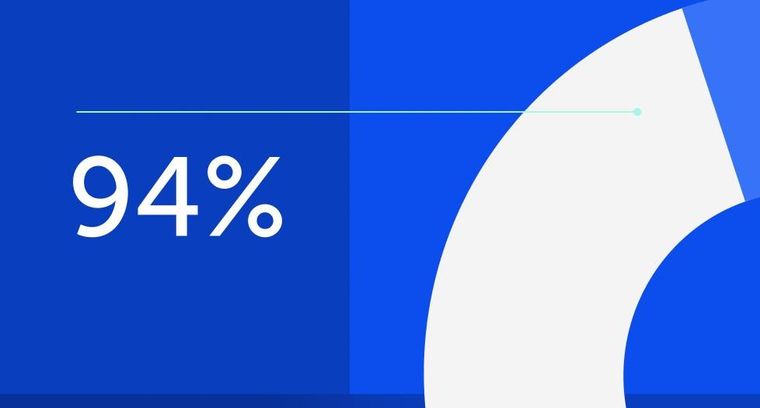
94% of researchers rate our articles as excellent or good
Learn more about the work of our research integrity team to safeguard the quality of each article we publish.
Find out more
REVIEW article
Front. Cell Dev. Biol., 27 March 2025
Sec. Cancer Cell Biology
Volume 13 - 2025 | https://doi.org/10.3389/fcell.2025.1558403
This article is part of the Research TopicInnovative Tumor Therapies: Targeting Angiogenesis and MetabolismView all 5 articles
Pediatric thoracic solid tumors encompass mediastinal tumors, chest wall tumors, and lung tumors. The pathogenesis is complex, and the clinical presentation is diverse, presenting numerous challenges in diagnosis and treatment, which severely threaten the life and health of the affected children. Angiogenesis provides nutritional and oxygen support for tumor growth and metastasis, while metabolic reprogramming meets the unique energy and material demands of tumor. Both processes play key roles in pediatric thoracic tumor development. Therefore, targeting tumor vasculature could be an important therapeutic strategy, and exploring the molecular mechanisms of metabolic reprogramming may provide a theoretical foundation for targeted treatment. This review summarizes relevant experimental research on angiogenesis and metabolic reprogramming in pediatric thoracic tumors, analyzes the limitations of current research, and proposes solutions and recommendations. Through this review, we aim to provide comprehensive information about pediatric thoracic solid tumors for clinicians and researchers, promoting personalized treatment, and ultimately improve survival rates and quality of life for affected children.
Pediatric thoracic tumors are a heterogeneous group of neoplasms, accounting for 15%–20% of all malignant tumors in childhood (Meazza and Gattuso, 2019). Pediatric thoracic tumor included tumors in areas such as the chest wall, lungs, airways, mediastinal organs, esophagus, and diaphragm (Zapala et al., 2017). They are primarily composed of abnormal tissue formed from residual embryonic tissue or metastatic tumors (Mullen et al., 2021). Clinically, neurogenic tumors and lymphomas are relatively common (Jaggers and Balsara, 2004). The insidious progression of many thoracic tumors frequently delays diagnosis, initial asymptomatic phases transition to clinically significant manifestations when mass effects compromise critical structures, such as airway compression precipitating stridor, mediastinal invasion causing superior vena cava syndrome, and diaphragmatic involvement leading to respiratory insufficiency (Guye et al., 2007). The incidence of malignant pediatric thoracic tumor has been an increasing trend in recent years (Bhakta et al., 2019). Pediatric thoracic tumors present a heterogeneous prognostic landscape (Figure 1). Many children with thoracic tumors have already progressed to the middle and late stages when they are diagnosed, which seriously affects the survival rate of the children, and has become one of the main causes of death in children (Lam et al., 2019).
Figure 1. The overview of pediatric thoracic tumors and its prognosis (Created with BioGDP.com (Jiang et al., 2025)).
Pediatric thoracic tumors are mainly divided into chest wall tumors, lung and airway tumors, and mediastinal tumors according to their origin. At present, the main treatment methods of pediatric thoracic tumors are still surgery and chemotherapy. We integrate the pathological features and clinical management of various pediatric thoracic tumors. Table 1 systematically summarizes the anatomic sites, origin cells, involved organs, recommended treatment options, and current treatment challenges of each pediatric thoracic tumor.
Annotation. HL: Hodgkin Lymphoma; NHL: Non-Hodgkin Lymphoma; GCT: Germ Cell Tumor; ALK: Anaplastic Lymphoma Kinase; EGFR: Epidermal Growth Factor Receptor; GD2: Disialoganglioside GD2; MEK: Mitogen-activated protein kinase; TKIs: Tyrosine Kinase Inhibitors; PAX3/7: Paired Box gene 3/7; FOXO1: Forkhead Box O1; CRTC1: CREB Regulated Transcription Coactivator 1; MAML2: Mastermind-Like Protein 2; DICER1: Ribonuclease III enzyme DICER1.
Pediatric thoracic tumors in children differ significantly from those in adults in terms of pathogenesis and genetic landscape (Chen X. et al., 2024). Firstly, the types of tumors are different. Pediatric thoracic tumors often arise from developmental tissues and are associated with genetic mutations present at birth or acquired early in life, whereas adult thoracic malignancies are primarily driven by lifestyle-induced somatic mutations (Kattner et al., 2019). Secondly, there are physiological differences between children and adults. For example, pediatric chest cavity is smaller, and compared to adults, thoracic tumors are more likely to compress the heart, airways, and esophagus, leading to respiratory and circulatory functional disorders (Pearson and Tan, 2015). Furthermore, pediatric organs are not fully mature, and pediatric medications carry higher risks, chemotherapy drugs at adult doses can be highly toxic to children (Weingart et al., 2018). Given the specific physiological, pathological, and pharmacokinetic differences in children, there is an urgent need to develop treatment specifically for pediatric thoracic tumors to improve therapeutic efficacy.
Pediatric thoracic tumors demonstrate aggressive growth patterns with frequent thoracic occupation, inducing severe compression or invasion of cardiopulmonary-vascular structures (Sandler and Hayes-Jordan, 2018). While surgery and chemotherapy remain cornerstone therapies, their efficacy diminishes significantly in recurrent or refractory cases (Burkhardt and Hermiston, 2019). Although targeted therapies against angiogenesis and tumor metabolism pathways hold transformative potential, clinical translation is hampered by insufficient pediatric-specific drug development and trial data (Dasgupta et al., 2017). This review will summarize the current research progress on targeting angiogenesis and tumor cell metabolism, and discuss their potential applications in the treatment of pediatric thoracic tumors, with the aim of providing new insights and directions for future treatment strategies.
Angiogenesis is a critical step in tumor growth and metastasis, serving to transport nutrients and remove metabolic waste products from tumor cells (Jiang et al., 2020). Tumors induce angiogenesis to promote metastasis and proliferation, inhibiting pro-angiogenic factors, such as vascular endothelial growth factor (VEGF), is a key anti-angiogenic therapy (Janes et al., 2024). Bevacizumab, a VEGF-specific antibody, was the first clinically approved anti-angiogenic drug (Garcia et al., 2020). Research data indicate that low doses of bevacizumab not only reduce the formation of pathological blood vessels but also repair existing vascular defects (Summers et al., 2010). This further demonstrates that appropriate doses of VEGF inhibitors can rebalance angiogenesis signaling within tumors, actively recruit pericytes, and enhance intercellular junctions (Jain et al., 2006). Researchers have found that bevacizumab treatment reduced microvessel density in alveolar RMS, increased tumor vessel maturity, enhanced the effectiveness of ionizing radiation for alveolar RMS, and improved patient prognosis (Myers et al., 2010). However, due to the relative specificity of these targets, their clinical application still faces some challenges. Research evidence suggests that anti-VEGF therapy may enhance the signaling of platelet-derived growth factor receptor, thereby promoting the recruitment of pericytes and reducing vascular permeability. The high coverage of pericytes supports the survival of tumor endothelial cells, and the hypoxic environment caused by the disruption of the tumor vasculature further stimulates tumor cells to develop stronger resistance and enhanced angiogenic capability (Greenberg et al., 2008). Therefore, new VEGF-targeted therapies are still under development. Sunitinib is a novel multi-targeted inhibitor, and multiple clinical trials have demonstrated its efficacy in patients with solid tumors. Sunitinib inhibits several tyrosine kinases, particularly the VEGFR, thereby blocking the angiogenesis process required for tumor growth, resulting in clinical benefits for patients with mediastinal germ cell tumors (Subbiah et al., 2014). Primary mediastinal germ cell tumors exhibit significant angiogenic characteristics, with the formation of abnormal tumor blood vessels in the incompletely matured stroma. These blood vessels may contribute to a certain degree of resistance to treatment (Levy et al., 2021). Researchers used CRISPR/Cas9 technology to knock out the REST gene in Ewing sarcoma cells. In vivo experiments revealed that REST knockout tumors showed a significant reduction in pericytes and blood vessel perfusion, with a marked decrease in the ratio of pericytes to endothelial cells, increased vascular leakage, and induced tumor hypoxic, leading to inhibition of tumor growth and metastasis (Zhou et al., 2020). These findings confirm the clinical potential of vascular target therapy in Ewing sarcoma.
Additional studies targeting anti-angiogenesis have focused on angiogenesis-related signal pathways. The FGF/FGFR signaling pathway also plays an important role in promoting tumor angiogenesis, and FGF is a pro-angiogenic factor that synergistically induces tumor angiogenesis with VEGF (Presta et al., 2017). Researchers found that the FGF pathway and neural cell adhesion molecule 1 (NCAM1) were activated in the gene expression profile of pleuropulmonary blastoma, and that intervening in the FGF signaling pathway by targeting NCAM1 could effectively inhibit tumor growth and progression, which provided a new idea for pleuropulmonary blastoma treatment (Shukrun et al., 2019). TGF-β has been shown to stimulate angiogenesis, and the abundant vasculature in carcinoid may be a result of the indirect effects of TGF-β within the tumor stroma. The role of TGF-β in pulmonary carcinoid depends on its microenvironment, and modulating TGF-β could become one of the strategies for targeted therapy in pulmonary carcinoid (Slodkowska et al., 2000). Activation of the EGFR signaling pathway induces upregulation of VEGF expression to drive tumor growth (Hung et al., 2016). The high-grade mucoepidermoid carcinomas exhibit stronger invasiveness. In high-grade MECs, the EGFR and ERK signaling pathways are often abnormally activated, targeting EGFR may provide additional therapeutic benefits, potentially improving patient survival prognosis and quality of life (Lujan et al., 2010).
Due to the excessive and sustained release of pro-angiogenic factors within the tumor microenvironment, the newly formed vascular network may fail to mature. The uneven caliber of blood vessels and the tortuous, disorganized structure of the vascular network subsequently contribute to increased localized hypoxia (Guelfi et al., 2024). Furthermore, the reduced density of pericytes and their decreased connection to endothelial cells lead to abnormal tumor vasculature. The characteristics of these abnormal blood vessels, including high permeability and low perfusion, impair drug delivery and thereby decrease the effectiveness of tumor treatment (Jiang Z. et al., 2023). In 2001, the concept of vascular normalization was formally introduced, which involves remodeling tumor blood vessels to restore their structure and function to improve vascular oxygenation and perfusion, rather than solely disrupting the growth of blood vessels (Jaszai and Schmidt, 2019). In the context of pediatric thoracic tumors, vascular normalization holds unique promise. Studies in pediatric thoracic tumor models, such as alveolar rhabdomyosarcoma, demonstrate that mTOR/VEGF inhibition (e.g., rapamycin) can normalize vasculature and enhance radiation efficacy (Myers et al., 2012). Studies in pediatric tumor neuroblastoma, demonstrate that anlotinib can normalize vasculature and induces tumor regression (Su et al., 2022). However, clinical translation remains limited, with few trials explicitly testing vascular normalization strategies in pediatric thoracic tumors. While anti-angiogenic drugs (e.g., VEGF inhibitors) reduce tumor vascularity, they may exacerbate hypoxia and promote treatment resistance in slow-growing pediatric tumors, which often rely on prolonged therapy (Lupo et al., 2016). Vascular normalization, by contrast, aims to stabilize the tumor microenvironment, potentially improving chemotherapy or radiation sensitivity and reducing metastatic shedding (Yu et al., 2023), a critical advantage for children with thoracic tumor. The scarcity of dedicated studies in pediatric thoracic tumors reflects challenges in modeling these rare cancers and concerns about long-term vascular toxicity in developing tissues. Nevertheless, emerging data from adult thoracic malignancies (e.g., lung cancer) and pediatric preclinical models suggest that normalization could mitigate hypoxia-driven aggression and therapy resistance in these tumors, justifying its inclusion in this review (Jiang S. et al., 2023).
Immune cells can coordinate the entire process of tumor angiogenesis. Innate immune cells, such as mature dendritic cells and M1 tumor-associated macrophages, produce cytokines that inhibit tumor angiogenesis. Adaptive immune cells, such as CD8+ T cells and T helper cells 1, secrete interferons, which are effective cytokines that suppress angiogenesis and induce vascular normalization (Delprat and Michiels, 2021). Immune cells play a complex role in tumor angiogenesis, and combining immune cell therapy and targeting tumor vasculature can help to personalize tumor therapy. Ewing sarcoma is a highly vascularized tumor with consistent expression of VEGFR2 on its blood vessels. Chimeric Antigen Receptor (CAR) T-cell engineering directly links T cells to specific tumor markers, enhancing their ability to recognize and kill tumor cells. Researchers used VEGFR2-specific CAR-T cells to selectively target and disrupt the Ewing sarcoma tumor-associated vasculature, inducing hypoxic tumor cell death and eradicating the tumor (Englisch et al., 2020). Neuroblastoma is characterized by a highly vascularized nature. Studies have shown that Anlotinib, by inhibiting pro-angiogenic factor receptors and reducing pro-angiogenic factors, reverses the early exhaustion of CD4+ T cells. This promotes tumor vascular normalization, leading to increased infiltration of immune effector cells and significantly inducing the regression of neuroblastoma (Su et al., 2022).
Tumor cells reprogram their metabolism to meet their energy demands, characterized by upregulation of aerobic glycolysis, increased fatty acid synthesis, and enhanced amino acid catabolism. As one of the important markers in the process of malignant tumor occurrence and development, metabolic reprogramming provides an important material and energy basis for tumor cell proliferation and metastasis, in which glucose metabolism, fatty acid metabolism and amino acid metabolism play important roles (Xiang et al., 2023). Metabolic reprogramming not only acts on tumor cells but also regulates tumor by influencing the tumor microenvironment (Zhang et al., 2024). Therefore, targeted therapies based on tumor metabolism have gained widespread attention. Targeted drugs developed for glucose metabolism, lipid metabolism, and amino acid metabolism have shown promising results in both basic research and clinical trials (Wang et al., 2024). Then we provide a systematic exposition of metabolic reprogramming in pediatric thoracic solid tumors, and this may help in recognizing tumor vulnerabilities and identifying new therapeutic targets.
Glycolysis and mitochondrial oxidative phosphorylation are two main pathways of cellular energy metabolism, and their roles in pediatric thoracic solid tumors are complex and diverse. Glycolysis is a process in the cytoplasm where glucose is broken down into pyruvate, and even under aerobic conditions, tumor cells tend to generate energy through glycolysis, a phenomenon known as the Warburg effect. The key enzymes in the glycolytic process, such as hexokinase, phosphofructokinase, and pyruvate kinase, have become research targets for cancer therapy (Tufail et al., 2024). Mitochondrial oxidative phosphorylation is a process that generates ATP through the electron transport chain and the tricarboxylic acid cycle. Mitochondrial oxidative phosphorylation not only provides energy to the cell, but also participates in cellular anabolism and signal transduction, which is essential for the proliferation and survival of tumor cells (Wu et al., 2022). Studying the role of glycolysis and mitochondrial oxidative phosphorylation not only provides clues for understanding metabolic reprogramming in pediatric thoracic tumors, but also offers potential targets for developing new therapeutic strategies. The metabolism of Ewing sarcoma cells relies on aerobic glycolysis. Melatonin reduces glycolytic metabolism by inhibiting the activity of HIF-1α in Ewing sarcoma cells, leading to decreased glucose uptake, lactate levels, and lactate dehydrogenase activity. This effectively suppresses the growth and survival of Ewing sarcoma cells, with potential therapeutic value (Sanchez-Sanchez et al., 2015). MYCN proto-oncogene (MYCN) acts as both an oncogenic driver and a metabolic reprogramming regulator, altering cellular energy metabolism to fuel neuroblastoma progression (Talapatra and Reddy, 2023). Research has shown that MYCN amplification can significantly enhance the uptake and consumption of glucose in neuroblastoma. Specifically, MYCN, as an oncogenic protein, directly regulates several key enzymes involved in glucose metabolism, including enzymes in the glycolytic pathway, thereby promoting the elevation of glycolysis (Oliynyk et al., 2019). Considering this metabolic characteristic, glycolysis inhibitors have been used to treat MYCN-amplified neuroblastoma. These glycolysis inhibitors effectively inhibit glycolytic activity in MYCN-amplified cells in neuroblastoma models, thereby reducing the energy supply to tumor cells and limiting their proliferative capacity, demonstrating potential antitumor effects (Levy et al., 2012; Hagenbuchner et al., 2016). MYCN also regulates mitochondrial respiration and oxidative phosphorylation by increasing the expression of various enzymes in neuroblastoma, thereby supporting the rapid proliferation of tumor cells. In this context, depletion of dihydrolipoamide succinyltransferase can significantly inhibit the production of reduced nicotinamide adenine dinucleotide, disrupt oxidative phosphorylation, and subsequently impair neuroblastoma formation and inhibit tumor invasion (Anderson et al., 2021). Moreover, the combination therapy of vorinostat and rapamycin significantly reduced levels of two critical glycolysis rate-limiting enzymes - Hexokinase 2 (HK2, catalyzing the first phosphorylation step) and Glucose-6-phosphate isomerase (GPI, mediating glucose-6-phosphate isomerization) - in neuroblastoma models. This suppression coincided with elevated reactive oxygen species (ROS) levels, effectively blocking tumor metabolic reprogramming and revealing therapeutic potential for advanced neuroblastoma (Bishayee et al., 2022).
Glutamine is a major amino acid in the body and a primary fuel source for tumor cells (Jain et al., 2012). The consumption of glutamine disrupts the redox equilibrium of tumor cells, thereby decreasing the proliferation capacity of neuroblastoma cells and enhancing the radiosensitivity of non-MYCN amplified tumor cell. Targeting glutamine metabolism might be a potential therapeutic approach for neuroblastoma (Le Grand et al., 2020). Research has found that rhabdomyosarcoma consumes more glucose and glutamine than healthy tissue. After receiving radiation therapy, the rhabdomyosarcoma undergoes metabolic reprogramming, characterized by a weakened tricarboxylic acid cycle involving glucose and a shift towards reactions dominated by glutamine metabolism. Inhibiting glutaminase in vivo can enhance the effectiveness of tumor radiation therapy, suggesting that drug targeting of glutamine metabolism could be a method to sensitize rhabdomyosarcoma patients to radiotherapy (Patel et al., 2024). One-carbon metabolism is a series of biochemical reactions that involve the transfer, oxidation, or reduction of single-carbon units. These units are crucial for the synthesis of essential biomolecules like nucleotides, certain amino acids, and other cellular components. The major pathways of one-carbon metabolism include serine metabolism, glycine metabolism, and folate metabolism (Yang et al., 2021). Researchers have discovered that the Serine-glycine-one-carbon (SGOC) biosynthetic pathway is significantly activated in MYCN amplified neuroblastoma cells. Tumor cells utilize the SGOC pathway to convert carbon sources from glucose into serine and glycine to meet their needs for rapid proliferation. Based on this finding, researchers have used small molecule inhibitors to effectively block the SGOC pathway, not only disrupting the amino acid synthesis but also enhancing metabolic stress, thereby promoting tumor cell death through autophagy (Xia et al., 2019). Methylenetetrahydrofolate dehydrogenase 1 (MTHFD1) is a key enzyme in the folate metabolic pathway, involved in one-carbon metabolism, and supports tumor cell growth by maintaining the redox homeostasis of NADPH. In neuroblastoma, by targeting and inhibiting the activity of MTHFD1, the folate metabolic pathway is further suppressed, increasing the level of ROS within tumor cells, enhancing their metabolic stress, and triggering tumor cell apoptosis, thereby exerting an anti-tumor effect (Guan et al., 2024). MYCN enhances the dependency of neuroblastoma cells on pyrimidine nucleotide synthesis by upregulating enzymes related to pyrimidine biosynthesis, such as dihydroorotate dehydrogenase (DHODH). Considering this metabolic characteristic, researchers have inhibited tumor progression by genetically editing or pharmacologically inhibiting DHODH. Additionally, using dipyridamole to inhibit nucleotide transport, in combination with DHODH inhibitors, has augmented the therapeutic efficacy against neuroblastoma (Yu et al., 2021). The EWS/FLI fusion gene (EF) drives metabolic reprogramming, diverting glycolytic intermediates towards the synthesis of serine and glycine to support the occurrence and development of Ewing sarcoma. Key regulatory enzymes in these metabolic reprogramming processes may become new therapeutic targets (Tanner et al., 2017).
Lipids are one of the three major nutrients essential for maintaining life functions, energy storage, and metabolic homeostasis (Snaebjornsson et al., 2020). Lipid metabolism reprogramming is a significant metabolic characteristic of tumor, referring to the process where tumor cells readjust their lipid metabolic pathways in response to various internal and external stimuli. This adaptation promotes tumor to cope with new physiological conditions and sustains its rapid growth (Broadfield et al., 2021). In normal cells, the majority of lipids required for metabolism are obtained through dietary intake or synthesized in the liver. However, in tumor cells, lipid metabolism reprogramming favors de novo lipid synthesis, with lipids being stored inside the cells in the form of lipid droplets, and enriched in hypoxic tissue (Cao, 2019; Shakya et al., 2021). Citrate located in the cytosol is transformed into acetyl-CoA through the catalysis of ATP-citrate lyase. Subsequently, under the influence of acetyl-CoA carboxylase (ACC) and fatty acid synthase (FASN), acetyl-CoA is utilized for the synthesis of palmitic acid. Furthermore, stearoyl-CoA desaturase 1 (SCD1), long-chain fatty acid elongases, and fatty acid desaturase two are capable of further modifying and elongating palmitic acid, thereby generating fatty acids with diverse chain lengths and degrees of saturation (Jeon et al., 2023). Fatty acids can be oxidized and decomposed under the catalysis of a series of enzymes, generating energy, with β - oxidation being the principal catabolic pathway, carnitine palmitoyltransferase 1 (CPT1), a crucial rate-limiting enzyme in fatty acid β-oxidation (Ngo et al., 2023). Malonyl - CoA decarboxylase (MCD) is an important enzyme in cellular fatty acid oxidation (Ussher et al., 2016). Clinically actionable targets in this rewired metabolism include:
FASN: As a key enzyme catalyzing de novo fatty acid synthesis, FASN is activated in pediatric osteosarcoma with lung metastasis, suggesting that FASN is an important target for pediatric thoracic solid tumors (Liu et al., 2012).
ACC: The ACC is also a rate-limiting enzyme for fatty acid synthesis. Targeted inhibition of ACC will block fatty acid synthesis, thereby promoting neuroblastoma differentiation and reducing tumor load (Ruiz-Perez et al., 2021).
CPT1: In neuroblastoma, the suppression of CPT1, a crucial rate-limiting enzyme in fatty acid β-oxidation, results in a reduced extent of fatty acid β-oxidation and subsequently inhibits tumor growth (Oliynyk et al., 2019).
MCD: In rhabdomyosarcoma models, pharmacological inhibition of malonyl-CoA decarboxylase disrupted this balance by elevating malonyl-CoA levels and suppressing fatty acid oxidation, ultimately arresting cell cycle progression and impairing proliferation (Miyagaki et al., 2021).
Pediatric thoracic solid tumors should be treated with a multidisciplinary approach. Targeting tumor blood vessels has emerged as an important strategy in tumor treatment, aimed at improving tumor blood supply, enhancing drug delivery, and increasing therapeutic effectiveness. However, targeting tumor vasculature still faces several challenges. One of the primary difficulties arises from the heterogeneous nature of tumor blood vessels. Tumors contain various cell types and tissues, which results in blood vessels with different morphological and functional characteristics across different regions of the tumor. This makes strategies to normalize tumor blood vessels more complex. The tumor microenvironment is composed of diverse cell types, including tumor cells, immune cells, and fibroblasts, which interact with the blood vessels in complex ways. These cells can release a variety of factors that influence the process of blood vessel normalization. Despite these challenges, ongoing research aims to develop more effective strategies to normalize tumor blood vessels and improve the delivery of tumor treatments. By better understanding the tumor vasculature and its interactions with the surrounding cells, scientists hope to overcome these obstacles and enhance the efficacy of vascular-targeted therapies. Tumor vascular normalization has emerged as a promising strategy to enhance the effectiveness of tumor treatments. However, Current methods to detect the therapeutic window for vascular normalization (histological/imaging techniques) face reproducibility and operational limitations, necessitating new biomarkers. Despite promising drugs, intrinsic/acquired resistance underscores the need to decipher vessel phenotypes and resistance mechanisms for personalized therapies. Recent clinical trials underscore the translational potential of vascular-targeting agents in pediatric thoracic tumors. For instance, bevacizumab, when combined with irinotecan, topotecan, or temozolomide in relapsed neuroblastoma, achieved a protocol-defined success criteria for overall response (complete or partial) rate and appeared to improve progression-free survival by normalizing aberrant vasculature and improving drug delivery (Moreno et al., 2024). Similarly, the multi-kinase inhibitor anlotinib reduced perfusion in pediatric soft tissue sarcomas, correlating with prolonged disease stabilization (Li, 2021). These findings highlight vascular modulation as a viable adjunct to cytotoxic regimens. By gaining deeper insights into these factors, we can develop more refined and personalized treatment strategies for patients, ultimately maximizing the chances of successful treatment outcomes for pediatric thoracic solid tumors.
The metabolic pathways of glycolysis and oxidative phosphorylation in tumor were highly complex and interconnected. For example, under the Warburg effect, tumor cells tend to favor glycolysis even when oxygen is abundant, yet oxidative phosphorylation within these cells is not entirely suppressed. The dynamic balance and switching mechanisms between these two pathways remain poorly understood. This complexity makes it challenging to precisely target metabolic pathways, as interventions aimed at a single metabolic step may trigger compensatory changes in other metabolic pathways. Such compensatory adaptations can undermine the effectiveness of targeted therapies, highlighting the need for a deeper understanding of these interconnected metabolic networks to develop more effective and specific therapeutic strategies. Metabolic interventions are gaining traction in pediatric oncology, with early-phase trials demonstrating feasibility. Notably, DCA restored mitochondrial respiration in neuroblastoma models, inhibits neuroblastoma growth by specifically acting against malignant undifferentiated cells—a critical advantage in pediatric populations (Vella et al., 2012). Amino acid and nucleic acid metabolic products play diverse roles in tumor. For instance, amino acids not only serve as building blocks for protein synthesis but also participate in cell signaling, energy metabolism, and various other processes. This multifunctionality complicates the development of drugs targeting amino acid metabolism, as inhibiting tumor cell growth may unintentionally affect other physiological processes. Genetic heterogeneity exists across different tumor types and even within the same tumor, leading to considerable variation in the reprogramming of amino acid and nucleic acid metabolism. For example, specific genetic mutations may alter the expression of amino acid transporters, thereby changing the intracellular amino acid metabolic state. This heterogeneity makes it nearly impossible to develop universal therapeutic strategies targeting amino acid and nucleic acid metabolism, necessitating personalized metabolic analysis and treatment design for each patient. Lipid metabolism encompasses the uptake, synthesis, and degradation of key lipid molecules, including fatty acids, phospholipids, and cholesterol, and these processes are continually dynamic in tumor. For instance, tumor cells can adapt the rates of lipid synthesis and degradation in response to nutrient availability and microenvironmental signals. This versatility and dynamism make it challenging to precisely identify the key regulatory points in lipid metabolism, which, in turn, complicates the development and application of targeted therapies aimed at lipid metabolism. The interplay between angiogenesis and metabolism offers untapped therapeutic synergies. Metabolic symbiosis induced by antiangiogenic therapy highlights cancer ability to evade therapeutic barriers by hijacking homeostatic mechanisms to fuel tumor evolution and undermine targeted treatments, dual inhibition of LDHA and VEGF receptors in murine models induced more tumor regression than monotherapy (Allen et al., 2016).
In summary, metabolic reprogramming encounters numerous challenges in the treatment of pediatric thoracic solid tumors. Although the metabolic pathways of pediatric thoracic solid tumors have been gradually discovered and continuously refined, most research has focused on tumor phenotypes rather than delving into downstream regulatory mechanisms. Given the complexity of metabolic mechanisms and the wide distribution of downstream targets for single regulatory factors, it is particularly important to identify the key metabolic dependency sites of tumors. It is hoped that future interdisciplinary approaches, utilizing multi-omics and organoid technologies, will provide new insights for targeted metabolic therapies in pediatric thoracic solid tumors, paving the way for the development of precision treatment strategies and improving patient prognosis.
YL: Conceptualization, Data curation, Writing–original draft, Writing–review and editing. Fanke Shu: Conceptualization, Data curation, Supervision, Writing–review and editing. DL: Data curation, Supervision, Writing–review and editing. RJ: Supervision, Writing–review and editing. YH: Data curation, Methodology, Supervision, Conceptualization, Writing–review and editing. CX: Conceptualization, Methodology, Supervision, Writing–review and editing.
The author(s) declare that no financial support was received for the research and/or publication of this article.
The authors declare that the research was conducted in the absence of any commercial or financial relationships that could be construed as a potential conflict of interest.
The author(s) declare that no Generative AI was used in the creation of this manuscript.
All claims expressed in this article are solely those of the authors and do not necessarily represent those of their affiliated organizations, or those of the publisher, the editors and the reviewers. Any product that may be evaluated in this article, or claim that may be made by its manufacturer, is not guaranteed or endorsed by the publisher.
Allen, E., Mieville, P., Warren, C. M., Saghafinia, S., Li, L., Peng, M. W., et al. (2016). Metabolic symbiosis enables adaptive resistance to anti-angiogenic therapy that is dependent on mTOR signaling. Cell Rep. 15 (6), 1144–1160. doi:10.1016/j.celrep.2016.04.029
Anderson, N. M., Qin, X., Finan, J. M., Lam, A., Athoe, J., Missiaen, R., et al. (2021). Metabolic enzyme DLST promotes tumor aggression and reveals a vulnerability to OXPHOS inhibition in high-risk neuroblastoma. Cancer Res. 81 (17), 4417–4430. doi:10.1158/0008-5472.CAN-20-2153
Bhakta, N., Force, L. M., Allemani, C., Atun, R., Bray, F., Coleman, M. P., et al. (2019). Childhood cancer burden: a review of global estimates. Lancet Oncol. 20 (1), e42–e53. doi:10.1016/S1470-2045(18)30761-7
Bishayee, K., Nazim, U. M., Kumar, V., Kang, J., Kim, J., Huh, S. O., et al. (2022). Reversing the HDAC-inhibitor mediated metabolic escape in MYCN-amplified neuroblastoma. Biomed. Pharmacother. 150, 113032. doi:10.1016/j.biopha.2022.113032
Broadfield, L. A., Pane, A. A., Talebi, A., Swinnen, J. V., and Fendt, S. M. (2021). Lipid metabolism in cancer: new perspectives and emerging mechanisms. Dev. Cell 56 (10), 1363–1393. doi:10.1016/j.devcel.2021.04.013
Bucklein, V. (2023). New treatment options for patients with Non-Hodgkin and Hodgkin lymphoma: changing treatment standards and improvements in prognosis. MMW Fortschr Med. 165 (1), 48–51. doi:10.1007/s15006-022-2185-0
Burkhardt, B., and Hermiston, M. L. (2019). Lymphoblastic lymphoma in children and adolescents: review of current challenges and future opportunities. Br. J. Haematol. 185 (6), 1158–1170. doi:10.1111/bjh.15793
Cao, Y. (2019). Adipocyte and lipid metabolism in cancer drug resistance. J. Clin. Invest 129 (8), 3006–3017. doi:10.1172/JCI127201
Casanova, M., Brennan, B., Alaggio, R., Kelsey, A., Orbach, D., van Noesel, M. M., et al. (2020). Inflammatory myofibroblastic tumor: the experience of the European pediatric soft tissue sarcoma study group (EpSSG). Eur. J. Cancer 127, 123–129. doi:10.1016/j.ejca.2019.12.021
Chen, S., Kelsey, A. M., and Rudzinski, E. R. (2024a). Rhabdomyosarcoma in children and young adults. Virchows Arch. 486, 101–116. doi:10.1007/s00428-024-03961-y
Chen, X., Yang, W., Roberts, C. W. M., and Zhang, J. (2024b). Developmental origins shape the paediatric cancer genome. Nat. Rev. Cancer 24 (6), 382–398. doi:10.1038/s41568-024-00684-9
Chmiel, P., Sl, O. A., Banaszek, L., Szumera, C. A., Szostakowski, B., Mj, S. P., et al. (2024). Inflammatory myofibroblastic tumor from molecular diagnostics to current treatment. Oncol. Res. 32 (7), 1141–1162. doi:10.32604/or.2024.050350
Dasgupta, R., Billmire, D., Aldrink, J. H., and Meyers, R. L. (2017). What is new in pediatric surgical oncology? Curr. Opin. Pediatr. 29 (1), 3–11. doi:10.1097/MOP.0000000000000439
De Felici, M., Klinger, F. G., Campolo, F., Balistreri, C. R., Barchi, M., and Dolci, S. (2021). To Be or not to Be a germ cell: the extragonadal germ cell tumor paradigm. Int. J. Mol. Sci. 22 (11), 5982. doi:10.3390/ijms22115982
Dehner, L. P., Schultz, K. A., and Hill, D. A. (2019). Pleuropulmonary blastoma: more than a lung neoplasm of childhood. Mo Med. 116 (3), 206–210.
Delprat, V., and Michiels, C. (2021). A bi-directional dialog between vascular cells and monocytes/macrophages regulates tumor progression. Cancer Metastasis Rev. 40 (2), 477–500. doi:10.1007/s10555-021-09958-2
Englisch, A., Altvater, B., Kailayangiri, S., Hartmann, W., and Rossig, C. (2020). VEGFR2 as a target for CAR T cell therapy of Ewing sarcoma. Pediatr. Blood Cancer 67 (10), e28313. doi:10.1002/pbc.28313
Garcia, J., Hurwitz, H. I., Sandler, A. B., Miles, D., Coleman, R. L., Deurloo, R., et al. (2020). Bevacizumab (Avastin®) in cancer treatment: a review of 15 years of clinical experience and future outlook. Cancer Treat. Rev. 86, 102017. doi:10.1016/j.ctrv.2020.102017
Greenberg, J. I., Shields, D. J., Barillas, S. G., Acevedo, L. M., Murphy, E., Huang, J., et al. (2008). A role for VEGF as a negative regulator of pericyte function and vessel maturation. Nature 456 (7223), 809–813. doi:10.1038/nature07424
Guan, J., Li, M., Wang, Y., Zhang, Y., Que, Y., Lu, S., et al. (2024). MTHFD1 regulates the NADPH redox homeostasis in MYCN-amplified neuroblastoma. Cell Death Dis. 15 (2), 124. doi:10.1038/s41419-024-06490-3
Guelfi, S., Hodivala-Dilke, K., and Bergers, G. (2024). Targeting the tumour vasculature: from vessel destruction to promotion. Nat. Rev. Cancer 24 (10), 655–675. doi:10.1038/s41568-024-00736-0
Guye, E., Lardy, H., Piolat, C., Bawab, F., Becmeur, F., Dyon, J. F., et al. (2007). Thoracoscopy and solid tumors in children: a multicenter study. J. Laparoendosc. Adv. Surg. Tech. A 17 (6), 825–829. doi:10.1089/lap.2007.0043
Hagenbuchner, J., Kiechl-Kohlendorfer, U., Obexer, P., and Ausserlechner, M. J. (2016). BIRC5/Survivin as a target for glycolysis inhibition in high-stage neuroblastoma. Oncogene 35 (16), 2052–2061. doi:10.1038/onc.2015.264
Horio, Y., Kuroda, H., Masago, K., Matsushita, H., Sasaki, E., and Fujiwara, Y. (2024). Current diagnosis and treatment of salivary gland-type tumors of the lung. Jpn. J. Clin. Oncol. 54 (3), 229–247. doi:10.1093/jjco/hyad160
Hulsker, C. C. C., El Mansori, I., Fiocco, M., Zsiros, J., Wijnen, M. H. W., Looijenga, L. H. J., et al. (2021). Treatment and survival of malignant extracranial germ cell tumours in the paediatric population: a systematic review and meta-analysis. Cancers (Basel) 13 (14), 3561. doi:10.3390/cancers13143561
Hung, M. S., Chen, I. C., Lin, P. Y., Lung, J. H., Li, Y. C., Lin, Y. C., et al. (2016). Epidermal growth factor receptor mutation enhances expression of vascular endothelial growth factor in lung cancer. Oncol. Lett. 12 (6), 4598–4604. doi:10.3892/ol.2016.5287
Jaggers, J., and Balsara, K. (2004). Mediastinal masses in children. Seminars Thorac. Cardiovasc. Surg. 16 (3), 201–208. doi:10.1053/j.semtcvs.2004.08.005
Jain, M., Nilsson, R., Sharma, S., Madhusudhan, N., Kitami, T., Souza, A. L., et al. (2012). Metabolite profiling identifies a key role for glycine in rapid cancer cell proliferation. Science 336 (6084), 1040–1044. doi:10.1126/science.1218595
Jain, R. K., Duda, D. G., Clark, J. W., and Loeffler, J. S. (2006). Lessons from phase III clinical trials on anti-VEGF therapy for cancer. Nat. Clin. Pract. Oncol. 3 (1), 24–40. doi:10.1038/ncponc0403
Janes, P. W., Parslow, A. C., Cao, D., Rigopoulos, A., Lee, F. T., Gong, S. J., et al. (2024). An anti-VEGF-B antibody reduces abnormal tumor vasculature and enhances the effects of chemotherapy. Cancers 16 (10), 1902. doi:10.3390/cancers16101902
Jaszai, J., and Schmidt, M. H. H. (2019). Trends and challenges in tumor anti-angiogenic therapies. Cells 8 (9), 1102. doi:10.3390/cells8091102
Jeon, Y. G., Kim, Y. Y., Lee, G., and Kim, J. B. (2023). Physiological and pathological roles of lipogenesis. Nat. Metab. 5 (5), 735–759. doi:10.1038/s42255-023-00786-y
Jiang, S., Li, H., Zhang, L., Mu, W., Zhang, Y., Chen, T., et al. (2025). Generic Diagramming Platform (GDP): a comprehensive database of high-quality biomedical graphics. Nucleic acids Res. 53 (D1), D1670–D1676. doi:10.1093/nar/gkae973
Jiang, S., Zhou, Y., Zou, L., Chu, L., Chu, X., Ni, J., et al. (2023b). Low-dose Apatinib promotes vascular normalization and hypoxia reduction and sensitizes radiotherapy in lung cancer. Cancer Med. 12 (4), 4434–4445. doi:10.1002/cam4.5113
Jiang, X., Wang, J., Deng, X., Xiong, F., Zhang, S., Gong, Z., et al. (2020). The role of microenvironment in tumor angiogenesis. J. Exp. Clin. Cancer Res. 39 (1), 204. doi:10.1186/s13046-020-01709-5
Jiang, Z., Zhou, J., Li, L., Liao, S., He, J., Zhou, S., et al. (2023a). Pericytes in the tumor microenvironment. Cancer Lett. 556, 216074. doi:10.1016/j.canlet.2023.216074
Kattner, P., Strobel, H., Khoshnevis, N., Grunert, M., Bartholomae, S., Pruss, M., et al. (2019). Compare and contrast: pediatric cancer versus adult malignancies. Cancer metastasis Rev. 38 (4), 673–682. doi:10.1007/s10555-019-09836-y
Knight, S., Knight, T., Khan, A., and Murphy, A. J. (2019). Current management of pleuropulmonary blastoma: a surgical perspective. Child. (Basel) 6 (8), 86. doi:10.3390/children6080086
Lam, C. G., Howard, S. C., Bouffet, E., and Pritchard-Jones, K. (2019). Science and health for all children with cancer. Science 363 (6432), 1182–1186. doi:10.1126/science.aaw4892
Le Grand, M., Mukha, A., Puschel, J., Valli, E., Kamili, A., Vittorio, O., et al. (2020). Interplay between MycN and c-Myc regulates radioresistance and cancer stem cell phenotype in neuroblastoma upon glutamine deprivation. Theranostics 10 (14), 6411–6429. doi:10.7150/thno.42602
Levy, A. G., Zage, P. E., Akers, L. J., Ghisoli, M. L., Chen, Z., Fang, W., et al. (2012). The combination of the novel glycolysis inhibitor 3-BrOP and rapamycin is effective against neuroblastoma. Invest New Drugs 30 (1), 191–199. doi:10.1007/s10637-010-9551-y
Levy, D. R., Agaram, N. P., Kao, C. S., Franks, S. E., Kesler, K. A., Stram, A. R., et al. (2021). Vasculogenic mesenchymal tumor: a clinicopathologic and molecular study of 55 cases of a distinctive neoplasm originating from mediastinal yolk sac tumor and an occasional precursor to angiosarcoma. Am. J. Surg. Pathol. 45 (4), 463–476. doi:10.1097/PAS.0000000000001615
Li, S. (2021). Anlotinib: a novel targeted drug for bone and soft tissue sarcoma. Front. Oncol. 11, 664853. doi:10.3389/fonc.2021.664853
Li, S., Zhang, Z., Tang, H., He, Z., Gao, Y., Ma, W., et al. (2017). Pathological complete response to gefitinib in a 10-year-old boy with EGFR-negative pulmonary mucoepidermoid carcinoma: a case report and literature review. Clin. Respir. J. 11 (3), 346–351. doi:10.1111/crj.12343
Liang, W. H., Federico, S. M., London, W. B., Naranjo, A., Irwin, M. S., Volchenboum, S. L., et al. (2020). Tailoring therapy for children with neuroblastoma on the basis of risk group classification: past, present, and future. JCO Clin. Cancer Inf. 4, 895–905. doi:10.1200/CCI.20.00074
Liu, Z. L., Wang, G., Peng, A. F., Luo, Q. F., Zhou, Y., and Huang, S. H. (2012). Fatty acid synthase expression in osteosarcoma and its correlation with pulmonary metastasis. Oncol. Lett. 4 (5), 878–882. doi:10.3892/ol.2012.862
Lujan, B., Hakim, S., Moyano, S., Nadal, A., Caballero, M., Diaz, A., et al. (2010). Activation of the EGFR/ERK pathway in high-grade mucoepidermoid carcinomas of the salivary glands. Br. J. Cancer 103 (4), 510–516. doi:10.1038/sj.bjc.6605788
Lupo, G., Caporarello, N., Olivieri, M., Cristaldi, M., Motta, C., Bramanti, V., et al. (2016). Anti-angiogenic therapy in cancer: downsides and new pivots for precision medicine. Front. Pharmacol. 7, 519. doi:10.3389/fphar.2016.00519
Mahajan, P., Casanova, M., Ferrari, A., Fordham, A., Trahair, T., and Venkatramani, R. (2021). Inflammatory myofibroblastic tumor: molecular landscape, targeted therapeutics, and remaining challenges. Curr. problems cancer 45 (4), 100768. doi:10.1016/j.currproblcancer.2021.100768
Mascarenhas, L., Chi, Y. Y., Hingorani, P., Anderson, J. R., Lyden, E. R., Rodeberg, D. A., et al. (2019). Randomized phase II trial of bevacizumab or temsirolimus in combination with chemotherapy for first relapse rhabdomyosarcoma: a report from the children's oncology group. J. Clin. Oncol. 37 (31), 2866–2874. doi:10.1200/JCO.19.00576
Mauz-Korholz, C., Stroter, N., Baumann, J., Botzen, A., Korholz, K., and Korholz, D. (2018). Pharmacotherapeutic management of pediatric lymphoma. Paediatr. drugs 20 (1), 43–57. doi:10.1007/s40272-017-0265-x
Meazza, C., and Gattuso, GJPM (2019). Multidisciplinary team approach for pediatric thoracic tumors: new horizons and oldies but goldies, 2. doi:10.21037/pm.2019.04.02
Metzger, M. L., and Mauz-Korholz, C. (2019). Epidemiology, outcome, targeted agents and immunotherapy in adolescent and young adult non-Hodgkin and Hodgkin lymphoma. Br. J. Haematol. 185 (6), 1142–1157. doi:10.1111/bjh.15789
Miyagaki, S., Kikuchi, K., Mori, J., Lopaschuk, G. D., Iehara, T., and Hosoi, H. (2021). Inhibition of lipid metabolism exerts antitumor effects on rhabdomyosarcoma. Cancer Med. 10 (18), 6442–6455. doi:10.1002/cam4.4185
Moreno, L., Weston, R., Owens, C., Valteau-Couanet, D., Gambart, M., Castel, V., et al. (2024). Bevacizumab, irinotecan, or topotecan added to temozolomide for children with relapsed and refractory neuroblastoma: results of the ITCC-SIOPEN BEACON-neuroblastoma trial. J. Clin. Oncol. official J. Am. Soc. Clin. Oncol. 42 (10), 1135–1145. doi:10.1200/JCO.23.00458
Mosbech, C. H., Rechnitzer, C., Brok, J. S., Rajpert-De Meyts, E., and Hoei-Hansen, C. E. (2014). Recent advances in understanding the etiology and pathogenesis of pediatric germ cell tumors. J. Pediatr. Hematol. Oncol. 36 (4), 263–270. doi:10.1097/MPH.0000000000000125
Mullen, C. J. R., Barr, R. D., and Franco, E. L. (2021). Timeliness of diagnosis and treatment: the challenge of childhood cancers. Br. J. cancer 125 (12), 1612–1620. doi:10.1038/s41416-021-01533-4
Myers, A. L., Orr, W. S., Denbo, J. W., Ng, C. Y., Zhou, J., Spence, Y., et al. (2012). Rapamycin-induced tumor vasculature remodeling in rhabdomyosarcoma xenografts increases the effectiveness of adjuvant ionizing radiation. J. Pediatr. Surg. 47 (1), 183–189. doi:10.1016/j.jpedsurg.2011.10.034
Myers, A. L., Williams, R. F., Ng, C. Y., Hartwich, J. E., and Davidoff, A. M. (2010). Bevacizumab-induced tumor vessel remodeling in rhabdomyosarcoma xenografts increases the effectiveness of adjuvant ionizing radiation. J. Pediatr. Surg. 45 (6), 1080–1085. doi:10.1016/j.jpedsurg.2010.02.068
Ngo, J., Choi, D. W., Stanley, I. A., Stiles, L., Molina, A. J. A., Chen, P. H., et al. (2023). Mitochondrial morphology controls fatty acid utilization by changing CPT1 sensitivity to malonyl-CoA. EMBO J. 42 (11), e111901. doi:10.15252/embj.2022111901
Oliynyk, G., Ruiz-Perez, M. V., Sainero-Alcolado, L., Dzieran, J., Zirath, H., Gallart-Ayala, H., et al. (2019). MYCN-Enhanced oxidative and glycolytic metabolism reveals vulnerabilities for targeting neuroblastoma. iScience 21, 188–204. doi:10.1016/j.isci.2019.10.020
Patel, R., Cooper, D. E., Kadakia, K. T., Allen, A., Duan, L., Luo, L., et al. (2024). Targeting glutamine metabolism improves sarcoma response to radiation therapy in vivo. Commun. Biol. 7 (1), 608. doi:10.1038/s42003-024-06262-x
Pearson, J. K., and Tan, G. M. (2015). Pediatric anterior mediastinal mass: a review article. Seminars Cardiothorac. Vasc. Anesth. 19 (3), 248–254. doi:10.1177/1089253215578931
Petursdottir, A., Sigurdardottir, J., Fridriksson, B. M., Johnsen, A., Isaksson, H. J., Hardardottir, H., et al. (2020). Pulmonary carcinoid tumours: incidence, histology, and surgical outcome. A population-based study. Gen. Thorac. Cardiovasc Surg. 68 (5), 523–529. doi:10.1007/s11748-019-01261-w
Presta, M., Chiodelli, P., Giacomini, A., Rusnati, M., and Ronca, R. (2017). Fibroblast growth factors (FGFs) in cancer: FGF traps as a new therapeutic approach. Pharmacol. Ther. 179, 171–187. doi:10.1016/j.pharmthera.2017.05.013
Riggi, N., Suva, M. L., and Stamenkovic, I. (2021). Ewing's sarcoma. N. Engl. J. Med. 384 (2), 154–164. doi:10.1056/NEJMra2028910
Ruiz-Perez, M. V., Sainero-Alcolado, L., Oliynyk, G., Matuschek, I., Balboni, N., Ubhayasekera, S., et al. (2021). Inhibition of fatty acid synthesis induces differentiation and reduces tumor burden in childhood neuroblastoma. iScience 24 (2), 102128. doi:10.1016/j.isci.2021.102128
Sanchez-Sanchez, A. M., Antolin, I., Puente-Moncada, N., Suarez, S., Gomez-Lobo, M., Rodriguez, C., et al. (2015). Melatonin cytotoxicity is associated to Warburg effect inhibition in ewing sarcoma cells. PLoS One 10 (8), e0135420. doi:10.1371/journal.pone.0135420
Sandler, G., and Hayes-Jordan, A. (2018). Chest wall reconstruction after tumor resection. Seminars Pediatr. Surg. 27 (3), 200–206. doi:10.1053/j.sempedsurg.2018.05.008
Shakya, S., Gromovsky, A. D., Hale, J. S., Knudsen, A. M., Prager, B., Wallace, L. C., et al. (2021). Altered lipid metabolism marks glioblastoma stem and non-stem cells in separate tumor niches. Acta Neuropathol. Commun. 9 (1), 101. doi:10.1186/s40478-021-01205-7
Shukrun, R., Golan, H., Caspi, R., Pode-Shakked, N., Pleniceanu, O., Vax, E., et al. (2019). NCAM1/FGF module serves as a putative pleuropulmonary blastoma therapeutic target. Oncogenesis 8 (9), 48. doi:10.1038/s41389-019-0156-9
Slodkowska, J., Hasleton, P., and Radomski, P. (2000). Expression of transforming growth factor beta 1 (TGF beta 1) and angiogenesis in broncho-pulmonary carcinoids. Pneumonol. Alergol. Pol. 68 (7-8), 319–326.
Snaebjornsson, M. T., Janaki-Raman, S., and Schulze, A. (2020). Greasing the wheels of the cancer machine: the role of lipid metabolism in cancer. Cell Metab. 31 (1), 62–76. doi:10.1016/j.cmet.2019.11.010
Su, Y., Luo, B., Lu, Y., Wang, D., Yan, J., Zheng, J., et al. (2022). Anlotinib induces a T cell-inflamed tumor microenvironment by facilitating vessel normalization and enhances the efficacy of PD-1 checkpoint blockade in neuroblastoma. Clin. cancer Res. official J. Am. Assoc. Cancer Res. 28 (4), 793–809. doi:10.1158/1078-0432.CCR-21-2241
Subbiah, V., Meric-Bernstam, F., Mills, G. B., Shaw, K. R., Bailey, A. M., Rao, P., et al. (2014). Next generation sequencing analysis of platinum refractory advanced germ cell tumor sensitive to Sunitinib (Sutent®) a VEGFR2/PDGFRβ/c-kit/FLT3/RET/CSF1R inhibitor in a phase II trial. J. Hematol. Oncol. 7, 52. doi:10.1186/s13045-014-0052-x
Summers, J., Cohen, M. H., Keegan, P., and Pazdur, R. (2010). FDA drug approval summary: bevacizumab plus interferon for advanced renal cell carcinoma. Oncologist 15 (1), 104–111. doi:10.1634/theoncologist.2009-0250
Talapatra, J., and Reddy, M. M. (2023). Lipid metabolic reprogramming in embryonal neoplasms with MYCN amplification. Cancers (Basel) 15 (7), 2144. doi:10.3390/cancers15072144
Tanner, J. M., Bensard, C., Wei, P., Krah, N. M., Schell, J. C., Gardiner, J., et al. (2017). EWS/FLI is a master regulator of metabolic reprogramming in ewing sarcoma. Mol. Cancer Res. 15 (11), 1517–1530. doi:10.1158/1541-7786.MCR-17-0182
Thacker, P. G., Iyer, R. S., Pace, E., States, L. J., and Guillerman, R. P. (2023). Imaging of pediatric pulmonary tumors: a cog diagnostic imaging committee/SPR oncology committee white paper. Pediatr. Blood Cancer 70 (Suppl. 4), e29964. doi:10.1002/pbc.29964
Tufail, M., Jiang, C. H., and Li, N. (2024). Altered metabolism in cancer: insights into energy pathways and therapeutic targets. Mol. Cancer 23 (1), 203. doi:10.1186/s12943-024-02119-3
Uprety, D., Halfdanarson, T. R., Molina, J. R., and Leventakos, K. (2020). Pulmonary neuroendocrine tumors: adjuvant and systemic treatments. Curr. Treat. Options Oncol. 21 (11), 86. doi:10.1007/s11864-020-00786-0
Ussher, J. R., Fillmore, N., Keung, W., Zhang, L., Mori, J., Sidhu, V. K., et al. (2016). Genetic and pharmacological inhibition of malonyl CoA decarboxylase does not exacerbate age-related insulin resistance in mice. Diabetes 65 (7), 1883–1891. doi:10.2337/db15-1145
van den Berg, H., van Rijn, R. R., and Merks, J. H. (2008). Management of tumors of the chest wall in childhood: a review. J. Pediatr. Hematol. Oncol. 30 (3), 214–221. doi:10.1097/MPH.0b013e318162bd54
Vella, S., Conti, M., Tasso, R., Cancedda, R., and Pagano, A. (2012). Dichloroacetate inhibits neuroblastoma growth by specifically acting against malignant undifferentiated cells. Int. J. Cancer 130 (7), 1484–1493. doi:10.1002/ijc.26173
Wahba, A., Wolters, R., and Foster, J. H. (2023). Neuroblastoma in the era of precision medicine: a clinical review. Cancers (Basel) 15 (19), 4722. doi:10.3390/cancers15194722
Wang, L., Yan, X., Zhao, J., Chen, C., Chen, C., Chen, J., et al. (2021). Expert consensus on resection of chest wall tumors and chest wall reconstruction. Transl. Lung Cancer Res. 10 (11), 4057–4083. doi:10.21037/tlcr-21-935
Wang, M., Qu, L., Du, X., Song, P., Ng, J. P. L., Wong, V. K. W., et al. (2024). Natural products and derivatives targeting metabolic reprogramming in colorectal cancer: a comprehensive review. Metabolites 14 (9), 490. doi:10.3390/metabo14090490
Weingart, S. N., Zhang, L., Sweeney, M., and Hassett, M. (2018). Chemotherapy medication errors. Lancet Oncol. 19 (4), e191–e199. doi:10.1016/S1470-2045(18)30094-9
Wu, C., Liu, Y., Liu, W., Zou, T., Lu, S., Zhu, C., et al. (2022). NNMT-DNMT1 Axis is essential for maintaining cancer cell sensitivity to oxidative phosphorylation inhibition. Adv. Sci. (Weinh) 10 (1), e2202642. doi:10.1002/advs.202202642
Wu, Y., He, Z., Li, S., Tang, H., Wang, L., Yang, S., et al. (2019). Gefitinib represses JAK-STAT signaling activated by CRTC1-MAML2 fusion in mucoepidermoid carcinoma cells. Curr. Cancer Drug Targets 19 (10), 796–806. doi:10.2174/1568009619666190103122735
Xia, Y., Ye, B., Ding, J., Yu, Y., Alptekin, A., Thangaraju, M., et al. (2019). Metabolic reprogramming by MYCN confers dependence on the serine-glycine-one-carbon biosynthetic pathway. Cancer Res. 79 (15), 3837–3850. doi:10.1158/0008-5472.CAN-18-3541
Xiang, H., Yang, R., Tu, J., Xi, Y., Yang, S., Lv, L., et al. (2023). Metabolic reprogramming of immune cells in pancreatic cancer progression. Biomed. Pharmacother. 157, 113992. doi:10.1016/j.biopha.2022.113992
Yang, C., Zhang, J., Liao, M., Yang, Y., Wang, Y., Yuan, Y., et al. (2021). Folate-mediated one-carbon metabolism: a targeting strategy in cancer therapy. Drug Discov. Today 26 (3), 817–825. doi:10.1016/j.drudis.2020.12.006
Yu, P., Wang, Y., Yuan, D., Sun, Y., Qin, S., and Li, T. (2023). Vascular normalization: reshaping the tumor microenvironment and augmenting antitumor immunity for ovarian cancer. Front. Immunol. 14, 1276694. doi:10.3389/fimmu.2023.1276694
Yu, Y., Ding, J., Zhu, S., Alptekin, A., Dong, Z., Yan, C., et al. (2021). Therapeutic targeting of both dihydroorotate dehydrogenase and nucleoside transport in MYCN-amplified neuroblastoma. Cell Death Dis. 12 (9), 821. doi:10.1038/s41419-021-04120-w
Zapala, M. A., Ho-Fung, V. M., and Lee, E. Y. (2017). Thoracic neoplasms in children: contemporary perspectives and imaging assessment. Radiol. Clin. North Am. 55 (4), 657–676. doi:10.1016/j.rcl.2017.02.008
Zarrabi, A., Perrin, D., Kavoosi, M., Sommer, M., Sezen, S., Mehrbod, P., et al. (2023). Rhabdomyosarcoma: current therapy, challenges, and future approaches to treatment strategies. Cancers (Basel) 15 (21), 5269. doi:10.3390/cancers15215269
Zhang, F., Guo, J., Yu, S., Zheng, Y., Duan, M., Zhao, L., et al. (2024). Cellular senescence and metabolic reprogramming: unraveling the intricate crosstalk in the immunosuppressive tumor microenvironment. Cancer Commun. (Lond) 44 (9), 929–966. doi:10.1002/cac2.12591
Zheng, M., Kumar, A., Sharma, V., Behl, T., Sehgal, A., Wal, P., et al. (2024). Revolutionizing pediatric neuroblastoma treatment: unraveling new molecular targets for precision interventions. Front. Cell Dev. Biol. 12, 1353860. doi:10.3389/fcell.2024.1353860
Keywords: pediatric, thoracic, tumor, angiogenesis, metabolism
Citation: Lv Y, Shu F, Luo D, Jia R, Huang Y and Xu C (2025) Targeting tumor angiogenesis and metabolism: a new perspective in pediatric thoracic tumor therapy. Front. Cell Dev. Biol. 13:1558403. doi: 10.3389/fcell.2025.1558403
Received: 10 January 2025; Accepted: 10 March 2025;
Published: 27 March 2025.
Edited by:
Kaiying Yang, Department of Pediatric Surgery, Guangzhou Women and Children’s Medical Center, ChinaReviewed by:
Ambra A Grolla, Department of Pharmaceutical Sciences, University of Eastern Piedmont, ItalyCopyright © 2025 Lv, Shu, Luo, Jia, Huang and Xu. This is an open-access article distributed under the terms of the Creative Commons Attribution License (CC BY). The use, distribution or reproduction in other forums is permitted, provided the original author(s) and the copyright owner(s) are credited and that the original publication in this journal is cited, in accordance with accepted academic practice. No use, distribution or reproduction is permitted which does not comply with these terms.
*Correspondence: YiDong Huang, YWRhbXNoeWRAMTYzLmNvbQ==; Chang Xu, aHVheGl4dWNoYW5nQDE2My5jb20=
†These authors have contributed equally to this work and share first authorship
Disclaimer: All claims expressed in this article are solely those of the authors and do not necessarily represent those of their affiliated organizations, or those of the publisher, the editors and the reviewers. Any product that may be evaluated in this article or claim that may be made by its manufacturer is not guaranteed or endorsed by the publisher.
Research integrity at Frontiers
Learn more about the work of our research integrity team to safeguard the quality of each article we publish.