- Division of Neurophysiology, Department of Physiology, School of Medicine, Tokyo Women’s Medical University, Tokyo, Japan
Synapses are junctions between a presynaptic neuron and a postsynaptic cell specialized for fast and precise information transfer. The presynaptic terminal secretes neurotransmitters via exocytosis of synaptic vesicles. Exocytosis is a tightly regulated reaction that occurs within a millisecond of the arrival of an action potential. One crucial parameter in determining the characteristics of the transmitter release kinetics is the coupling distance between the release site and the Ca2+ channel. Still, the technical limitations have hindered detailed analysis from addressing how the coupling distance is regulated depending on the development or activity of the synapse. However, recent technical advances in electrophysiology and imaging are unveiling their different configurations in different conditions. Here, I will summarize developmental- and activity-dependent changes in the coupling distances revealed by recent studies.
Introduction
The transmitter release from the presynaptic nerve terminal is triggered by an influx of Ca2+ from the voltage-gated calcium channels (VGCCs). Because the Ca2+ needs to diffuse from the channel pore to vesicular Ca2+ sensors, the coupling distance between VGCCs and Ca2+ sensors of releasable vesicles is a critical determinant of the release probability of the synaptic vesicles (Meinrenken et al., 2002; Wadel et al., 2007; Eggermann et al., 2011; Nakamura et al., 2015). The coupling distance is one of the key factors in producing functional heterogeneity of different synapses on the presynaptic side, which is also known to be modulated depending on development or synaptic activity (Ohana and Sakmann, 1998; Meinrenken et al., 2002; Fedchyshyn and Wang, 2005; Kochubey et al., 2009; Nakamura et al., 2015; Midorikawa and Miyata, 2021). It is one of the refinement processes at the synapse to establish an adaptive neural network.
The developmental change has been investigated mainly from unusually large presynaptic structures, such as the calyx of Held (Borst and Sakmann, 1996; Fedchyshyn and Wang, 2005; Nakamura et al., 2015) and the endbulb of Held (Oleskevich et al., 2004; Zhuang et al., 2017, 2020) at the auditory pathway. Technological advances have recently enabled recording from lemniscal fiber terminals (LFTs) at the sensory thalamus (Midorikawa and Miyata, 2021), providing another presynaptic model to digest developmental change in the coupling distance. The LFTs also provide an interesting model synapse to study selective strengthening and elimination (Midorikawa, 2022), but in this minireview, I will focus on the developmental change in the coupling distance. Because the calyx of Held terminal, endbulb of Held, and LFTs are all located in the middle of sensory pathways, they are good model synapses to investigate not only developmental but also experience-dependent modulation of the coupling distance.
The developmental/experience-dependent modulation is chronic changes that proceed over days. In addition to the chronic modulations, synapses are amenable to changing their function more acutely via acute intense activity, known as long-term synaptic potentiation and depression. Hippocampal mossy fiber bouton (hMFB) is a suitable model to investigate coupling distance before and after the long-term potentiation (LTP) since it is applicable for patch-clamp recording, and the LTP can be induced by a pharmacological manipulation (Weisskopf et al., 1994; Nicoll and Schmitz, 2005). Another form of acute plasticity is the so-called homeostatic plasticity of the Drosophila neuromuscular junction (NMJ) that occurs in response to postsynaptic impairments and leads to a compensatory increase of presynaptic transmitter release (Davis and Müller, 2015).
These studies have provided detailed information about acute and chronic modulation of coupling distance in different synapses. In this article, I would like to overview these various forms of coupling distance modulations.
Manuscript formatting
Headings
How voltage-gated calcium channel-releasable vesicle coupling distance affects the transmitter release kinetics
The coupling distance of VGCCs and release-ready synaptic vesicles is a key factor in determining the fidelity of the synaptic transmission. When an action potential (AP) arrives at the presynaptic terminal, Ca2+ influx through VGCCs triggers synaptic vesicle fusion and the release of transmitters stored in the synaptic vesicles. Therefore, the spatio-temporal profile of Ca2+ influx is the crucial determinant of the transmitter release, which is strongly affected by the AP waveform and the amount/distribution of functional Ca2+ channels. As for the AP waveform, broader AP is usually associated with a larger Ca2+ current because of the slower downstroke (Geiger and Jonas, 2000), resulting in a larger transmitter release (Augustine, 1990; Borst and Sakmann, 1999). Since the spatio-temporal profile of the Ca2+ is generally steep due to endogenous Ca2+ buffers (Nakamura et al., 2018), not only their numbers but also the distribution of VGCCs relative to the release-ready vesicles also plays a critical role in the efficacy of the transmitter release. The synaptic response can be described by multiplying a fixed number of the transmitter release site, mean release probability, and quantal response size (Takahashi, 2015; Sakaba, 2018). VGCCs-releasable vesicle coupling distance is a major determinant of the release probability among these parameters (Meinrenken et al., 2002; Eggermann et al., 2011; Nakamura et al., 2015).
Among central nervous system (CNS), some synapses have “loose” couplings (Rozov et al., 2001; Fedchyshyn and Wang, 2005; Vyleta and Jonas, 2014; Kawaguchi and Sakaba, 2017), while others have “tight” couplings (Bucurenciu et al., 2008; Eggermann et al., 2011; Schmidt et al., 2013; Kawaguchi and Sakaba, 2015). The different coupling distance results in a distinct pattern of transmitter release in response to incoming APs, which characterize various properties of CNS synapses. In general, “tight” coupling synapses have higher release probabilities than “loose” ones because they are exposed to a higher concentration of Ca2+. Because a large fraction of the readily releasable vesicles is depleted with the first AP, “tight” coupling synapses often demonstrate short-term depression, characterized by progressive weakening of transmitter release upon repetitive stimulations (Zucker and Regehr, 2002; Regehr, 2012). On the other hand, at some synapses with a low initial release probability due to “loose” coupling, repetitive stimuli can result in a progressive strengthening of synaptic responses, known as short-term facilitation (Zucker and Regehr, 2002; Abbott and Regehr, 2004).
It should be noted that besides AP waveform, amount of Ca2+ channels and the coupling distance of VGCCs and release-ready vesicles as shown above, there still are other factors that could affect synaptic vesicles’ release probability. A major alternative possible factor is heterogeneous Ca2+-sensitivity for transmitter release. Ca2+-sensors for the synaptic vesicle fusion synchronized to APs at the CNS are mediated mainly by Synaptotagmin-1, 2, or -9 (Südhof, 2013), but recent accumulating results indicate the critical role of synaptotagmin-7 on the asynchronous transmitter release (Bacaj et al., 2013; Luo and Südhof, 2017). Synaptotagmin-7 is also proposed to play a crucial role in short-term facilitation (Jackman et al., 2016; Turecek et al., 2017).
Developmental change of the coupling distance
In addition to the intrinsic differences among different synapses, the coupling distance changes with development within the individual synapse. A number of studies have shown that the coupling is typically “loose” during early development, but the association becomes “tight” as synapses mature (Ohana and Sakmann, 1998; Meinrenken et al., 2002; Fedchyshyn and Wang, 2005; Figure 1). The coupling distance between VGCCs and releasable vesicles has been examined by investigating the sensitivity to a calcium chelator, e.g., EGTA (ethylene glycol tetraacetic acid), as a large amount of intracellular EGTA predominantly blocks exocytosis of loosely coupled vesicles (Adler et al., 1991; Borst and Sakmann, 1996). At the LFTs, located in the whisker-sensory pathway, the coupling distance is loose before the maturation (Midorikawa and Miyata, 2021). The coupling distance becomes tight at the beginning of the third postnatal week (∼P16), right after the timing when active whisking of rodents begins (Figure 2). Interestingly, the developmental tightening proceeds only at to-be-strengthened LFTs, but not at to-be-eliminated LFTs, despite synapsing onto the same postsynaptic neurons before the maturation (Midorikawa and Miyata, 2021). The developmental strengthening of the to-be-strengthened LFTs caused by the tightening of the coupling distance is postulated as one of the requirements to survive (Midorikawa, 2022). The study indicates that the developmental tightening of the coupling distance proceeds differently at distinct pathways.
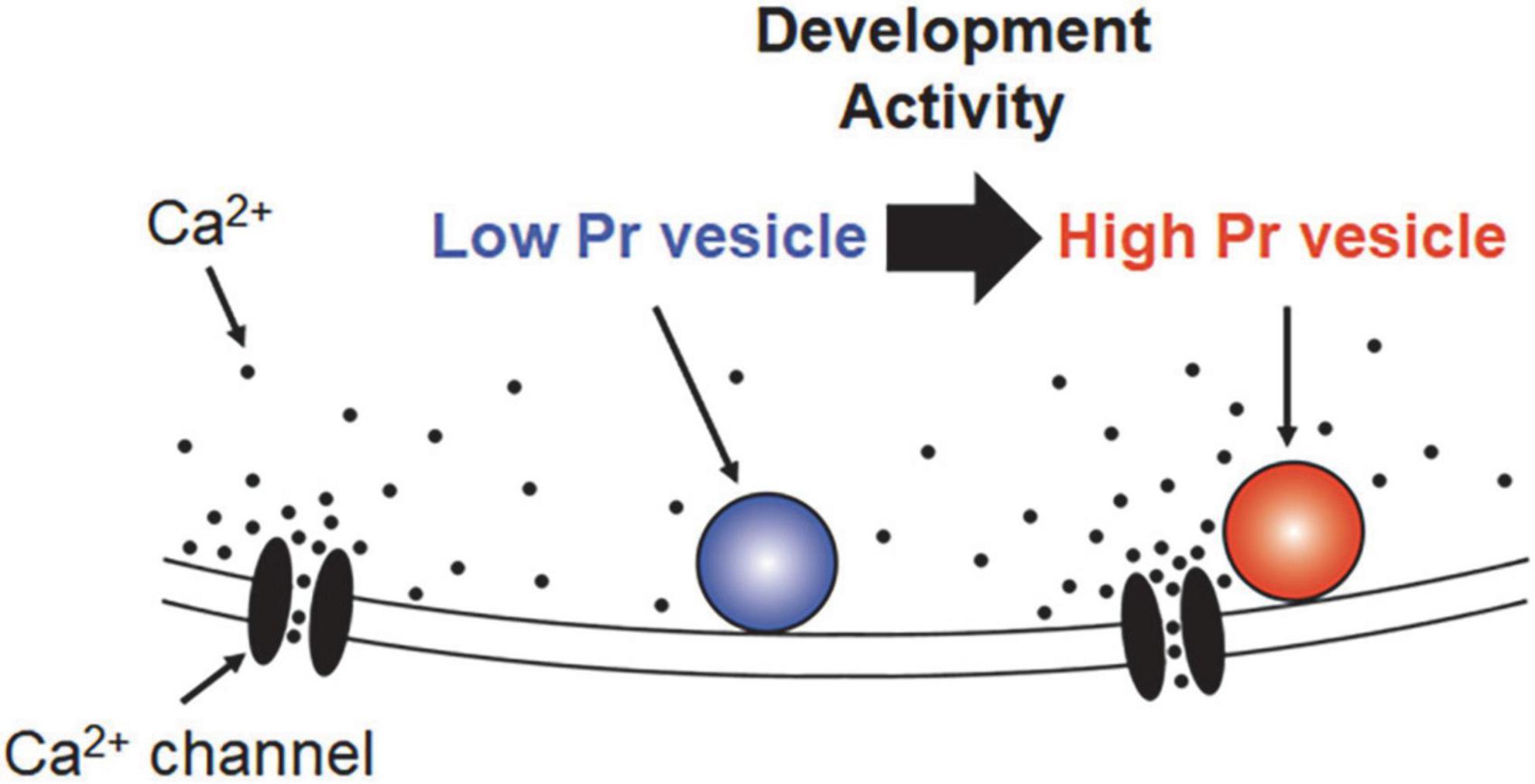
Figure 1. Schematic view of “loose” and “tight” coupling distance. The distance from the voltage-gated calcium channels (VGCCs) (or VGCC clusters) is different between “tight” (red) and “loose” (blue) coupled vesicles. The release probability of “tight” coupled vesicles is high because they are located close to the Ca2+ source, VGCCs, and hence exposed to a high concentration of Ca2+. On the other hand, the release probability of “loose” coupled vesicles is low because Ca2+ is diluted and buffered before reaching its location. The coupling distance could change from “loose” to “tight” depending on the development and/or activity.
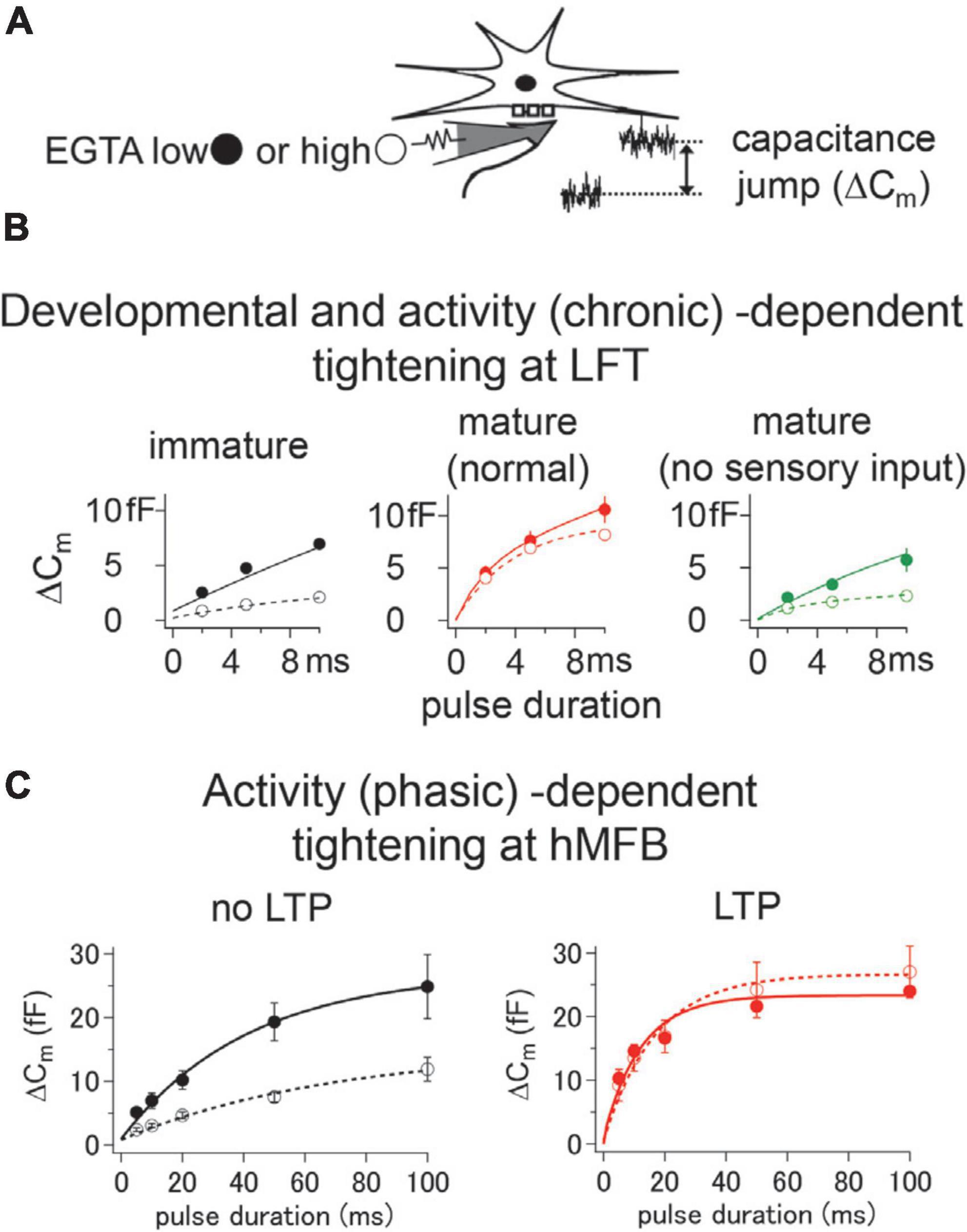
Figure 2. Developmental and chronic/phasic activity-dependent tightening of the coupling distance. (A) The coupling distance was examined by ethylene glycol tetraacetic acid (EGTA) sensitivity. Different amount of EGTA was injected into the presynaptic terminals through the patch-pipette, and the amount of transmitter release was detected by the capacitance measurement technique. (B) At lemniscal fiber terminal (LFT), the coupling distance showed developmental tightening, which can be seen by reduced sensitivity of ΔCm after maturation (compare black and red traces). The developmental tightening was impaired when the animal was grown up in a sensory-deprived condition (green traces). (C) At hippocampal mossy fiber bouton (hMFB), chemical-induced LTP caused tightening of the coupling distance, which was indicated by the reduction of the EGTA sensitivity in an LTP condition. All traces are adapted from Midorikawa and Sakaba (2017) and Midorikawa and Miyata (2021).
The shortening of presynaptic AP wavelength is thought to represent a possible general mechanism underlying the developmental decrease of the release probability among different CNS synapses (Bolshakov and Siegelbaum, 1995; Pouzat and Hestrin, 1997; Taschenberger and Von Gersdorff, 2000; Midorikawa and Miyata, 2021). On the other hand, it has been reported that the amplitude of Ca2+ current enlarges to the mature level before the AP waveform maturation (shortening) (Taschenberger et al., 2002; Nakamura et al., 2015; Midorikawa and Miyata, 2021). At the calyx of Held, it is also shown that the number of VGCCs per cluster and the cluster area increase with development simultaneously (Nakamura et al., 2015). Developmental AP shortening decreases release probability while increasing the VGCCs, and/or the tighter coupling exhibit an antagonistic effect. Another comparison study between “weak” (low release probability) granule cell synapse and “strong” (high release probability) stellate cell synapse demonstrated that weaker granule cell synapse exhibited threefold more VGCCs than stronger stellate cell synapse, while the coupling distance was fivefold longer (Rebola et al., 2019). These studies indicate that the AP waveform, the number of VGCCs, and the coupling distance comprehensively regulate the developmental modulation of the transmitter release probability.
Because the coupling distance is crucial to determine the synaptic transmission property, extensive studies have been performed to investigate the regulatory mechanisms. For the coupling between VGCCs and release-ready synaptic vesicles, it has been shown that several vital proteins such as CAST/ELKS (Dong et al., 2018), neurexins (Missler et al., 2003; Luo et al., 2020), RIMs (Kiyonaka et al., 2007; Han et al., 2015), RIM-binding proteins (Acuna et al., 2015; Grauel et al., 2016; Butola et al., 2021), and (M)Unc13s (Böhme et al., 2016; Reddy-Alla et al., 2017; Kusch et al., 2018) have been proposed. Super molecular complexes consist of these proteins that differ from synapse to synapse, resulting in various synaptic transmission properties. Also, the expression and transportation of these proteins change with development, which could be postulated to underly a maturational tightening of the coupling distance. An interesting finding has been reported from studies of Drosophila NMJ, where Unc13A and -B are simultaneously present at mature presynaptic boutons, but those two isoforms arrive at different timing during synaptic development (Böhme et al., 2016). Unc13B, which mediates loose coupling, comes first and functions during the initial synapse assembly process. Unc13A, which mediates tight coupling, arrives later and mediates the vast majority of AP-evoked release at the mature synapse. Activity-dependent accumulations of the Unc13s are also observed (Böhme et al., 2016), which may shed light on the mechanism of activity-dependent coupling distance modulation in the future. Interestingly, (M)Unc13 proteins themselves might affect the coupling distance shift during maturation by recruiting Ca2+ channels to the active zone as shown at mammalian parallel fiber to Purkinje Cell synapse (Kusch et al., 2018), consistent with a direct interaction of VGCCs to Munc13s (Calloway et al., 2015). The presence of multiple isoforms of Munc13s and their loose redundancy on the transmitter release kinetics have also been reported at the calyx of Held synapse (Chen et al., 2013), at which developmental tightening of the coupling distance occurs (Nakamura et al., 2015).
Activity-dependent modulation of the coupling distance
The synapse formation can be roughly divided into three phases, initial axon targeting and contact with a target cell dendrite, organization of synapse structures to build the canonical synaptic construct shared by all synapses, and specification of synapse properties to confer unique features on each synapse (Südhof, 2017). Although the formation of long-distance axon tracts is restricted mainly to development and activity-independent, local branching of an axon is affected by neuronal activity (Uesaka et al., 2005, 2007). The following step, synapse formation, is a balancing process between formation and elimination. This phase is known to be activity-dependent and has been well studied in several brain regions (LeVay et al., 1980; Kano and Watanabe, 2019; Hooks and Chen, 2020; Midorikawa, 2022). The final step of synapse maturation is a specification of the synapse properties, which are largely affected by the neuronal activity to establish an adaptive neural network against the outer environment.
At the LFTs, continuous deprivation of sensory inputs from just before the onset of the active whisking prevents the developmental tightening of the coupling distance (Midorikawa and Miyata, 2021), which indicates that the process is experience-dependent (Figure 2). In this case, days of deprivation are required to impair the maturation process, suggesting that the tightening of the coupling distance is dependent on chronic activation of the pathway. On the other hand, the developmental shortening of the AP waveform is not affected by sensory deprivation at the LFTs (Midorikawa and Miyata, 2021). Therefore, at the LFTs developed without sensory experience, the shortened AP and the impaired coupling distance may result in unreliable synaptic transmission, leading to impaired sensory processing. In contrast to the unaffected AP waveform by sensory deprivation at LFTs, richer sensory inputs could induce shortenings of the AP waveform at other synapses. AP waveforms of specific neurons in the amygdala, cochlear nucleus, and cerebellum have been shown to be shortened by fear extinction, noise exposure, and enriched environment, respectively (Senn et al., 2014; Ngodup et al., 2015; Eshra et al., 2019). In these cases, tightening of the coupling distance or/and enlargement of the Ca2+ current can be postulated as compensatory mechanisms to maintain or facilitate reliable synaptic transmission.
Unlike LFTs, the kinetics of the transmitter release is not affected by the sensory deprivation at the calyx of Held synapse (Oleskevich et al., 2004), suggesting that developmental tightening of the coupling distance is not dependent on the hearing experience at this synapse. Interestingly at the endbulb synapse, which is located at the hearing pathway one synapse before the calyx of Held synapse, release probability is increased via hearing deprivation during the development (Oleskevich et al., 2004). Here, the increase in the release probability is not due to the tightening of the coupling distance but to the enhancement of the Ca2+ current (Zhuang et al., 2020).
These studies indicate that the activity-dependent developmental modulation of the release probability could work either in a Hebbian plasticity mode (inactive synapse becomes weak, i.e., LFT) or homeostatic plasticity mode (inactive synapse becomes strong, i.e., endbulb of Held).
Besides the chronic activity-dependent modulation of the coupling distance and/or transmitter release kinetics, as shown above, more phasic activity also could change the kinetics of transmitter release by the coupling distance modulation. One such kind of presynaptic plasticity is the homeostatic plasticity of the Drosophila NMJ, which induces a compensatory increase of presynaptic transmitter release in response to postsynaptic receptor blockade (Davis and Müller, 2015). Here, it has been shown that the enhanced transmitter release following the homeostatic plasticity had increased sensitivity to Ca2+ chelator EGTA, indicating that more loosely coupled vesicles are triggered for release under such a condition (Wentzel et al., 2018). Another presynaptic plasticity induced by phasic activity is the LTP of the neuron implicated in learning and memory. Although early studies of long-term synaptic plasticity described a potentiation of postsynaptic signal transduction mechanisms, it is now apparent that there is a vast array of presynaptic mechanisms for LTP (Yang and Calakos, 2013). Arguably best-characterized locus of the presynaptic LTP is the hippocampal mossy fiber synapse, where presynaptic plasticity was first found (Zalutsky and Nicoll, 1990). Several lines of studies, including quantal analysis of EPSCs (Malinow and Tsien, 1990), paired-pulse ratio analysis (Zalutsky and Nicoll, 1990), and monitoring progressive irreversible blockade of NMDA receptor-mediated EPSCs (Weisskopf and Nicoll, 1995) indicate that the LTP of this synapse is caused by an increase in release probability. Here, LTP depends on elevated cAMP levels, protein kinase A activation, and the phosphorylation of presynaptic substrates (Huang et al., 1994; Weisskopf et al., 1994). Because of its large size, hMFB is one of the few presynaptic structures amenable to direct patch-clamp recording (Geiger and Jonas, 2000; Hallermann et al., 2003; Vyleta and Jonas, 2014; Midorikawa and Sakaba, 2017). Direct infusion of cAMP into hMFBs through the patch pipette increased the release probability, accompanied by a reduced EGTA sensitivity, suggesting a tightening of the coupling distance (Midorikawa and Sakaba, 2017; Figure 2). Further study using super-resolution microscopy revealed that the cAMP-induced tighter coupling was caused by the physical expansion of VGCC clusters (Fukaya et al., 2021). It has been shown that the mobility of Ca2+ channels is high with presynaptic terminals (Schneider et al., 2015), and active zones recruit Ca2+ channels via active zone scaffolding proteins such as RIM, RIM-binding proteins, and Neurexins (Kaeser et al., 2011; Liu et al., 2011; Acuna et al., 2015; Han et al., 2015; Luo et al., 2020). Tightening of the coupling distance accompanied with LTP at the hMFB may be caused by the recruitment of VGCCs to active zones by scaffolding factors (Lübbert et al., 2019; Held et al., 2020).
Conclusion and future direction
As overviewed here, the coupling distance between VGCCs and release-ready vesicles is one of the key regulatory principles for determining synaptic behavior. It varies remarkably among different types of synapses, and even within the individual synapse, the coupling distance can be modulated by the chronic (e.g., development, experience-dependent) or phasic (e.g., LTP) activity of the synapse. Recently, the coupling distance has been estimated based on the kinetics of transmitter release measured via electrophysiology, electron microscopy, and model simulation (Budisantoso et al., 2012; Vyleta and Jonas, 2014; Nakamura et al., 2015; Böhme et al., 2016; Rebola et al., 2019). The studies have suggested various coupling distances and distribution patterns of VGCCs and release sites at different synapses under different conditions. Furthermore, the recent evolution of super-resolution microscopy enables us to visualize VGCCs and release site markers, such as Munc13 (Sakamoto et al., 2018), simultaneously to directly measure the coupling distance (Fukaya et al., 2021). The technical advances are shedding light on variable coupling distances among different synapses and their phasic and chronic plasticity.
It is interesting to ask what is shared and what is different between developmental and activity-dependent modulation of coupling distance. The initial triggering signal might be different since basal synaptogenesis mechanisms, and functional maturation of at least a subset of synapses are activity-independent (Verhage et al., 2000; Sando et al., 2017; Sigler et al., 2017). However, the signaling cascade might be converged and shared to some degree.
The ideal experiment will be to visualize the exocytosis of a single synaptic vesicle with Ca2+ influx from VGCCs. Simultaneous measurement of exocytosis of individual synaptic vesicle together with Ca2+ influx (Midorikawa et al., 2007; Midorikawa and Sakaba, 2015) or an active zone marker (Midorikawa et al., 2007; Zenisek, 2008; Joselevitch and Zenisek, 2020) has been performed using total internal reflection fluorescence microscopy, but the diffraction-limited spatial resolution (∼ 200 nm in) is far from sufficient to argue the coupling distance, which is typically in the range of tens to a hundred nanometers. The rapid expansion of live imaging techniques equipped with spatial resolution above the diffraction limit and fast temporal resolution will identify the coupling distance of various synapses in a different state, which will be fruitful for our understanding of the adaptive refinement of the presynaptic transmitter release machinery in the future.
Author contributions
MM wrote the manuscript and prepared the figures.
Funding
This work was supported by the KAKENHI grants from the JSPS/MEXT, Japan (22K19367, 21H02583, 19H03343, and the Grant-in-aid for Transformative Research Area 20H05916), the Brain Science Foundation, and the Takeda Science Foundation.
Conflict of interest
The author declares that the research was conducted in the absence of any commercial or financial relationships that could be construed as a potential conflict of interest.
Publisher’s note
All claims expressed in this article are solely those of the authors and do not necessarily represent those of their affiliated organizations, or those of the publisher, the editors and the reviewers. Any product that may be evaluated in this article, or claim that may be made by its manufacturer, is not guaranteed or endorsed by the publisher.
References
Abbott, L. F., and Regehr, W. G. (2004). Synaptic computation. Nature 431, 796–803. doi: 10.1038/nature03010
Acuna, C., Liu, X., Gonzalez, A., and Südhof, T. C. (2015). RIM-BPs mediate tight coupling of action potentials to Ca(2+)-triggered neurotransmitter release. Neuron 87, 1234–1247. doi: 10.1016/j.neuron.2015.08.027
Adler, E. M., Augustine, G. J., Duffy, S. N., and Charlton, M. P. (1991). Alien intracellular calcium chelators attenuate neurotransmitter release at the squid giant synapse. J. Neurosci. 11, 1496–1507. doi: 10.1523/jneurosci.11-06-01496.1991
Augustine, G. J. (1990). Regulation of transmitter release at the squid giant synapse by presynaptic delayed rectifier potassium current. J Physiol. 431, 343–364. doi: 10.1113/jphysiol.1990.sp018333
Bacaj, T., Wu, D., Yang, X., Morishita, W., Zhou, P., Xu, W., et al. (2013). Synaptotagmin-1 and synaptotagmin-7 trigger synchronous and asynchronous phases of neurotransmitter release. Neuron 80, 947–959. doi: 10.1016/j.neuron.2013.10.026
Böhme, M. A., Beis, C., Reddy-Alla, S., Reynolds, E., Mampell, M. M., Grasskamp, A. T., et al. (2016). Active zone scaffolds differentially accumulate Unc13 isoforms to tune Ca(2+) channel-vesicle coupling. Nat. Neurosci. 19, 1311–1320. doi: 10.1038/nn.4364
Bolshakov, V. Y., and Siegelbaum, S. A. (1995). Regulation of hippocampal transmitter release during development and long-term potentiation. Science 269, 1730–1734. doi: 10.1126/science.7569903
Borst, J. G., and Sakmann, B. (1996). Calcium influx and transmitter release in a fast CNS synapse. Nature 383, 431–434. doi: 10.1038/383431a0
Borst, J. G., and Sakmann, B. (1999). Effect of changes in action potential shape on calcium currents and transmitter release in a calyx-type synapse of the rat auditory brainstem. Philos Trans R Soc Lond B Biol Sci. 354, 347–355. doi: 10.1098/rstb.1999.0386
Bucurenciu, I., Kulik, A., Schwaller, B., Frotscher, M., and Jonas, P. (2008). Nanodomain coupling between Ca2+ channels and Ca2+ sensors promotes fast and efficient transmitter release at a cortical GABAergic synapse. Neuron 57, 536–545. doi: 10.1016/j.neuron.2007.12.026
Budisantoso, T., Matsui, K., Kamasawa, N., Fukazawa, Y., and Shigemoto, R. (2012). Mechanisms underlying signal filtering at a multisynapse contact. J. Neurosci. 32, 2357–2376. doi: 10.1523/jneurosci.5243-11.2012
Butola, T., Alvanos, T., Hintze, A., Koppensteiner, P., Kleindienst, D., Shigemoto, R., et al. (2021). RIM-binding protein 2 organizes Ca2+ channel topography and regulates release probability and vesicle replenishment at a fast central synapse. J. Neurosci. 41, 7742–7767. doi: 10.1523/jneurosci.0586-21.2021
Calloway, N., Gouzer, G., Xue, M., and Ryan, T. A. (2015). The active-zone protein munc13 controls the use-dependence of presynaptic voltage-gated calcium channels. Elife 4:728. doi: 10.7554/eLife.07728
Chen, Z., Cooper, B., Kalla, S., Varoqueaux, F., and Young, S. M. Jr. (2013). The Munc13 proteins differentially regulate readily releasable pool dynamics and calcium-dependent recovery at a central synapse. J. Neurosci. 33, 8336–8351. doi: 10.1523/jneurosci.5128-12.2013
Davis, G. W., and Müller, M. (2015). Homeostatic control of presynaptic neurotransmitter release. Annu. Rev. Physiol. 77, 251–270. doi: 10.1146/annurev-physiol-021014-071740
Dong, W., Radulovic, T., Goral, R. O., Thomas, C., Suarez Montesinos, M., Guerrero-Given, D., et al. (2018). CAST/ELKS proteins control voltage-gated Ca2+ channel density and synaptic release probability at a mammalian central synapse. Cell Rep. 24, 284–293.e286. doi: 10.1016/j.celrep.2018.06.024
Eggermann, E., Bucurenciu, I., Goswami, S. P., and Jonas, P. (2011). Nanodomain coupling between Ca2+ channels and sensors of exocytosis at fast mammalian synapses. Nat. Rev. Neurosci. 13, 7–21. doi: 10.1038/nrn3125
Eshra, A., Hirrlinger, P., and Hallermann, S. (2019). Enriched environment shortens the duration of action potentials in cerebellar granule cells. Front Cell Neurosci. 13:289. doi: 10.3389/fncel.2019.00289
Fedchyshyn, M. J., and Wang, L. Y. (2005). Developmental transformation of the release modality at the calyx of held synapse. J. Neurosci. 25, 4131–4140. doi: 10.1523/JNEUROSCI.0350-05.2005
Fukaya, R., Maglione, M., Sigrist, S. J., and Sakaba, T. (2021). Rapid Ca2+ channel accumulation contributes to cAMP-mediated increase in transmission at hippocampal mossy fiber synapses. Proc. Natl. Acad. Sci. U.S.A. 118:e2016754118. doi: 10.1073/pnas.2016754118
Geiger, J. R., and Jonas, P. (2000). Dynamic control of presynaptic Ca(2+) inflow by fast-inactivating K(+) channels in hippocampal mossy fiber boutons. Neuron 28, 927–939. doi: 10.1016/s0896-6273(00)00164-1
Grauel, M. K., Maglione, M., Reddy-Alla, S., Willmes, C. G., Brockmann, M. M., Trimbuch, T., et al. (2016). RIM-binding protein 2 regulates release probability by fine-tuning calcium channel localization at murine hippocampal synapses. Proc. Natl. Acad. Sci. U.S.A. 113, 11615–11620. doi: 10.1073/pnas.1605256113
Hallermann, S., Pawlu, C., Jonas, P., and Heckmann, M. (2003). A large pool of releasable vesicles in a cortical glutamatergic synapse. Proc Natl. Acad. Sci. U.S.A. 100, 8975–8980. doi: 10.1073/pnas.1432836100
Han, Y., Babai, N., Kaeser, P., Südhof, T. C., and Schneggenburger, R. (2015). RIM1 and RIM2 redundantly determine Ca2+ channel density and readily releasable pool size at a large hindbrain synapse. J. Neurophysiol. 113, 255–263. doi: 10.1152/jn.00488.2014
Held, R. G., Liu, C., Ma, K., Ramsey, A. M., Tarr, T. B., De Nola, G., et al. (2020). Synapse and active zone assembly in the absence of presynaptic Ca2+ channels and Ca2+ entry. Neuron 107, 667–683.e669. doi: 10.1016/j.neuron.2020.05.032
Hooks, B. M., and Chen, C. (2020). Circuitry underlying experience-dependent plasticity in the mouse visual system. Neuron 106, 21–36. doi: 10.1016/j.neuron.2020.01.031
Huang, Y. Y., Li, X. C., and Kandel, E. R. (1994). cAMP contributes to mossy fiber LTP by initiating both a covalently mediated early phase and macromolecular synthesis-dependent late phase. Cell 79, 69–79. doi: 10.1016/0092-8674(94)90401-4
Jackman, S. L., Turecek, J., Belinsky, J. E., and Regehr, W. G. (2016). The calcium sensor synaptotagmin 7 is required for synaptic facilitation. Nature 529, 88–91. doi: 10.1038/nature16507
Joselevitch, C., and Zenisek, D. (2020). Direct observation of vesicle transport on the synaptic ribbon provides evidence that vesicles are mobilized and prepared rapidly for release. J. Neurosci. 40, 7390–7404. doi: 10.1523/JNEUROSCI.0605-20.2020
Kaeser, P. S., Deng, L., Wang, Y., Dulubova, I., Liu, X., Rizo, J., et al. (2011). RIM proteins tether Ca2+ channels to presynaptic active zones via a direct PDZ-domain interaction. Cell 144, 282–295. doi: 10.1016/j.cell.2010.12.029
Kano, M., and Watanabe, T. (2019). Developmental synapse remodeling in the cerebellum and visual thalamus. F1000Res 8:18903. doi: 10.12688/f1000research.18903.1
Kawaguchi, S. Y., and Sakaba, T. (2015). Control of inhibitory synaptic outputs by low excitability of axon terminals revealed by direct recording. Neuron 85, 1273–1288. doi: 10.1016/j.neuron.2015.02.013
Kawaguchi, S. Y., and Sakaba, T. (2017). Fast Ca2+ buffer-dependent reliable but plastic transmission at small CNS synapses revealed by direct bouton recording. Cell Rep. 21, 3338–3345. doi: 10.1016/j.celrep.2017.11.072
Kiyonaka, S., Wakamori, M., Miki, T., Uriu, Y., Nonaka, M., Bito, H., et al. (2007). RIM1 confers sustained activity and neurotransmitter vesicle anchoring to presynaptic Ca2+ channels. Nat. Neurosci. 10, 691–701. doi: 10.1038/nn1904
Kochubey, O., Han, Y., and Schneggenburger, R. (2009). Developmental regulation of the intracellular Ca2+ sensitivity of vesicle fusion and Ca2+-secretion coupling at the rat calyx of Held. J Physiol. 587, 3009–3023. doi: 10.1113/jphysiol.2009.172387
Kusch, V., Bornschein, G., Loreth, D., Bank, J., Jordan, J., Baur, D., et al. (2018). Munc13-3 is required for the developmental localization of Ca2+ channels to active zones and the nanopositioning of Cav2.1 near release sensors. Cell Rep. 22, 1965–1973. doi: 10.1016/j.celrep.2018.02.010
LeVay, S., Wiesel, T. N., and Hubel, D. H. (1980). The development of ocular dominance columns in normal and visually deprived monkeys. J. Comput. Neurol. 191, 1–51. doi: 10.1002/cne.901910102
Liu, K. S. Y., Siebert, M., Mertel, S., Knoche, E., Wegener, S., Wichmann, C., et al. (2011). RIM-binding protein, a central part of the active zone, is essential for neurotransmitter release. Science 334, 1565–1569. doi: 10.1126/science.1212991
Lübbert, M., Goral, R. O., Keine, C., Thomas, C., Guerrero-Given, D., Putzke, T., et al. (2019). CaV2.1 α1 subunit expression regulates presynaptic CaV2.1 abundance and synaptic strength at a central synapse. Neuron 101, 260–273e266. doi: 10.1016/j.neuron.2018.11.028
Luo, F., and Südhof, T. C. (2017). Synaptotagmin-7-mediated asynchronous release boosts high-fidelity synchronous transmission at a central synapse. Neuron 94, 826–839.e823. doi: 10.1016/j.neuron.2017.04.020
Luo, F., Sclip, A., Jiang, M., and Südhof, T. C. (2020). Neurexins cluster Ca2+ channels within the presynaptic active zone. EMBO J. 39:e103208. doi: 10.15252/embj.2019103208
Malinow, R., and Tsien, R. W. (1990). Presynaptic enhancement shown by whole-cell recordings of long-term potentiation in hippocampal slices. Nature 346, 177–180. doi: 10.1038/346177a0
Meinrenken, C. J., Borst, J. G., and Sakmann, B. (2002). Calcium secretion coupling at calyx of held governed by nonuniform channel-vesicle topography. J. Neurosci. 22, 1648–1667. doi: 10.1523/jneurosci.22-05-01648.2002
Midorikawa, M. (2022). Pathway-specific maturation of presynaptic functions of the somatosensory thalamus. Neurosci. Res. 181, 1–8. doi: 10.1016/j.neures.2022.04.008
Midorikawa, M., and Miyata, M. (2021). Distinct functional developments of surviving and eliminated presynaptic terminals. Proc. Natl. Acad. Sci. U.S.A. 2021:118. doi: 10.1073/pnas.2022423118
Midorikawa, M., and Sakaba, T. (2015). Imaging exocytosis of single synaptic vesicles at a fast CNS presynaptic terminal. Neuron 88, 492–498. doi: 10.1016/j.neuron.2015.09.047
Midorikawa, M., and Sakaba, T. (2017). Kinetics of releasable synaptic vesicles and their plastic changes at hippocampal mossy fiber synapses. Neuron 96, 1033–1040.e1033. doi: 10.1016/j.neuron.2017.10.016
Midorikawa, M., Tsukamoto, Y., Berglund, K., Ishii, M., and Tachibana, M. (2007). Different roles of ribbon-associated and ribbon-free active zones in retinal bipolar cells. Nat. Neurosci. 10, 1268–1276. doi: 10.1038/nn1963
Missler, M., Zhang, W., Rohlmann, A., Kattenstroth, G., Hammer, R. E., Gottmann, K., et al. (2003). Alpha-neurexins couple Ca2+ channels to synaptic vesicle exocytosis. Nature 423, 939–948. doi: 10.1038/nature01755
Nakamura, Y., Harada, H., Kamasawa, N., Matsui, K., Rothman, J. S., Shigemoto, R., et al. (2015). Nanoscale distribution of presynaptic Ca(2+) channels and its impact on vesicular release during development. Neuron 85, 145–158. doi: 10.1016/j.neuron.2014.11.019
Nakamura, Y., Reva, M., and DiGregorio, D. A. (2018). Variations in Ca2+ influx can alter chelator-based estimates of Ca2+ channel-synaptic vesicle coupling distance. J. Neurosci. 38, 3971–3987. doi: 10.1523/JNEUROSCI.2061-17.2018
Ngodup, T., Goetz, J. A., McGuire, B. C., Sun, W., Lauer, A. M., and Xu-Friedman, M. A. (2015). Activity-dependent, homeostatic regulation of neurotransmitter release from auditory nerve fibers. Proc. Natl. Acad. Sci. U.S.A. 112, 6479–6484. doi: 10.1073/pnas.1420885112
Nicoll, R. A., and Schmitz, D. (2005). Synaptic plasticity at hippocampal mossy fibre synapses. Nat. Rev. Neurosci. 6, 863–876. doi: 10.1038/nrn1786
Ohana, O., and Sakmann, B. (1998). Transmitter release modulation in nerve terminals of rat neocortical pyramidal cells by intracellular calcium buffers. J. Physiol. 513, 135–148. doi: 10.1111/j.1469-7793.1998.135by.x
Oleskevich, S., Youssoufian, M., and Walmsley, B. (2004). Presynaptic plasticity at two giant auditory synapses in normal and deaf mice. J. Physiol. 560, 709–719. doi: 10.1113/jphysiol.2004.066662
Pouzat, C., and Hestrin, S. (1997). Developmental regulation of basket/stellate cell–>Purkinje cell synapses in the cerebellum. J. Neurosci. 17, 9104–9112. doi: 10.1523/jneurosci.17-23-09104.1997
Rebola, N., Reva, M., Kirizs, T., Szoboszlay, M., Lorincz, A., Moneron, G., et al. (2019). Distinct nanoscale calcium channel and synaptic vesicle topographies contribute to the diversity of synaptic function. Neuron 104, 693–710e699. doi: 10.1016/j.neuron.2019.08.014
Reddy-Alla, S., Böhme, M. A., Reynolds, E., Beis, C., Grasskamp, A. T., Mampell, M. M., et al. (2017). Stable positioning of Unc13 restricts synaptic vesicle fusion to defined release sites to promote synchronous neurotransmission. Neuron 95, 1350–1364.e1312. doi: 10.1016/j.neuron.2017.08.016
Regehr, W. G. (2012). Short-term presynaptic plasticity. Cold Spring Harb Perspect Biol. 4:a005702. doi: 10.1101/cshperspect.a005702
Rozov, A., Burnashev, N., Sakmann, B., and Neher, E. (2001). Transmitter release modulation by intracellular Ca2+ buffers in facilitating and depressing nerve terminals of pyramidal cells in layer 2/3 of the rat neocortex indicates a target cell-specific difference in presynaptic calcium dynamics. J. Physiol. 531, 807–826. doi: 10.1111/j.1469-7793.2001.0807h.x
Sakaba, T. (2018). Kinetics of transmitter release at the calyx of Held synapse. Proc. JPN Acad. Ser. B Phys. Biol. Sci. 94, 139–152. doi: 10.2183/pjab.94.010
Sakamoto, H., Ariyoshi, T., Kimpara, N., Sugao, K., Taiko, I., Takikawa, K., et al. (2018). Synaptic weight set by Munc13-1 supramolecular assemblies. Nat. Neurosci. 21, 41–49. doi: 10.1038/s41593-017-0041-9
Sando, R., Bushong, E., Zhu, Y., Huang, M., Considine, C., Phan, S., et al. (2017). Assembly of excitatory synapses in the absence of glutamatergic neurotransmission. Neuron 94, 312–321.e313. doi: 10.1016/j.neuron.2017.03.047
Schmidt, H., Brachtendorf, S., Arendt, O., Hallermann, S., Ishiyama, S., Bornschein, G., et al. (2013). Nanodomain coupling at an excitatory cortical synapse. Curr. Biol. 23, 244–249. doi: 10.1016/j.cub.2012.12.007
Schneider, R., Hosy, E., Kohl, J., Klueva, J., Choquet, D., Thomas, U., et al. (2015). Mobility of calcium channels in the presynaptic membrane. Neuron 86, 672–679. doi: 10.1016/j.neuron.2015.03.050
Senn, V., Wolff, S. B., Herry, C., Grenier, F., Ehrlich, I., Gründemann, J., et al. (2014). Long-range connectivity defines behavioral specificity of amygdala neurons. Neuron 81, 428–437. doi: 10.1016/j.neuron.2013.11.006
Sigler, A., Oh, W. C., Imig, C., Altas, B., Kawabe, H., Cooper, B. H., et al. (2017). Formation and Maintenance of Functional Spines in the Absence of Presynaptic Glutamate Release. Neuron 94, 304–311.e304. doi: 10.1016/j.neuron.2017.03.029
Südhof, T. C. (2013). Neurotransmitter release: the last millisecond in the life of a synaptic vesicle. Neuron 80, 675–690. doi: 10.1016/j.neuron.2013.10.022
Südhof, T. C. (2017). Synaptic neurexin complexes: a molecular code for the logic of neural circuits. Cell 171, 745–769. doi: 10.1016/j.cell.2017.10.024
Takahashi, T. (2015). Strength and precision of neurotransmission at mammalian presynaptic terminals. Proc. JPN Acad. Ser. B Phys. Biol. Sci. 91, 305–320. doi: 10.2183/pjab.91.305
Taschenberger, H., and Von Gersdorff, H. (2000). Fine-tuning an auditory synapse for speed and fidelity: developmental changes in presynaptic waveform, epsc kinetics, and synaptic plasticity. J. Neurosci. 20, 9162–9173. doi: 10.1523/jneurosci.20-24-09162.2000
Taschenberger, H., Leão, R. M., Rowland, K. C., Spirou, G. A., and Von Gersdorff, H. (2002). Optimizing synaptic architecture and efficiency for high-frequency transmission. Neuron 36, 1127–1143. doi: 10.1016/s0896-6273(02)01137-6
Turecek, J., Jackman, S. L., and Regehr, W. G. (2017). Synaptotagmin 7 confers frequency invariance onto specialized depressing synapses. Nature 551, 503–506. doi: 10.1038/nature24474
Uesaka, N., Hayano, Y., Yamada, A., and Yamamoto, N. (2007). Interplay between laminar specificity and activity-dependent mechanisms of thalamocortical axon branching. J. Neurosci. 27, 5215–5223. doi: 10.1523/jneurosci.4685-06.2007
Uesaka, N., Hirai, S., Maruyama, T., Ruthazer, E. S., and Yamamoto, N. (2005). Activity dependence of cortical axon branch formation: a morphological and electrophysiological study using organotypic slice cultures. J. Neurosci. 25, 1–9. doi: 10.1523/jneurosci.3855-04.2005
Verhage, M., Maia, A. S., Plomp, J. J., Brussaard, A. B., Heeroma, J. H., Vermeer, H., et al. (2000). Synaptic assembly of the brain in the absence of neurotransmitter secretion. Science 287, 864–869. doi: 10.1126/science.287.5454.864
Vyleta, N. P., and Jonas, P. (2014). Loose coupling between Ca2+ channels and release sensors at a plastic hippocampal synapse. Science 343, 665–670. doi: 10.1126/science.1244811
Wadel, K., Neher, E., and Sakaba, T. (2007). The coupling between synaptic vesicles and Ca2+ channels determines fast neurotransmitter release. Neuron 53, 563–575. doi: 10.1016/j.neuron.2007.01.021
Weisskopf, M. G., and Nicoll, R. A. (1995). Presynaptic changes during mossy fibre LTP revealed by NMDA receptor-mediated synaptic responses. Nature 376, 256–259. doi: 10.1038/376256a0
Weisskopf, M. G., Castillo, P. E., Zalutsky, R. A., and Nicoll, R. A. (1994). Mediation of hippocampal mossy fiber long-term potentiation by cyclic AMP. Science 265, 1878–1882. doi: 10.1126/science.7916482
Wentzel, C., Delvendahl, I., Sydlik, S., Georgiev, O., and Müller, M. (2018). Dysbindin links presynaptic proteasome function to homeostatic recruitment of low release probability vesicles. Nat Commun. 9:267. doi: 10.1038/s41467-017-02494-0
Yang, Y., and Calakos, N. (2013). Presynaptic long-term plasticity. Front. Synaptic Neurosci. 5:8. doi: 10.3389/fnsyn.2013.00008
Zalutsky, R. A., and Nicoll, R. A. (1990). Comparison of two forms of long-term potentiation in single hippocampal neurons. Science 248, 1619–1624. doi: 10.1126/science.2114039
Zenisek, D. (2008). Vesicle association and exocytosis at ribbon and extraribbon sites in retinal bipolar cell presynaptic terminals. Proc. Natl. Acad. Sci. U.S.A. 105, 4922–4927. doi: 10.1073/pnas.0709067105
Zhuang, X., Sun, W., and Xu-Friedman, M. A. (2017). Changes in properties of auditory nerve synapses following conductive hearing loss. J. Neurosci. 37, 323–332. doi: 10.1523/jneurosci.0523-16.2016
Zhuang, X., Wong, N. F., Sun, W., and Xu-Friedman, M. A. (2020). Mechanisms and functional consequences of presynaptic homeostatic plasticity at auditory nerve synapses. J. Neurosci. 40, 6896–6909. doi: 10.1523/jneurosci.1175-19.2020
Keywords: transmitter release, presynaptic terminal, coupling distance, development, long-term plasticity
Citation: Midorikawa M (2022) Developmental and activity-dependent modulation of coupling distance between release site and Ca2+ channel. Front. Cell. Neurosci. 16:1037721. doi: 10.3389/fncel.2022.1037721
Received: 06 September 2022; Accepted: 11 October 2022;
Published: 26 October 2022.
Edited by:
Haruyuki Kamiya, Hokkaido University, JapanReviewed by:
Takeshi Sakaba, Max Planck Institute for Biophysical Chemistry, GermanyRyuichi Shigemoto, Institute of Science and Technology Austria (IST Austria), Austria
Copyright © 2022 Midorikawa. This is an open-access article distributed under the terms of the Creative Commons Attribution License (CC BY). The use, distribution or reproduction in other forums is permitted, provided the original author(s) and the copyright owner(s) are credited and that the original publication in this journal is cited, in accordance with accepted academic practice. No use, distribution or reproduction is permitted which does not comply with these terms.
*Correspondence: Mitsuharu Midorikawa, bWlkb3Jpa2F3YS5taXRzdWhhcnVAdHdtdS5hYy5qcA==