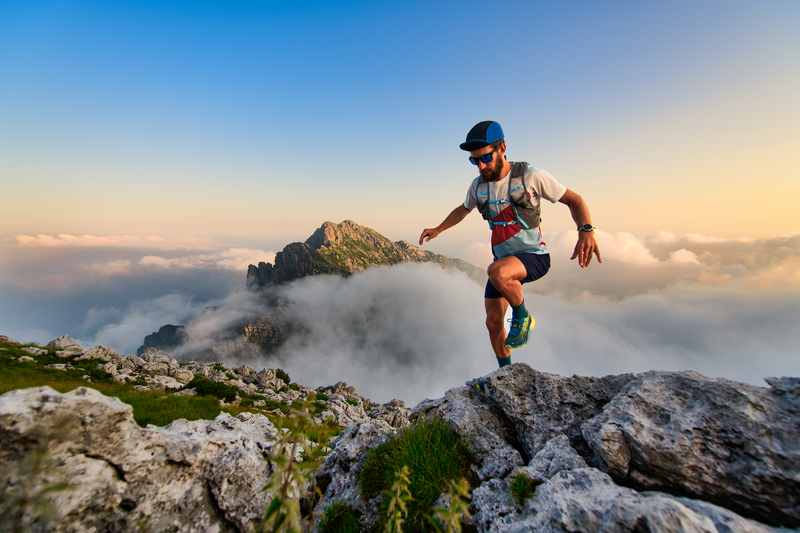
95% of researchers rate our articles as excellent or good
Learn more about the work of our research integrity team to safeguard the quality of each article we publish.
Find out more
REVIEW article
Front. Cell Dev. Biol. , 01 April 2025
Sec. Cancer Cell Biology
Volume 13 - 2025 | https://doi.org/10.3389/fcell.2025.1558393
This article is part of the Research Topic Innovative Tumor Therapies: Targeting Angiogenesis and Metabolism View all 5 articles
Photodynamic therapy (PDT) holds considerable promise as a tumor treatment modality, characterized by its targeted action, compatibility with other therapeutic approaches, and non - invasive features. PDT can achieve remarkable spatiotemporal precision in tumor ablation through the generation of reactive oxygen species (ROS). Nevertheless, despite its potential in tumor treatment, PDT encounters multiple challenges in practical applications. PDT is highly oxygen - dependent, and thus the effectiveness of PDT can be markedly influenced by tumor hypoxia. The co-existence of abnormal vasculature and metabolic deregulation gives rise to a hypoxic microenvironment, which not only sustains tumor survival but also undermines the therapeutic efficacy of PDT. Consequently, targeting tumor angiogenesis and metabolism is essential for revitalizing PDT. This review emphasizes the mechanisms and strategies for revitalizing PDT in tumor treatment, predominantly concentrating on interfering with tumor angiogenesis and reprogramming tumor cell metabolism. Lastly, the outlining future perspectives and current limitations of PDT are also summarized. This could provide new insights and methodologies for overcoming the challenges associated with PDT in tumor treatment, ultimately advancing the field of PDT.
Malignant tumors have threatened human health and are one of the major causes of death. Currently, tumor treatment modes in clinical practice are mainly based on surgery, radiation therapy and chemotherapy. However, in many cases, surgical treatment does not achieve complete resection of tumor tissues, and there is a risk of tumor metastasis and recurrence (Raskov et al., 2020). Additionally, radiotherapy and chemotherapy lack tumor specificity, resulting in significant toxic side effects that can damage normal tissues and lead to various adverse reactions in patients (Wang et al., 2024). Therefore, there is an urgent need to develop novel tumor treatment modalities to address the limitations of traditional therapeutic approaches. In recent years, emerging therapeutic modalities such as photodynamic therapy (PDT) have demonstrated significant potential in the treatment of malignant tumors. PDT has garnered attention for its minimal invasiveness, excellent spatiotemporal controllability, and high biocompatibility (Overchuk et al., 2023). PDT utilizes photosensitizers that selectively accumulate in tumor cells. Upon activation by specific light wavelengths, these photosensitizers generate reactive oxygen species (ROS), leading to the destruction of tumor cells. PDT revolutionizes oncological treatment through its cell-selective photochemical reactions, offering new dimensions for therapeutic optimization (Zolyniak-Brzuchacz et al., 2024). We review the latest strategies focused on two primary approaches, regulating tumor angiogenesis and disrupting tumor cell metabolism (Figure 1). Furthermore, the review outlines future perspectives and current limitations of PDT, highlighting the challenges that remain in optimizing these strategies for clinical use. These insights not only provide a comprehensive overview of potential interventions, but also serve as a guide to effectively translating PDT into clinical oncology practice.
Figure 1. Schematic illustration of different strategies for rejuvenating photodynamic therapy. (A) Nanoparticles passively accumulate in tumors due to the enhanced permeability and retention (EPR) effect, where they can be activated with light to produce ROS; (B) The latest strategies for rejuvenating PDT, from vascular normalization, regulating extracellular matrix (ECM), and metabolic reprogramming to reverse hypoxia.
PDT is a therapeutic method in which a photosensitizer (PS), stimulated by an external light source, converts oxygen inside the tumor cells into toxic ROS, which induces cell death by oxidizing intracellular biomolecules (Wang K. et al., 2022). Upon activation by light, the PS undergoes an energy transition from the ground state to a short-lived singlet state, which then undergoes intersystem crossing to a more stable triplet state (Jiang et al., 2023). From there, the PS can either release energy as heat or fluorescence, or interact with endogenous substances to generate free radicals, such as hydrogen peroxide and superoxide anion. The formation of ROS occurs through two mechanisms (Aebisher et al., 2024). Type I Mechanism, the PS participates in electron transfer processes, generating radicals. These radicals can react with oxygen to produce ROS such as superoxide anion (O2−), hydroxyl radical (OH·), and hydrogen peroxide (H2O2). Type II Mechanism, the PS transfers energy from its triplet state to molecular oxygen (3O2), resulting in the formation of highly reactive singlet oxygen (1O2), which is capable of inducing cell damage and death (Figure 2). Type I and type II reactions can be carried out simultaneously, and the ratio between them mainly depends on the photochemical and photophysical properties of PS and the concentrations of substrates and cellular oxygen (Yuan et al., 2021). Meanwhile, the two reactions can trigger different modes of cell death, in addition to the “conventional” cell death pathways such as apoptosis, necrosis and autophagy, other “unconventional” cell death modes such as ferroptosis and pyroptosis were also triggered. These findings provide new insights into the PDT-induced death signaling pathway (Mishchenko et al., 2022).
Figure 2. Schematic representation of photodynamic processes in tumor treatment. PS, Photosensitizer; S0, Ground state; S1, Excited singlet state; T1, Excited triplet state; ISC, Intersystem crossing.
Despite the promising potential of PDT in tumor treatment, several drawbacks have emerged during its clinical application, garnering increasing attention in current research. The hypoxic tumor microenvironment, the existence of metabolic reprogramming defense mechanism, and abnormal tumor angiogenesis greatly limit the efficacy of PDT, and make the clinical application of PDT face great challenges. Firstly, most PDT processes are oxygen-dependent, which often leads to reduced efficacy when treating hypoxic tumors (Srivastava et al., 2023). Secondly, tumor hypoxia primarily arises from structural abnormalities and functional dysregulation of tumor blood vessels, which lead to insufficient oxygen supply, thereby limiting the overall efficacy of photodynamic therapy (Guo et al., 2023). Thirdly, tumor metabolic abnormalities, such as aberrant glycolysis, abnormal mitochondrial metabolism, significantly influence the efficacy of PDT (Lin et al., 2024).
In recent years, various strategies have been reported to improve tumor hypoxia with the aim of enhancing the efficacy of PDT. One of the strategies lies in the nano-delivery systems that carry oxygen to ameliorate the hypoxic microenvironment in tumors (Luo et al., 2018). Another significant consideration is the supply of oxygen, strategies focuses on in situ oxygen generation within tumors to alleviate oxygen deficiency (Gao et al., 2020). In addition to oxygenation strategies, some studies have applied oxygen-independent PDT mechanisms to improve treatment outcomes (Yi et al., 2020). Recent advancements in oxygen-independent PDT leverage nanomaterials that generate cytotoxic free radicals under hypoxia. For example, AIE-active phenanthroimidazole derivatives (AQPO and AQPI) with minimized singlet–triplet splitting, which shift ROS production from oxygen-dependent singlet oxygen (Type-II) to hypoxia-tolerant free radicals (Type-I), demonstrating enhanced therapeutic efficacy in vivo (Xiao Y.-F. et al., 2022). This approach not only bypasses hypoxia limitations but also disrupts redox homeostasis, amplifying metabolic stress in tumors. Manganese oxide nanosystems catalytically convert H2O2 to O2, addressing hypoxia while enhancing PS activation (Liu et al., 2019). Furthermore, Mn2+-coordinated PSs exhibit photocatalytic O2 regeneration, sustaining ROS production in deep tumor regions, these systems synergize with anti-angiogenic therapies by normalizing vessel permeability and reducing VEGF expression (Zuo et al., 2024). However, studies aimed at improving the tumor hypoxic microenvironment by intervening in tumor angiogenesis and metabolism to enhance PDT have not received adequate attention.
Extracellular matrix (ECM), a structure that not only supports blood vessel formation and stability, but also plays an important role in tumor growth and metastasis (Yao et al., 2020). The ECM is a critical regulator of tumor hypoxia and therapeutic resistance. Excessive ECM deposition increases tumor stiffness and interstitial fluid pressure, impairing both oxygen diffusion and photosensitizer penetration into tumors (Hu et al., 2020). Emerging strategies now prioritize ECM remodeling to alleviate hypoxia and amplify PDT efficacy. For instance, Li et al. designed ultrasound-responsive nanoparticles (UNPS) that generate ROS under hyperbaric oxygen to degrade collagen, thereby reducing ECM density. This dual-action approach enhanced oxygen perfusion and nanoparticle penetration, achieving superior PDT effects even at low laser doses (Li et al., 2018). Similarly, Cheng et al. developed a CAF-reprogramming nanomaterial (FPC@S) that degrades ECM proteins via localized ROS while releasing SIS3 to suppress CAF-driven ECM overproduction. This dual modulation normalized tumor vasculature and oxygenation, creating a favorable microenvironment for PDT (Qiu et al., 2024). Deformable nanomaterials like T-PFRT further address spatial barriers: MMP2-responsive size-shrinking enables ECM penetration, while TGF-β inhibition (via LY364947) prevents pathological ECM deposition. Combined with oxygen-loaded hemoglobin (OxyHb) and photosensitizers, this platform synergistically alleviates hypoxia and enhances ROS generation (Liang et al., 2021). Pancreatic ductal adenocarcinoma (PDAC) presents a unique challenge for PDT due to its dense desmoplastic stroma and hypovascular microenvironment, which severely limits nanomedicine penetration and oxygen availability. Recent studies have addressed this by developing stroma-targeting nanoplatforms. For instance, iron oxide-based nanoparticles conjugated with collagenase-I were shown to degrade extracellular matrix components in PDAC models, enhancing PDT efficacy by improving both photosensitizer delivery and oxygen perfusion (Shah et al., 2022). Similarly, in glioblastoma multiforme (GBM), where the blood-brain barrier (BBB) restricts drug accumulation, BBB-penetrating nanoparticles loaded with chlorin e6 and catalase demonstrated dual functionality: they alleviated hypoxia via H2O2 decomposition while achieving deep-tissue PDT activation under MRI guidance (Wu C. et al., 2024).
Angiogenesis plays a pivotal role in tumorigenesis, serving as a prerequisite for tumor initiation and a foundation for its growth, invasion, and metastasis. Metabolic reprogramming refers to the process by which tumor cells alter their metabolic pathways to meet the demands of rapid proliferation and survival. Building on the identified challenges in PDT, this part explores emerging photodynamic nanotechnological approaches that demonstrate enhanced PDT efficacy, with key methodologies and comparative advantages summarized in Table 1.
Abnormal vascular structures within the tumor microenvironment often lead to inadequate accumulation of photosensitizers, thereby weakening the therapeutic efficacy of PDT (Yue et al., 2021). Tumors subjected to low-dose PDT not only fail to eliminate tumor cells but also activate signaling pathway molecules crucial for tumor survival, thereby facilitating tumor survival and recurrence (Wan et al., 2021). Therefore, how to solve the obstacles of photosensitizer delivery, improve the uptake and aggregation of photosensitizer in the tumor, reduce tumor recurrence, and thus improve the efficacy of photodynamic therapy is a scientific problem that needs to be solved urgently. Recent strategies have focused on combining PDT with anti-angiogenic agents, offering a potential therapeutic synergy. However, growing evidence suggests that anti-angiogenic drugs are unable to sustain long-term inhibition of tumor angiogenesis, and do not significantly improve long-term survival outcomes (Broekgaarden et al., 2015). Furthermore, excessive anti-angiogenic treatment can exacerbate tumor hypoxia, impairing PDT efficacy (Loges et al., 2009). In recent years, some approaches have emerged to improve photodynamic antitumor efficacy, including the use of vascular normalization strategies.
PDT-induced vascular damage acts as a double-edged sword in cancer therapy. The rapid generation of reactive oxygen species (ROS) during PDT induces endothelial cell apoptosis, platelet activation, and thrombosis, leading to vascular occlusion within minutes to hours post-treatment (Tao et al., 2022). While this “vascular shutdown” effectively starves tumors, surviving hypoxic cells at the tumor periphery often upregulate pro-angiogenic factors (e.g., VEGF, HIF-1α) and adopt aggressive metabolic phenotypes (e.g., enhanced glycolysis, lactate shuttling), driving recurrence and metastasis (Jung et al., 2021). To address this paradox, recent advances in photodynamic nanomedicine have focused on two synergistic strategies.
First, spatiotemporal control of vascular damage, nanoparticle-based PDT platforms (e.g., liposomes, polymeric micelles) enable precise spatial targeting of tumor vasculature while sparing normal tissues. For example, RGD peptide-modified nanoparticles selectively bind to αvβ3 integrins overexpressed on tumor endothelial cells, enhancing vascular disruption efficiency and reducing off-target effects (Javid et al., 2024). Additionally, light-triggered ROS generation from photosensitizers (e.g., chlorin e6) encapsulated in pH-responsive carriers allows controlled ROS release only within the acidic tumor microenvironment, minimizing premature vascular damage (Yang et al., 2018). Second, combinatorial vascular modulation, co-delivery of PDT agents with anti-angiogenic drugs (e.g., bevacizumab-conjugated nanoparticles) to prevent compensatory revascularization (Tangutoori et al., 2016). These strategies underscore the transformative potential of photodynamic nanomedicine in balancing acute vascular destruction with long-term metabolic regulation, ultimately mitigating relapse risks.
Tumors often exhibit rapid and aberrant angiogenesis, which results in malformed blood vessels that hinder the effective delivery of photosensitizers in PDT. Targeting these pathological blood vessels presents a rational therapeutic strategy (Yang et al., 2021). In 2005, Jain and colleagues introduced the concept of “vascular normalization,” suggesting that anti-angiogenic therapies, when administered within specific dosages and time windows, could restore balance in tumor vasculature, leading to more organized blood vessels, increased perfusion, and a temporary normalization of the tumor vasculature. This normalization alleviates tumor hypoxia, improves the delivery and accumulation of therapeutic agents, and enhances the overall therapeutic efficacy (Xiao M. et al., 2022). Vascular normalization has thus emerged as a promising approach to enhance PDT outcomes, ultimately improving PDT effectiveness (Figure 3).
Figure 3. Vascular normalization strategies for enhancing PDT tumor therapy. [Created with BioGDP.com (Jiang et al., 2025)].
A recent study reported a novel nanomaterial, SA-PEG-MPDA, which is loaded with indocyanine green and SPMI/3. After irradiation with 808 nm NIR light, this nanomaterial demonstrated significant antitumor efficacy. Moreover, SA-PEG-MPDA facilitated the normalization of tumor vasculature by increasing the coverage of laminin and pericytes, decreased the permeability of blood vessels, and improved the hypoxia of tissues, thus effectively enhancing the therapeutic effect of PDT (Xu et al., 2021). Previous studies have shown that the multi-targeted tyrosine kinase inhibitor, Lenvatinib, can inhibit vascular endothelial growth factor receptors (VEGFRs) and induce tumor vascular normalization (Liu et al., 2021). Tan et al. developed a Combo-NP, a nanomaterial loaded with lenvatinib. Upon near-infrared (NIR) light irradiation, the Combo-NP is cleaved by ROS, releasing lenvatinib. The released lenvatinib normalizes tumor blood vessels, reduces tumor hypoxia, and significantly enhances the effectiveness of photodynamic therapy (Zheng XQ. et al., 2023). Zhang et al. introduced a novel nanomaterial, LCL/ZnO, which demonstrates significant potential in tumor vascular normalization. LCL/ZnO promotes the normalization of abnormal tumor vasculature, reducing tumor hypoxia, and enhancing the overall therapeutic effect of PDT. Beyond merely normalizing tumor blood vessels, LCL/ZnO also extends the duration of the vascular normalization window, improving tumor vascular perfusion. The singlet oxygen (1O2) generated by LCL/ZnO activates signaling pathways in tumor-derived endothelial cells, including transient receptor potential vanilloid-4 (TRPV4) and endothelial nitric oxide synthase (eNOS), which contribute to vascular normalization (Zhang et al., 2022a).
Metabolic reprogramming is often characterized by enhanced glycolysis, increased lipid synthesis, and alterations in amino acid metabolism, enabling tumor cells to thrive in nutrient-deprived and hypoxic environments. PDT has emerged as a promising treatment modality, where a photosensitizer generates ROS under specific light irradiation to selectively induce tumor cell death. However, the metabolic adaptability of tumor cells may profoundly influence the efficacy of PDT. Recent studies suggest that metabolic reprogramming can not only affect the uptake and activation of photosensitizers but may also contribute to tumor microenvironmental hypoxia, limiting ROS generation. Therefore, exploring the impact of tumor metabolic reprogramming on photodynamic therapy can help provide new ideas and strategies to improve the clinical outcomes of PDT. This section will focus on recent metabolic reprogramming strategies closely associated with PDT.
Aerobic glycolysis stands as one of the most extensively studied metabolic reprogramming phenomena within tumors. This process was first identified by the German scientist Warburg, who observed that tumor even in an environment rich in oxygen, preferentially engage in aerobic glycolysis to sustain the rapid proliferation (Ma and Zong, 2020). Glycolysis allows tumor cells to competitively consume large amounts of glucose to fuel their growth (Wu D. et al., 2024). As tumor cells enhance their aerobic glycolysis, they produce substantial amounts of metabolic byproducts. This results in an accumulation of lactate and exacerbates hypoxia within the tumor microenvironment. Furthermore, photodynamic therapy, which induces oxygen consumption and microvascular damage, further contributes to the local hypoxia in the tumor (Zhou Y. et al., 2022). This hypoxic condition drives additional glycolytic activity, creating a vicious cycle. Therefore, targeting tumor glycolysis to enhance the efficacy of PDT in treating hypoxic tumors presents a highly feasible and promising approach (Figure 4). For example, Zhao et al. developed a tumor-targeting nanomaterial C&S/Fe@S-S-OSCLMs to enhance PDT efficacy by specifically inhibiting glycolysis. The loaded Sal-B component selectively suppresses cancer-specific HK2 and GLUT1, reducing normal tissue damage while regulating tumor glucose metabolism. The Sal-B and Fe3+ complex releases under high glutathione (GSH) in tumors, simultaneously inhibiting aerobic glycolysis and decreasing oxygen consumption. This dual action alleviates tumor hypoxia and significantly improves PDT therapeutic outcomes (Su et al., 2023). Lactate, an inevitable product of glycolysis, is both an important metabolic energy source and a key gluconeogenic precursor and signaling molecule. Lactic acid acts as both an energy donor and signaling molecule, promoting tumor metastasis. Its extracellular accumulation creates an acidic, hypoxic tumor microenvironment, compromising therapeutic efficacy. The presence of lactate in the tumor microenvironment is closely related to biological behaviors such as tumor cell growth, metastasis, neovascularization, and immune escape (Bogdanov et al., 2022). Therefore, targeted inhibition of lactate metabolism may be an effective strategy to enhance photodynamic antitumor therapy (Tian et al., 2022). The nanomaterial Dc&Ce6@MBNP designed by Zhou et al. carries the photosensitizer Ce6 and the lactate dehydrogenase inhibitor diclofenac (Dc). This nanomaterial releases Dc during PDT for tumor treatment, inhibiting the activity and expression of LDHA, reducing lactate production, and further weakening tumor cell glycolysis and angiogenesis, thus enhancing the therapeutic effect of PDT (Zhou Y. et al., 2022). Zhao et al. introduced a novel self-delivery ternary bioregulators (named TerBio). The construction of TerBio is based on the self-assembly of a photosensitizer (Ce6), a TGF-β antagonist SB505124 (SB), and the anti-tumor drug lonidamine (Lon). The release of SB and Lon effectively reprograms the tumor microenvironment (TME), inhibits the TGF-β signaling pathway and lactate (LA) efflux, thereby reversing the immunosuppressive microenvironment and enhancing the photodynamic anti-tumor effect (Zhao et al., 2022b).
Figure 4. Salvianolic acid B (Sal-B) alleviate the tumor hypoxia through the inhibition of the aerobic glycolysis and improve the antitumor performance of PDT. [Created with BioGDP.com (Jiang et al., 2025)].
Although tumor cells predominantly rely on glycolysis for energy production, mitochondrial oxidative phosphorylation (OXPHOS) also plays a crucial role. Numerous studies have shown that mitochondria can adapt to the metabolic demands of tumor cells, a phenomenon referred to as “mitochondrial plasticity” (Ward and Thompson, 2012). The mitochondrial OXPHOS, remains indispensable for tumor cell growth and proliferation. While most tumor cells rely on aerobic glycolysis, they also require OXPHOS to generate ATP. Some tumor cell can even switch flexibly from aerobic glycolysis to OXPHOS to meet energy demands (Smolkova et al., 2011). As the energy hub of the cell, mitochondria are the site of cellular respiration. Research has shown that mitochondrial-targeted PDT can effectively inhibit tumor ATP synthesis and reduce oxygen consumption, thereby reversing tumor hypoxia and accelerating tumor cell apoptosis (Zhao et al., 2020). Therefore, inhibition of mitochondrial OXPHOS could represent a promising strategy to enhance the efficacy of PDT (Figure 5). Wu et al. proposed a novel nanoparticle platform (TNPs/IA) designed to alleviate the hypoxic environment of tumors by targeting OXPHOS, which in turn enhances the efficacy of PDT. The nanomaterial enhances PDT through encapsulated atovaquone by inhibiting OXPHOS activity and reducing the oxygen consumption rate to alleviate tumor hypoxia, thereby improving ROS generation (Gao et al., 2024). Shen et al. developed MB@Bu@MnO2 nanoparticles to enhance PDT by targeting mitochondrial oxidative phosphorylation. The butformin (Bu) component inhibits tumor cell oxygen consumption, alleviating tumor hypoxia, while methylene blue (MB) generates cytotoxic ROS under laser irradiation to induce immunogenic cell death. This dual-action system synergistically improves therapeutic outcomes (Zhou Z. et al., 2022). Xu et al. proposed a multistage drug delivery nano-system for synergistic photodynamic therapy of tumors by sequential delivery of TPE-Py and RC. Mitochondria, the target organelles of TPE-Py, mediated the efficient aggregation of TPE-Py, and TPE-Py induced apoptosis and autophagy in tumor cells under light conditions. Meanwhile, Rubioncolin C, a natural naphthoquinone dimer, inhibited mitochondria-associated OXPHOS, which enhanced the PDT effect of TPE-Py by alleviating hypoxia (Wang J. et al., 2022).
Figure 5. Schematic diagram of the mitochondrial targeting nanoparticles for efficient photodynamic therapy against the solid tumor. [Created with BioGDP.com (Jiang et al., 2025)].
Glutamine is a non-essential amino acid and a precursor of many biomolecules, providing energy for cell growth, maintaining cell redox homeostasis, and playing an important role in cell growth and proliferation. Tumor cells have a significant increase in the demand for glutamine to satisfy their energy needs for rapid growth (Scalise et al., 2017). Glutamine is converted by aminotransferase into α-ketoglutarate (α-KG), which enters the tricarboxylic acid cycle (TCA) to provide energy and synthesize precursors for tumor cells (Mates et al., 2020). In 2021, Rathmell et al. reported that tumor cells even preferred glutamine to glucose for energy metabolism (Reinfeld et al., 2021). In addition, glutamine is a precursor of GSH, which helps tumor cells counteract oxidative stress, thus promoting their survival and proliferation (Bansal and Simon, 2018). Blocking glutamine metabolism in tumor cells reduces reductive substances and may enhance oxidative antitumor effects of PDT (Byun et al., 2020). A variety of mechanisms associated with glutathione metabolism inhibition in tumors can enhanced the antitumor effect of PDT (Figure 6). In light of this, Zhao and colleagues developed a nanomaterial, CeV, to enhance the antitumor efficacy of PDT (Zhao et al., 2022c). CeV utilizes a self-assembly technique to combine chlorine e6 (Ce6) with V9302, creating a novel nanomedicine platform. V9302 inhibits the alanine-serine-cysteine transporter of type-2, which significantly reduces glutamine uptake in tumor cells. This mechanism decreases intracellular GSH levels, leading to increased oxidative stress, which further amplifies the antitumor effects of PDT. In another study, BVC nanoparticles enhanced photodynamic therapy by targeting glutamine metabolism, inhibiting glutamine transport and glutathione synthesis, and inducing tumor immunogenic cell death (Zhao et al., 2023).
Figure 6. Strategies of intervention in glutamine metabolism for cancer photodynamic therapy. [Created with BioGDP.com (Jiang et al., 2025)].
Cholesterol is one of the essential molecules for eukaryotic cell survival and a key component of intracellular membrane structures. Cholesterol-derived metabolites also serve as important signaling molecules. Aberrant activation of the cholesterol biosynthetic pathway is a hallmark of many tumor, as it supports the rapid growth of tumor cells by providing the lipids required for cell membrane synthesis and facilitating the transmission of critical signaling pathways (Xue et al., 2020). Abnormal cholesterol metabolism in tumor cells is primarily characterized by increased cholesterol biosynthesis, enhanced exogenous cholesterol uptake, elevated cholesterol esterification and derivative production, as well as alterations in the tumor immune microenvironment (Chimento et al., 2018). In addition to directly influencing the biological behavior of tumor cells, cholesterol metabolism reprogramming also modulates the antitumor activity of immune cells within the tumor microenvironment. Cholesterol and its derivatives play a crucial role in the regulation of both innate and adaptive immunity, including processes such as monocyte expansion, neutrophil activation, NK cell activation, and T lymphocyte proliferation (Huang et al., 2020). Thus reprogramming cholesterol metabolism may enhance the therapeutic effect of PDT (Liu et al., 2023). H/S@hNP is a dual-drug-loaded nanoparticle that combines the photosensitizer hematoporphyrin monomethyl ether (HMME) and cholesterol synthesis inhibitor simvastatin (Figure 7). By depleting cholesterol, H/S@hNP blocks mechanical signaling between tumor cells and the ECM, thereby reducing the stiffness of the tumor stroma, facilitating drug penetration, and enhancing the efficacy of PDT (Peng et al., 2024). Zhang et al. designed a biological modulator, HN@CaCL-R, which successfully reversed the immunosuppressive state of the tumor microenvironment by regulating cholesterol metabolism, thereby enhancing the efficacy of PDT. HN@CaCL-R catalyzes the conversion of cholesterol to hydrogen peroxide (H2O2) within the tumor microenvironment, which enhances the luminescence of chemiluminescent reagent, further improving the effectiveness of PDT (Yin et al., 2023).
Figure 7. Scheme illustration of H/S@hNP-mediated cholesterol depletion strategy reduces tumor physical stiffness for enhanced photodynamic antitumor therapy (Peng et al., 2024).
The limited tissue penetration of activating light remains a fundamental challenge in PDT. Traditional PDT relies on ultraviolet-visible (UV-Vis) light (400–700 nm), which is strongly absorbed by hemoglobin and melanin, restricting penetration to superficial tissues (Fan et al., 2016). Although near-infrared (NIR) light (700–1,100 nm) offers deeper penetration, its lower energy reduces reactive oxygen species (ROS) generation efficiency (Ethirajan et al., 2011). Strategies like upconversion nanoparticles (UCNPs) and X-ray-activated scintillators have been explored to enable indirect excitation of photosensitizers (PSs) in deep tissues (Chen et al., 2014).
Tumor hypoxia severely limits PDT efficacy, as oxygen is essential for ROS generation via Type II photochemical reactions (Li M. L. et al., 2020). Hypoxia not only reduces ROS production but also upregulates hypoxia-inducible factor 1α (HIF-1α), promoting tumor survival and angiogenesis (Cheng et al., 2016). To address this, self-oxygenating nanosystems and Type I PSs have been developed (Cheng et al., 2015). Despite promising preclinical results, clinical translation remains hindered by inconsistent oxygenation effects and potential off-target metabolic disruptions.
Compared to anti-angiogenic agents (e.g., bevacizumab), PDT shows unique advantages but faces distinct challenges. Bevacizumab (anti-VEGF) normalizes tumor vasculature, enhancing drug delivery but exacerbating hypoxia (Willett et al., 2004). In contrast, PDT transiently disrupts vasculature while inducing immunogenic cell death (ICD), potentially synergizing with checkpoint inhibitors (Zheng X. et al., 2023). Clinical trials combining PDT with bevacizumab demonstrated improved outcomes in colorectal cancer but highlighted risks of systemic toxicity (Peng et al., 2018).
Malignant tumors are a leading global cause of death. While surgery remains primary treatment, current therapies for inoperable patients (e.g., organ dysfunction or advanced cases) often cause severe toxicities, highlighting the need for safer, more effective therapies. PDT shows promise but remains underdeveloped, hindered by tumor hypoxia, metabolic reprogramming, and abnormal vasculature. We review strategies targeting tumor vasculature and metabolism to enhance PDT efficacy and overcome clinical limitations.
The rationale for using nanomedicine in PDT lies in its ability to overcome critical limitations of traditional PDT while amplifying therapeutic efficacy through multifunctional design. First, nanocarriers enhance the delivery and bioavailability of hydrophobic PS, thereby improving solubility and prolonging circulation time for targeted accumulation in tumors (Rodrigues et al., 2020). Second, nanomedicine enables precise modulation of the tumor microenvironment, alleviating hypoxia and boosting ROS production during PDT (Di et al., 2023). Third, nanoplatforms facilitate combinatorial therapies, enabling organelle targeting to disrupt cancer cell metabolism and amplify ROS-induced apoptosis (Wu et al., 2023). Collectively, these strategies highlight pivotal role nanomedicine in optimizing PDT.
The concept of the tumor vascular normalization window—a transient phase post-anti-angiogenic therapy during which aberrant tumor vasculature acquires structural and functional stability—has emerged as a promising strategy to potentiate PDT. While preclinical studies demonstrate that synchronizing PDT with this window enhances therapeutic outcomes (Li Q. et al., 2020), its clinical translation remains hampered by the window’s elusive and transient nature. Current approaches rely on low-dose anti-angiogenic regimens to induce normalization, yet precise spatiotemporal control over drug delivery remains a critical unmet challenge (Shen et al., 2022). To address this, next-generation photosensitive nanomedicines are being engineered with three key features: (1) precision drug release to maintain tumor-localized, low-dose anti-angiogenic activity, thereby stabilizing vascular normalization; (2) sustained-release kinetics to prolong the therapeutic window; and (3) dose-tunable modulation to convert the indiscriminate “scissors” effect of anti-angiogenics into a “glue” effect that enhances pericyte coverage and vessel maturation (Shen et al., 2023).
Notably, the efficacy of PDT nanomedicines is further constrained by the tumor ECM, a dense physicochemical barrier that impedes nanoparticle penetration. While enzymatic ECM degradation, pharmacological inhibition, and hyperthermia-mediated ECM remodeling are under investigation, current strategies lack mechanistic depth. Most studies focus narrowly on collagen and hyaluronic acid, leaving other ECM components (e.g., fibrinogen, fibronectin) underexplored. Critically, no consensus exists on dominant signaling pathways governing ECM-PDT interactions, underscoring the need for multi-omics-driven target discovery and novel combinatorial approaches to modulate the tumor ECM-immune-metabolic axis.
Metabolic reprogramming, a hallmark of malignancy, presents another strategic lever to amplify PDT. While targeting tumor-specific metabolic vulnerabilities (e.g., dysregulated enzymes, nutrient transporters) holds potential, three key limitations persist: (1) incomplete mapping of tumor-specific metabolic networks due to gaps in single-cell metabolomics; (2) metabolic plasticity enabling therapeutic escape; and (3) off-target effects from metabolic inhibitors that disrupt normal cell physiology. To overcome these, next-generation PDT platforms must integrate dual/multi-pathway metabolic targeting—for instance, simultaneously inhibiting glycolysis and glutamine metabolism—to minimize compensatory adaptation. Furthermore, emerging “smart” nanomedicines with tumor microenvironment-responsive drug release could achieve selective metabolic targeting while sparing normal tissues. However, the interplay between tumor metabolism and immune cell function adds complexity: overzealous metabolic suppression may inadvertently cripple anti-tumor immunity. Thus, future designs must balance metabolic intervention with immune preservation, potentially through spatiotemporally controlled nano-delivery or orthogonal targeting of immune-metabolic checkpoints.
Despite these advances, clinical translation faces three major hurdles. First, the biocompatibility and long-term toxicity of multifunctional nanomaterials require rigorous evaluation using physiologically relevant models (e.g., orthotopic or patient-derived xenografts) rather than conventional subcutaneous tumors. Non-degradable nanoparticles (e.g., silica-based carriers) may accumulate in the liver/spleen, potentially causing chronic inflammation (Wei et al., 2024). Cationic lipid-based formulations can trigger complement-related hypersensitivity, as observed in failed Phase I trials of cationic porphyrin liposomes (Obaid et al., 2019). Second, the lack of standardized protocols for monitoring vascular normalization windows in humans necessitates innovative imaging biomarkers or liquid biopsy-based tools. Third, deeper mechanistic studies are needed to unravel how nanomaterials interact with tumor stroma, metabolism, and immune cells at single-cell resolution.
The integration of photodynamic therapy (PDT) with nanotechnology has unlocked unprecedented opportunities for precision oncology. To inspire future developments, we outline key directions to expand the horizons of photodynamic nanomedicine in targeting tumor angiogenesis and metabolism. Develop stimuli-responsive nanocarriers that sequentially release anti-angiogenic drugs and activate photosensitizers under tumor-specific conditions (e.g., hypoxia, pH). Design “metabolic traps” that simultaneously achieve selective metabolic targeting while sparing normal tissues and enhance ROS generation through Fenton-like reactions. Integrate real-time oxygen sensors (e.g., phosphorescent porphyrins) into nanoplatforms to dynamically monitor and modulate TME oxygenation during treatment. The next-generation of photodynamic nanomedicine must embrace complexity—targeting angiogenesis and metabolism. By converging advances in materials science and systems biology, we can design multifunctional, patient-specific therapies that transcend traditional monotherapy limitations. Prioritizing translational studies and clinical-grade manufacturing will accelerate the transition from bench to bedside.
In conclusion, the convergence of vascular normalization, ECM remodeling, and metabolic reprogramming within PDT nanoplatforms represents a paradigm shift in oncology. By prioritizing tumor-selective multi-targeting, microenvironment-responsive drug release, and systems-level mechanistic validation, this strategy could transcend current limitations, ushering in a new era of precision photodynamic therapy.
YL: Conceptualization, Investigation, Visualization, Writing – original draft, Writing – review and editing. LP: Data curation, Investigation, Writing – original draft. BR: Conceptualization, Data curation, Investigation, Supervision, Writing – review and editing. BX: Conceptualization, Supervision, Writing – review and editing.
The author(s) declare that no financial support was received for the research and/or publication of this article.
The authors declare that the research was conducted in the absence of any commercial or financial relationships that could be construed as a potential conflict of interest.
The author(s) declare that no Generative AI was used in the creation of this manuscript.
All claims expressed in this article are solely those of the authors and do not necessarily represent those of their affiliated organizations, or those of the publisher, the editors and the reviewers. Any product that may be evaluated in this article, or claim that may be made by its manufacturer, is not guaranteed or endorsed by the publisher.
Aebisher, D., Rogoz, K., Mysliwiec, A., Dynarowicz, K., Wiench, R., Cieslar, G., et al. (2024). The use of photodynamic therapy in medical practice. Front. Oncol. 14, 1373263. doi:10.3389/fonc.2024.1373263
Bansal, A., and Simon, M. C. (2018). Glutathione metabolism in cancer progression and treatment resistance. J. Cell Biol. 217 (7), 2291–2298. doi:10.1083/jcb.201804161
Bogdanov, A., Bogdanov, A., Chubenko, V., Volkov, N., Moiseenko, F., and Moiseyenko, V. (2022). Tumor acidity: from hallmark of cancer to target of treatment. Front. Oncol. 12, 979154. doi:10.3389/fonc.2022.979154
Broekgaarden, M., Weijer, R., van Gulik, T. M., Hamblin, M. R., and Heger, M. (2015). Tumor cell survival pathways activated by photodynamic therapy: a molecular basis for pharmacological inhibition strategies. Cancer Metastasis Rev. 34 (4), 643–690. doi:10.1007/s10555-015-9588-7
Byun, J. K., Park, M., Lee, S., Yun, J. W., Lee, J., Kim, J. S., et al. (2020). Inhibition of glutamine utilization synergizes with immune checkpoint inhibitor to promote antitumor immunity. Mol. Cell 80 (4), 592–606. doi:10.1016/j.molcel.2020.10.015
Chen, G. Y., Qju, H. L., Prasad, P. N., and Chen, X. Y. (2014). Upconversion nanoparticles: design, nanochemistry, and applications in theranostics. Chem. Rev. 114 (10), 5161–5214. doi:10.1021/cr400425h
Cheng, H., Zhu, J. Y., Li, S. Y., Zeng, J. Y., Lei, Q., Chen, K. W., et al. (2016). An O2 self-sufficient biomimetic nanoplatform for highly specific and efficient photodynamic therapy. Adv. Funct. Mater 26 (43), 7847–7860. doi:10.1002/adfm.201603212
Cheng, Y. H., Cheng, H., Jiang, C. X., Qiu, X. F., Wang, K. K., Huan, W., et al. (2015). Perfluorocarbon nanoparticles enhance reactive oxygen levels and tumour growth inhibition in photodynamic therapy. Nat. Commun. 6, 8785. doi:10.1038/ncomms9785
Chimento, A., Casaburi, I., Avena, P., Trotta, F., De Luca, A., Rago, V., et al. (2018). Cholesterol and its metabolites in tumor growth: therapeutic potential of statins in cancer treatment. Front. Endocrinol. 9, 807. doi:10.3389/fendo.2018.00807
Di, Y., Deng, R., Liu, Z., Mao, Y., Gao, Y., Zhao, Q., et al. (2023). Optimized strategies of ROS-based nanodynamic therapies for tumor theranostics. Biomaterials 303, 122391. doi:10.1016/j.biomaterials.2023.122391
Ethirajan, M., Chen, Y. H., Joshi, P., and Pandey, R. K. (2011). The role of porphyrin chemistry in tumor imaging and photodynamic therapy. Chem. Soc. Rev. 40 (1), 340–362. doi:10.1039/b915149b
Fan, W., Huang, P., and Chen, X. (2016). Overcoming the Achilles’ heel of photodynamic therapy. Chem. Soc. Rev. 45 (23), 6488–6519. doi:10.1039/c6cs00616g
Gao, Y., Li, Y., Pan, Z., Xu, C., Zhang, X., Li, M., et al. (2024). OXPHOS-targeted nanoparticles for boosting photodynamic therapy against hypoxia tumor. Int. J. Pharm. 654, 123943. doi:10.1016/j.ijpharm.2024.123943
Gao, Z., Li, Y., Zhang, Y., Cheng, K., An, P., Chen, F., et al. (2020). Biomimetic platinum nanozyme immobilized on 2D metal-organic frameworks for mitochondrion-targeting and oxygen self-supply photodynamic therapy. ACS Appl. Mater. Interf. 12 (2), 1963–1972. doi:10.1021/acsami.9b14958
Guo, X., Niu, Y., Han, W., Han, X., Chen, Q., Tian, S., et al. (2023). The ALK1-Smad1/5-ID1 pathway participates in tumour angiogenesis induced by low-dose photodynamic therapy. Int. J. Oncol. 62 (4), 55. doi:10.3892/ijo.2023.5503
Hu, Q. L., Huang, Z. M., Duan, Y. K., Fu, Z. W., and Liu, B. (2020). Reprogramming tumor microenvironment with photothermal therapy. Bioconj. Chem. 31 (5), 1268–1278. doi:10.1021/acs.bioconjchem.0c00135
Huang, B., Song, B. L., and Xu, C. (2020). Cholesterol metabolism in cancer: mechanisms and therapeutic opportunities. Nat. Metab. 2 (2), 132–141. doi:10.1038/s42255-020-0174-0
Huang, J. X., Zhuang, C., Chen, J., Chen, X. M., Li, X. J., Zhang, T., et al. (2022). Targeted drug/gene/photodynamic therapy via a stimuli-responsive dendritic-polymer-based nanococktail for treatment of EGFR-TKI-resistant non-small-cell lung cancer. Adv. Mater. 34 (27), e2201516. doi:10.1002/adma.202201516
Javid, H., Oryani, M. A., Rezagholinejad, N., Esparham, A., Tajaldini, M., and Karimi-Shahri, M. (2024). RGD peptide in cancer targeting: benefits, challenges, solutions, and possible integrin-RGD interactions. Cancer Med. 13 (2), e6800. doi:10.1002/cam4.6800
Jian, H., Wang, X., Song, P., Wu, X., Zheng, R., Wang, Y., et al. (2022). Tumor microcalcification-mediated relay drug delivery for photodynamic immunotherapy of breast cancer. Acta Biomater. 140, 518–529. doi:10.1016/j.actbio.2021.12.014
Jiang, S., Li, H., Zhang, L., Mu, W., Zhang, Y., Chen, T., et al. (2025). Generic diagramming platform (GDP): a comprehensive database of high-quality biomedical graphics. Nucleic Acids Res. 53 (D1), D1670–D1676. doi:10.1093/nar/gkae973
Jiang, W., Liang, M., Lei, Q., Li, G., and Wu, S. (2023). The current status of photodynamic therapy in cancer treatment. Cancers (Basel) 15 (3), 585. doi:10.3390/cancers15030585
Jung, E., Lee, J., Lee, Y., Seon, S., Park, M., Song, C., et al. (2021). Tumor-targeting H2O2-responsive photosensitizing nanoparticles with antiangiogenic and immunogenic activities for maximizing anticancer efficacy of phototherapy. ACS Appl. Bio Mater 4 (5), 4450–4461. doi:10.1021/acsabm.1c00210
Li, J. Q., Huang, J. Z., Ao, Y. X., Li, S. Y., Miao, Y., Yu, Z. Z., et al. (2018). Synergizing upconversion nanophotosensitizers with hyperbaric oxygen to remodel the extracellular matrix for enhanced photodynamic cancer therapy. ACS Appl. Mater. Interf. 10 (27), 22985–22996. doi:10.1021/acsami.8b07090
Li, M. L., Shao, Y. J., Kim, J. H., Pu, Z. J., Zhao, X. Z., Huang, H. Q., et al. (2020). Unimolecular photodynamic O2-economizer to overcome hypoxia resistance in phototherapeutics. J. Am. Chem. Soc. 142 (11), 5380–5388. doi:10.1021/jacs.0c00734
Li, Q., Wang, Y., Jia, W., Deng, H., Li, G., Deng, W., et al. (2020). Low-dose anti-angiogenic therapy sensitizes breast cancer to PD-1 blockade. Clin. Cancer Res. 26 (7), 1712–1724. doi:10.1158/1078-0432.CCR-19-2179
Liang, T. X. Z., Zhang, B. H., Xing, Z. J., Dong, Y. X., Xu, H. M., Chen, X. Q., et al. (2021). Adapting and remolding: orchestrating tumor microenvironment normalization with photodynamic therapy by size transformable nanoframeworks. Angew. Chem-Int Ed. 60 (20), 11464–11473. doi:10.1002/anie.202102180
Lin, G., Tillman, L., Luo, T., Jiang, X., Fan, Y., Liu, G., et al. (2024). Nanoscale metal-organic layer reprograms cellular metabolism to enhance photodynamic therapy and antitumor immunity. Angewandte Chemie Int. Ed Engl. 63 (37), e202410241. doi:10.1002/anie.202410241
Liu, J., Chen, J., Liu, H., Zhang, K., Zeng, Q., Yang, S., et al. (2021). Bi/Se-Based nanotherapeutics sensitize CT image-guided stereotactic body radiotherapy through reprogramming the microenvironment of hepatocellular carcinoma. ACS Appl. Mater. Interf. 13 (36), 42473–42485. doi:10.1021/acsami.1c11763
Liu, X., Zhao, Z., Sun, X., Wang, J., Yi, W., Wang, D., et al. (2023). Blocking cholesterol metabolism with tumor-penetrable nanovesicles to improve photodynamic cancer immunotherapy. Small Methods 7 (5), e2200898. doi:10.1002/smtd.202200898
Liu, Y., Ma, X. X., Zhu, Y. J., Lv, X., Wang, P. P., and Feng, L. Z. (2022). pH-responsive nanomedicine co-encapsulated with Erlotinib and chlorin e6 can enable effective treatment of triple negative breast cancer via reprogramming tumor vasculature. Chem. Eng. J. 437, 135305. doi:10.1016/j.cej.2022.135305
Liu, Y., Pan, Y., Cao, W., Xia, F., Liu, B., Niu, J., et al. (2019). A tumor microenvironment responsive biodegradable CaCO(3)/MnO(2)- based nanoplatform for the enhanced photodynamic therapy and improved PD-L1 immunotherapy. Theranostics 9 (23), 6867–6884. doi:10.7150/thno.37586
Loges, S., Mazzone, M., Hohensinner, P., and Carmeliet, P. (2009). Silencing or fueling metastasis with VEGF inhibitors: antiangiogenesis revisited. Cancer Cell 15 (3), 167–170. doi:10.1016/j.ccr.2009.02.007
Luo, Z., Tian, H., Liu, L., Chen, Z., Liang, R., Chen, Z., et al. (2018). Tumor-targeted hybrid protein oxygen carrier to simultaneously enhance hypoxia-dampened chemotherapy and photodynamic therapy at a single dose. Theranostics 8 (13), 3584–3596. doi:10.7150/thno.25409
Ma, L., and Zong, X. (2020). Metabolic symbiosis in chemoresistance: refocusing the role of aerobic glycolysis. Front. Oncol. 10, 5. doi:10.3389/fonc.2020.00005
Mai, Z., Zhong, J., Zhang, J., Chen, G., Tang, Y., Ma, W., et al. (2023). Carrier-free immunotherapeutic nano-booster with dual synergistic effects based on glutaminase inhibition combined with photodynamic therapy. ACS Nano 17 (2), 1583–1596. doi:10.1021/acsnano.2c11037
Mates, J. M., Di Paola, F. J., Campos-Sandoval, J. A., Mazurek, S., and Marquez, J. (2020). Therapeutic targeting of glutaminolysis as an essential strategy to combat cancer. Semin. Cell Dev. Biol. 98, 34–43. doi:10.1016/j.semcdb.2019.05.012
Mishchenko, T., Balalaeva, I., Gorokhova, A., Vedunova, M., and Krysko, D. V. (2022). Which cell death modality wins the contest for photodynamic therapy of cancer? Cell Death Dis. 13 (5), 455. doi:10.1038/s41419-022-04851-4
Obaid, G., Jin, W., Bano, S., Kessel, D., and Hasan, T. (2019). Nanolipid formulations of benzoporphyrin derivative: exploring the dependence of nanoconstruct photophysics and photochemistry on their therapeutic index in ovarian cancer cells. Photochem Photobiol. 95 (1), 364–377. doi:10.1111/php.13002
Overchuk, M., Weersink, R. A., Wilson, B. C., and Zheng, G. (2023). Photodynamic and photothermal therapies: synergy opportunities for nanomedicine. ACS Nano 17 (9), 7979–8003. doi:10.1021/acsnano.3c00891
Peng, C. L., Lin, H. C., Chiang, W. L., Shih, Y. H., Chiang, P. F., Luo, T. Y., et al. (2018). Anti-angiogenic treatment (Bevacizumab) improves the responsiveness of photodynamic therapy in colorectal cancer. Photodiagnosis Photodyn. Ther. 23, 111–118. doi:10.1016/j.pdpdt.2018.06.008
Peng, Z., Yi, Y., Nie, Y., Wang, T., Tang, J., Hong, S., et al. (2024). Softening the tumor matrix through cholesterol depletion breaks the physical barrier for enhanced antitumor therapy. J. Control. Release 371, 29–42. doi:10.1016/j.jconrel.2024.05.027
Qin, X. H., Zhang, M. Z., Hu, X., Du, Q., Zhao, Z. P., Jiang, Y., et al. (2021). Nanoengineering of a newly designed chlorin e6 derivative for amplified photodynamic therapy via regulating lactate metabolism. Nanoscale 13 (27), 11953–11962. doi:10.1039/d1nr01083b
Qiu, Z. W., Zhong, Y. T., Lu, Z. M., Yan, N., Kong, R. J., Huang, J. Q., et al. (2024). Breaking physical barrier of fibrotic breast cancer for photodynamic immunotherapy by remodeling tumor extracellular matrix and reprogramming cancer-associated fibroblasts. ACS Nano 18 (13), 9713–9735. doi:10.1021/acsnano.4c01499
Raskov, H., Orhan, A., Salanti, A., and Gogenur, I. (2020). Premetastatic niches, exosomes and circulating tumor cells: early mechanisms of tumor dissemination and the relation to surgery. Int. J. Cancer 146 (12), 3244–3255. doi:10.1002/ijc.32820
Reinfeld, B. I., Madden, M. Z., Wolf, M. M., Chytil, A., Bader, J. E., Patterson, A. R., et al. (2021). Cell-programmed nutrient partitioning in the tumour microenvironment. Nature 593 (7858), 282–288. doi:10.1038/s41586-021-03442-1
Rodrigues, M. C., Vieira, L. G., Horst, F. H., de Araujo, E. C., Ganassin, R., Merker, C., et al. (2020). Photodynamic therapy mediated by aluminium-phthalocyanine nanoemulsion eliminates primary tumors and pulmonary metastases in a murine 4T1 breast adenocarcinoma model. J. Photochem. Photobiol. B, Biol. 204, 111808. doi:10.1016/j.jphotobiol.2020.111808
Scalise, M., Pochini, L., Galluccio, M., Console, L., and Indiveri, C. (2017). Glutamine transport and mitochondrial metabolism in cancer cell growth. Front. Oncol. 7, 306. doi:10.3389/fonc.2017.00306
Shah, N., Squire, J., Guirguis, M., Saha, D., Hoyt, K., Wang, K. K., et al. (2022). Deep-tissue activation of photonanomedicines: an update and clinical perspectives. Cancers 14 (8), 2004. doi:10.3390/cancers14082004
Shen, R., Jiang, Q., Li, P., Wang, D., Yu, C., Meng, T., et al. (2023). “Targeted plus controlled” - composite nano delivery system opens the tumor vascular and microenvironment normalization window for anti-tumor therapy. Int. J. Pharm. 647, 123512. doi:10.1016/j.ijpharm.2023.123512
Shen, R., Peng, L., Zhou, W., Wang, D., Jiang, Q., Ji, J., et al. (2022). Anti-angiogenic nano-delivery system promotes tumor vascular normalizing and micro-environment reprogramming in solid tumor. J. Control. Release 349, 550–564. doi:10.1016/j.jconrel.2022.07.015
Smolkova, K., Plecita-Hlavata, L., Bellance, N., Benard, G., Rossignol, R., and Jezek, P. (2011). Waves of gene regulation suppress and then restore oxidative phosphorylation in cancer cells. Int. J. Biochem. Cell Biol. 43 (7), 950–968. doi:10.1016/j.biocel.2010.05.003
Song, C. H., Zhang, X. Y., Cao, Z. C., Wei, Z., Zhou, M., Wang, Y. F., et al. (2023). Regulating tumor cholesterol microenvironment to enhance photoimmunotherapy in oral squamous cell carcinoma. Chem. Eng. J. 462, 142160. doi:10.1016/j.cej.2023.142160
Srivastava, N., Usmani, S. S., Subbarayan, R., Saini, R., and Pandey, P. K. (2023). Hypoxia: syndicating triple negative breast cancer against various therapeutic regimens. Front. Oncol. 13, 1199105. doi:10.3389/fonc.2023.1199105
Su, Y., Lu, K., Huang, Y., Zhang, J., Sun, X., Peng, J., et al. (2023). Targeting Warburg effect to rescue the suffocated photodynamic therapy: a cancer-specific solution. Biomaterials 294, 122017. doi:10.1016/j.biomaterials.2023.122017
Tangutoori, S., Spring, B. Q., Mai, Z., Palanisami, A., Mensah, L. B., and Hasan, T. (2016). Simultaneous delivery of cytotoxic and biologic therapeutics using nanophotoactivatable liposomes enhances treatment efficacy in a mouse model of pancreatic cancer. Nanomedicine 12 (1), 223–234. doi:10.1016/j.nano.2015.08.007
Tao, W., Zhao, D., Li, G., Li, L., Li, S., Ye, H., et al. (2022). Artificial tumor microenvironment regulated by first hemorrhage for enhanced tumor targeting and then occlusion for synergistic bioactivation of hypoxia-sensitive platesomes. Acta Pharm. Sin. B 12 (3), 1487–1499. doi:10.1016/j.apsb.2021.08.010
Tian, H., Zhou, L., Wang, Y., Nice, E. C., Huang, C., and Zhang, H. (2022). A targeted nanomodulator capable of manipulating tumor microenvironment against metastasis. J. Control. Release 348, 590–600. doi:10.1016/j.jconrel.2022.06.022
Wan, Y., Fu, L. H., Li, C., Lin, J., and Huang, P. (2021). Conquering the hypoxia limitation for photodynamic therapy. Adv. Mater 33 (48), e2103978. doi:10.1002/adma.202103978
Wang, J., Wu, H., Zhao, Q., Zou, Y., Ding, D., Yin, H., et al. (2022). Aggregation-induced emission photosensitizer synergizes photodynamic therapy and the inhibition of the NF-κB signaling pathway to overcome hypoxia in breast cancer. ACS Appl. Mater. Interf. 14 (26), 29613–29625. doi:10.1021/acsami.2c06063
Wang, J., Zhang, X., Xing, J., Gao, L., and Lu, H. (2024). Nanomedicines in diagnosis and treatment of prostate cancers: an updated review. Front. Bioeng. Biotechnol. 12, 1444201. doi:10.3389/fbioe.2024.1444201
Wang, K., Xu, Y., Chen, Z., Li, H., Hu, R., Qu, J., et al. (2022). NIR-II light-activated two-photon squaric acid dye with Type I photodynamics for antitumor therapy. Nanophotonics 11 (22), 5089–5100. doi:10.1515/nanoph-2022-0482
Wang, S., Jin, S., Li, G., Xu, M., Deng, D., Xiao, Z., et al. (2021). Transmucosal delivery of self-assembling photosensitizer–nitazoxanide nanocomplexes with fluorinated chitosan for instillation-based photodynamic therapy of orthotopic bladder tumors. ACS Biomater. Sci. Eng. 7 (4), 1485–1495. doi:10.1021/acsbiomaterials.0c01786
Ward, P. S., and Thompson, C. B. (2012). Metabolic reprogramming: a cancer hallmark even warburg did not anticipate. Cancer Cell 21 (3), 297–308. doi:10.1016/j.ccr.2012.02.014
Wei, K., Wu, Y., Zheng, X., Ouyang, L., Ma, G., Ji, C., et al. (2024). A light-triggered J-aggregation-regulated therapy conversion: from photodynamic/photothermal therapy to long-lasting chemodynamic therapy for effective tumor ablation. Angewandte Chemie Int. Ed Engl. 63 (23), e202404395. doi:10.1002/anie.202404395
Wen, J. X., Luo, Y., Gao, H., Zhang, L., Wang, X., Huang, J., et al. (2021). Mitochondria-targeted nanoplatforms for enhanced photodynamic therapy against hypoxia tumor. J. Nanobiotechnol. 19 (1), 440. doi:10.1186/s12951-021-01196-6
Willett, C. G., Boucher, Y., di Tomaso, E., Duda, D. G., Munn, L. L., Tong, R. T., et al. (2004). Direct evidence that the VEGF-specific antibody bevacizumab has antivascular effects in human rectal cancer. Nat. Med. 10 (2), 145–147. doi:10.1038/nm988
Wu, C., Chen, W., Yan, S., Zhong, J., Du, L., Yang, C., et al. (2024). MRI-guided photothermal/photodynamic immune activation combined with PD-1 inhibitor for the multimodal combination therapy of melanoma and metastases. Regen. Biomater. 11, rbae019. doi:10.1093/rb/rbae019
Wu, C., Jiao, Q. S., Wang, C. L., Zheng, Y. X., Pan, X. H., Zhong, W. Y., et al. (2023). Nanofibrillar peptide hydrogels for self-delivery of lonidamine and synergistic photodynamic therapy. Acta Biomater. 155, 139–153. doi:10.1016/j.actbio.2022.11.008
Wu, D., Li, M., Wang, M., Yan, Z., and Meng, Y. (2024). PCAF acetylates AIB1 to form a transcriptional coactivator complex to promote glycolysis in endometrial cancer. Front. Oncol. 14, 1442965. doi:10.3389/fonc.2024.1442965
Xiao, M., Shi, Y., Jiang, S., Cao, M., Chen, W., Xu, Y., et al. (2022). Recent advances of nanomaterial-based anti-angiogenic therapy in tumor vascular normalization and immunotherapy. Front. Oncol. 12, 1039378. doi:10.3389/fonc.2022.1039378
Xiao, Y.-F., Chen, W.-C., Chen, J.-X., Lu, G., Tian, S., Cui, X., et al. (2022). Amplifying free radical generation of AIE photosensitizer with small singlet–triplet splitting for hypoxia-overcoming photodynamic therapy. ACS Appl. Mater. Interf. 14 (4), 5112–5121. doi:10.1021/acsami.1c23797
Xu, X. L., Chen, M. X., Lou, X. F., Du, Y. Y., Shu, G. F., Qi, J., et al. (2021). Sialic acid-modified mesoporous polydopamine induces tumor vessel normalization to enhance photodynamic therapy by inhibiting VE-cadherin internalization. Chem. Eng. J. 414, 128743. doi:10.1016/j.cej.2021.128743
Xue, L., Qi, H., Zhang, H., Ding, L., Huang, Q., Zhao, D., et al. (2020). Targeting SREBP-2-regulated mevalonate metabolism for cancer therapy. Front. Oncol. 10, 1510. doi:10.3389/fonc.2020.01510
Yan, Y., Chen, B. L., Wang, Z. H., Yin, Q. Q., Wang, Y. Q., Wan, F. J., et al. (2021). Sequential modulations of tumor vasculature and stromal barriers augment the active targeting efficacy of antibody-modified nanophotosensitizer in desmoplastic ovarian carcinoma. Adv. Sci. 8 (3), 2002253. doi:10.1002/advs.202002253
Yang, T., Xiao, H., Liu, X., Wang, Z., Zhang, Q., Wei, N., et al. (2021). Vascular normalization: a new window opened for cancer therapies. Front. Oncol. 11, 719836. doi:10.3389/fonc.2021.719836
Yang, Z., Chen, Q., Chen, J., Dong, Z., Zhang, R., Liu, J., et al. (2018). Tumor-pH-Responsive dissociable albumin-tamoxifen nanocomplexes enabling efficient tumor penetration and hypoxia relief for enhanced cancer photodynamic therapy. Small 14 (49), e1803262. doi:10.1002/smll.201803262
Yao, H., Xu, K. K., Zhou, J. H., Zhou, L., and Wei, S. H. (2020). A tumor microenvironment destroyer for efficient cancer suppression. ACS Biomater. Sci. Eng. 6 (1), 450–462. doi:10.1021/acsbiomaterials.9b01544
Yi, C., Yu, Z., Ren, Q., Liu, X., Wang, Y., Sun, X., et al. (2020). Nanoscale ZnO-based photosensitizers for photodynamic therapy. Photodiagnosis Photodyn. Ther. 30, 101694. doi:10.1016/j.pdpdt.2020.101694
Yi, Y., Yu, M., Feng, C., Hao, H., Zeng, W., Lin, C., et al. (2022). Transforming “cold” tumors into “hot” ones via tumor-microenvironment-responsive siRNA micelleplexes for enhanced immunotherapy. Matter 5 (7), 2285–2305. doi:10.1016/j.matt.2022.04.032
Yin, N., Wang, Y., Liu, Y., Niu, R., Zhang, S., Cao, Y., et al. (2023). A cholesterol metabolic regulated hydrogen-bonded organic framework (HOF)-based biotuner for antibody non-dependent immunotherapy tailored for glioblastoma. Adv. Mater 35 (44), e2303567. doi:10.1002/adma.202303567
Yu, C. C., Wang, N. N., Chen, X. W., Jiang, Y., Luan, Y. X., Qin, W., et al. (2023). A photodynamic-mediated glutamine metabolic intervention nanodrug for triple negative breast cancer therapy. Mater Today Bio 19, 100577. doi:10.1016/j.mtbio.2023.100577
Yu, Q., Li, X., Wang, J., Guo, L. P., Gao, W. Y., and Huang, L. Q. (2024). Recent advances in reprogramming strategy of tumor microenvironment for rejuvenating photosensitizers-mediated photodynamic therapy. Small 20 (16), e2305708. doi:10.1002/smll.202305708
Yuan, B., Wu, H., Wang, H., Tang, B., Xu, J. F., and Zhang, X. (2021). A self-degradable supramolecular photosensitizer with high photodynamic therapeutic efficiency and improved safety. Angewandte Chemie Int. Ed Engl. 60 (2), 706–710. doi:10.1002/anie.202012477
Yue, D., Cai, X., Fan, M., Zhu, J., Tian, J., Wu, L., et al. (2021). An alternating irradiation strategy-driven combination therapy of PDT and RNAi for highly efficient inhibition of tumor growth and metastasis. Adv. Healthc. Mater. 10 (8), e2001850. doi:10.1002/adhm.202001850
Zhang, H., Yan, X. S., Zhang, Y. K., Bao, C. L., and Li, C. H. (2022b). An oxygen-economical nano-photosensitizer with a high photodynamic therapeutic outcome via simultaneous reduction of the cellular respiration and oxygen depletion of PDT. J. Mater. Chem. B 10 (24), 4623–4631. doi:10.1039/d2tb00309k
Zhang, S. Q., Li, Y. H., Li, Z. Y., Wang, G. X., Liao, A., Wang, J. R., et al. (2022a). Intelligent nanodelivery system-generated 1O2 mediates tumor vessel normalization by activating endothelial TRPV4-eNOS signaling. Small 18 (17), e2200038. doi:10.1002/smll.202200038
Zhao, L., Rao, X., Zheng, R., Huang, C., Kong, R., Yu, X., et al. (2023). Targeting glutamine metabolism with photodynamic immunotherapy for metastatic tumor eradication. J. Control. Release 357, 460–471. doi:10.1016/j.jconrel.2023.04.027
Zhao, L. P., Chen, S. Y., Zheng, R. R., Kong, R. J., Rao, X. N., Chen, A. L., et al. (2022a). Self-delivery nanomedicine for glutamine-starvation enhanced photodynamic tumor therapy. Adv. Healthc. Mater. 11 (3), e2102038. doi:10.1002/adhm.202102038
Zhao, L. P., Chen, S. Y., Zheng, R. R., Kong, R. J., Rao, X. N., Chen, A. L., et al. (2022c). Self-delivery nanomedicine for glutamine-starvation enhanced photodynamic tumor therapy. Adv. Healthc. Mater. 11 (3), e2102038. doi:10.1002/adhm.202102038
Zhao, L. P., Zheng, R. R., Chen, H. Q., Liu, L. S., Zhao, X. Y., Liu, H. H., et al. (2020). Self-delivery nanomedicine for O(2)-economized photodynamic tumor therapy. Nano Lett. 20 (3), 2062–2071. doi:10.1021/acs.nanolett.0c00047
Zhao, L. P., Zheng, R. R., Kong, R. J., Huang, C. Y., Rao, X. N., Yang, N., et al. (2022b). Self-delivery ternary bioregulators for photodynamic amplified immunotherapy by tumor microenvironment reprogramming. ACS Nano 16 (1), 1182–1197. doi:10.1021/acsnano.1c08978
Zheng, X., Shi, Y., Tang, D., Xiao, H., Shang, K., Zhou, X., et al. (2023). Near-infrared-II nanoparticles for vascular normalization combined with immune checkpoint blockade via photodynamic immunotherapy inhibit uveal melanoma growth and metastasis. Adv. Sci. (Weinh) 10 (35), e2206932. doi:10.1002/advs.202206932
Zheng, X. Q., Shi, Y. Y., Tang, D. S., Xiao, H. H., Shang, K., Zhou, X. Z., et al. (2023). Near-infrared-II nanoparticles for vascular normalization combined with immune checkpoint blockade via photodynamic immunotherapy inhibit uveal melanoma growth and metastasis. Adv. Sci. 10 (35), e2206932. doi:10.1002/advs.202206932
Zhou, Y., Tong, F., Gu, W., He, S., Yang, X., Li, J., et al. (2022). Co-delivery of photosensitizer and diclofenac through sequentially responsive bilirubin nanocarriers for combating hypoxic tumors. Acta Pharm. Sin. B 12 (3), 1416–1431. doi:10.1016/j.apsb.2021.12.001
Zhou, Z., Liu, Y., Song, W., Jiang, X., Deng, Z., Xiong, W., et al. (2022). Metabolic reprogramming mediated PD-L1 depression and hypoxia reversion to reactivate tumor therapy. J. Control. Release 352, 793–812. doi:10.1016/j.jconrel.2022.11.004
Zhu, D. M., Duo, Y. H., Suo, M., Zhao, Y. H., Xia, L. G., Zheng, Z., et al. (2020). Tumor-exocytosed exosome/aggregation-induced emission luminogen hybrid nanovesicles facilitate efficient tumor penetration and photodynamic therapy. Angew. Chem-Int Ed. 59 (33), 13836–13843. doi:10.1002/anie.202003672
Zolyniak-Brzuchacz, A., Barnas, E., Bartusik-Aebisher, D., and Aebisher, D. (2024). The use of photodynamic therapy in the treatment of endometrial cancer-A review of the literature. Int. J. Mol. Sci. 25 (16), 8772. doi:10.3390/ijms25168772
Keywords: vascular normalization, metabolic reprogramming, tumor hypoxia, photodynamic therapy, nanomedicine
Citation: Lv Y, Pu L, Ran B and Xiang B (2025) Targeting tumor angiogenesis and metabolism with photodynamic nanomedicine. Front. Cell Dev. Biol. 13:1558393. doi: 10.3389/fcell.2025.1558393
Received: 10 January 2025; Accepted: 24 March 2025;
Published: 01 April 2025.
Edited by:
Kaiying Yang, Guangzhou Women and Children’s Medical Center, ChinaCopyright © 2025 Lv, Pu, Ran and Xiang. This is an open-access article distributed under the terms of the Creative Commons Attribution License (CC BY). The use, distribution or reproduction in other forums is permitted, provided the original author(s) and the copyright owner(s) are credited and that the original publication in this journal is cited, in accordance with accepted academic practice. No use, distribution or reproduction is permitted which does not comply with these terms.
*Correspondence: Bo Xiang, eGJfc2N1LmVkdUBob3RtYWlsLmNvbQ==
†These authors have contributed equally to this work and share first authorship
Disclaimer: All claims expressed in this article are solely those of the authors and do not necessarily represent those of their affiliated organizations, or those of the publisher, the editors and the reviewers. Any product that may be evaluated in this article or claim that may be made by its manufacturer is not guaranteed or endorsed by the publisher.
Research integrity at Frontiers
Learn more about the work of our research integrity team to safeguard the quality of each article we publish.