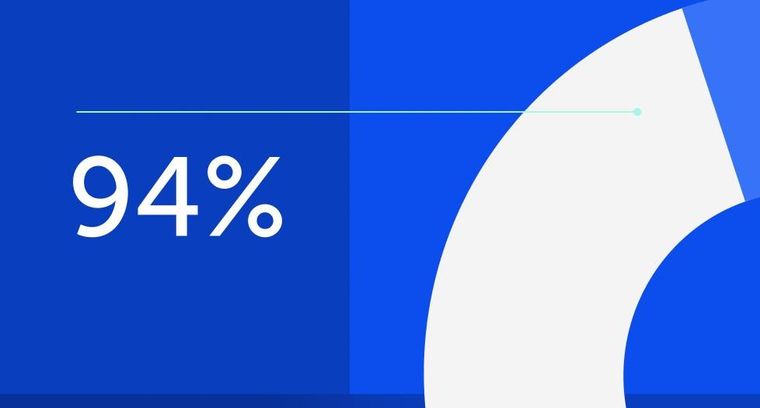
94% of researchers rate our articles as excellent or good
Learn more about the work of our research integrity team to safeguard the quality of each article we publish.
Find out more
ORIGINAL RESEARCH article
Front. Cell. Infect. Microbiol., 05 May 2020
Sec. Fungal Pathogenesis
Volume 10 - 2020 | https://doi.org/10.3389/fcimb.2020.00199
This article is part of the Research TopicSupplement of the 9th Trends in Medical Mycology (TIMM-9)View all 11 articles
Malassezia furfur and Malassezia pachydermatis are lipophilic and lipid dependent yeasts, associated with the skin microbiota in humans and domestic animals, respectively. Although they are commensals, under specific conditions they become pathogens, causing skin conditions, such as pityriasis versicolor, dandruff/seborrheic dermatitis, folliculitis in humans, and dermatitis and otitis in dogs. Additionally, these species are associated with fungemia in immunocompromised patients and low-weight neonates in intensive care units with intravenous catheters or with parenteral nutrition and that are under-treatment of broad-spectrum antibiotics. The host-pathogen interaction mechanism in these yeasts is still unclear; for this reason, it is necessary to implement suitable new host systems, such as Galleria mellonella. This infection model has been widely used to assess virulence, host-pathogen interaction, and antimicrobial activity in bacteria and fungi. Some advantages of the G. mellonella model are: (1) the immune response has phagocytic cells and antimicrobial peptides that are similar to those in the innate immune response of human beings; (2) no ethical implications; (3) low cost; and (4) easy to handle and inoculate. This study aims to establish G. mellonella as an in vivo infection model for M. furfur and M. pachydermatis. To achieve this objective, first, G. mellonella larvae were first inoculated with different inoculum concentrations of these two Malassezia species, 1.5 × 106 CFU/mL, 1.5 × 107 CFU/mL, 1.5 × 108 CFU/mL, and 11.5 × 109 CFU/mL, and incubated at 33 and 37°C. Then, for 15 days, the mortality and melanization were evaluated daily. Finally, the characterization of hemocytes and fungal burden assessment were as carried out. It was found that at 33 and 37°C both M. furfur and M. pachydermatis successfully established a systemic infection in G. mellonella. M. pachydermatis proved to be slightly more virulent than M. furfur at a temperature of 37°C. The results suggest that larvae mortality and melanization is dependent on the specie of Malassezia, the inoculum concentration and the temperature. According to the findings, G. mellonella can be used as an in vivo model of infection to conduct easy and reliable approaches to boost our knowledge of the Malassezia genus.
The genus Malassezia is comprised of 18 lipophilic species characterized as commensal yeasts, which are part of the skin mycobiota of both humans and animals (Nagata et al., 2012; Cabañes, 2014; Cabañes et al., 2016; Honnavar et al., 2016; Prohic et al., 2016), and are commonly located in lipid-rich body areas, such as the scalp, face, and trunk (Gupta et al., 2004; DeAngelis et al., 2005). Some species of this genus have been associated with skin diseases (Gupta et al., 2004; Prohic et al., 2016) and systemic infections (Gaitanis et al., 2012; Kaneko et al., 2012; Nagata et al., 2012; Iatta et al., 2014, 2018; Roman et al., 2016; Lee et al., 2018; Theelen et al., 2018; Chen et al., 2020).
Malassezia furfur and Malassezia pachydermatis are two representative species of this genus which are found mainly in the skin of humans and pets such as dogs and cats, respectively (Cabañes, 2014). These two species have been associated with systemic infections in premature infants (Zomorodain et al., 2008; Iatta et al., 2014, 2018) as well as in low-weight infants with central venous catheters (CVC), parenteral lipid infusions and long hospital stays (Bell et al., 1988; Chang et al., 1998; Gupta et al., 2014), The two species have also been shown to infect immunosuppressed children and adults (Gaitanis et al., 2012; Kaneko et al., 2012; Roman et al., 2016); in fact, in some cases the percentage of fungemia by M. furfur (2.1%) is higher than fungemia by Candida spp. (1.4%) (Iatta et al., 2014), and prevalence can range from 2.1 to 4.4% in a neonatal intensive care unit depending on the diagnostic method (Iatta et al., 2018).
The skin colonization of neonates is the first step for the subsequent systemic invasion (Zomorodain et al., 2008; Iatta et al., 2014), a process that is not yet clear, and it has been reported that this may be facilitated by lipid infusion (Tragiannidis et al., 2010). This colonization has been reported from day zero to day 1 of life (Nagata et al., 2012), and it has been associated with transmission from the mother (Zomorodain et al., 2008; Nagata et al., 2012; Paul et al., 2019), healthcare personnel with pets (Chang et al., 1998), poor hygiene practices by healthcare personnel (handwashing before and after having contact with patients) leading to patient-patient transmission (Chang et al., 1998; Gaitanis et al., 2012), and persistence of M. pachydermatis yeasts in incubators and sheets (Iatta et al., 2014). Additionally, using fluconazole to prevent Candida spp. infections promotes colonization of resistant M. furfur (Chen et al., 2020).
Owing to the increase in the number of cases of M. furfur and M. pachydermatis systemic infections and the lack of knowledge of the infection mechanism, it is important to study the process of the systemic infection in-vivo. For this purpose, it is necessary to implement infection animal models. Different models have been used for the study of fungal diseases, such as zebrafish, chickens, mice, guinea pigs, dogs, and rabbits (McGinnis and Borgers, 1989; Capilla et al., 2007; Rosowski et al., 2018; Schlemmer et al., 2018; Sparber et al., 2019). These animal models have advantages such as the fact that they mimic human infectious diseases; however, their disadvantages are that they have high requirements for their maintenance, handling, space, and ethical considerations, which are the reasons as to why it is pivotal to implement alternatives (Capilla et al., 2007).
Among the alternatives are invertebrate organisms, such as Drosophila melanogaster used as a model for the study of M. pachydermatis infection (Merkel et al., 2018), Caenorhabditis elegans as an infection model of Cryptococcus neoformans (Capilla et al., 2007) and Galleria mellonella, which is widely used as a bacterial (Loh et al., 2013; Lacharme-Lora et al., 2019) and fungal infection model (Eisenman et al., 2014; Amorim-Vaz et al., 2015; Kloezen et al., 2015; Wuensch et al., 2018; Maurer et al., 2019; Sheehan and Kavanagh, 2019). One of the main features of Galleria mellonella that makes it a good model of mycosis are the phagocytic cells, which are the first line of defense against fungal infections (Trevijano-Contador and Zaragoza, 2019).
One of the advantages of using G. mellonella larvae as a model of host-pathogen interaction is the versatility of methods of inoculation, which can be topical (Scully and Bidochka, 2005), oral (Freitak et al., 2014) or the most common, microinjection (Eisenman et al., 2014; Amorim-Vaz et al., 2015; Kloezen et al., 2015; Wuensch et al., 2018; Sheehan and Kavanagh, 2019). Some other advantages include the feasibility of handling larvae due to their size, the fact that it is not necessary to feed them since they stop feeding in the fifth instar when experiments start (Singkum et al., 2019), the exclusion of ethical implications like those used in mammalian models, the ease of following-up on the progress of infection due to the use of standardized health scores (Champion et al., 2018), and the good antifungal immune response that includes cellular and humoral components (Kay et al., 2019; Trevijano-Contador and Zaragoza, 2019).
Due to the aforementioned factors, G. mellonella is an attractive in-vivo model of host-pathogen interaction that has been widely used in the evaluation of the host-pathogen interaction of yeasts such as Candida and Cryptococcus, but there are no reports, so far, on the use of G. mellonella as an infection model for M. furfur or M. pachydermatis. Therefore, the objective of this study is to establish G. mellonella as an infection model for M. furfur and M. pachydermatis, which will allow other mycologists to use this model in future studies. In this work, the survival of G. mellonella inoculated with different inoculum concentrations of M. furfur and M. pachydermatis was evaluated, and also the progress of the infection was followed by monitoring the melanization of the larvae and histological samples.
Strains of M. furfur CBS 1878 and M. pachydermatis CBS 1879 (Westerdijk institute, Utrecht, The Netherlands) were used. The strains were cultured for 72 h in the modified Dixon (mDixon) agar (36 g.L−1 mycosel agar [BD, USA], 20 g L−1 Oxgall [BD, USA], 36 g L−1 malt extract [Oxoid, UK], 2 mL L−1 glycerol [Sigma Aldrich, USA], 2 mL L−1 oleic acid [Sigma Aldrich, USA], and 10 mL L−1 Tween 40 [Sigma Aldrich, USA]) at 33°C. The inoculum was prepared with 5 mL of 0.5% Tween 80 [Sigma Aldrich, USA]. Then it was filtered and cells were counted in a Neubauer chamber to reach desirable inoculum concentrations: 1.5 × 106 CFU/mL, 1.5 × 107 CFU/mL, 1.5 × 108 CFU/mL, and 1.5 × 109 CFU/mL.
Killing assays were performed in G. mellonella as previously described (Stephens, 1962; Fallon et al., 2012). Briefly, the larvae in late stages (fifth and sixth instar) were obtained from Perkins LTDA (Palmira, Colombia). Once the larvae arrived, they were kept at room temperature for 24 h, in that order, they could recover until challenge assay; then, in continuous movement, the larvae were decontaminated in 0.1% sodium hypochlorite for 30 s and rinsed in two different containers with sterile distilled water, in the first recipient for 30 s and the second recipient for 10 s. Larvae with a weight between 250 and 330 mg and a size of ~2 cm were selected and sorted in five different treatment groups of fifteen larvae, avoiding those with any kind of mark. Insulin syringes (BD Veo insulin syringe with the BD Ultra-Fine 6 mm needle) were used to microinject the larvae, holding them between the fingers, allowing the needle to insert at the last left proleg to deliver the inoculum directly into the hemocoel. Fifteen larvae for each treatment were inoculated with 20 μL of 0.5% Tween 80 as a negative control, and four inoculum concentrations (range 1.5 × 106-1.5 × 109 CFU/mL) Additionally, fifteen larvae were used as absolute control; these larvae were kept in the same conditions as the other five groups, but without any intervention to evaluate each lot of larvae that was received. After that, larvae were incubated at 33 and 37°C in darkness. Every 24 h during a 15 days period, larval silk was removed and the insects' death and melanization were recorded (Amorim-Vaz et al., 2015; Maurer et al., 2019). Larvae were scored as dead when they did not respond to a mechanical stimulus (Eisenman et al., 2014; Cools et al., 2019). Melanization was scored according to a modified health index scoring system (Figure 1) (Loh et al., 2013; Tsai et al., 2016; Champion et al., 2018; Kay et al., 2019). To compare mortality, three biological replicates were performed.
Figure 1. Melanization scores based on the health index scoring system. (A) melanization score 4, no melanization; (B) melanization score 3, <3 spots on beige larva (black arrows showing the spots); (C) melanization score 2, more than three spots on beige larva; (D) melanization score 1, black spots on brown larva; and (E) melanization score 0, full melanization.
To characterize hemocytes, two larvae from each treatment were collected at random, then a puncture in the last segment of the insect was made, allowing a drop of hemolymph to drain over a glass slide. The hemolymph drop was then spread over and stained with Wright's stain. According to previous reports (Arteaga Blanco et al., 2017; Wojda, 2017; Boguś et al., 2018), hemocytes were characterized and counted under a light Leica DM500 microscope.
To access the fungal burden, three larvae per treatment were randomly collected. Their surfaces were cleaned by submerging the larvae in 0.1% sodium hypochlorite for 5 min and rinsing them twice in sterile distilled water for 1 min each time. Then, each larva was placed in 2 mL Eppendorf tubes with 1 mL of 0.5% Tween 80 each and ground for 5 min (Mylonakis et al., 2005). 100 μL of these solutions were used to make two serial dilutions, which were plated on mDixon agar at 33°C. The numbers of colony-forming units (CFU) were counted daily until no new colonies were observed.
On day 5, two random larvae incubated at 37°C were fixed for 10 days at 4°C in 4% paraformaldehyde in PBS (4% PFA/BS), also these larvae were injected in the left proleg with 10 μL of 4% PFA/PBS (Perdoni et al., 2014; Kloezen et al., 2015; Wuensch et al., 2018). After 10 days, they were transferred to 70% ethanol for storage (Eisenman et al., 2014; Maurer et al., 2019). Before the paraffin protocol, larvae were dissected and observed under a Leica Zoom TM2000 stereomicroscope; then, these larvae were embedded in paraffin, cut in sections of 5 μm and stained with hematoxylin-eosin. Nodules of larvae infected with M. furfur and M. pachydermatis inoculum concentration of 1.5 × 109 CFU/mL were dissected apart and stained with calcofluor white [Sigma Aldrich, USA] and 2 mL of 10% KOH [Sigma Aldrich, USA] as described elsewhere (Sheehan and Kavanagh, 2019). Then, the nodules were observed under an Olympus FV1000 laser scanning confocal microscope at the microscopy core, μ-core, at the Universidad de los Andes.
All experiments were performed on three independent biological replicates; survival curves were constructed using the method of Kaplan and Meier, then the curves were compared using the Log-Rank (Mantel-Cox) test. Fungal burden, melanization score, and hemocyte count analysis were conducted using the 2-way ANOVA test. Statistical models were constructed and analyzed using the GraphPad Prism 8 software (version 8.2.0). A p-value under 0.05 was considered to be statistically significant.
To determine the virulence of M. furfur and M. pachydermatis at 33 and 37°C, larvae were inoculated with four different inoculum concentrations. The first temperature (33°C) was used to identify whether M. furfur and M pachydermatis could establish a systemic infection in G. mellonella. At 33°C, it was found that G. mellonella larvae showed a differential survival behavior depending on the inoculum concentration (Figures 2A,B). Larvae inoculated with an inoculum concentration of 1.5 × 109 CFU/mL of the two species of Malassezia showed significantly decreased survival (p < 0.0001, log-rank [Mantel-Cox] test). In the case of larvae infected with M. furfur, 50% of mortality was reached on day 13 (Figure 2A), while larvae infected with M. pachydermatis reached 50% of mortality on day 12 (Figure 2B).
Figure 2. Survival curves of G. mellonella infected with M. furfur and M. pachydermatis. (A) The survival curve of G. mellonella infected with M. furfur and incubated at 33°C, 50% of mortality is reached on day 13. (B) The survival curve of G. mellonella infected with M. pachydermatis and incubated at 33°C, 50% of mortality is reached at day 12. (C) The survival curve of G. mellonella infected with M. furfur and incubated at 37°C, 50% of mortality is reached at day 13. (D) The survival curve of G. mellonella infected with M. pachydermatis and incubated at 37°C, 50% of mortality is reached at day 12. In general, larvae infected with an inoculum of 1.5 × 109 CFU/mL had higher mortality (p < 0.0001, log-rank (Mantel-Cox) test). (E) Comparison of survival curves of larvae infected with inoculum of 1.5 × 109 CFU/mL of M. furfur and M. pachydermatis incubated at 33 and 37°C, no significant differences were found between the four treatments (p = 0.2046, log-rank (Mantel-Cox) test). In all cases, survival depended on inoculum concentration. Mf, M. furfur; Mp, M. Pachydermatis; Gm, G. mellonella.
Survival of control larvae and larvae infected with the remaining three concentrations of M. furfur and M. pachydermatis (1.5 × 106, 1.5 × 107, and 1.5 × 108 CFU/mL) exhibited different patterns; larvae infected with M. furfur showed that at a lower concentration the larval survival increases, but it is still lower compared to the control larval survival (p = 0.014, log-rank [(Mantel-Cox]) test). In the case of larvae infected with M. pachydermatis, no significant difference in larval survival was found between control larvae and inoculum concentration of 1.5 × 106 CFU/mL, and for the two remaining inoculum concentrations (1.5 × 107 and 1.5 × 108 CFU/mL), it was observed that larval survival decreased (p < 0.0001, log-rank [(Mantel-Cox]) test). After, it was demonstrated that M. furfur and M. pachydermatis were able to establish a systemic infection in G. mellonella larvae at 33°C, experiments were conducted at 37°C to evaluate the systemic infection at the human corporal temperature.
Larvae infection assays conducted at 37°C showed a decrease in survival (Figures 2C,D). Since day one, dead larvae were found in challenged larvae groups infected with different inoculum concentrations of M. furfur and M. pachydermatis. A significant decrease in larval survival was observed in larvae infected with M. furfur inoculum concentration of 1.5 × 109 CFU/mL (p < 0.0001, log-rank (Mantel-Cox) test), reaching 50% of mortality on day 13, and larval death was observed since day 1. However, no larvae were reported dead from day 6 until day 10 (Figure 2C). In the case of larvae infected with M. pachydermatis, a significant difference in survival was found (p < 0.0001, log-rank [Mantel-Cox] test); larvae inoculated with the highest inoculum concentration (1.5 × 109 CFU/mL) reached 50% of larval mortality on day 11, and larval death paused from 7 to day 10 (Figure 2D).
Control larvae and larvae inoculated with M. furfur inoculum concentrations of 1.5 × 106, 1.5 × 107, and 1.5 × 108 CFU/mL did not show a significant difference in survival (p = 0.5439, log-rank [(Mantel-Cox]) test). On the other hand, larvae inoculated with M. pachydermatis showed a difference in survival when comparing the larval survival of those infected with the three remaining inoculum concentrations and the control larvae (p = 0.0010, log-rank [(Mantel-Cox]) test). A higher survival in control larvae and larvae inoculated with inoculum concentration of 1.5 × 106 CFU/mL than in larvae with inoculum concentrations of 1.5 × 107 and 1.5 × 108 CFU/mL was found. The resulting data shows that survival at 37°C also depends on inoculum concentration. Survival curves of larvae inoculated with M. furfur and M. pachydermatis inoculum concentration of 1.5 × 109 CFU/mL and incubated at 33 and 37°C were compared (Figure 2E), and no significant difference was found (p = 0.2046, log-rank [(Mantel-Cox]) test).
The development of nodules with deposition of melanin is one of the main components of the immune response of G. mellonella. These nodules are visible macroscopically as brown spots, and in order to follow the progression of infection, the development of melanin nodules was monitored at 37°C. According to the scoring system used in this study, the melanization depends on time and the inoculum concentration of M. furfur and M. pachydermatis (Figures 3A,B). Melanization in larvae infected with both species of Malassezia showed to increase over time, with a significant decrease in the melanization score (p < 0.0001, 2-way ANOVA test). This melanization score showed a significant difference between control larvae and inoculated larvae (p < 0.0001, 2-way ANOVA test). The development of brown spots increased proportionally with the increase of inoculum concentration. The lowest melanization score was recorded in larvae inoculated with the highest concentration of both species of Malassezia (the melanization score range from 2 to 1). Finally, for M. furfur, it was observed that larvae incubated at 33°C exhibited higher melanization than those incubated at 37°C (Figures 3C–F).
Figure 3. Average melanization scores of larvae infected with M. furfur and M. pachydermatis at 37°C and melanization comparison between G. mellonella infected with M. furfur at 33 and 37°C on day 7. As can be seen, melanization score depends on time and treatment; scores decreased as inoculum concentrations increase (p < 0.0001, 2-way ANOVA test). (A) Larvae infected with M. furfur. (B) Larvae infected with M. pachydermatis, moreover in (C) G. mellonella infected with 1.5 × 108 CFU/mL inoculum concentration of M. furfur and incubated at 37°C, melanization was scored as 2 with beige larvae; but in (D) G. mellonella infected with 1.5 × 108 CFU/mL inoculum concentration of M. furfur and incubated at 33°C, melanization score was recorded as two with darker larvae. Finally, in (E) G. mellonella infected with 1.5 × 109 CFU/mL inoculum concentration of M. furfur and incubated at 37°C showed larvae with lower melanization than (F) G. mellonella larvae infected with 1.5 × 109 CFU/mL inoculum concentration of M. furfur and incubated at 33°C. Larval melanization is higher in larvae incubated at 33°C.
Hemocyte population percentages were obtained and tabulated to characterize the immune response of G. mellonella and determine whether or not the infection of the two species of Malassezia has an effect on hemocyte populations. Cell classification was carried out based on size and morphology (Figure 4) (Arteaga Blanco et al., 2017; Wojda, 2017; Boguś et al., 2018). In both cases of M. furfur and M. pachydermatis infection assays at 33 or 37°C, a significant difference was found between cell populations (p < 0.0001, 2-way ANOVA test). No significant differences were found between any of the five treatments at 33 and 37°C (for larvae infected with M. furfur incubated at 33°C, p = 0.8076; at 37°C p = 0.9582 and for larvae infected with M. pachydermatis at 33°C, p = 0.9999; at 37°C, p = 0.9907, 2-way ANOVA test). However, the interaction between type of hemocytes and the treatment showed a significant difference in the percentage of hemocyte populations for larvae infected with M. furfur and M. pachydermatis at any of the two temperatures (p < 0.05, 2-way ANOVA test). The pattern observed indicates that the percentage of plasmocytes tends to increase, and the percentages of granulocytes, spherulocytes, oenocytoids, and prohemocytes decrease as the inoculum concentration increases for both larvae infected with M. furfur or M. pachydermatis (Figure 5).
Figure 4. Hemocytes of G. mellonella under the light microscope. (A) Plasmatocytes (Pl) with M. furfur yeast; (B) oenocyte (Oe) and plasmatocyte (PI); (C) granulocyte (Gr); (D) spherulocyte (Sp); (E) granulocyte and prohemocyte (Pr); and (F) plasmatocyte and granulocyte.
Figure 5. Percentage of hemocyte population. A significant difference was found in hemocyte populations when the interaction between hemocyte class and inoculum concentrations was analyzed (p < 0.05, 2-way ANOVA test). As can be noted, plasmatocytes tend to increase as the inoculum concentracion increases; on the other hand, Granulocytes, spherulocytes, oenocytes, and prohemocytes tend to decrease as the inoculum concentracion increases. (A) Percentage of hemocytes population of G. mellonella larvae infected with M. furfur at 33°C; (B) percentage of hemocytes population of G. mellonella larvae infected with M. pachydermatis at 33°C; (C) percentage of hemocytes population of G. mellonella larvae infected with M. furfur at 37°C; and (D) percentage of hemocyte population of G. mellonella larvae infected with M. pachydermatis at 37°C. Pl, plasmatocytes; Gr, granulocyte; Sp, spherulocyte; Oe, oenocyte; and Pr, prohemocyte.
No significant difference in yeast isolation was found between any treatment (larvae infected with M. furfur or M. pachydermatis and incubated at 33 and 37°C) (p < 0.4068, 2-way ANOVA test). However, as can be seen in Figure 6, M. furfur isolation from larvae incubated at 37°C was lower than from those incubated at 33°C. The yeast isolation from larvae inoculated with 1.5 × 109 CFU/mL and incubated at 37°C was 10-folds lower than from those incubated at 33°C; as matter of fact, there was no isolation of yeasts from larvae inoculated with the lowest inoculum concentration and incubated at 37°C.
Figure 6. M. furfur and M. pachydermatis isolated from challenged G. mellonella larvae. No significant difference was found in yeast isolation of the two Malassezia species (p < 0.4068, 2-way ANOVA test). However, the recovery of yeasts from larvae challenged with M. furfur differed depending on temperature. At 33°C the fungal burden decreased but, in less proportion, than at 37°C. On the contrary, M. pachydermatis isolated from G. mellonella showed a better recovery at 33°C, similar yeast recovery was obtained for the highest inoculum concentration used. In all cases, the fungal burden demonstrated a decrease from the original inoculum concentration. Mf, M. furfur; Mp, M. pachydermatis.
On the contrary, M. pachydermatis was isolated in higher proportion from larvae incubated at 37°C than from larvae incubated at 33°C, with the isolation of yeast from larvae inoculated with 1.5 × 109 CFU/mL and incubated at 37°C, 2-folds higher than from larvae incubated at 33°C. At both temperatures, the M. pachydermatis burden was higher than the M. furfur burden in G. mellonella larvae, with the exception of larvae inoculated with the highest concentration and incubated at 33°C, in which the yeast isolation was 1.5-folds higher from larvae inoculated with M. furfur than from larvae inoculated with M. pachydermatis. At 37°C and using the highest inoculum concentration, the yeast recovery was 12-folds higher from larvae inoculated with M. pachydermatis than from larvae inoculated with M. furfur. In all cases, the fungal burden decreased compared to the original inoculum concentrations and it depended on its original inoculum concentration.
In order to evaluate the dissemination of M. furfur and M. pachydermatis at 37°C, larvae were sectioned into two halves and observed. As can be seen in Figure 7, larvae infected with either of the two species of Malassezia showed an increase in nodule occurrence as the inoculum concentration increased. In the case of larvae infected with M. furfur, it was perceived that the occurrence of nodules was concentrated to the periphery near the site of microinjection; in fact, this was easier to see in larvae infected with the highest concentration (Figures 7A–E). On the other hand, larvae infected with M. pachydermatis exhibited higher dissemination and occurrence of small nodules through the hemocoel (Figures 7F–I).
Figure 7. G. mellonella larvae half sectioned under the stereoscope. (A) Control larva showing no presence of nodules, in contrast (B–E) larvae infected with M. furfur displaying nodule formation close to the cuticle and lesser dissemination than (F–I) larvae infected with M. pachydermatis. In both cases, larvae present different quantity of nodules depending on the inoculum concentration, as the concentration increases, the number of nodules arise. Larvae infected with M. furfur (B) 1.5 × 106 CFU/mL inoculum concentration, (C) 1.5 × 107 CFU/mL inoculum concentration, (D) 1.5 × 108 CFU/mL inoculum concentration, and (E) 1.5 × 109 CFU/mL inoculum concentration. Larvae infected with M. pachydermatis (F) 1.5 × 106 CFU/mL inoculum concentration, (G) 1.5 × 107 CFU/mL inoculum concentration, (H) 1.5 × 108 CFU/mL inoculum concentration, and (I) 1.5 × 109 CFU/mL inoculum concentration. Red arrows show the position of nodules.
Once the presence of the nodules and the pattern of dissemination was observed, larvae were embedded in paraffin and sectioned to identify changes in larval tissue and the immune response, as well as to confirm the establishment of the infection. First, comparing infected larvae with control larvae, the loss of tissue integrity is evident (Figure 8). Besides, larvae infected with M. furfur inoculum concentration of 1.5 × 109 CFU/mL showed the presence of several nodules with melanin deposition of different sizes (Figure 8B). An enlarged image of one of the nodules (100X) shows the presence of newly recruited hemocyte around it (Figure 8C). Finally, yeasts of M. furfur are shown in the magnified nodule (1000X) (Figure 8D).
Figure 8. Histology of G. mellonella infected with M. furfur and M. pachydermatis. (A) Control larvae display high tissue integrity and no sign of nodule formation, different from this (B–D) larvae infected with M. furfur presented different size nodules with melanin deposition and recruitment of new hemocytes; this is similar to what happens to (E–G) larvae infected with M. pachydermatis, which present different nodule formation with hemocytes recruited around it and proximity to fat bodies. Red arrows point to the presence of M. furfur and M pachydermatis yeasts; blue arrows indicate the presence of nodules. Dissected nodules stained with Calcofluor white confirm infection by (H) M. furfur and (I) M. pachydermatis. Fb, fat bodies; He, hemocytes; Me, Melanin deposition.
Compared to M. furfur, larvae infected with M. pachydermatis also exhibited nodule formations and higher hemocyte recruitment (Figures 8E,F). Inside the nodule, it is possible to observe yeasts of M. pachydermatis (Figure 8G); in both cases, the structures were found close to the fat bodies. To confirm the infection by either of the species of Malassezia, yeasts were stained with calcofluor white and observed under the confocal microscope. In contrast to M. furfur, a higher number of M. pachydermatis was found inside the nodules (Figures 8H,I).
G. mellonella has been successfully implemented as an infection model of fungal pathogens, like Madurella mycetomatis (Kloezen et al., 2015), Cryptococcus neoformans (Eisenman et al., 2014), Aspergillus flavus (Scully and Bidochka, 2005), Candida albicans (Amorim-Vaz et al., 2015), Candida tropicalis (Mesa-Arango et al., 2013), and dermatophytes (Achterman et al., 2011; Ishii et al., 2017). This model allows the evaluation of virulence factors, anti-fungal agents and immune response to a pathogen. Compared to other animal models, G. mellonella larvae are easy to handle, cheap, have no ethical limitations, and their innate immune system is similar to that of humans (Fuchs et al., 2010; Pereira et al., 2018; Singkum et al., 2019; Trevijano-Contador and Zaragoza, 2019). Besides, as shown in this study, larvae can be easily roomed at the desired temperature in an incubator, allowing the researchers to have better control of the experimental condition and to get reliable and reproducible data.
In this study, the establishment of the systemic infection of M. furfur and M. pachydermatis in G. mellonella was tested, two species belonging to the Genus Malassezia that have been related to opportunistic infection in the bloodstream in newborns and immunocompromised patients (Dokos et al., 2011; Gaitanis et al., 2012; Al-Sweih et al., 2014; Iatta et al., 2014; Chen et al., 2020). For the first time, it has been demonstrated that M. furfur and M. pachydermatis can establish a systemic infection in G. mellonella larvae. Also, it was shown that the larval survival depends on inoculum concentration; at the highest inoculum concentration tested (1.5 × 109 CFU/mL), 50% of mortality was reached between day 12 and 13 for both species.
Furthermore, it was found that M. furfur at low concentrations can affect larval survival when they are incubated at 33°C, contrary to larvae incubated at 37°C in which larval survival is only affected at the highest inoculum concentration (1.5 × 109 CFU/mL). In contrast, larvae inoculated with M. pachydermatis showed a decrease in larval survival at any temperature, even in low concentrations. Larval survival variations at different temperatures for M. furfur and M. pachydermatis infection could be related to their ecology, since M. pachydermatis is mainly found in domestic animals, like dogs and cats, which mean temperatures are 38.2 and 38.1°C, respectively (Kabatchnick et al., 2016). On the other hand, M. furfur is primarily found in human beings with a lower corporal temperature than the mentioned animals, leading to the belief that M. pachydermatis is better adapted to a higher temperature than M. furfur. Indeed, it has been reported in previous studies that M. pachydermatis has its optimal growing temperature between 32 and 37°C (Bond and Lloyd, 1996); on the other hand, the optimal growing temperature of M. furfur is around 34°C (Leeming and Notman, 1987).
Phospholipase activity is an important virulence factor in tissue invasion in chronic otitis in animals; in fact, strains of M. pachydermatis isolated from dogs with otitis showed higher expression and activity of phospholipases than strains isolated from healthy animals (Cafarchia and Otranto, 2004; Ortiz et al., 2013). Also, it was shown that M. pachydermatis has a high lipase and phospholipase activity in comparison to M. furfur (Juntachai et al., 2009). Herein, M. pachydermatis showed to decrease larval survival at any concentration and at both temperatures. In contrast, M. furfur showed a decrease in the negative effect on larval survival at 37°C and the negative effect on larval survival at 33°C was lower than the negative effect of M. pachydermatis. This may be a consequence of the phospholipase activity of M. pachydermatis that allows invasion causing damage to the host, making it more pathogenic than M. furfur.
Larval mortality paused from day 6 to 10 for larvae infected with either M. furfur or M. pachydermatis and incubated at 37°C. This may be due to the fact that hemocytes in G. mellonella larvae tend to decrease at the end of the last larval instar until pupation. Also, it has been reported that fungal infection can lead to a decrease in the number of hemocytes (Boguś et al., 2018), which can suggest that cellular innate immune response is responsible for controlling Malassezia spp. Owing to the fact that once the percentage of hemocytes decreases as part of the physiology of the insect or as part of a virulence factor of the two species of Malassezia, the progression of infection retakes, agreeing with previous findings in which M. pachydermatis infection could only be established in Toll-deficient Drosophila melanogaster flies and immunosuppressed Swiss mice (Merkel et al., 2018; Schlemmer et al., 2018).
In contrast to other studies related to fungal infection assays in G. mellonella (Achterman et al., 2011; Mesa-Arango et al., 2013; Eisenman et al., 2014; Merkel et al., 2018; Sheehan and Kavanagh, 2019), the highest inoculum concentration (1.5 × 109 CFU/mL) was needed to successfully establish the M. furfur and M. pachydermatis systemic infection. This agrees with a previous report where nematodes challenged with M. pachydermatis showed higher survival than those infected with C. albicans (Brilhante et al., 2018). The aforementioned results may be due to the immune response and the low virulence of these yeasts that usually are found in skin mycobiota as commensal microorganisms (Yamasaki et al., 2009; Grice and Dawson, 2017; Schlemmer et al., 2018). The high load inoculum needed and the fact that the infection progress is improved as the number of hemocytes decreases can be an approach to better understand the reason why these two species of Malassezia can cause systemic infections in low weight newborns and immunosuppressed patients with a way of entry (intravascular catheter) and nutritional supply (parenteral nutrition with lipid infusion) for Malassezia.
The progress of the infection was evaluated through the melanization score, which depended on time and inoculum concentration; moreover, dissemination of the infection was evidenced by the observation of half sectioned larvae under a stereoscope, where a higher presence of nodules close to the microinjection site was found. Also, the number of nodules appeared to increase as the concentration of the inoculum was augmented, agreeing with the previous report on fungal pathogens (Perdoni et al., 2014; Sheehan and Kavanagh, 2019). Furthermore, as previously demonstrated, fungal burden decreased over time, which may be related to the immune response (Merkel et al., 2018; Schlemmer et al., 2018), but it did not show a significant difference between the two species at 33 and 37°C.
A previous study showed that the hydrophobicity of the cell wall of M. pachydermatis was higher than the cell wall of M. furfur, and also the hydrophobicity of M. pachydermatis was the same at 25 and 37°C, while the hydrophobicity of M. furfur was stronger at 25°C than at 37°C (Shibata et al., 2009). Hydrophobicity has been related to adherence and biofilm formation, the latter may provide protection from the host immune system (Cannizzo et al., 2007; Buommino et al., 2016; Angiolella et al., 2018), as has been shown before in C. albicans (Katragkou et al., 2010; Xie et al., 2012). This may explain why M. pachydermatis isolation was higher than M. furfur isolation; as matter of fact, the Malassezia pachydermatis isolation was 12-folds higher at 37°C than the M. furfur isolation, leading to the belief that M. pachydermatis can better avoid the immune system than M. furfur, and this is more evident at 37°C.
With respect to the percentage of hemocyte populations, the analysis of the interaction between the hemocyte class and the inoculum concentration showed an effect on the percentage of hemocyte populations. It was observed that the percentage of plasmocytes tends to increase with respect to the other four hemocyte classes. This may be due to the fact that these cells are responsible for adherence and phagocytosis, which is the main antifungal immune response, and also that these cells participated in nodule formation (Arteaga Blanco et al., 2017; Boguś et al., 2018; Pereira et al., 2018).
The histological findings revealed the presence of nodules surrounded by hemocytes with melanin deposition as part of the immune response of G. mellonella. The location of these nodules was mainly close to the cuticle in association with fat bodies; besides, tissue integrity was disrupted in larvae infected with the highest concentrations, similar to previous findings in other fungal infection assays (Eisenman et al., 2014; Perdoni et al., 2014; Wuensch et al., 2018; Sheehan and Kavanagh, 2019). The presence of yeast of M. furfur and M. pachydermatis inside the nodules was observed using calcofluor white, confirming infection by these two agents.
In conclusion, it has been demonstrated in the present study that G. mellonella is a suitable infection model for M. furfur and M. pachydermatis. The larvae have shown to successfully respond and control Malassezia infection through the innate immune response. However, in this study, the reference strains used were originally isolated from skin lesions (Guého-Kellermann et al., 2010), which may be a bias. Further studies must be done to evaluate different strains from symptomatic and asymptomatic hosts, the systemic infection in immunosuppressed larvae, virulence factor expression and antifungal resistance, allowing mycologists to unravel the host-pathogen interaction that leads Malassezia spp. to stay as a commensal microorganism in the skin of healthy patients or to become an opportunistic pathogen in immunosuppressed ones.
The datasets generated for this study are available on request to the corresponding author.
AC, MT, CP, and EP contributed to the design of the work. AC, MT, EP, FR, and HM performed the experiments. AC, MT, and FR were involved in the analysis and interpretation of data. MT wrote the manuscript. AC and CP made revisions. All authors approved the version to be published and agreed to be accountable for all aspects of the work.
This work was supported by the Faculty of Sciences at the Universidad de los Andes through grant No. INV-2019-62-1658.
The authors declare that the research was conducted in the absence of any commercial or financial relationships that could be construed as a potential conflict of interest.
We thank the microscopy core, μ-core, of the Vice-presidency of research at the Universidad de los Andes, Colombia.
Achterman, R. R., Smith, A. R., Oliver, B. G., and White, T. C. (2011). Sequenced dermatophyte strains: growth rate, conidiation, drug susceptibilities, and virulence in an invertebrate model. Fungal Genet. Biol. 48, 335–341. doi: 10.1016/j.fgb.2010.11.010
Al-Sweih, N., Ahmad, S., Joseph, L., Khan, S., and Khan, Z. (2014). Malassezia pachydermatis fungemia in a preterm neonate resistant to fluconazole and flucytosine. Med. Mycol. Case Rep. 5, 9–11. doi: 10.1016/j.mmcr.2014.04.004
Amorim-Vaz, S., Delarze, E., Ischer, F., Sanglard, D., and Coste, A. T. (2015). Examining the virulence of Candida albicans transcription factor mutants using Galleria mellonella and mouse infection models. Front. Microbiol. 6:367. doi: 10.3389/fmicb.2015.00367
Angiolella, L., Leone, C., Rojas, F., Mussin, J., Angeles de los Sosa, M., and Giusiano, G. (2018). Biofilm, adherence, and hydrophobicity as virulence factors in Malassezia furfur. Med. Mycol. 56, 110–116. doi: 10.1093/mmy/myx014
Arteaga Blanco, L. A., Crispim, J. S., Fernandes, K. M., de Oliveira, L. L., Pereira, M. F., Bazzolli, D. M. S., et al. (2017). Differential cellular immune response of Galleria mellonella to Actinobacillus pleuropneumoniae. Cell Tissue Res. 370, 153–168. doi: 10.1007/s00441-017-2653-5
Bell, L. M., Alpert, G., Slight, P. H., and Campos, J. M. (1988). Malassezia furfur skin colonization in infancy. Infect. Control Hosp. Epidemiol. 9, 151–153. doi: 10.2307/30145422
Boguś, M. I., Ligeza-Zuber, M., Polanska, M. A., Mosiewicz, M., Włóka, E., and Sobocinska, M. (2018). Fungal infection causes changes in the number, morphology and spreading ability of Galleria mellonella haemocytes. Physiol. Entomol. 43, 214–226. doi: 10.1111/phen.12246
Bond, R., and Lloyd, D. H. (1996). Comparison of media and conditions of incubation for the quantitative culture of Malassezia pachydermatis from canine skin. Res. Vet. Sci. 61, 273–274. doi: 10.1016/S0034-5288(96)90078-8
Brilhante, R. S. N., da Rocha, M. G., de Melo Guedes, G. M., de Oliveira, J. S., dos Santos Araújo, G., España, J. D. A., et al. (2018). Malassezia pachydermatis from animals: planktonic and biofilm antifungal susceptibility and its virulence arsenal. Vet. Microbiol. 220, 47–52. doi: 10.1016/j.vetmic.2018.05.003
Buommino, E., Nocera, F. P., Parisi, A., Rizzo, A., Donnarumma, G., Mallardo, K., et al. (2016). Correlation between genetic variability and virulence factors in clinical strains of Malassezia pachydermatis of animal origin. New Microbiol. 39, 216–223.
Cabañes, F. J. (2014). Malassezia yeasts: how many species infect humans and animals? PLoS Pathog. 10:e1003892. doi: 10.1371/journal.ppat.1003892
Cabañes, F. J., Coutinho, S. D. A., Puig, L., Bragulat, M. R., and Castellá, G. (2016). New lipid-dependent Malassezia species from parrots. Rev. Iberoam. Micol. 33, 92–99. doi: 10.1016/j.riam.2016.03.003
Cafarchia, C., and Otranto, D. (2004). Association between phospholipase production by Malassezia pachydermatis and skin lesions. J. Clin. Microbiol. 42, 4868–4869. doi: 10.1128/JCM.42.10.4868-4869.2004
Cannizzo, F. T., Eraso, E., Ezkurra, P. A., Villar-Vidal, M., Bollo, E., Castellá, G., et al. (2007). Biofilm development by clinical isolates of Malassezia pachydermatis. Med. Mycol. 45, 357–361. doi: 10.1080/13693780701225767
Capilla, J., Clemons, K. V., and Stevens, D. A. (2007). Animal models: an important tool in mycology. Med. Mycol. 45, 657–684. doi: 10.1080/13693780701644140
Champion, O. L., Titball, R. W., and Bates, S. (2018). Standardization of G. Mellonella larvae to provide reliable and reproducible results in the study of fungal pathogens. J. Fungi 4:108. doi: 10.3390/jof4030108
Chang, H. J., Miller, H. L., Watkins, N., Arduino, M. J., Ashford, D. A., Midgley, G., et al. (1998). An epidemic of Malassezia pachydermatis in an intensive care nursery associated with colonization of health care workers' pet dogs. N. Engl. J. Med. 338, 706–711. doi: 10.1056/NEJM199803123381102
Chen, I. T., Chen, C. C., Huang, H. C., and Kuo, K. C. (2020). Malassezia furfur emergence and candidemia trends in a neonatal intensive care unit during 10 years: the experience of fluconazole prophylaxis in a single hospital. Adv. Neonatal Care 20, E3–E8. doi: 10.1097/ANC.0000000000000640
Cools, F., Torfs, E., Aizawa, J., Vanhoutte, B., Maes, L., Caljon, G., et al. (2019). Optimization and characterization of a Galleria mellonella larval infection model for virulence studies and the evaluation of therapeutics against Streptococcus pneumoniae. Front. Microbiol. 10:311. doi: 10.3389/fmicb.2019.00311
DeAngelis, Y. M., Gemmer, C. M., Kaczvinsky, J. R., Kenneally, D. C., Schwartz, J. R., and Dawson, T. L. (2005). Three etiologic facets of dandruff and seborrheic dermatitis: Malassezia fungi, sebaceous lipids, and individual sensitivity. J. Investig. Dermatol. Symp. Proc. 10, 295–297. doi: 10.1111/j.1087-0024.2005.10119.x
Dokos, C., Pana, Z. D., and Tragiannidis, A. (2011). Malassezia species: a rare cause of invasive fungal infections in immunocompromised patients. Curr. Fungal Infect. Rep. 5, 18–22. doi: 10.1007/s12281-010-0037-3
Eisenman, H. C., Duong, R., Chan, H., Tsue, R., and McClelland, E. E. (2014). Reduced virulence of melanized Cryptococcus neoformans in Galleria mellonella. Virulence 5, 611–618. doi: 10.4161/viru.29234
Fallon, J., Kelly, J., and Kavanagh, K. (2012). “Galleria mellonella as a model for fungal pathogenicity testing,” in Host-Fungus Interactions: Methods and Protocols, eds A. Brand and D. MacCallum (Totowa, NJ: Humana Press), 469–485. doi: 10.1007/978-1-61779-539-8_33
Freitak, D., Schmidtberg, H., Dickel, F., Lochnit, G., Vogel, H., and Vilcinskas, A. (2014). The maternal transfer of bacteria can mediate trans-generational immune priming in insects. Virulence 5, 547–554. doi: 10.4161/viru.28367
Fuchs, B. B., O'Brien, E., El Khoury, J. B., and Mylonakis, E. (2010). Methods for using Galleria mellonella as a model host to study fungal pathogenesis. Virulence 1, 475–482. doi: 10.4161/viru.1.6.12985
Gaitanis, G., Magiatis, P., Hantschke, M., Bassukas, I. D., and Velegraki, A. (2012). The Malassezia genus in skin and systemic diseases. Clin. Microbiol. Rev. 25, 106–141. doi: 10.1128/CMR.00021-11
Grice, E. A., and Dawson, T. L. (2017). Host–microbe interactions: Malassezia and human skin. Curr. Opin. Microbiol. 40, 81–87. doi: 10.1016/j.mib.2017.10.024
Guého-Kellermann, E., Boekhout, T., and Begerow, D. (2010). “Biodiversity, phylogeny and ultrastructure,” in Malassezia and the Skin: Science and Clinical Practice eds T. Boekhout, P. Mayser, E. Guého-Kellermann, A. Velegraki (Berlin Heidelberg: Springer), 17–63. doi: 10.1007/978-3-642-03616-3_2
Gupta, A. K., Batra, R., Bluhm, R., Boekhout, T., and Dawson, T. L. (2004). Skin diseases associated with Malassezia species. J. Am. Acad. Dermatol. 51, 785–798. doi: 10.1016/j.jaad.2003.12.034
Gupta, P., Chakrabarti, A., Singhi, S., Kumar, P., Honnavar, P., and Rudramurthy, S. M. (2014). Skin colonization by Malassezia spp. in hospitalized neonates and infants in a tertiary care centre in North India. Mycopathologia 178, 267–272. doi: 10.1007/s11046-014-9788-7
Honnavar, P., Prasad, G. S., Ghosh, A., Dogra, S., Handa, S., and Rudramurthy, S. M. (2016). Malassezia arunalokei sp. nov., a novel yeast species isolated from seborrheic dermatitis patients and healthy individuals from India. J. Clin. Microbiol. 54, 1826–1834. doi: 10.1128/JCM.00683-16
Iatta, R., Battista, M., Miragliotta, G., Boekhout, T., Otranto, D., and Cafarchia, C. (2018). Blood culture procedures and diagnosis of Malassezia furfur bloodstream infections: strength and weakness. Med. Mycol. 56, 828–833. doi: 10.1093/mmy/myx122
Iatta, R., Cafarchia, C., Cuna, T., Montagna, O., Laforgia, N., Gentile, O., et al. (2014). Bloodstream infections by Malassezia and Candida species in critical care patients. Med. Mycol. 52, 264–269. doi: 10.1093/mmy/myt004
Ishii, M., Matsumoto, Y., Yamada, T., Abe, S., and Sekimizu, K. (2017). An invertebrate infection model for evaluating anti-fungal agents against dermatophytosis. Sci. Rep. 7:12289. doi: 10.1038/s41598-017-12523-z
Juntachai, W., Oura, T., Murayama, S. Y., and Kajiwara, S. (2009). The lipolytic enzymes activities of Malassezia species. Med. Mycol. 47, 477–484. doi: 10.1080/13693780802314825
Kabatchnick, E., Langston, C., Olson, B., and Lamb, K. E. (2016). Hypothermia in uremic dogs and cats. J. Vet. Intern. Med. 30, 1648–1654. doi: 10.1111/jvim.14525
Kaneko, T., Murotani, M., Ohkusu, K., Sugita, T., and Makimura, K. (2012). Genetic and biological features of catheter-associated Malassezia furfur from hospitalized adults. Med. Mycol. 50, 74–80. doi: 10.3109/13693786.2011.584913
Katragkou, A., Kruhlak, M. J., Simitsopoulou, M., Chatzimoschou, A., Taparkou, A., Cotten, C. J., et al. (2010). Interactions between human phagocytes and Candida albicans biofilms alone and in combination with antifungal agents. J. Infect. Dis. 201, 1941–1949. doi: 10.1086/652783
Kay, S., Edwards, J., Brown, J., and Dixon, R. (2019). Galleria mellonella infection model identifies both high and low lethality of Clostridium perfringens toxigenic strains and their response to antimicrobials. Front. Microbiol. 10:1281. doi: 10.3389/fmicb.2019.01281
Kloezen, W., van Helvert-van Poppel, M., Fahal, A. H., and van de Sande, W. W. J. (2015). A Madurella mycetomatis grain model in Galleria mellonella larvae. PLoS Negl. Trop. Dis. 9, 1–14. doi: 10.1371/journal.pntd.0003926
Lacharme-Lora, L., Owen, S. V., Blundell, R., Canals, R., Wenner, N., Perez-Sepulveda, B., et al. (2019). The use of chicken and insect infection models to assess the virulence of African Salmonella typhimurium ST313. PLoS Negl. Trop. Dis. 13:e0007540. doi: 10.1371/journal.pntd.0007540
Lee, J., Cho, Y. G., Kim, D. S., Choi, S. I., and Lee, H. S. (2018). First case of catheter-related Malassezia pachydermatis fungemia in an adult. Ann. Lab. Med. 39, 99–101. doi: 10.3343/alm.2019.39.1.99
Leeming, J. P., and Notman, F. H. (1987). Improved methods for isolation and enumeration of Malassezia furfur from human skin. J. Clin. Microbiol. 25, 2017–2019. doi: 10.1128/JCM.25.10.2017-2019.1987
Loh, J. M. S., Adenwalla, N., Wiles, S., and Proft, T. (2013). Galleria mellonella larvae as an infection model for group A streptococcus. Virulence 4, 419–428. doi: 10.4161/viru.24930
Maurer, E., Hörtnagl, C., Lackner, M., Grässle, D., Naschberger, V., Moser, P., et al. (2019). Galleria mellonella as a model system to study virulence potential of mucormycetes and evaluation of antifungal treatment. Med. Mycol. 57, 351–362. doi: 10.1093/mmy/myy042
McGinnis, M. R., and Borgers, M. (1989). Current Topics in Medical Mycology. New York, NY: Springer-Verlag, 1–35. doi: 10.1007/978-1-4612-3624-5
Merkel, S., Heidrich, D., Danilevicz, C. K., Scroferneker, M. L., and Zanette, R. A. (2018). Drosophila melanogaster as a model for the study of Malassezia pachydermatis infections. Vet. Microbiol. 224, 31–33. doi: 10.1016/j.vetmic.2018.08.021
Mesa-Arango, A. C., Forastiero, A., Bernal-Martínez, L., Cuenca-Estrella, M., Mellado, E., and Zaragoza, O. (2013). The non-mammalian host Galleria mellonella can be used to study the virulence of the fungal pathogen Candida tropicalis and the efficacy of antifungal drugs during infection by this pathogenic yeast. Med. Mycol. 51, 461–472. doi: 10.3109/13693786.2012.737031
Mylonakis, E., Moreno, R., El Khoury, J. B., Idnurm, A., Heitman, J., Calderwood, S. B., et al. (2005). Galleria mellonella as a model system to study Cryptococcus neoformans pathogenesis. Infect. Immun. 73, 3842–3850. doi: 10.1128/IAI.73.7.3842-3850.2005
Nagata, R., Nagano, H., Ogishima, D., Nakamura, Y., Hiruma, M., and Sugita, T. (2012). Transmission of the major skin microbiota, Malassezia, from mother to neonate. Pediatr. Int. 54, 350–355. doi: 10.1111/j.1442-200X.2012.03563.x
Ortiz, G., Martín, M. C., Carrillo-Muñoz, A. J., and Payá, M. J. (2013). Phospholipase and proteinase production by Malassezia pachydermatis isolated in dogs with and without otitis. Rev. Iberoam. Micol. 30, 235–238. doi: 10.1016/j.riam.2013.01.006
Paul, A. A., Hoffman, K. L., Hagan, J. L., Sampath, V., Petrosino, J. F., and Pammi, M. (2019). Fungal cutaneous microbiome and host determinants in preterm and term neonates. Pediatr. Res. doi: 10.1038/s41390-019-0719-7
Perdoni, F., Falleni, M., Tosi, D., Cirasola, D., Romagnoli, S., Braidotti, P., et al. (2014). A histological procedure to study fungal infection in the wax moth Galleria mellonella. Eur. J. Histochem. 58, 258–262. doi: 10.4081/ejh.2014.2428
Pereira, T., de Barros, P., Fugisaki, L., Rossoni, R., Ribeiro, F., de Menezes, R., et al. (2018). Recent advances in the use of Galleria mellonella model to study immune responses against human pathogens. J. Fungi 4:128. doi: 10.3390/jof4040128
Prohic, A., Jovovic Sadikovic, T., Krupalija-Fazlic, M., and Kuskunovic-Vlahovljak, S. (2016). Malassezia species in healthy skin and in dermatological conditions. Int. J. Dermatol. 55, 494–504. doi: 10.1111/ijd.13116
Roman, J., Bagla, P., Ren, P., Blanton, L. S., and Berman, M. A. (2016). Malassezia pachydermatis fungemia in an adult with multibacillary leprosy. Med. Mycol. Case Rep. 12, 1–3. doi: 10.1016/j.mmcr.2016.05.002
Rosowski, E. E., Knox, B. P., Archambault, L. S., Huttenlocher, A., Keller, N. P., Wheeler, R. T., et al. (2018). The zebrafish as a model host for invasive fungal infections. J. Fungi 4:136. doi: 10.3390/jof4040136
Schlemmer, K. B., Jesus, F. P. K., Loreto, É. S., Tondolo, J. S. M., Ledur, P. C., Dallabrida, A., et al. (2018). An experimental murine model of otitis and dermatitis caused by Malassezia pachydermatis. Mycoses 61, 954–958. doi: 10.1111/myc.12839
Scully, L. R., and Bidochka, M. J. (2005). Serial passage of the opportunistic pathogen Aspergillus flavus through an insect host yields decreased saprobic capacity. Can. J. Microbiol. 51, 185–189. doi: 10.1139/w04-124
Sheehan, G., and Kavanagh, K. (2019). Proteomic analysis of the responses of Candida albicans during infection of Galleria mellonella larvae. J. Fungi 5:7. doi: 10.3390/jof5010007
Shibata, N., Saitoh, T., Tadokoro, Y., and Okawa, Y. (2009). The cell wall galactomannan antigen from Malassezia furfur and Malassezia pachydermatis contains β-1,6-linked linear galactofuranosyl residues and its detection has diagnostic potential. Microbiology 155, 3420–3429. doi: 10.1099/mic.0.029967-0
Singkum, P., Suwanmanee, S., Pumeesat, P., and Luplertlop, N. (2019). A powerful in vivo alternative model in scientific research: Galleria mellonella. Acta Microbiol. Immunol. Hung. 66, 31–55. doi: 10.1556/030.66.2019.001
Sparber, F., De Gregorio, C., Steckholzer, S., Ferreira, F. M., Dolowschiak, T., Ruchti, F., et al. (2019). The skin commensal yeast Malassezia triggers a type 17 response that coordinates anti-fungal immunity and exacerbates skin inflammation. Cell Host Microbe 25, 389–403. doi: 10.1016/j.chom.2019.02.002
Stephens, J. M. (1962). Bactericidal activity of the blood of actively immunized wax moth larvae. Can. J. Microbiol. 8, 491–499. doi: 10.1139/m62-064
Theelen, B., Cafarchia, C., Gaitanis, G., Bassukas, I. D., Boekhout, T., and Dawson, T. L. (2018). Malassezia ecology, pathophysiology, and treatment. Med. Mycol. 56, S10–S25. doi: 10.1093/mmy/myx134
Tragiannidis, A., Bisping, G., Koehler, G., and Groll, A. H. (2010). Minireview: Malassezia infections in immunocompromised patients. Mycoses 53, 187–195. doi: 10.1111/j.1439-0507.2009.01814.x
Trevijano-Contador, N., and Zaragoza, O. (2019). Immune response of Galleria mellonella against human fungal pathogens. J. Fungi 5:3. doi: 10.3390/jof5010003
Tsai, C. J. Y., Loh, J. M. S., and Proft, T. (2016). Galleria mellonella infection models for the study of bacterial diseases and for antimicrobial drug testing. Virulence 7, 214–229. doi: 10.1080/21505594.2015.1135289
Wojda, I. (2017). Immunity of the greater wax moth Galleria mellonella. Insect Sci. 24, 342–357. doi: 10.1111/1744-7917.12325
Wuensch, A., Trusch, F., Iberahim, N. A., and van West, P. (2018). Galleria melonella as an experimental in vivo host model for the fish-pathogenic oomycete Saprolegnia parasitica. Fungal Biol. 122, 182–189. doi: 10.1016/j.funbio.2017.12.011
Xie, Z., Thompson, A., Sobue, T., Kashleva, H., Xu, H., Vasilakos, J., et al. (2012). Candida albicans biofilms do not trigger reactive oxygen species and evade neutrophil killing. J. Infect. Dis. 206, 1936–1945. doi: 10.1093/infdis/jis607
Yamasaki, S., Matsumoto, M., Takeuchi, O., Matsuzawa, T., Ishikawa, E., Sakuma, M., et al. (2009). C-type lectin mincle is an activating receptor for pathogenic fungus, Malassezia. Proc. Natl. Acad. Sci. U. S. A. 106, 1897–1902. doi: 10.1073/pnas.0805177106
Keywords: Galleria mellonella, Malassezia furfur, Malassezia pachydermatis, infection model, host-pathogen interaction
Citation: Torres M, Pinzón EN, Rey FM, Martinez H, Parra Giraldo CM and Celis Ramírez AM (2020) Galleria mellonella as a Novelty in vivo Model of Host-Pathogen Interaction for Malassezia furfur CBS 1878 and Malassezia pachydermatis CBS 1879. Front. Cell. Infect. Microbiol. 10:199. doi: 10.3389/fcimb.2020.00199
Received: 26 February 2020; Accepted: 15 April 2020;
Published: 05 May 2020.
Edited by:
Martin Hoenigl, Medical University of Graz, AustriaReviewed by:
Claudia Cafarchia, University of Bari Aldo Moro, ItalyCopyright © 2020 Torres, Pinzón, Rey, Martinez, Parra Giraldo and Celis Ramírez. This is an open-access article distributed under the terms of the Creative Commons Attribution License (CC BY). The use, distribution or reproduction in other forums is permitted, provided the original author(s) and the copyright owner(s) are credited and that the original publication in this journal is cited, in accordance with accepted academic practice. No use, distribution or reproduction is permitted which does not comply with these terms.
*Correspondence: Adriana Marcela Celis Ramírez, YWNlbGlzQHVuaWFuZGVzLmVkdS5jbw==
Disclaimer: All claims expressed in this article are solely those of the authors and do not necessarily represent those of their affiliated organizations, or those of the publisher, the editors and the reviewers. Any product that may be evaluated in this article or claim that may be made by its manufacturer is not guaranteed or endorsed by the publisher.
Research integrity at Frontiers
Learn more about the work of our research integrity team to safeguard the quality of each article we publish.