been made to reduce unintended effects in naïve cells. Microbubbles (MBs, more details in next section) have been used in conjunction with mechanosensitive ion channels to robustly activate modified cells with low-intensity US, significantly reducing non-specific effects in naive cells (Heureaux et al., 2014; Ibsen et al., 2015; Huang et al., 2021). By using heterogeneously expressing mechanosensing proteins, sonogenetics can be employed to non-invasively modulate specific neurons, target circuits, and downstream behaviors, showing promise in the treatment of brain-related diseases.
Cavitation-based sonogenetics
MBs are particles with diameters ranging from 1 to 10 micrometers, enclosed within shells made of lipids, proteins, or polymers, and containing gaseous cores. The impedance mismatch between the gaseous core of MBs and the surrounding biological tissues causes MBs to expand and contract in response to the positive and negative phases of US waves (Wu and Nyborg, 2008; Kooiman et al., 2014). At low acoustic pressures, MBs undergo symmetrical linear oscillations, and their oscillation amplitudes are influenced by the driving acoustic pressures (Lentacker et al., 2014). As US acoustic pressure increases, the oscillations become asymmetric, promoting the expansion phase (Sboros, 2008; Lentacker et al., 2014; Gu et al., 2016), which can disturb membrane integrity (Qin et al., 2018). Additionally, the fluid micro-streaming generated by oscillating MBs can lead to membrane pore formation (Chen et al., 2016). The shear stress from microstreaming can enhance cell membrane permeability, rearrange the cytoskeleton, and promote nuclear contraction (Chen et al., 2022). Under high US acoustic pressure (several hundred kilopascals), MBs undergo asymmetric oscillations with large amplitudes, resulting in collapse and fragmentation, a phenomenon called inertial cavitation. When inertial cavitation occurs near the cell membrane, the pressure can temporarily disrupt the membrane and rupture the cytoskeleton (Fan et al., 2013). Changes in cell membrane permeability induced by US sonication with MBs can enhance ion influx, leading to membrane potential changes for neuromodulation. Shear-force-induced membrane tension variations can also activate mechanosensitive ion channels and their downstream effects.
Recently, gas vesicles (GVs) are the gas-filled protein nanostructures which naturally exist in many bacteria and archaea (Hill and Salmond, 2020) have emerged their values in US therapy. GVs have nanoscale dimensions, typically ranging from 45 to 250 nanometers in width and 100 to 600 nanometers in length (Pfeifer, 2012), and they possess a hollow structure filled with air. The shells of GVs primarily consist of hydrophobic proteins on the inner surface and hydrophilic proteins on the outer surface, rendering them highly physically stable since gases can permeate but not liquid water (Pfeifer, 2012; Shapiro et al., 2014). GVs serve as flotation devices for microorganisms in aqueous environments. The acoustic properties of GVs are remarkably similar to those of MBs, as they also have a gaseous core surrounded by liquid. As expected, it has demonstrated that US can induce cavitation effects via GVs (Hou et al., 2021). GVs produce strong ultrasonic contrast signals and have been employed as markers for various genes, chemicals, and cellular processes (Rabut et al., 2020; Wang et al., 2022). Furthermore, the oscillations of GVs have the potential to cause mechanical disturbances in their surroundings (Rabut et al., 2020). Due to these unique acoustic characteristics, GVs may amplify low-frequency US sonication, inducing oscillations and activating mechanosensitive ion channels to produce neuromodulation effects. With their nanoscale dimensions and gene-encodable structures, engineered genes can be delivered to extravascular vessels in the target area (Lakshmanan et al., 2016; Lu et al., 2018), enhancing the efficiency of targeted therapy. Additionally, GVs' shells can be chemically modified to load antibodies and biomolecule medications, making them a potentially powerful tool for neuromodulation therapeutic strategies for neural disorders. For example, a recent study combined low-intensity US sonication (1 MHz, burst width 200 μs, burst interval 2 ms, 0.28 MPa peak negative pressure) with GVs to enhance calcium influx and activate neuron activities (Hou et al., 2021). Mechanosensitive ion channels, especially the mechanosensitive MscL-G22S channel, played a critical role in inducing the stimulation effect in neurons (Hou et al., 2021). Biogenic GVs offer several advantages over MBs, as they can be precisely introduced to target tissues using genetic tools, whereas delivering MBs to extravascular tissues is challenging. Moreover, GVs exhibit better tissue penetrability due to their smaller size compared to MBs.
Electromobility-based sonogenetics
Many US sensing proteins were explored mainly dependent on their expected responses to US-indued bio-effects. However, few studies have adopted alternative strategies to address this matter. It is well-known that numerous species possess the ability to hear US and echolocate in their environment. Recently, prestin has gained attention as a promising candidate for contributing to high-frequency hearing in mammals due to the following findings. (1) Prestin is a membrane protein exclusively expressed in the cochlear outer hair cells, an essential auditory cell type crucial for high-frequency hearing in animals (Zheng et al., 2000; Dallos and Fakler, 2002; Rossiter et al., 2011). (2) In vitro characterization of prestin has demonstrated that transmembrane voltage fluctuations can induce changes in prestin's conformation, resulting in cell contraction and elongation, a phenomenon referred to as electromobility (Ludwig et al., 2001; Bavi et al., 2021). (3) Defects in prestin have been associated with hearing loss in both humans and mice (Liberman et al., 2002; Liu, 2003; Dallos, 2008; Walton et al., 2018). These findings raise a possibility that prestin may sense US-induced transmembrane voltage fluctuations and covert to mechanical effects through its electromobility. Evolutionary studies have shown that prestin is present in nearly all mammals, and its protein sequence is highly conserved across different species (Liu et al., 2010). By comparing prestin protein sequences between echolocating and non-echolocating species, Huang et al. observed two evolutionarily significant amino acid substitutions, N7T and N308S, which are frequently found in echolocating species but not in non-echolocating ones. Intriguingly, the introduction of N7T and N308S mutants to the mouse prestin gene intensifies its sensitivity to the US (Huang et al., 2020). Accumulated studies have found that prestin forms clusters on the cell membranes (Greeson et al., 2006; Mio et al., 2008). A conformational change of prestin in response to transmembrane voltage fluctuations causes vibration in the prestin clusters, resulting in membrane deformation (Dehghani-Ghahnaviyeh et al., 2022). Interestingly, prestin (N7T, N308S) forms more clusters on the cell membrane compared to wild-type prestin (Huang et al., 2020, 2021). High-speed imaging showed that vibration of prestin (N7T, N308S)-positive cluster occurs upon US stimulation. This vibration is dependent on the electromobility of prestin and plays a crucial role in downstream calcium influx (Huang et al., 2020). These findings assume that prestin clusters may act as molecular amplifiers, lowering the threshold of US-induced bio-effects. With this capability, specific neuronal activation in the ventral tegmental area can be achieved through US stimulation (Huang et al., 2020; Wu et al., 2020). Furthermore, Fan et al. successfully stimulated dopaminergic neuron activity to induce neurotrophic expression in Parkinson's disease mice, ameliorating their motor symptoms (Fan et al., 2021). Notably, mouse prestin (N7T, N308S) only responds to 500 kHz, not other US frequencies such as 80 kHz, which are often encountered in nature. This suggests that prestin (N7T, N308S)-dependent US response involves different mechanisms compared to high-frequency hearing in auditory organs. Prestin itself is not an ion channel and, therefore, requires assistance from other endogenous components, such as mechanosensitive ion channels, to transform prestin's electromobility into ion influx and subsequent changes in membrane potential. The expression profile of downstream molecules may play a pivotal role in determining the efficiency and effects of prestin-based sonogenetics. While further evidence is necessary to elucidate the detailed mechanisms involved, these studies have demonstrated the potential of using auditory sensing proteins in the field of sonogenetics.
Conclusion, challenges, and perspectives
Sonogenetics is an emerging approach enabling non-invasive modulation of target neurons and potential therapeutic applications. However, several issues need to be addressed to make sonogenetics more practical. First, an issue commonly encountered in sonogenetics is off-target effects. Multiple independent research teams have discovered that transcranial FUS, despite using frequencies beyond the range of hearing, can activate the peripheral auditory system as well as the motor cortex in different species (Foster and Wiederhold, 1978; Guo et al., 2018; Sato et al., 2018). To exclude indirect auditory response upon transcranial FUS stimulation, Mohammadjavadi et al. found that the smooth US rectangular waveform envelopes could directly induce motor responses without unintended peripheral auditory responses (Mohammadjavadi et al., 2019). Such parameters of FUS stimulation need to be considered in future animal studies. Second, the heterogeneous expression of US-sensing proteins in target cells makes them sensitive to US stimulation. However, many US-sensing proteins, such as various mechanosensitive ion channels, are ubiquitously present in different tissues, which raises the possibility of unexpected activation in naive cells. Orthogonality can be achieved by either utilizing sensing proteins from evolutionarily divergent species or engineering wild-type sensing proteins to boost their sensitivity to the US. For example, Huang et al. found that introducing N7T and N308S mutants to the wild-type prestin protein rendered it sensitive to the US (Huang et al., 2020). Ye et al. and Qiu et al. discovered that MscL-I92L and MscL-G22s, two prokaryotic MscL variants with higher mechanosensitivity compared to the wild-type, could sensitize neurons to FUS (Ye et al., 2018; Qiu et al., 2020). The introduction of GVs originally derived from cyanobacteria to target tissues could amplify FUS stimulation in cells bound with GVs (Hou et al., 2021). The optimized US stimulation for these engineering proteins could theoretically reduce the off-target effects in non-modified cells. Identifying more orthogonal US-sensing proteins from non-primate species or through protein engineering will enable specific modulation through FUS stimulation in clinical applications.
Although many efforts have been made to evaluate the correlation between FUS parameters and induced bio-effects (Collins and Mesce, 2022), most of them focus on only one type of tissue or cell. Tissues with different acoustic properties could exhibit distinct bio-effects upon FUS stimulation. In fact, FUS stimulation with almost the same parameters can induce opposite neuronal activities (Wang et al., 2020). The key factors determining the cellular sensitivity to the US and the resulting bio-effects need to be comprehensively explored. For example, factors such as cell stiffness (Bergman et al., 2021) and cytoskeleton composition (Noriega et al., 2013; Duque et al., 2022) are important for US sensitivity. The correlations among cells with different acoustic properties and FUS stimulation need to be comprehensively studied to better understand the mechanisms of sonogenetics. Besides the complexity of biocomponents, dependent on the design of transducers, various types of US wave propagation can be triggered, potentially resulting in distinct bioeffects on cells (Lo et al., 2021; Chu et al., 2022). Standardizing transducer designs and conducting comparative studies on different transducers are crucial for condition optimization in different physiological situations.
One advantage of optogenetics is multiplexing, offering the capability to use different light wavelengths to stimulate corresponding light-sensitive sensors and their related signaling pathways. For example, channelrhodopsin-2 and halorhodopsin, two photosensitive cation and anion channels, can be specifically activated by 470 and 589 nm light, respectively (Pastrana, 2011). Therefore, optogenetics can depolarize and hyperpolarize target neurons as needed. In addition to ion specificity, the identification of various photosensitive ion channels with different gating and opening durations allows for transient and sustained effects through pulsed light (Yizhar et al., 2011). Multiplexing in optogenetics provides flexibility in experimental design as well as clinical applications. Sonogenetics also has the potential for multiplexing, as a few studies have shown that different parameters of FUS can modulate distinct responses of US-sensing proteins. For instance, it has been demonstrated that GVs can be vibrated and collapsed by low-intensity and high-intensity US, respectively, opening up various applications (Bar-Zion et al., 2021; Wu et al., 2023). Huang et al. further showed that prestin (N7T, N308S) can be efficiently activated by 0.5 MHz FUS but not by other tested frequencies (Huang et al., 2020). The discovery of more US-sensitive proteins responsive to specific US parameters will greatly extend the toolkits of sonogenetics.
Author contributions
H-CW: Writing – original draft. T-NP: Writing – original draft. C-LK: Writing – original draft. C-KY: Writing – review & editing. Y-CL: Writing – original draft, Writing – review & editing.
Funding
The author(s) declare financial support was received for the research, authorship, and/or publication of this article. This work was supported by the Ministry of Science and Technology (MOST), Taiwan (MOST grant numbers 112-2636-B-007-008 and 112-2628-B-007-004 to Y-CL), and National Tsing Hua University (grant number 112Q2713E1 to Y-CL).
Conflict of interest
The authors declare that the research was conducted in the absence of any commercial or financial relationships that could be construed as a potential conflict of interest.
Publisher's note
All claims expressed in this article are solely those of the authors and do not necessarily represent those of their affiliated organizations, or those of the publisher, the editors and the reviewers. Any product that may be evaluated in this article, or claim that may be made by its manufacturer, is not guaranteed or endorsed by the publisher.
References
Azadeh, S. S., Lordifard, P., Soheilifar, M. H., Djavid, G. E., and Neghab, H. K. (2021). Ultrasound and sonogenetics: a new perspective for controlling cells with sound. Iran. J. Pharm. Res. 20, 151–160. doi: 10.22037/ijpr.2021.114868.15079
Bar-Zion, A., Nourmahnad, A., Mittelstein, D. R., Shivaei, S., Yoo, S., Buss, M. T., et al. (2021). Acoustically triggered mechanotherapy using genetically encoded gas vesicles. Nat. Nanotechnol. 16, 1403–1412. doi: 10.1038/s41565-021-00971-8
Bavi, N., Clark, M. D., Contreras, G. F., Shen, R., Reddy, B. G., Milewski, W., et al. (2021). Prestin's conformational cycle underlies outer hair cell electromotility. Nature 600, 1–11. doi: 10.1038/s41586-021-04152-4
Bergman, E., Goldbart, R., Traitel, T., Amar-Lewis, E., Zorea, J., Yegodayev, K., et al. (2021). Cell stiffness predicts cancer cell sensitivity to ultrasound as a selective superficial cancer therapy. Bioeng Transl. Med. 6:e10226. doi: 10.1002/btm2.10226
Bernstein, J. G., Garrity, P. A., and Boyden, E. S. (2012). Optogenetics and thermogenetics: technologies for controlling the activity of targeted cells within intact neural circuits. Curr. Opin. Neurobiol. 22, 61–71. doi: 10.1016/j.conb.2011.10.023
Boissenot, T., Bordat, A., Fattal, E., and Tsapis, N. (2016). Ultrasound-triggered drug delivery for cancer treatment using drug delivery systems: from theoretical considerations to practical applications. J. Control. Release 241, 144–163. doi: 10.1016/j.jconrel.2016.09.026
Bystritsky, A., and Korb, A. S. (2015). A review of low-intensity transcranial focused ultrasound for clinical applications. Curr. Behav. Neurosci. Rep. 2, 60–66. doi: 10.1007/s40473-015-0039-0
Cadoni, S., Demené, C., Alcala, I., Provansal, M., Nguyen, D., Nelidova, D., et al. (2023). Ectopic expression of a mechanosensitive channel confers spatiotemporal resolution to ultrasound stimulations of neurons for visual restoration. Nat. Nanotechnol. 18, 667–676. doi: 10.1038/s41565-023-01359-6
Chen, C., Gu, Y., Tu, J., Guo, X., and Zhang, D. (2016). Microbubble oscillating in a microvessel filled with viscous fluid: a finite element modeling study. Ultrasonics 66, 54–64. doi: 10.1016/j.ultras.2015.11.010
Chen, S., Nazeri, A., Baek, H., Ye, D., Yang, Y., Yuan, J., et al. (2022). A review of bioeffects induced by focused ultrasound combined with microbubbles on the neurovascular unit. J. Cereb. Blood Flow Metab. 42, 3–26. doi: 10.1177/0271678X211046129
Chu, Y. -C., Lim, J., Chien, A., Chen, C. -C., and Wang, J. -L. (2022). Activation of mechanosensitive ion channels by ultrasound. Ultrasound Med. Biol. 48, 1981–1994. doi: 10.1016/j.ultrasmedbio.2022.06.008
Chuang, Y. -C., and Chen, C. -C. (2022). Force from filaments: the role of the cytoskeleton and extracellular matrix in the gating of mechanosensitive channels. Front. Cell Dev. Biol. 10:886048. doi: 10.3389/fcell.2022.886048
Clark, K., Middelbeek, J., and van Leeuwen, F. N. (2008). Interplay between TRP channels and the cytoskeleton in health and disease. Eur. J. Cell Biol. 87, 631–640. doi: 10.1016/j.ejcb.2008.01.009
Collins, M. N., and Mesce, K. A. (2022). A review of the bioeffects of low-intensity focused ultrasound and the benefits of a cellular approach. Front. Physiol. 13:1047324. doi: 10.3389/fphys.2022.1047324
Dallos, P. (2008). Cochlear amplification, outer hair cells and prestin. Curr. Opin. Neurobiol. 18, 370–376. doi: 10.1016/j.conb.2008.08.016
Dallos, P., and Fakler, B. (2002). Prestin, a new type of motor protein. Nat. Rev. Mol. Cell Biol. 3, 104–111. doi: 10.1038/nrm730
Dehghani-Ghahnaviyeh, S., Zhao, Z., and Tajkhorshid, E. (2022). Lipid-mediated prestin organization in outer hair cell membranes and its implications in sound amplification. Nat. Commun. 13, 6877. doi: 10.1038/s41467-022-34596-9
Del Sol-Fernández, S., Martínez-Vicente, P., Gomollón-Zueco, P., Castro-Hinojosa, C., Gutiérrez, L., Fratila, R. M., et al. (2022). Magnetogenetics: remote activation of cellular functions triggered by magnetic switches. Nanoscale 14, 2091–2118. doi: 10.1039/D1NR06303K
Duque, M., Lee-Kubli, C. A., Tufail, Y., Magaram, U., Patel, J., Chakraborty, A., et al. (2022). Sonogenetic control of mammalian cells using exogenous transient receptor potential A1 channels. Nat. Commun. 13, 600. doi: 10.1038/s41467-022-28205-y
Fan, C. H., Wei, K. C., Chiu, N. H., Liao, E. C., Wang, H. C., Wu, R. Y., et al. (2021). Sonogenetic-based neuromodulation for the amelioration of Parkinson's disease. Nano Lett. 21, 5967–5976. doi: 10.1021/acs.nanolett.1c00886
Fan, Z., Sun, Y., Chen, D., Tay, D., Chen, W., Deng, C. X., et al. (2013). Acoustic tweezing cytometry for live-cell subcellular modulation of intracellular cytoskeleton contractility. Sci. Rep. 3:2176. doi: 10.1038/srep02176
Fernandes, E. S., Fernandes, M. A., and Keeble, J. E. (2012). The functions of TRPA1 and TRPV1: moving away from sensory nerves. Br. J. Pharmacol. 166, 510–521. doi: 10.1111/j.1476-5381.2012.01851.x
Foster, K. R., and Wiederhold, M. L. (1978). Auditory responses in cats produced by pulsed ultrasound. J. Acoust. Soc. Am. 63, 1199–1205. doi: 10.1121/1.381829
Greeson, J. N., Organ, L. E., Pereira, F. A., and Raphael, R. M. (2006). Assessment of prestin self-association using fluorescence resonance energy transfer. Brain Res. 1091, 140–150. doi: 10.1016/j.brainres.2006.02.084
Gu, Y., Chen, C., Tu, J., Guo, X., Wu, H., and Zhang, D. (2016). Harmonic responses and cavitation activity of encapsulated microbubbles coupled with magnetic nanoparticles. Ultrason. Sonochem. 29, 309–316. doi: 10.1016/j.ultsonch.2015.10.006
Guo, H., Hamilton, M., Offutt, S. J., Gloeckner, C. D., Li, T., Kim, Y., et al. (2018). Ultrasound produces extensive brain activation via a cochlear pathway. Neuron 98, 1020–1030.e4. doi: 10.1016/j.neuron.2018.04.036
Heureaux, J., Chen, D., Murray, V. L., Deng, C. X., and Liu, A. P. (2014). Activation of a bacterial mechanosensitive channel in mammalian cells by cytoskeletal stress. Cell. Mol. Bioeng. 7, 307–319. doi: 10.1007/s12195-014-0337-8
Hill, A. M., and Salmond, G. P. C. (2020). Microbial gas vesicles as nanotechnology tools: exploiting intracellular organelles for translational utility in biotechnology, medicine and the environment. Microbiol. 166, 501–509. doi: 10.1099/mic.0.000912
Hou, X., Qiu, Z., Xian, Q., Kala, S., Jing, J., Wong, K. F., et al. (2021). Precise ultrasound neuromodulation in a deep brain region using nano gas vesicles as actuators. Adv. Sci. 8:e2101934. doi: 10.1002/advs.202101934
Huang, Y. S., Fan, C. H., Hsu, N., Chiu, N. H., Wu, C. Y., Chang, C. Y., et al. (2020). Sonogenetic modulation of cellular activities using an engineered auditory-sensing protein. Nano Lett. 20, 1089–1100. doi: 10.1021/acs.nanolett.9b04373
Huang, Y. S., Fan, C. H., Yang, W. T., Yeh, C. K., and Lin, Y. C. (2021). Sonogenetic modulation of cellular activities in mammalian cells. Methods Mol. Biol. 2312, 109–124. doi: 10.1007/978-1-0716-1441-9_7
Ibsen, S., Tong, A., Schutt, C., Esener, S., and Chalasani, S. H. (2015). Sonogenetics is a non-invasive approach to activating neurons in Caenorhabditis elegans. Nat. Commun. 6, 1–12. doi: 10.1038/ncomms9264
Jung, H. H., Kim, S. J., Roh, D., Chang, J. G., Chang, W. S., Kweon, E. J., et al. (2015). Bilateral thermal capsulotomy with MR-guided focused ultrasound for patients with treatment-refractory obsessive-compulsive disorder: a proof-of-concept study. Mol. Psychiatry 20, 1205–1211. doi: 10.1038/mp.2014.154
Kim, M. S., Ra, E. A., Kweon, S. H., Seo, B. A., Ko, H. S., Oh, Y., et al. (2023). Advanced human iPSC-based preclinical model for Parkinson's disease with optogenetic alpha-synuclein aggregation. Cell Stem Cell 30, 973–986.e11. doi: 10.1016/j.stem.2023.05.015
Kooiman, K., Vos, H. J., Versluis, M., and de Jong, N. (2014). Acoustic behavior of microbubbles and implications for drug delivery. Adv. Drug Deliv. Rev. 72, 28–48. doi: 10.1016/j.addr.2014.03.003
Kubanek, J., Shukla, P., Das, A., Baccus, S. A., and Goodman, M. B. (2018). Ultrasound elicits behavioral responses through mechanical effects on neurons and ion channels in a simple nervous system. J. Neurosci. 38, 3081–3091. doi: 10.1523/JNEUROSCI.1458-17.2018
Lakshmanan, A., Farhadi, A., Nety, S. P., Lee-Gosselin, A., Bourdeau, R. W., Maresca, D., et al. (2016). Molecular engineering of acoustic protein nanostructures. ACS Nano 10, 7314–7322. doi: 10.1021/acsnano.6b03364
Lentacker, I., De Cock, I., Deckers, R., De Smedt, S. C., and Moonen, C. T. W. (2014). Understanding ultrasound induced sonoporation: definitions and underlying mechanisms. Adv. Drug Deliv. Rev. 72, 49–64. doi: 10.1016/j.addr.2013.11.008
Li, G., Qiu, W., Zhang, Z., Jiang, Q., Su, M., Cai, R., et al. (2019). Noninvasive ultrasonic neuromodulation in freely moving mice. IEEE Trans. Biomed. Eng. 66, 217–224. doi: 10.1109/TBME.2018.2821201
Liang, X., Madrid, J., Gärtner, R., Verbavatz, J. M., Schiklenk, C., Wilsch-Bräuninger, M., et al. (2013). A NOMPC-dependent membrane-microtubule connector is a candidate for the gating spring in fly mechanoreceptors. Curr. Biol. 23, 755–763. doi: 10.1016/j.cub.2013.03.065
Liao, D., Hsiao, M. Y., Xiang, G., and Zhong, P. (2021). Optimal pulse length of insonification for Piezo1 activation and intracellular calcium response. Sci. Rep. 11, 709. doi: 10.1038/s41598-020-78553-2
Liberman, M. C., Gao, J., He, D. Z. Z., Wu, X., Jia, S., and Zuo, J. (2002). Prestin is required for electromotility of the outer hair cell and for the cochlear amplifier. Nature 419, 300–304. doi: 10.1038/nature01059
Lim, C.-G., Jang, J., and Kim, C. (2018). Cellular machinery for sensing mechanical force. BMB Rep. 51, 623–629. doi: 10.5483/BMBRep.2018.51.12.237
Lim, J., Tai, H. -H., Liao, W. -H., Chu, Y. -C., Hao, C. -M., Huang, Y. -C., et al. (2021). ASIC1a is required for neuronal activation via low-intensity ultrasound stimulation in mouse brain. Elife 10, e61660. doi: 10.7554/eLife.61660.sa2
Liu, X. Z. (2003). Prestin, a cochlear motor protein, is defective in non-syndromic hearing loss. Hum. Mol. Genet. 12, 1155–1162. doi: 10.1093/hmg/ddg127
Liu, Y., Cotton, J. A., Shen, B., Han, X., Rossiter, S. J., and Zhang, S. (2010). Convergent sequence evolution between echolocating bats and dolphins. Curr. Biol. 20, R53–R54. doi: 10.1016/j.cub.2009.11.058
Lo, W. C., Fan, C. H., Ho, Y. J., Lin, C. W., and Yeh, C. K. (2021). Tornado-inspired acoustic vortex tweezer for trapping and manipulating microbubbles. Proc. Natl. Acad. Sci. USA. 118:e2023188118. doi: 10.1073/pnas.2023188118
Lu, G. J., Farhadi, A., Szablowski, J. O., Lee-Gosselin, A., Barnes, S. R., Lakshmanan, A., et al. (2018). Acoustically modulated magnetic resonance imaging of gas-filled protein nanostructures. Nat. Mater. 17, 456–463. doi: 10.1038/s41563-018-0023-7
Ludwig, J., Oliver, D., Frank, G., Klöcker, N., Gummer, A. W., Fakler, B., et al. (2001). Reciprocal electromechanical properties of rat prestin: the motor molecule from rat outer hair cells. Proc. Natl. Acad. Sci. USA. 98, 4178–4183. doi: 10.1073/pnas.071613498
Magaram, U., Weiss, C., Vasan, A., Reddy, K. C., Friend, J., and Chalasani, S. H. (2022). Two pathways are required for ultrasound-evoked behavioral changes in Caenorhabditis elegans. PLoS ONE 17:e0267698. doi: 10.1371/journal.pone.0267698
Mazzitelli, M., Yakhnitsa, V., Neugebauer, B., and Neugebauer, V. (2022). Optogenetic manipulations of CeA-CRF neurons modulate pain- and anxiety-like behaviors in neuropathic pain and control rats. Neuropharmacology 210:109031. doi: 10.1016/j.neuropharm.2022.109031
Mio, K., Kubo, Y., Ogura, T., Yamamoto, T., Arisaka, F., and Sato, C. (2008). The motor protein prestin is a bullet-shaped molecule with inner cavities. J. Biol. Chem. 283, 1137–1145. doi: 10.1074/jbc.M702681200
Mirzayi, P., Shobeiri, P., Kalantari, A., Perry, G., and Rezaei, N. (2022). Optogenetics: implications for Alzheimer's disease research and therapy. Mol. Brain 15, 20. doi: 10.1186/s13041-022-00905-y
Mohammadjavadi, M., Ye, P. P., Xia, A., Brown, J., Popelka, G., and Pauly, K. B. (2019). Elimination of peripheral auditory pathway activation does not affect motor responses from ultrasound neuromodulation. Brain Stimul. 12, 901–910. doi: 10.1016/j.brs.2019.03.005
Noriega, S., Hasanova, G., and Subramanian, A. (2013). The effect of ultrasound stimulation on the cytoskeletal organization of chondrocytes seeded in three-dimensional matrices. Cells Tissues Organs 197, 14–26. doi: 10.1159/000339772
Oh, S. J., Lee, J. M., Kim, H. B., Lee, J., Han, S., Bae, J. Y., et al. (2019). Ultrasonic neuromodulation via astrocytic TRPA1. Curr. Biol. 29, 3386–3401.e8. doi: 10.1016/j.cub.2019.08.021
Pastrana, E. (2011). Optogenetics: controlling cell function with light. Nat. Methods 8, 24–25. doi: 10.1038/nmeth.f.323
Pecze, L., Blum, W., Henzi, T., and Schwaller, B. (2016). Endogenous TRPV1 stimulation leads to the activation of the inositol phospholipid pathway necessary for sustained Ca2+ oscillations. Biochim. Biophys. Acta. 1863, 2905–2915. doi: 10.1016/j.bbamcr.2016.09.013
Petterson, S., Plancher, K., Klyve, D., Draper, D., and Ortiz, R. (2020). Low-intensity continuous ultrasound for the symptomatic treatment of upper shoulder and neck pain: a randomized, double-blind placebo-controlled clinical trial. J. Pain Res. 13, 1277–1287. doi: 10.2147/JPR.S247463
Pfeifer, F. (2012). Distribution, formation and regulation of gas vesicles. Nat. Rev. Microbiol. 10, 705–715. doi: 10.1038/nrmicro2834
Pinton, G., Pernot, M., Bossy, E., Aubry, J.-F., Muller, M., and Tanter, M. (2010). “Mechanisms of attenuation and heating dissipation of ultrasound in the skull bone: Comparison between simulation models and experiments,” in 2010 IEEE International Ultrasonics Symposium (San Diego, CA: IEEE), 225–228. doi: 10.1109/ULTSYM.2010.5935461
Prieto, M. L., Firouzi, K., Khuri-Yakub, B. T., Madison, D. V., and Maduke, M. (2020). Spike frequency-dependent inhibition and excitation of neural activity by high-frequency ultrasound. J. Gen. Physiol. 152:e202012672. doi: 10.1085/jgp.202012672
Prieto, M. L., Firouzi, K., Khuri-Yakub, B. T., and Maduke, M. (2018). Activation of piezo1 but not NaV1.2 channels by ultrasound at 43 MHz. Ultrasound Med. Biol. 44, 1217–1232. doi: 10.1016/j.ultrasmedbio.2017.12.020
Qin, P., Han, T., Yu, A. C. H., and Xu, L. (2018). Mechanistic understanding the bioeffects of ultrasound-driven microbubbles to enhance macromolecule delivery. J. Control. Release 272, 169–181. doi: 10.1016/j.jconrel.2018.01.001
Qiu, Z., Guo, J., Kala, S., Zhu, J., Xian, Q., Qiu, W., et al. (2019). The mechanosensitive ion channel piezo1 significantly mediates in vitro ultrasonic stimulation of neurons. iScience 21, 448–457. doi: 10.1016/j.isci.2019.10.037
Qiu, Z., Kala, S., Guo, J., Xian, Q., Zhu, J., Zhu, T., et al. (2020). Targeted neurostimulation in mouse brains with non-invasive ultrasound. Cell Rep. 32:108033. doi: 10.1016/j.celrep.2020.108033
Rabut, C., Yoo, S., Hurt, R. C., Jin, Z., Li, H., Guo, H., et al. (2020). Ultrasound technologies for imaging and modulating neural activity. Neuron 108, 93–110. doi: 10.1016/j.neuron.2020.09.003
Roet, M., Jansen, A., Hoogland, G., Temel, Y., and Jahanshahi, A. (2019). Endogenous TRPV1 expression in the human cingulate- and medial frontal gyrus. Brain Res. Bull. 152, 184–190. doi: 10.1016/j.brainresbull.2019.07.018
Rossiter, S. J., Zhang, S., and Liu, Y. (2011). Prestin and high frequency hearing in mammals. Commun. Integr. Biol. 4, 236–239. doi: 10.4161/cib.4.2.14647
Rossmanna, C., and Haemmerich, D. (2014). Review of temperature dependence of thermal properties, dielectric properties, and perfusion of biological tissues at hyperthermic and ablation temperatures. Crit. Rev. Biomed. Eng. 42, 467–492. doi: 10.1615/CritRevBiomedEng.2015012486
Sato, T., Shapiro, M. G., and Tsao, D. Y. (2018). Ultrasonic neuromodulation causes widespread cortical activation via an indirect auditory mechanism. Neuron 98, 1031–1041.e5. doi: 10.1016/j.neuron.2018.05.009
Sboros, V. (2008). Response of contrast agents to ultrasound. Adv. Drug Deliv. Rev. 60, 1117–1136. doi: 10.1016/j.addr.2008.03.011
Shapiro, M. G., Goodwill, P. W., Neogy, A., Yin, M., Foster, F. S., Schaffer, D. V., et al. (2014). Biogenic gas nanostructures as ultrasonic molecular reporters. Nat. Nanotechnol. 9, 311–316. doi: 10.1038/nnano.2014.32
Shen, X., Song, Z., Xu, E., Zhou, J., and Yan, F. (2021). Sensitization of nerve cells to ultrasound stimulation through Piezo1-targeted microbubbles. Ultrason. Sonochem. 73, 105494. doi: 10.1016/j.ultsonch.2021.105494
Sorum, B., Rietmeijer, R. A., Gopakumar, K., Adesnik, H., and Brohawn, S. G. (2021). Ultrasound activates mechanosensitive TRAAK K+ channels through the lipid membrane. Proc. Nat. Acad. Sci. 118:e2006980118. doi: 10.1073/pnas.2006980118
Tyler, W. J., Tufail, Y., Finsterwald, M., Tauchmann, M. L., Olson, E. J., and Majestic, C. (2008). Remote excitation of neuronal circuits using low-intensity, low-frequency ultrasound. PLoS ONE 3:e3511. doi: 10.1371/journal.pone.0003511
Vasan, A., Orosco, J., Magaram, U., Duque, M., Weiss, C., Tufail, Y., et al. (2022). Ultrasound mediated cellular deflection results in cellular depolarization. Adv. Sci. 9:e2101950. doi: 10.1002/advs.202101950
Vidal-Jove, J., Serres, X., Vlaisavljevich, E., Cannata, J., Duryea, A., Miller, R., et al. (2022). First-in-man histotripsy of hepatic tumors: the THERESA trial, a feasibility study. Int. J. Hyperthermia 39, 1115–1123. doi: 10.1080/02656736.2022.2112309
Walton, J. P., Dziorny, A. C., Vasilyeva, O. N., and Luebke, A. E. (2018). Loss of the cochlear amplifier prestin reduces temporal processing efficacy in the central auditory system. Front. Cell. Neurosci. 12:291. doi: 10.3389/fncel.2018.00291
Wang, R., Wang, L., Chen, Y., Xie, Y., He, M., Zhu, Y., et al. (2022). Biogenic gas vesicles for ultrasound imaging and targeted therapeutics. Curr. Med. Chem. 29, 1316–1330. doi: 10.2174/0929867328666210705145642
Wang, S., Meng, W., Ren, Z., Li, B., Zhu, T., Chen, H., et al. (2020). Ultrasonic neuromodulation and sonogenetics: a new era for neural modulation. Front. Physiol. 11:787. doi: 10.3389/fphys.2020.00787
Wu, C. Y., Fan, C. H., Chiu, N. H., Ho, Y. J., Lin, Y. C., and Yeh, C. K. (2020). Targeted delivery of engineered auditory sensing protein for ultrasound neuromodulation in the brain. Theranostics 10, 3546–3561. doi: 10.7150/thno.39786
Wu, D., Baresch, D., Cook, C., Ma, Z., Duan, M., Malounda, D., et al. (2023). Biomolecular actuators for genetically selective acoustic manipulation of cells. Sci. Adv. 9:e add9186. doi: 10.1126/sciadv.add9186
Wu, J., and Nyborg, W. L. (2008). Ultrasound, cavitation bubbles and their interaction with cells. Adv. Drug Deliv. Rev. 60, 1103–1116. doi: 10.1016/j.addr.2008.03.009
Xian, Q., Qiu, Z., Murugappan, S., Kala, S., Wong, K. F., Li, D., et al. (2023). Modulation of deep neural circuits with sonogenetics. Proc. Natl. Acad. Sci. USA. 120:e2220575120. doi: 10.1073/pnas.2220575120
Xu, K., Yang, Y., Hu, Z., Yue, Y., Gong, Y., Cui, J., et al. (2023). TRPV1-mediated sonogenetic neuromodulation of motor cortex in freely moving mice. J. Neural Eng. 20, 016055. doi: 10.1088/1741-2552/acbba0
Yang, Y., Pacia, C. P., Ye, D., Zhu, L., Baek, H., Yue, Y., et al. (2021). Sonothermogenetics for noninvasive and cell-type specific deep brain neuromodulation. Brain Stimul. 14, 790–800. doi: 10.1016/j.brs.2021.04.021
Ye, J., Tang, S., Meng, L., Li, X., Wen, X., Chen, S., et al. (2018). Ultrasonic control of neural activity through activation of the mechanosensitive channel MscL. Nano Lett. 18, 4148–4155. doi: 10.1021/acs.nanolett.8b00935
Yizhar, O., Fenno, L. E., Davidson, T. J., Mogri, M., and Deisseroth, K. (2011). Optogenetics in neural systems. Neuron 71, 9–34. doi: 10.1016/j.neuron.2011.06.004
Yoo, S., Mittelstein, D. R., Hurt, R. C., Lacroix, J., and Shapiro, M. G. (2022). Focused ultrasound excites cortical neurons via mechanosensitive calcium accumulation and ion channel amplification. Nat. Commun. 13, 493. doi: 10.1038/s41467-022-28040-1
Zhao, L., Feng, Y., Shi, A., Zhang, L., Guo, S., and Wan, M. (2017). Neuroprotective effect of low-intensity pulsed ultrasound against MPP+-induced neurotoxicity in PC12 cells: involvement of K2P channels and stretch-activated ion channels. Ultrasound Med. Biol. 43, 1986–1999. doi: 10.1016/j.ultrasmedbio.2017.04.020
Zheng, J., Shen, W., He, D. Z. Z., Long, K. B., Madison, L. D., and Dallos, P. (2000). Prestin is the motor protein of cochlear outer hair cells. Nature 405, 149–155. doi: 10.1038/35012009
Zhou, W., Wang, X., Wang, K., Farooq, U., Kang, L., Niu, L., et al. (2022). Ultrasound activation of mechanosensory ion channels in Caenorhabditis Elegans. IEEE Trans. Ultrason. Ferroelectr. Freq. Control 69, 473–479. doi: 10.1109/TUFFC.2021.3120750
Zhu, J., Xian, Q., Hou, X., Wong, K. F., Zhu, T., Chen, Z., et al. (2023). The mechanosensitive ion channel piezo1 contributes to ultrasound neuromodulation. Proc. Natl. Acad. Sci. USA. 120:e2300291120. doi: 10.1073/pnas.2300291120
Keywords: ultrasound, sonogenetics, gas vesicles, prestin, mechanosensitive ion channels
Citation: Wang H-C, Phan T-N, Kao C-L, Yeh C-K and Lin Y-C (2023) Genetically encoded mediators for sonogenetics and their applications in neuromodulation. Front. Cell. Neurosci. 17:1326279. doi: 10.3389/fncel.2023.1326279
Received: 23 October 2023; Accepted: 05 December 2023;
Published: 22 December 2023.
Edited by:
Chen Yang, Boston University, United StatesReviewed by:
Zhenbo Huang, Texas A&M Health Science Center, United StatesCopyright © 2023 Wang, Phan, Kao, Yeh and Lin. This is an open-access article distributed under the terms of the Creative Commons Attribution License (CC BY). The use, distribution or reproduction in other forums is permitted, provided the original author(s) and the copyright owner(s) are credited and that the original publication in this journal is cited, in accordance with accepted academic practice. No use, distribution or reproduction is permitted which does not comply with these terms.
*Correspondence: Yu-Chun Lin, eWNsJiN4MDAwNDA7bGlmZS5udGh1LmVkdS50dw==; Chih-Kuang Yeh, Y2t5ZWgmI3gwMDA0MDtteC5udGh1LmVkdS50dw==
†These authors have contributed equally to this work