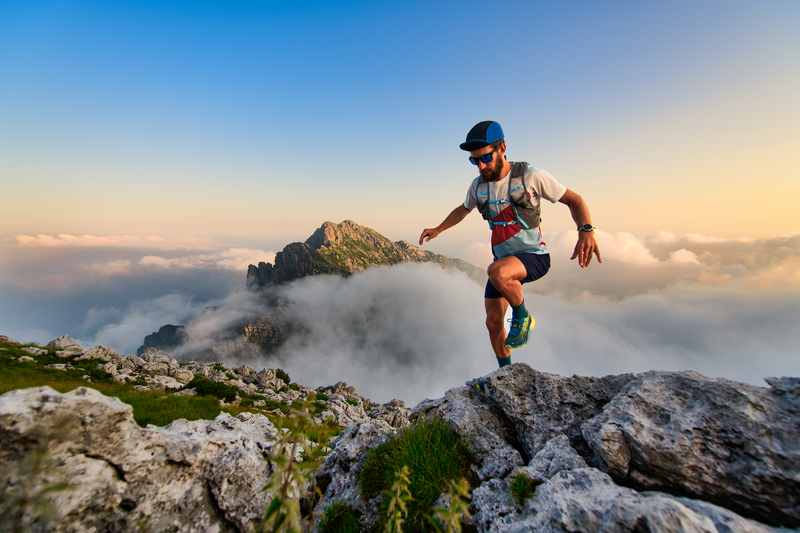
95% of researchers rate our articles as excellent or good
Learn more about the work of our research integrity team to safeguard the quality of each article we publish.
Find out more
REVIEW article
Front. Cell. Infect. Microbiol. , 18 March 2025
Sec. Virus and Host
Volume 15 - 2025 | https://doi.org/10.3389/fcimb.2025.1547873
Mosquito-borne diseases such as chikungunya, dengue, and Zika represent a major burden on global public health. To fight against these arboviruses, vector control strategies are a priority. One existing strategy is based on the use of an endosymbiotic bacterium, Wolbachia, which reduces the transmission of arboviruses by the mosquito Aedes aegypti via a pathogen blocking effect. Wolbachia in Ae. aegypti disrupts several pathways of the host’s metabolism. Trehalose is a carbohydrate circulating mainly in insect hemolymph and plays a role in numerous mechanisms as energy source or stress recovery molecule and in chitin synthesis. This study explores the importance of trehalose in the interactions between Wolbachia and Ae. aegypti, and attempts to understand the pathogen blocking effect.
Vector-borne diseases exert a significant impact on public health contributing to global morbidity and mortality (Bhatt et al., 2013; LaBeaud et al., 2011). For example, 400 million people are infected by dengue virus (DENV) in the world each year (Bhatt et al., 2013; WHO, 2023a), costing $9 billion (Shepard et al., 2016). Aedes aegypti is a primary vector of several arboviruses including DENV (WHO, 2023b). There is no specific treatment against dengue fever and vector control remains the main strategy to limit its emergence and rapid spread. Among vector control strategies (Achee et al., 2019), insecticide treatments are mainly implemented to interrupt dengue transmission (Van Den Berg et al., 2021). However, control efforts have failed to limit dengue fever epidemics and targeted mosquito populations developed resistance to insecticides (Asgarian et al., 2023), urging the development of alternative vector control strategies. Among them, vector control using Wolbachia pipientis has met with significant success (Hilgenfeld and Vasudevan, 2018).
Wolbachia are Gram-negative bacteria belonging to the Alphaproteobacteria class and the Rickettsiales order. Discovered in 1924 (Hertig and Wolbach, 1924), Wolbachia are obligate intracellular bacteria found in nematodes, insects and other arthropods; 40-60% of insects are infected by Wolbachia (Weinert et al., 2015). These bacteria are vertically transmitted and manipulate their hosts to secure their own transmission to the progeny by: (i) inducing a sex ratio distortion in favor of Wolbachia-infected females via parthenogenesis, feminization, male killing or (ii) sterilizing certain individuals via cytoplasmic incompatibility (Landmann, 2019). These effects are not shared by all Wolbachia strains, e.g. Wolbachia from filarial nematodes. In arthropods, Wolbachia strains manipulate the host reproduction and inhibit the transmission of some pathogens, making them potential candidates for vector control (Gill et al., 2014). In insects, Wolbachia is mainly present in reproductive tissues (ovaries) but also in somatic tissues such as the midgut, fat body, salivary glands and muscles (Mejia et al., 2022; Zouache et al., 2009). The relationship between the host and the endosymbiont can be parasitic, mutualistic or commensal (Newton and Rice, 2020), depending on cellular host conditions and the availability of certain nutrients (Lindsey et al., 2018). In addition, the bacterium modifies the host intracellular environment (Lindsey et al., 2018) affecting the cytoskeleton (microtubule and actin) (Ferree et al., 2005; Sheehan et al., 2016) and also modulates gene expression in the host cell; differential expression of genes related to metabolism (Molloy et al., 2016), immunity (Xi et al., 2008) or synthesis of antioxidant molecules [21, 22]. Additionally, Wolbachia induce oxidative stress (Pan et al., 2012), which indirectly affects host metabolism (Bhardwaj and He, 2020) and immunity (Pan et al., 2012; Zug and Hammerstein, 2015). All these changes induced by Wolbachia affect the replication and transmission of DENV in Ae. aegypti (Reyes et al., 2021; Terradas and McGraw, 2017). This phenomenon is known as pathogen blocking effect or pathogen interference. The exact mechanisms leading to the pathogen-blocking effect are not yet fully elucidated, but they appear to be multifactorial, involving immunity, competition for resources, lipids, and oxidative stress (Reyes et al., 2021; Terradas and McGraw, 2017).
Wolbachia strategy is at the base of two distinct and complementary approaches in vector control: (i) incompatible insect technique based on cytoplasmic incompatibility to reduce the size of vector populations and (ii) pathogen-blocking effect to limit the transmission of arboviruses (Caragata et al., 2021). The most commonly Wolbachia strains used for vector control are wMel from Drosophila melanogaster and wAlbB from Aedes albopictus trans-infected in Ae. aegypti (Caragata et al., 2021; Ross, 2021). The pathogen blocking phenotype is Wolbachia strain- and host-dependent (Jiménez et al., 2019); wAlbB enhances, rather than inhibits, West Nile virus infections in Culex tarsalis (Dodson et al., 2014; Glaser and Meola, 2010). The effects produced by Wolbachia are based on a variety of cellular and molecular mechanisms. Indeed, Wolbachia have an effect on the carbohydrate and lipid metabolism and among carbohydrates, trehalose is an essential molecule for growth, fertility, and vitality.
Trehalose (α-D-glucopyranosyl-α-D-glucopyranoside) is a non-reducing disaccharide found in insects (Figure 1A). Present in soluble form at high concentrations (5 to 50mM) in insect hemolymph, its concentration is highly dependent on environmental conditions, nutrition and the insect state of stress (homeostasis disturbance) (Becker et al., 1996; Tamayo et al., 2022; Thompson, 2003). Trehalose participates in several physiological processes such as metabolism, development, chitin synthesis, flight, recovery from stress, and more globally in maintaining homeostasis (Matsuda et al., 2015; Shukla et al., 2015).
Figure 1. Trehalose molecule, synthesis and pathway. (A) Haworth representation of trehalose, a glucose dimer linked by a α,α-1,1 bond. (B) Trehaloneogenesis, the trehalose-forming metabolic pathway. (C) Trehalose involved in trehaloneogenesis and glycolysis. UDP, Uridine diphosphate glucose; iP, inorganic phosphate; TPS, Trehalose 6-phosphate synthase; TPP, Trehalose 6-phosphatase; TREH, Trehalase; GLUT1, Glucose transporter I; HK, Hexokinase; PGM, Phosphoglucomutase; GP, Glycogen phosphorylase; G1P, Glucose 1-phsophate; G6P, glucose 6-phosphate; T6P, trehalose 6-phosphate; HTH, Hypertrehalosemic hormone;TRET1, Trehalose transporter I (Created in https://BioRender.com).
We examine the role of trehalose in interactions between Wolbachia and an arbovirus in Ae. aegypti, and discuss implications of these relationships for the control of mosquito-borne diseases.
Trehalose is a molecule synthesized in the fat body through trehaloneogenesis from glucose acquired with food. The trehaloneogenesis pathway diverts one of the intermediates of glycolysis: glucose-6-phosphate (G6P) as substrate for trehalose-6-phosphate synthase (TPS) producing trehalose-6-phosphate (T6P) which is dephosphorylated via trehalose-6-phosphate-phosphatase (TPP) giving trehalose (Figure 1B) (Shukla et al., 2015). Then, this molecule is released in the hemolymph via the trehalose transporters TRET1 (Tamayo et al., 2022) and cleaved into two glucose molecules by trehalases (TREH), which operate in two forms: TREH-1 soluble and TREH-2 anchored in the membrane of all insect cell types (muscle, midgut, salivary glands, fat body) (Tamayo et al., 2022). Finally, glucose molecules produced by cleavage are imported into cells via glucose transporters (GLUT1), initiating multiple metabolic pathways including glycolysis (Figure 1C) (Tamayo et al., 2022). Trehalose can also be imported into various types of cells via TRET1 or through active transporters. Indeed, TRET1 is highly expressed in fat body cells and muscles (Kanamori et al., 2010) while Malpighian tubules possess active transporters for trehalose (Figure 1C) (Kanamori et al., 2010).
Trehalose plays a fundamental role in insects due to its versatility in many physiological processes (Thompson, 2003). As the main circulating carbohydrate, it serves not only as an energy source for metabolic activities (Becker et al., 1996; Shukla et al., 2015; Thompson, 2003), but is also involved in energy storage in the form of glycogen (Zeng et al., 2020). Additionally, trehalose acts as a protector against extreme environmental conditions, providing cryoprotection against cold (Huang et al., 2022) and shielding cells from desiccation (Thorat et al., 2012). It is also involved in chitin synthesis (Yang et al., 2024), an essential component of insect exoskeleton, and plays a role in key biological processes such as oogenesis and diapause (Becker et al., 1996; Zeng et al., 2020). Finally, trehalose is crucial for recovery after periods of stress, demonstrating its vital importance for insect survival and adaptation to changing environments (Shukla et al., 2015; Thompson, 2003; Yasugi et al., 2017; Yu et al., 2020).
In insects, trehaloneogenesis is a pathway regulated by hormones (Tellis et al., 2023; Thompson, 2003). Indeed, trehaloneogenesis is activated in fat body cells by hormones secreted from the nervous system (e.g. juvenile hormone analogs, 20-Hydroxyecdysone (20E) and diuretic hormone (DH)) (Shukla et al., 2015; Tellis et al., 2023). Some factors such as exposure to pesticides and temperature shifts, increase trehalase activity while others (hormonal regulation via regulating factors) reduce it (Shukla et al., 2015; Tellis et al., 2023). Thus, trehalose concentration varies throughout insect life. The hypertrehalosemic hormone (HTH) is responsible for regulating trehalose levels in insects (Thompson, 2003). HTH is primarily released in response to increased energy demand or stressful conditions, and it stimulates the release of stored trehalose from tissues to provide an additional energy source for the insect (Thompson, 2003). This helps to maintain trehalose homeostasis and ensures that insects have a readily available energy source when needed (Tellis et al., 2023); HTH is released when insects need additional energy (e.g. for flight) (Thompson, 2003). This stimulates the breakdown of glycogen stored in tissues into glucose (Thompson, 2003). Subsequently, glucose is converted into trehalose, which is released into the hemolymph (Tellis et al., 2023; Thompson, 2003). Additionally, the regulation of trehalose concentration in the hemolymph is ensured by modulating the expression of trehalose transporters, such as TRET1, in the fat body cells (Kanamori et al., 2010; Tamayo et al., 2022).
The presence of Wolbachia disrupts various cellular dynamics of its host. Indeed, Wolbachia can modify the expression of certain host genes (Caragata et al., 2017; Lindsey et al., 2021). Genes involved in membrane transport (glucose transporter, permeases, monocarboxylate or cholesterol transporters) and carbohydrate metabolism are over-expressed, activating the host cell global metabolism (Caragata et al., 2017). Induced changes increase the amount of nutrients imported and metabolic activity, as well as trehalose concentration which depends on iron availability in the organism (Currin-Ross et al., 2021). However, it has been shown that Wolbachia can use trehalose and glycogen reserves, increasing the amount of glucose (Zhang et al., 2021). Wolbachia wMel strain lacks a trehalose-forming pathway, but synthesizes enzymes that can import and use trehalose (Jiménez et al., 2019). Thus, the Wolbachia-infected host may use trehalose to obtain glucose, a main source of energy (Currin-Ross et al., 2021), as well as other nutrients such as glyceraldehyde 3-phosphate (GP3) (Jiménez et al., 2019; Lindsey et al., 2018). The bacterium induces an increased level of glucose (Zhang et al., 2021) and therefore participates in energy metabolism such as glycolysis (Shukla et al., 2015), providing it with essential metabolic intermediates and precursors (G3P) and energy. NADH synthetized could indirectly benefit Wolbachia via the pentose phosphate pathway using G6P as a metabolic precursor.
Wolbachia also affects host hormone regulation. Indeed, this bacterium impacts the regulation of its host’s insulin/IGF-like signaling pathways (Ikeya et al., 2009). The insulin/IGF pathway, in turn, is known to affect trehalose carbohydrate storage in insects (Bobrovskikh and Gruntenko, 2023; Broughton et al., 2005). Furthermore, it has been shown that the level of juvenile hormone (JH) is elevated in Wolbachia-infected Drosophila melanogaster males (Zhang et al., 2021). This hormone is known to regulate trehalose synthesis (Xu et al., 2013). Finally, Wolbachia-induced oxidative stress (Pan et al., 2012) leads to a hormonal response with synthesis of hormones belonging to the adipokinetic hormones (AKH) family (Chaitanya et al., 2016); this hormone also plays a role in increasing the amount of trehalose, using glycogen reserves for energy purposes (Huang et al., 2012; Lu et al., 2019). The increased amount of energy produced would compensate for losses of resources diverted by Wolbachia. Glycolysis is an essential component of intracellular bacteria-host interactions; it was shown that pyruvate maintains the symbiotic relationship (Melnikow et al., 2013; Voronin et al., 2019, 2016). In addition, in immune cells, sugar metabolism (from trehalose and glucose), particularly the pentose phosphate pathway (PPP cycle), is crucial not only for fighting and regulating infections but also for protecting the host from the effects of its own immune response and for ensuring the fitness (Kazek et al., 2024). This phenomenon could be accentuated with DENV infection. Indeed, competition for resources and energy becomes critical, and trehalose regulation may help in host cell survival.
As previously mentioned, Wolbachia induces oxidative stress, resulting in increased concentrations of reactive oxygen species (ROS) (H2O2, O2•, OH•) (Pan et al., 2012). Oxidative stress is unsustainable for the cell if prolonged, as ROS induce lipid peroxidation, damage to genetic material or apoptosis (Kodrík et al., 2015; Martemucci et al., 2022). To preserve cell integrity, it is vital to counterbalance this oxidative stress with antioxidant mechanisms (Felton and Summers, 1995). These can be antioxidant enzymes such as Glutathione S-transferase (GST), or antioxidant molecules such as glutathione, ascorbic acid, uric acid and carbohydrates (Felton and Summers, 1995), which are ubiquitous in insect tissues (fat bodies, midgut, Malpighian tubes) (Felton and Duffey, 1992). Antioxidant molecules trap molecules responsible for oxidative stress (Felton and Summers, 1995). As trehalose is a carbohydrate, it could be a key molecule in the regulation of Wolbachia-induced oxidative stress in Ae. aegypti. Indeed, trehalose can scavenge free radicals, thus acting as an antioxidant, at least in insect hemolymph (Felton and Summers, 1995). Thus, oxidative stress can be attenuated by regulating trehalose metabolism (Peng et al., 2024; Thorat et al., 2016). Similarly, trehalose intake reduces the amount of oxidizing molecules, giving it at least an antioxidant effect (Peng et al., 2024). The increase in trehalose seems to be involved in regulating redox balance (Thorat et al., 2016). Furthermore, trehalose is also involved in redox balance in many other organisms (antioxidant molecule), as in yeast, certain bacteria, mammals and plants (Benaroudj et al., 2001; Luo et al., 2008; Reyes-DelaTorre et al., 2012; Zhang et al., 2023), trehalose accumulation during cellular stress such as oxidative stress may reduce free radical damage via mechanisms that remain unclear (Benaroudj et al., 2001; Reyes-DelaTorre et al., 2012). We therefore suggest a similar accumulation mechanism in Ae. aegypti, participating in the regulation of oxidative stress, as Wolbachia does (Pan et al., 2012).
Interactions between Wolbachia and Ae. aegypti can take various forms and lead to different effects: metabolic (Lindsey et al., 2018; Ponton et al., 2015; Reyes et al., 2021), immune (Caragata et al., 2017; McGraw, 2004; Souza-Neto et al., 2009; Ye et al., 2013) or others (Lindsey et al., 2018). All together they impact DENV replication, leading to a pathogen-blocking effect (Reyes et al., 2021; Terradas and McGraw, 2017). Exchanges and interactions between Wolbachia and its mosquito host take place via diverse detection and signaling processes (Lindsey, 2020). In addition, Wolbachia interacts with its host’s RNA (Terradas et al., 2017); the miRNA (micro) pathway participates in “pathogen-blocking” effect by wMelPop strain in Ae. aegypti (Hedges et al., 2008). Inhibition of certain miRNAs leads to a decrease in Wolbachia density, suggesting that the endosymbiont facilitates its maintenance in the host by manipulating host gene expression via miRNAs (Zhang et al., 2013), as piRNAs (Mayoral et al., 2014). piRNAs are important in cell signaling and host immune responses. Nevertheless, the nature of all the interactions between Wolbachia and its host is not fully understood.
Trehalose may play a role in these interactions. This molecule has been shown to be crucial in interactions between the bean bug and a Gram-negative symbiont bacterium (Lee et al., 2023). The same role can be suggested in the relationship between Ae. aegypti and Wolbachia. Indeed, the trehalose imported by the cell carrying Wolbachia could directly serve the endosymbiont itself. It is believed that Wolbachia do not have their own trehaloneogenesis pathway (Jiménez et al., 2019). However, the Wolbachia wMel strain possesses a PEP (phosphoenolpyruvate) system for trehalose transport within the bacterium (Jiménez et al., 2019), as well as a trehalose 6-phosphate-specific phosphohydrolase, resulting in trehalose production (Jiménez et al., 2019). A portion of the trehalose could be used for the synthesis of trehalose-forming glycolipids, as it is the case in other types of intracellular bacteria (Asselineau and Asselineau, 1978; Reinink et al., 2019). A hypothesis here is that these glycolipids could play a role in the Wolbachia-induced pathogen-blocking effect in Ae. aegypti. Indeed, these molecules are reported as having immunostimulant properties (Asselineau and Asselineau, 1978; Vanaporn and Titball, 2020) and can be brought into contact with the host through as yet unknown mechanisms, supporting the immune system priming hypothesis in pathogen blocking effect.
Autophagy is a self-degradative process pivotal for re-equilibrating energy sources at critical times in development and response to stress (Jo et al., 2021; Li et al., 2022; Tracy and Baehrecke, 2013). Autophagy is a highly conserved intracellular mechanism in eukaryotes (Glick et al., 2010; Kuo et al., 2018). Although the mechanism remains universal, some proteins involved in this process are different in insects (Brackney, 2017; Jain et al., 2015; Kuo et al., 2018). Autophagy is an important process involved in interactions between Wolbachia and Ae. aegypti. Host autophagy directly affects Wolbachia density (Deehan et al., 2021), but also Wolbachia infection (Hargitai et al., 2022). Autophagy could also indirectly allow Wolbachia colonization in Ae. aegypti, since this mechanism regulates oxidative stress (Chaitanya et al., 2016; Wu et al., 2009), avoiding apoptosis. Activation of autophagy can also eliminate pathogens such as arboviruses (Jo et al., 2021; Mahanta et al., 2023; Wu et al., 2009), since arboviruses also influence autophagy in infected cells (Barletta et al., 2016; Echavarria-Consuegra et al., 2019; Heaton and Randall, 2011). Trehalose has been shown to induce and regulate autophagy in many organisms, with mechanisms that need to be determined. This molecule induces autophagy leading to various effects in human cells: an antiviral effect (Belzile et al., 2016), an antioxidant effect (Honma et al., 2018; Mizunoe et al., 2018), an induction of apoptosis in the cell (Darabi et al., 2018) or a renewal effect of intracellular components (Xu et al., 2019). Trehalose-inducing autophagy effect is also described in plants (Williams et al., 2015). It is conceivable that the highly conserved process of autophagy could also be induced by trehalose in insect cells and participates in interactions between Wolbachia and Ae. aegypti.
Trehalose is an essential disaccharide for insects. Its role in maintaining metabolism in the presence of Wolbachia, in stress recovery, as an antioxidant and potentially as an inducer of autophagy and priming of the immune system through glycolipid synthesis, makes it a key molecule to understand the Ae. aegypti - Wolbachia - arbovirus relationship (Figure 2).
Figure 2. The role of trehalose in interactions between Wolbachia and Aedes aegypti. The green circles with a W represent Wolbachia bacteria. TREH, Trehalase; GLUT1, Glucose transporter; TRET, Trehalose transporter; Tnut, Transporter of nutrients; G6P, glucose 6-phosphate; G3P, Glycéraldéhyde-3-phosphate; JH, Juvenile hormone; AKH, Adipokinetic hormones; miRNA, microRNA. (Created in https://BioRender.com).
Despite the knowledge recently acquired on the interactions between Wolbachia and Ae. aegypti, many questions remain unresolved. Future research avenues are proposed, including experimental studies to elucidate the precise role of trehalose in Wolbachia colonization and pathogen blocking effect. The potential role of trehalose in autophagy induction should be addressed by experimentally testing if trehalose supplementation leads to autophagic structure formation. Similarly, the presence of trehalose forming lipids should be confirmed.
It has been shown that inactivation of TRET decreases significantly trehalose concentration in the hemolymph of Anopheles gambiae, responding differently to various stresses including infection with Plasmodium falciparum; low levels of circulating trehalose significantly reduced parasite infection, suggesting that trehalose plays a role in the sporogonic development of the parasite (Liu et al., 2013). These results could inspire further studies on arboviral infections, by carrying out inactivation or RNAi studies targeting trehaloneogenesis proteins to find out whether viral replication and vectorial competence of Ae. aegypti are affected.
Finally, the additional knowledge gained could contribute to a better understanding of the pathogen blocking effect, enabling the conception of transgenic mosquito lines devoid of Wolbachia but having kept the pathogen-blocking effect. In addition, this knowledge will help in designing new-generation insecticides targeting trehaloneogenesis proteins, as is currently being done (García and Argüelles, 2021; Matassini et al., 2020).
BD: Writing – original draft. NP: Writing – review & editing. A-BF: Writing – review & editing.
The author(s) declare that financial support was received for the research and/or publication of this article. This study was supported by the Institut Pasteur (Project Explore) and the Laboratoire d’Excellence “Integrative Biology of Emerging Infectious Diseases” (grant n°ANR-10-LABX-62-IBEID). BD received a doctoral fellowship from ANRS-MIE (ArboFrance). The funders had no role in study design, data collection and interpretation, or decision to submit the work for publication.
We thank Christian Mitri, Marie Vazeille, Lazare Brézillon-Dubus, Adrien Blisnick, and Marine Viglietta for their comments, and Patricia Smiley for editing the manuscript.
The authors declare that the research was conducted in the absence of any commercial or financial relationships that could be construed as a potential conflict of interest.
The author(s) declare that no Generative AI was used in the creation of this manuscript.
All claims expressed in this article are solely those of the authors and do not necessarily represent those of their affiliated organizations, or those of the publisher, the editors and the reviewers. Any product that may be evaluated in this article, or claim that may be made by its manufacturer, is not guaranteed or endorsed by the publisher.
Achee, N. L., Grieco, J. P., Vatandoost, H., Seixas, G., Pinto, J., Ching-NG, L., et al. (2019). Alternative strategies for mosquito-borne arbovirus control. PloS Negl. Trop. Dis. 13, e0006822. doi: 10.1371/journal.pntd.0006822
Asgarian, T. S., Vatandoost, H., Hanafi-Bojd, A. A., Nikpoor, F. (2023). Worldwide Status of Insecticide Resistance of Aedes aEgypti and Ae. albopictus, Vectors of Arboviruses of Chikungunya, Dengue, Zika and Yellow Fever. J. Arthropod-Borne Dis. 17, 1–27. doi: 10.18502/jad.v17i1.13198
Asselineau, C., Asselineau, J. (1978). Trehalose-containing glycolipids. Prog. Chem. Fats Other Lipids 16, 59–99. doi: 10.1016/0079-6832(78)90037-X
Barletta, A. B. F., Alves, L. R., Nascimento Silva, M. C. L., Sim, S., Dimopoulos, G., Liechocki, S., et al. (2016). Emerging role of lipid droplets in Aedes aEgypti immune response against bacteria and Dengue virus. Sci. Rep. 6, 19928. doi: 10.1038/srep19928
Becker, A., Schlöder, P., Steele, J. E., Wegener, G. (1996). The regulation of trehalose metabolism in insects. Experientia 52, 433–439. doi: 10.1007/BF01919312
Belzile, J.-P., Sabalza, M., Craig, M., Clark, E., Morello, C. S., Spector, D. H. (2016). Trehalose, an mTOR-independent inducer of autophagy, inhibits human cytomegalovirus infection in multiple cell types. J. Virol. 90, 1259–1277. doi: 10.1128/JVI.02651-15
Benaroudj, N., Lee, D. H., Goldberg, A. L. (2001). Trehalose accumulation during cellular stress protects cells and cellular proteins from damage by oxygen radicals. J. Biol. Chem. 276, 24261–24267. doi: 10.1074/jbc.M101487200
Bhardwaj, V., He, J. (2020). Reactive oxygen species, metabolic plasticity, and drug resistance in cancer. Int. J. Mol. Sci. 21, 3412. doi: 10.3390/ijms21103412
Bhatt, S., Gething, P. W., Brady, O. J., Messina, J. P., Farlow, A. W., Moyes, C. L., et al. (2013). The global distribution and burden of dengue. Nature 496, 504–507. doi: 10.1038/nature12060
Bobrovskikh, M. A., Gruntenko, N. E. (2023). Mechanisms of neuroendocrine stress response in drosophila and its effect on carbohydrate and lipid metabolism. Insects 14, 474. doi: 10.3390/insects14050474
Brackney, D. E. (2017). Implications of autophagy on arbovirus infection of mosquitoes. Curr. Opin. Insect Sci. 22, 1–6. doi: 10.1016/j.cois.2017.05.001
Broughton, S. J., Piper, M. D. W., Ikeya, T., Bass, T. M., Jacobson, J., Driege, Y., et al. (2005). Longer lifespan, altered metabolism, and stress resistance in Drosophila from ablation of cells making insulin-like ligands. Proc. Natl. Acad. Sci. 102, 3105–3110. doi: 10.1073/pnas.0405775102
Caragata, E. P., Dutra, H. L. C., Sucupira, P. H. F., Ferreira, A. G. A., Moreira, L. A. (2021). Wolbachia as translational science: controlling mosquito-borne pathogens. Trends Parasitol. 37, 1050–1067. doi: 10.1016/j.pt.2021.06.007
Caragata, E. P., Pais, F. S., Baton, L. A., Silva, J. B. L., Sorgine, M. H. F., Moreira, L. A. (2017). The transcriptome of the mosquito Aedes fluviatilis (Diptera: Culicidae), and transcriptional changes associated with its native Wolbachia infection. BMC Genomics 18, 6. doi: 10.1186/s12864-016-3441-4
Chaitanya, R. K., Shashank, K., Sridevi, P. (2016). “Oxidative stress in invertebrate systems,” in Free Radicals and Diseases. Ed. Ahmad, R. (London, UK: InTech). doi: 10.5772/64573
Currin-Ross, D., Husdell, L., Pierens, G. K., Mok, N. E., O’Neill, S. L., Schirra, H. J., et al. (2021). The metabolic response to infection with wolbachia implicates the insulin/insulin-like-growth factor and hypoxia signaling pathways in drosophila melanogaster. Front. Ecol. Evol. 9. doi: 10.3389/fevo.2021.623561
Darabi, S., Noori-Zadeh, A., Abbaszadeh, H. A., Rajaei, F. (2018). Trehalose activates autophagy and prevents hydrogen peroxide-induced apoptosis in the bone marrow stromal cells. Iran. J. Pharm. Res. 17 (3), 1141–1149. doi: 10.22037/ijpr.2018.2277
Deehan, M., Lin, W., Blum, B., Emili, A., Frydman, H. (2021). Intracellular Density of Wolbachia Is Mediated by Host Autophagy and the Bacterial Cytoplasmic Incompatibility Gene cifB in a Cell Type-Dependent Manner in Drosophila melanogaster. mBio 12, e02205–e02220. doi: 10.1128/mBio.02205-20
Dodson, B. L., Hughes, G. L., Paul, O., Matacchiero, A. C., Kramer, L. D., Rasgon, J. L. (2014). Wolbachia enhances west nile virus (WNV) infection in the mosquito culex tarsalis. PloS Negl. Trop. Dis. 8, e2965. doi: 10.1371/journal.pntd.0002965
Echavarria-Consuegra, L., Smit, J. M., Reggiori, F. (2019). Role of autophagy during the replication and pathogenesis of common mosquito-borne flavi- and alphaviruses. Open Biol. 9, 190009. doi: 10.1098/rsob.190009
Felton, G. W., Duffey, S. S. (1992). Ascorbate oxidation reduction in Helicoverpa zea as a scavenging system against dietary oxidants. Arch. Insect Biochem. Physiol. 19, 27–37. doi: 10.1002/arch.940190104
Felton, G. W., Summers, C. B. (1995). Antioxidant systems in insects. Arch. Insect Biochem. Physiol. 29, 187–197. doi: 10.1002/arch.940290208
Ferree, P. M., Frydman, H. M., Li, J. M., Cao, J., Wieschaus, E., Sullivan, W. (2005). Wolbachia utilizes host microtubules and dynein for anterior localization in the drosophila oocyte. PloS Pathog. 1, e14. doi: 10.1371/journal.ppat.0010014
García, M., Argüelles, J. (2021). Trehalase inhibition by validamycin A may be a promising target to design new fungicides and insecticides. Pest Manage. Sci. 77, 3832–3835. doi: 10.1002/ps.6382
Gill, A. C., Darby, A. C., Makepeace, B. L. (2014). Iron necessity: the secret of wolbachia’s success? PloS Negl. Trop. Dis. 8, e3224. doi: 10.1371/journal.pntd.0003224
Glaser, R. L., Meola, M. A. (2010). The Native Wolbachia Endosymbionts of Drosophila melanogaster and Culex quinquefasciatus Increase Host Resistance to West Nile Virus Infection. PloS One 5, e11977. doi: 10.1371/journal.pone.0011977
Glick, D., Barth, S., Macleod, K. F. (2010). Autophagy: cellular and molecular mechanisms. J. Pathol. 221, 3–12. doi: 10.1002/path.2697
Hargitai, D., Kenéz, L., Al-Lami, M., Szenczi, G., Lőrincz, P., Juhász, G. (2022). Autophagy controls Wolbachia infection upon bacterial damage and in aging Drosophila. Front. Cell Dev. Biol. 10. doi: 10.3389/fcell.2022.976882
Heaton, N. S., Randall, G. (2011). Dengue virus and autophagy. Viruses 3, 1332–1341. doi: 10.3390/v3081332
Hedges, L. M., Brownlie, J. C., O’Neill, S. L., Johnson, K. N. (2008). Wolbachia and virus protection in insects. Science 322, 702–702. doi: 10.1126/science.1162418
Hertig, M., Wolbach, S. B. (1924). Studies on rickettsia-like micro-organisms in insects. J. Med. Res. 44, 329–374.7.
Hilgenfeld, R., Vasudevan, S. G. (2018). Dengue and Zika: Control and Antiviral Treatment Strategies, Advances in Experimental Medicine and Biology (Singapore: Springer Singapore). doi: 10.1007/978-981-10-8727-1
Honma, Y., Sato-Morita, M., Katsuki, Y., Mihara, H., Baba, R., Harada, M. (2018). Trehalose activates autophagy and decreases proteasome inhibitor-induced endoplasmic reticulum stress and oxidative stress-mediated cytotoxicity in hepatocytes. Hepatol. Res. 48, 94–105. doi: 10.1111/hepr.12892
Huang, J.-H., Bellés, X., Lee, H.-J. (2012). Functional characterization of hypertrehalosemic hormone receptor in relation to hemolymph trehalose and to oxidative stress in the cockroach blattella germanica. Front. Endocrinol. 2. doi: 10.3389/fendo.2011.00114
Huang, Q., Ma, Q., Li, F., Zhu-Salzman, K., Cheng, W. (2022). Metabolomics Reveals Changes in Metabolite Profiles among Pre-Diapause, Diapause and Post-Diapause Larvae of Sitodiplosis mosellana (Diptera: Cecidomyiidae). Insects 13, 339. doi: 10.3390/insects13040339
Ikeya, T., Broughton, S., Alic, N., Grandison, R., Partridge, L. (2009). The endosymbiont Wolbachia increases insulin/IGF-like signalling in Drosophila. Proc. R. Soc B Biol. Sci. 276, 3799–3807. doi: 10.1098/rspb.2009.0778
Jain, A., Rusten, T. E., Katheder, N., Elvenes, J., Bruun, J.-A., Sjøttem, E., et al. (2015). p62/sequestosome-1, autophagy-related gene 8, and autophagy in drosophila are regulated by nuclear factor erythroid 2-related factor 2 (NRF2), independent of transcription factor TFEB. J. Biol. Chem. 290, 14945–14962. doi: 10.1074/jbc.M115.656116
Jiménez, N. E., Gerdtzen, Z. P., Olivera-Nappa, Á., Salgado, J. C., Conca, C. (2019). A systems biology approach for studying Wolbachia metabolism reveals points of interaction with its host in the context of arboviral infection. PloS Negl. Trop. Dis. 13, e0007678. doi: 10.1371/journal.pntd.0007678
Jo, Y. H., Lee, J. H., Patnaik, B. B., Keshavarz, M., Lee, Y. S., Han, Y. S. (2021). Autophagy in tenebrio molitor immunity: conserved antimicrobial functions in insect defenses. Front. Immunol. 12. doi: 10.3389/fimmu.2021.667664
Kanamori, Y., Saito, A., Hagiwara-Komoda, Y., Tanaka, D., Mitsumasu, K., Kikuta, S., et al. (2010). The trehalose transporter 1 gene sequence is conserved in insects and encodes proteins with different kinetic properties involved in trehalose import into peripheral tissues. Insect Biochem. Mol. Biol. 40, 30–37. doi: 10.1016/j.ibmb.2009.12.006
Kazek, M., Chodáková, L., Lehr, K., Strych, L., Nedbalová, P., McMullen, E., et al. (2024). Glucose and trehalose metabolism through the cyclic pentose phosphate pathway shapes pathogen resistance and host protection in Drosophila. PloS Biol. 22, e3002299. doi: 10.1371/journal.pbio.3002299
Kodrík, D., Bednářová, A., Zemanová, M., Krishnan, N. (2015). Hormonal regulation of response to oxidative stress in insects—An update. Int. J. Mol. Sci. 16, 25788–25816. doi: 10.3390/ijms161025788
Kuo, C.-J., Hansen, M., Troemel, E. (2018). Autophagy and innate immunity: Insights from invertebrate model organisms. Autophagy 14, 233–242. doi: 10.1080/15548627.2017.1389824
LaBeaud, A. D., Bashir, F., King, C. H. (2011). Measuring the burden of arboviral diseases: the spectrum of morbidity and mortality from four prevalent infections. Popul. Health Metr. 9, 1. doi: 10.1186/1478-7954-9-1
Landmann, F. (2019). The wolbachia endosymbionts. Microbiol. Spectr. 7, 7.2.25. doi: 10.1128/microbiolspec.BAI-0018-2019
Lee, J., Jeong, B., Bae, H. R., Jang, H. A., Kim, J. K. (2023). Trehalose Biosynthesis Gene otsA Protects against Stress in the Initial Infection Stage of Burkholderia -Bean Bug Symbiosis. Microbiol. Spectr. 11, e03510–e03522. doi: 10.1128/spectrum.03510-22
Li, R., Xiao, Y., Li, K., Tian, L. (2022). Transcription and post-translational regulation of autophagy in insects. Front. Physiol. 13. doi: 10.3389/fphys.2022.825202
Lindsey, A. R. I. (2020). Sensing, signaling, and secretion: A review and analysis of systems for regulating host interaction in wolbachia. Genes 11, 813. doi: 10.3390/genes11070813
Lindsey, A. R. I., Bhattacharya, T., Hardy, R. W., Newton, I. L. G. (2021). Wolbachia and virus alter the host transcriptome at the interface of nucleotide metabolism pathways. mBio 12, e03472–e03420. doi: 10.1128/mBio.03472-20
Lindsey, A., Bhattacharya, T., Newton, I., Hardy, R. (2018). Conflict in the intracellular lives of endosymbionts and viruses: A mechanistic look at wolbachia-mediated pathogen-blocking. Viruses 10, 141. doi: 10.3390/v10040141
Liu, K., Dong, Y., Huang, Y., Rasgon, J. L., Agre, P. (2013). Impact of trehalose transporter knockdown on Anopheles Gambiae stress adaptation and susceptibility to Plasmodium falciparum infection. Proc. Natl. Acad. Sci. 110, 17504–17509. doi: 10.1073/pnas.1316709110
Lu, K., Wang, Y., Chen, X., Zhang, X., Li, W., Cheng, Y., et al. (2019). Adipokinetic hormone receptor mediates trehalose homeostasis to promote vitellogenin uptake by oocytes in nilaparvata lugens. Front. Physiol. 9. doi: 10.3389/fphys.2018.01904
Luo, Y., Li, W.-M., Wang, W. (2008). Trehalose: Protector of antioxidant enzymes or reactive oxygen species scavenger under heat stress? Environ. Exp. Bot. 63, 378–384. doi: 10.1016/j.envexpbot.2007.11.016
Mahanta, D. K., Bhoi, T. K., Komal, J., Samal, I., Nikhil, R. M., Paschapur, A. U., et al. (2023). Insect-pathogen crosstalk and the cellular-molecular mechanisms of insect immunity: uncovering the underlying signaling pathways and immune regulatory function of non-coding RNAs. Front. Immunol. 14. doi: 10.3389/fimmu.2023.1169152
Martemucci, G., Costagliola, C., Mariano, M., D’andrea, L., Napolitano, P., D’Alessandro, A. G. (2022). Free radical properties, source and targets, antioxidant consumption and health. Oxygen 2, 48–78. doi: 10.3390/oxygen2020006
Matassini, C., Parmeggiani, C., Cardona, F. (2020). New frontiers on human safe insecticides and fungicides: an opinion on trehalase inhibitors. Molecules 25, 3013. doi: 10.3390/molecules25133013
Matsuda, H., Yamada, T., Yoshida, M., Nishimura, T. (2015). Flies without trehalose. J. Biol. Chem. 290, 1244–1255. doi: 10.1074/jbc.M114.619411
Mayoral, J. G., Etebari, K., Hussain, M., Khromykh, A. A., Asgari, S. (2014). Wolbachia infection modifies the profile, shuttling and structure of microRNAs in a mosquito cell line. PloS One 9, e96107. doi: 10.1371/journal.pone.0096107
McGraw, E. (2004). Wolbachia pipientis: intracellular infection and pathogenesis in Drosophila. Curr. Opin. Microbiol. 7, 67–70. doi: 10.1016/j.mib.2003.12.003
Mejia, A. J., Dutra, H. L. C., Jones, M. J., Perera, R., McGraw, E. A. (2022). Cross-tissue and generation predictability of relative Wolbachia densities in the mosquito Aedes aEgypti. Parasitol. Vectors 15, 128. doi: 10.1186/s13071-022-05231-9
Melnikow, E., Xu, S., Liu, J., Bell, A. J., Ghedin, E., Unnasch, T. R., et al. (2013). A potential role for the interaction of wolbachia surface proteins with the brugia malayi glycolytic enzymes and cytoskeleton in maintenance of endosymbiosis. PloS Negl. Trop. Dis. 7, e2151. doi: 10.1371/journal.pntd.0002151
Mizunoe, Y., Kobayashi, M., Sudo, Y., Watanabe, S., Yasukawa, H., Natori, D., et al. (2018). Trehalose protects against oxidative stress by regulating the Keap1–Nrf2 and autophagy pathways. Redox Biol. 15, 115–124. doi: 10.1016/j.redox.2017.09.007
Molloy, J. C., Sommer, U., Viant, M. R., Sinkins, S. P. (2016). Wolbachia modulates lipid metabolism in aedes albopictus mosquito cells. Appl. Environ. Microbiol. 82, 3109–3120. doi: 10.1128/AEM.00275-16
Newton, I. L. G., Rice, D. W. (2020). The jekyll and hyde symbiont: could wolbachia be a nutritional mutualist? J. Bacteriol 202, e00589-19. doi: 10.1128/JB.00589-19
Pan, X., Zhou, G., Wu, J., Bian, G., Lu, P., Raikhel, A. S., et al. (2012). Wolbachia induces reactive oxygen species (ROS)-dependent activation of the Toll pathway to control dengue virus in the mosquito Aedes aEgypti. Proc. Natl. Acad. Sci. 109, E23-31. doi: 10.1073/pnas.1116932108
Peng, H., Guo, D., Peng, H., Guo, H., Wang, H., Wang, Y., et al. (2024). The gene AccCyclin H mitigates oxidative stress by influencing trehalose metabolism in Apis cerana cerana. J. Sci. Food Agric 104, 225–234. doi: 10.1002/jsfa.12900
Ponton, F., Wilson, K., Holmes, A., Raubenheimer, D., Robinson, K. L., Simpson, S. J. (2015). Macronutrients mediate the functional relationship between Drosophila and Wolbachia. Proc. R. Soc B Biol. Sci. 282, 20142029. doi: 10.1098/rspb.2014.2029
Reinink, P., Buter, J., Mishra, V. K., Ishikawa, E., Cheng, T.-Y., Willemsen, P. T. J., et al. (2019). Discovery of Salmonella trehalose phospholipids reveals functional convergence with mycobacteria. J. Exp. Med. 216, 757–771. doi: 10.1084/jem.20181812
Reyes, J. I. L., Suzuki, Y., Carvajal, T., Muñoz, M. N. M., Watanabe, K. (2021). Intracellular interactions between arboviruses and wolbachia in aedes aEgypti. Front. Cell. Infect. Microbiol. 11. doi: 10.3389/fcimb.2021.690087
Reyes-DelaTorre, A., Teresa, M., Rafael, J. (2012). “Carbohydrate Metabolism in Drosophila: Reliance on the Disaccharide Trehalose,” in Carbohydrates - Comprehensive Studies on Glycobiology and Glycotechnology. Ed. Chang, C.-F. (London, UK: InTech). doi: 10.5772/50633
Ross, P. A. (2021). Designing effective Wolbachia release programs for mosquito and arbovirus control. Acta Trop. 222, 106045. doi: 10.1016/j.actatropica.2021.106045
Sheehan, K. B., Martin, M., Lesser, C. F., Isberg, R. R., Newton, I. L. G. (2016). Identification and characterization of a candidate wolbachia pipientis type IV effector that interacts with the actin cytoskeleton. mBio 7, e00622–e00616. doi: 10.1128/mBio.00622-16
Shepard, D. S., Undurraga, E. A., Halasa, Y. A., Stanaway, J. D. (2016). The global economic burden of dengue: a systematic analysis. Lancet Infect. Dis. 16, 935–941. doi: 10.1016/S1473-3099(16)00146-8
Shukla, E., Thorat, L. J., Nath, B. B., Gaikwad, S. M. (2015). Insect trehalase: Physiological significance and potential applications. Glycobiology 25, 357–367. doi: 10.1093/glycob/cwu125
Souza-Neto, J. A., Sim, S., Dimopoulos, G. (2009). An evolutionary conserved function of the JAK-STAT pathway in anti-dengue defense. Proc. Natl. Acad. Sci. 106, 17841–17846. doi: 10.1073/pnas.0905006106
Tamayo, B., Kercher, K., Vosburg, C., Massimino, C., Jernigan, M. R., Hasan, D. L., et al. (2022). Annotation of glycolysis, gluconeogenesis, and trehaloneogenesis pathways provide insight into carbohydrate metabolism in the Asian citrus psyllid. Gigabyte 2022, 1–19. doi: 10.46471/gigabyte.41
Tellis, M. B., Kotkar, H. M., Joshi, R. S. (2023). Regulation of trehalose metabolism in insects: from genes to the metabolite window. Glycobiology 33, 262–273. doi: 10.1093/glycob/cwad011
Terradas, G., Joubert, D. A., McGraw, E. A. (2017). The RNAi pathway plays a small part in Wolbachia-mediated blocking of dengue virus in mosquito cells. Sci. Rep. 7, 43847. doi: 10.1038/srep43847
Terradas, G., McGraw, E. A. (2017). Wolbachia -mediated virus blocking in the mosquito vector Aedes aEgypti. Curr. Opin. Insect Sci. 22, 37–44. doi: 10.1016/j.cois.2017.05.005
Thompson, S. N. (2003). Trehalose – the insect ‘Blood’ Sugar. Adv. Insect Physiol. Elsevier 31, 205–285. doi: 10.1016/S0065-2806(03)31004-5
Thorat, L. J., Gaikwad, S. M., Nath, B. B. (2012). Trehalose as an indicator of desiccation stress in Drosophila melanogaster larvae: A potential marker of anhydrobiosis. Biochem. Biophys. Res. Commun. 419, 638–642. doi: 10.1016/j.bbrc.2012.02.065
Thorat, L., Mani, K.-P., Thangaraj, P., Chatterjee, S., Nath, B. B. (2016). Downregulation of dTps1 in Drosophila melanogaster larvae confirms involvement of trehalose in redox regulation following desiccation. Cell Stress Chaperones 21, 285–294. doi: 10.1007/s12192-015-0658-0
Tracy, K., Baehrecke, E. H. (2013). The role of autophagy in drosophila metamorphosis, in: current topics in developmental biology. Elsevier pp, 101–125. doi: 10.1016/B978-0-12-385979-2.00004-6
Vanaporn, M., Titball, R. W. (2020). Trehalose and bacterial virulence. Virulence 11, 1192–1202. doi: 10.1080/21505594.2020.1809326
Van Den Berg, H., Da Silva Bezerra, H. S., Al-Eryani, S., Chanda, E., Nagpal, B. N., Knox, T. B., et al. (2021). Recent trends in global insecticide use for disease vector control and potential implications for resistance management. Sci. Rep. 11, 23867. doi: 10.1038/s41598-021-03367-9
Voronin, D., Bachu, S., Shlossman, M., Unnasch, T. R., Ghedin, E., Lustigman, S. (2016). Glucose and glycogen metabolism in brugia malayi is associated with wolbachia symbiont fitness. PloS One 11, e0153812. doi: 10.1371/journal.pone.0153812
Voronin, D., Schnall, E., Grote, A., Jawahar, S., Ali, W., Unnasch, T. R., et al. (2019). Pyruvate produced by Brugia spp. via glycolysis is essential for maintaining the mutualistic association between the parasite and its endosymbiont, Wolbachia. PloS Pathog. 15, e1008085. doi: 10.1371/journal.ppat.1008085
Weinert, L. A., Araujo-Jnr, E. V., Ahmed, M. Z., Welch, J. J. (2015). The incidence of bacterial endosymbionts in terrestrial arthropods. Proc. R. Soc B Biol. Sci. 282, 20150249. doi: 10.1098/rspb.2015.0249
WHO (2023a). Dengue and severe dengue. Available online at: https://www.who.int/news-room/fact-sheets/detail/dengue-and-severe-dengue. (Accessed October 5, 2024)
WHO (2023b). Dengue - Global situation. Available online at: https://www.who.int/emergencies/disease-outbreak-news/item/2023-DON498. (Accessed February 23, 2024)
Williams, B., Njaci, I., Moghaddam, L., Long, H., Dickman, M. B., Zhang, X., et al. (2015). Trehalose accumulation triggers autophagy during plant desiccation. PloS Genet. 11, e1005705. doi: 10.1371/journal.pgen.1005705
Wu, H., Wang, M. C., Bohmann, D. (2009). JNK protects Drosophila from oxidative stress by trancriptionally activating autophagy. Mech. Dev. 126, 624–637. doi: 10.1016/j.mod.2009.06.1082
Xi, Z., Gavotte, L., Xie, Y., Dobson, S. L. (2008). Genome-wide analysis of the interaction between the endosymbiotic bacterium Wolbachia and its Drosophila host. BMC Genomics 9, 1. doi: 10.1186/1471-2164-9-1
Xu, C., Chen, X., Sheng, W.-B., Yang, P. (2019). Trehalose restores functional autophagy suppressed by high glucose. Reprod. Toxicol. 85, 51–58. doi: 10.1016/j.reprotox.2019.02.005
Xu, J., Sheng, Z., Palli, S. R. (2013). Juvenile hormone and insulin regulate trehalose homeostasis in the red flour beetle, tribolium castaneum. PloS Genet. 9, e1003535. doi: 10.1371/journal.pgen.1003535
Yang, Y., Liang, Y., Zhi, J., Li, D., Li, C. (2024). Regulatory effect of trehalose metabolism on chitin synthesis in Spodoptera frugiperda (J. E. Smith) (Lepidoptera: Noctuidae) as determined using RNAi. J. Asia-Pac. Entomol. 27, 102179. doi: 10.1016/j.aspen.2023.102179
Yasugi, T., Yamada, T., Nishimura, T. (2017). Adaptation to dietary conditions by trehalose metabolism in Drosophila. Sci. Rep. 7, 1619. doi: 10.1038/s41598-017-01754-9
Ye, Y. H., Woolfit, M., Rancès, E., O’Neill, S. L., McGraw, E. A. (2013). Wolbachia-associated bacterial protection in the mosquito aedes aEgypti. PloS Negl. Trop. Dis. 7, e2362. doi: 10.1371/journal.pntd.0002362
Yu, L., Chen, X., Wei, Y., Ding, Y., Wang, Q., Wang, S., et al. (2020). Effects of long-term cadmium exposure on trehalose metabolism, growth, and development of Aedes albopictus (Diptera: Culicidae). Ecotoxicol. Environ. Saf. 204, 111034. doi: 10.1016/j.ecoenv.2020.111034
Zeng, B., Wang, S., Li, Y., Xiao, Z., Zhou, M., Wang, S., et al. (2020). Effect of long-term cold storage on trehalose metabolism of pre-wintering Harmonia axyridis adults and changes in morphological diversity before and after wintering. PloS One 15, e0230435. doi: 10.1371/journal.pone.0230435
Zhang, H.-B., Cao, Z., Qiao, J.-X., Zhong, Z.-Q., Pan, C.-C., Liu, C., et al. (2021). Metabolomics provide new insights into mechanisms of Wolbachia-induced paternal defects in Drosophila melanogaster. PloS Pathog. 17, e1009859. doi: 10.1371/journal.ppat.1009859
Zhang, G., Hussain, M., O’Neill, S. L., Asgari, S. (2013). Wolbachia uses a host microRNA to regulate transcripts of a methyltransferase, contributing to dengue virus inhibition in Aedes aEgypti. Proc. Natl. Acad. Sci. 110, 10276–10281. doi: 10.1073/pnas.1303603110
Zhang, S., Qiu, X., Zhang, Y., Huang, C., Lin, D. (2023). Metabolomic analysis of trehalose alleviating oxidative stress in myoblasts. Int. J. Mol. Sci. 24, 13346. doi: 10.3390/ijms241713346
Zouache, K., Voronin, D., Tran-Van, V., Mousson, L., Failloux, A.-B., Mavingui, P. (2009). Persistent wolbachia and cultivable bacteria infection in the reproductive and somatic tissues of the mosquito vector aedes albopictus. PloS One 4, e6388. doi: 10.1371/journal.pone.0006388
Keywords: trehalose, Wolbachia, pathogen-blocking effect, Aedes aegypti, antioxidant, autophagy
Citation: Dupuis B, Pocquet N and Failloux A-B (2025) Understanding the role of trehalose in interactions between Wolbachia and Aedes aegypti. Front. Cell. Infect. Microbiol. 15:1547873. doi: 10.3389/fcimb.2025.1547873
Received: 18 December 2024; Accepted: 04 March 2025;
Published: 18 March 2025.
Edited by:
Shengzhang Dong, Johns Hopkins University, United StatesReviewed by:
Amit Sinha, New England Biolabs, United StatesCopyright © 2025 Dupuis, Pocquet and Failloux. This is an open-access article distributed under the terms of the Creative Commons Attribution License (CC BY). The use, distribution or reproduction in other forums is permitted, provided the original author(s) and the copyright owner(s) are credited and that the original publication in this journal is cited, in accordance with accepted academic practice. No use, distribution or reproduction is permitted which does not comply with these terms.
*Correspondence: Anna-Bella Failloux, YW5uYS1iZWxsYS5mYWlsbG91eEBwYXN0ZXVyLmZy
Disclaimer: All claims expressed in this article are solely those of the authors and do not necessarily represent those of their affiliated organizations, or those of the publisher, the editors and the reviewers. Any product that may be evaluated in this article or claim that may be made by its manufacturer is not guaranteed or endorsed by the publisher.
Research integrity at Frontiers
Learn more about the work of our research integrity team to safeguard the quality of each article we publish.