- Laboratory of Developmental Cardiology, Institute of Physiology of the Czech Academy of Sciences, Prague, Czechia
Fat mass and obesity-associated (FTO) protein, a key enzyme integral to the dynamic regulation of epitranscriptomic modifications in RNAs, significantly influences crucial RNA lifecycle processes, including splicing, export, decay, and translation. The role of FTO in altering the epitranscriptome manifests across a spectrum of physiological and pathological conditions. This review aims to consolidate current understanding regarding the implications of FTO in health and disease, with a special emphasis on its involvement in obesity and non-communicable diseases associated with obesity, such as diabetes, cardiovascular disease, and cancer. It also summarizes the established molecules with FTO-inhibiting activity. Given the extensive impact of FTO on both physiology and pathophysiology, this overview provides illustrative insights into its roles, rather than an exhaustive account. A proper understanding of FTO function in human diseases could lead to new treatment approaches, potentially unlocking novel avenues for addressing both metabolic disorders and malignancies. The evolving insights into FTO’s regulatory mechanisms hold great promise for future advancements in disease treatment and prevention.
1 Discovering FTO and its function
In 1999, Fto was identified as one of the several genes deleted in mice with the Ft (Fused toes) mutation. It was named Fatso due to its large size, which is where the abbreviation Fto comes from (Peters et al., 1999). However, its role and function were unknown for a long time. In 2007, human genome-wide association studies (GWAS) revealed that single nucleotide polymorphisms (SNPs) in the human FTO gene were associated with increased body mass index (BMI) and obesity, which is where the gene and its product derive their full name now: fat mass and obesity-associated (Hinney et al., 2007; Frayling et al., 2007; Scuteri et al., 2007).
The initial mechanistic insights into FTO’s function emerged already in 2007, demonstrating that FTO could catalyze the 2-oxoglutarate-dependent oxidative demethylation of 3-methylthymine in single-stranded DNA and 3-methyluracil in single-stranded RNA in vitro (Gerken et al., 2007; Jia et al., 2008). However, the main biological substrate of FTO was unknown until 2011, when it was reported that FTO had efficient oxidative demethylation activity towards N6-methyladenosine (m6A), an abundant mRNA modification known from the 1970s (Jia et al., 2011; Desrosiers et al., 1974). This breakthrough discovery revealed the dynamic nature of mRNA modifications and renewed the interest of scientists in this field (Dieterich et al., 2021). However, in 2017, another study indicated that FTO preferentially demethylates N6,2′-O-dimethyladenosine (m6Am) rather than m6A (Mauer et al., 2017; Mauer and Jaffrey, 2018). Most recently, it was suggested that the substrate preference of FTO might depend on its cellular localization which varies between cell types. While m6A is the preferable target of FTO in the nucleus, cytosolic FTO demethylates particularly m6Am (Wei et al., 2018; Relier et al., 2021). Notably, this substrate selectivity may also be influenced by regulatory proteins such as ZBTB48 (zinc finger and BTB domain containing 48), a telomeric zinc finger protein that recruits FTO to specific m6A/m6Am-modified RNAs, thereby enhancing the specificity of FTO (Nabeel-Shah et al., 2024). Although FTO’s substrate preference appears context-dependent, the functional implications of these preferences across diseases are not fully understood. Further research is needed to clarify how FTO’s selective demethylation of m6A and m6Am influences pathophysiological processes, as distinct mechanisms may underlie FTO’s roles in metabolic, cardiovascular, and oncological disorders. Accurately distinguishing m6A- and m6Am-dependent pathways in specific disease contexts could provide valuable insights for targeted therapeutic strategies. However, due to the similarity of these modifications, some methods cannot differentiate between m6A and m6Am, complicating studies of FTO’s substrate-specific effects (Benak et al., 2023a). Besides m6A and m6Am, FTO has also an affinity to N1-methyladenosine (m1A) in transfer RNA (tRNA) (Mauer et al., 2017; Wei et al., 2018). Dynamic regulation of these epitranscriptomic modifications by FTO significantly affects the lifecycle of modified RNAs and consequently influences gene expression. Thus, epitranscriptomic regulations by FTO vastly affect cellular physiology and pathophysiology.
The timeline of discoveries related to FTO is summarized in Figure 1.
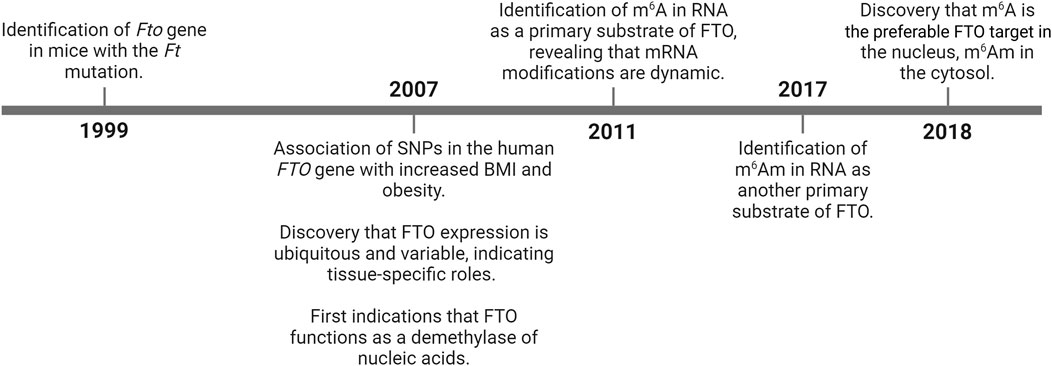
Figure 1. History of FTO research milestones. BMI – body mass index; FTO – fat mass and obesity-associated; m6A – N6-methyladenosine; m6Am – N6,2‘-O-dimethyladenosine; SNPs – single nucleotide polymorphisms.
2 Protein structure of FTO
The human FTO gene encodes a protein consisting of 505 amino acids with a molecular weight of 58,282 Da. Although the sequence of this protein is highly conserved across species, minor differences exist. For instance, the mouse Fto gene encodes a slightly shorter protein with 502 amino acids and a molecular weight of 58,007 Da. Similarly, the rat FTO protein also contains 502 amino acids, though its molecular weight is slightly lower at 57,972 Da (UniProt Consortium, 2023).
Structurally, FTO contains two main domains: the N-terminal domain (NTD) and the C-terminal domain (CTD) (Han et al., 2010). The NTD (residues 1–322) is catalytically active and includes binding sites for the metal cofactor, 2-oxoglutarate, and the methylated nucleobase. The CTD (residues 331–505 does not interact with FTO’s primary or secondary substrates but forms extensive contacts with the NTD (Khatiwada et al., 2022). The catalytic core of NTC is formed by a jelly-roll motif, characterized by a distorted double-stranded β-helix, which is supported on one side by two α-helices (α3 and α4) and on the other by a stabilizing loop between β5 and β6. The CTD’s structure includes a three-helix bundle with α7, α8, and α10, which extensively interacts with the NTD, providing essential structural stabilization (Han et al., 2010). This interaction between the NTD and CTD is necessary for FTO’s catalytic function, as the NTD alone is inactive. Disruption of the NTD-CTD interface leads to a loss of FTO’s catalytic capabilities, underscoring the essential role of inter-domain interactions in the enzyme’s function (Han et al., 2010; Khatiwada et al., 2022).
3 FTO in obesity
Obesity is characterized by an abnormal or excessive accumulation of fat, which can negatively impact health. High BMI is a major risk factor for many non-communicable diseases including diabetes, cardiovascular diseases (CVDs), and various cancers, which will be covered in the following chapters of this review. Unfortunately, the prevalence of overweight and obesity is reaching pandemic proportions, affecting 60%–70% of the adult population in industrialized countries and continuous to rise rapidly (Avgerinos et al., 2019; WHO. Obesity and overweight, 2021). Notably, FTO has been associated with obesity and each of the aforementioned diseases (Gholami, 2024).
The link between SNPs in the FTO gene and obesity has been uncovered through GWAS as mentioned in the previous chapter (Hinney et al., 2007; Frayling et al., 2007; Scuteri et al., 2007; Piwonska et al., 2022). Interestingly, most of these SNPs are in intronic regions, with introns 1 and 2 alone containing 89 identified variants. This observation has given rise to two hypotheses: either the introns within the FTO gene function as cis-regulatory sites affecting the expression of adjacent genes (such as IRX3 or RPGRIP1L), or they serve as auto-regulators for the FTO gene itself (Azzam et al., 2022; Smemo et al., 2014; Stratigopoulos et al., 2014; Berulava and Horsthemke, 2010). Interestingly, research has shown that male individuals carrying FTO risk alleles respond differently to weight management interventions compared to females, with exercise leading to greater weight loss in FTO risk allele carriers compared to those who do not carry these alleles, but only in males (Wang W. et al., 2022).
Experimental studies confirmed the link between FTO and obesity also in animal models. Loss of FTO in mice resulted in reduced body weight and lower fat mass, while higher levels of FTO caused the opposite (Fischer et al., 2009; Church et al., 2009; McMurray et al., 2013; Church et al., 2010).
In individuals carrying FTO risk variants, the observed increase in BMI was primarily associated with higher energy consumption and diminished food satiety, rather than reduced energy expenditure (Speakman et al., 2008; Haupt et al., 2009; Wardle et al., 2008; Cecil et al., 2008; Wardle et al., 2009). However, altered energy expenditure has been also reported in mice with Fto deletions (Wu et al., 2021).
FTO is ubiquitously expressed, but the most prominent expression occurs in the brain, particularly within the hypothalamic nuclei responsible for the regulation of energy balance, such as the arcuate nucleus. The hypothalamic nuclei play a central role in regulating appetite and energy balance by integrating hormonal and nutrient signals that influence feeding behavior and metabolic processes. The arcuate nucleus is a key hypothalamic region that contains specialized neurons responsive to hormones like leptin and ghrelin, which signal states of satiety and hunger, respectively. These nuclei modulate energy intake and expenditure, acting as central coordinators of body weight and fat accumulation (Dhillo, 2007).
Expression of FTO in the arcuate nucleus is influenced by feeding and fasting cycles (Gerken et al., 2007). Selective alteration of FTO levels in the arcuate nucleus was able to influence food intake in rats (Tung et al., 2010). Inhibition of hypothalamic FTO activated STAT3 (signal transducer and activator of transcription 3) through ERK1/2 (extracellular signal-regulated kinase 1/2), which resulted in reductions in food intake and body weight (Hu et al., 2023). Moreover, it was reported that FTO colocalizes with the long isoform of leptin receptor within the arcuate nucleus and that leptin administration can result in a reduction of hypothalamic FTO levels both in vitro and in vivo (Wang et al., 2011). Several studies demonstrated that FTO promoted the hypothalamic leptin resistance induced by high-fat diet (Tung et al., 2015; Liu et al., 2024). Also, risk variants of the human FTO gene were associated with higher serum leptin levels (Mehrdad et al., 2020; Genis-Mendoza et al., 2020; Magno et al., 2018). Besides leptin, a link between FTO and hunger hormone ghrelin has been suggested as Fto knockout (KO) mice exhibited higher circulating ghrelin levels after a 16-h overnight fast. Moreover, FTO overexpression in cell cultures (MGN3-1 and HEK293T cells) reduced m6A methylation of ghrelin mRNA (Ghrl) and resulted in its upregulation (Karra et al., 2013). This association was also observed in humans carrying risk FTO alleles, whose peripheral blood cells exhibited an increased abundance of FTO and GHRL mRNA (Karra et al., 2013). However, in women with morbid obesity, the FTO risk variant was associated with decreased ghrelin levels in the postprandial period (Magno et al., 2018). This intricate interplay highlights the pivotal role of FTO in the neuroendocrine regulation of appetite and energy homeostasis.
Besides the regulation of food consumption and food satiety, FTO also plays a role in adipogenesis. FTO expression gradually decreased while m6A levels steadily increased during adipogenesis. Moreover, FTO regulated alternative splicing of adipogenic regulatory factor RUNX1T1 (runt-related transcription factor 1) modulating preadipocyte differentiation (Zhao et al., 2014). Another study showed that Fto KO in 3T3-L1 cells inhibited preadipocyte differentiation, while its overexpression enhanced the process (Zhang et al., 2015). Other experiments on 3T3-L1 cells presented that KO of Fto was linked with higher m6A levels on transcripts of early mitotic events regulators (Ccna2, Cdk2) and also autophagy-related transcripts (Atg5, Atg7), which led to their degradation, resulting in impairment of cell-cycle progression (Wu et al., 2018), autophagy (Wang X. et al., 2020), and inhibition of adipogenesis (Wu et al., 2018; Wang X. et al., 2020). Further research indicated that FTO is a target of NADP (nicotinamide adenine dinucleotide phosphate), which increases its activity, promoting m6A demethylation and adipogenesis (Wang L. et al., 2020). Merkestein et al. (Merkestein et al., 2015) reported that primary adipocytes and mouse embryonic fibroblasts (MEFs) derived from FTO-4 mice (Fto overexpression) exhibited increased potential for adipogenic differentiation, whereas MEFs derived from Fto-KO mice displayed reduced adipogenesis. Also in this study, the effect of FTO on adipogenesis appeared to be mediated via enhanced expression of the pro-adipogenic short isoform of RUNX1T1 (Merkestein et al., 2015). FTO deficiency has been also associated with browning, the conversion of white adipocytes into adipocytes with brown fat characteristics. It has been reported that FTO inhibited the expression of UCP-1 (uncoupling protein 1) in adipocytes, inhibiting the transformation of adipose tissue into brown adipose tissue (Tews et al., 2013). In line with this, a risk variant of the human FTO gene has been associated with a cell-autonomous shift from white adipocyte browning and thermogenesis to lipid storage, resulting in increased fat stores and body-weight gain (Claussnitzer et al., 2015). A recent study revealed that loss of FTO in adipose tissue can increase the m6A methylation of Hif1a (hypoxia-inducible factor 1 α) mRNA, which is recognized by m6A-binding protein YTHDC2, which promotes its translation resulting in increased protein abundance of HIF-1α. This well-known transcription factor then activates the transcription of thermogenic genes (Ppaggc1a, Prdm16, Pparg) and promotes Ucp1 expression and the browning process (Wu et al., 2021).
Under physiological conditions, skeletal muscles serve as the main peripheral organs responsible for lipid utilization. Under pathological conditions, lipid accumulation becomes excessive. It has been suggested that AMPK (AMP-activated protein kinase) negatively regulates skeletal muscle lipid accumulation through FTO-dependent demethylation of m6A (Wu et al., 2017). Mechanistically, AMPK decreased FTO protein levels in C2C12 cells, therefore increasing m6A levels. A subsequent investigation revealed that m6A methylation upregulates skeletal muscle lipid accumulation, likely through upregulation of lipid synthase related genes and downregulation of lipolysis and oxidation related genes (Wu et al., 2017).
In conclusion, the FTO gene plays a pivotal role in the regulation of body weight and fat mass through complex mechanisms involving appetite regulation, energy balance, and adipogenesis (Figure 2). The intricate interplay between genetic variants of FTO, environmental factors, and metabolic pathways underscores the significance of this demethylase in the pathogenesis of obesity. Further research into the molecular mechanisms and potential therapeutic targets related to FTO could open new avenues for the prevention and treatment of obesity and its associated metabolic disorders.
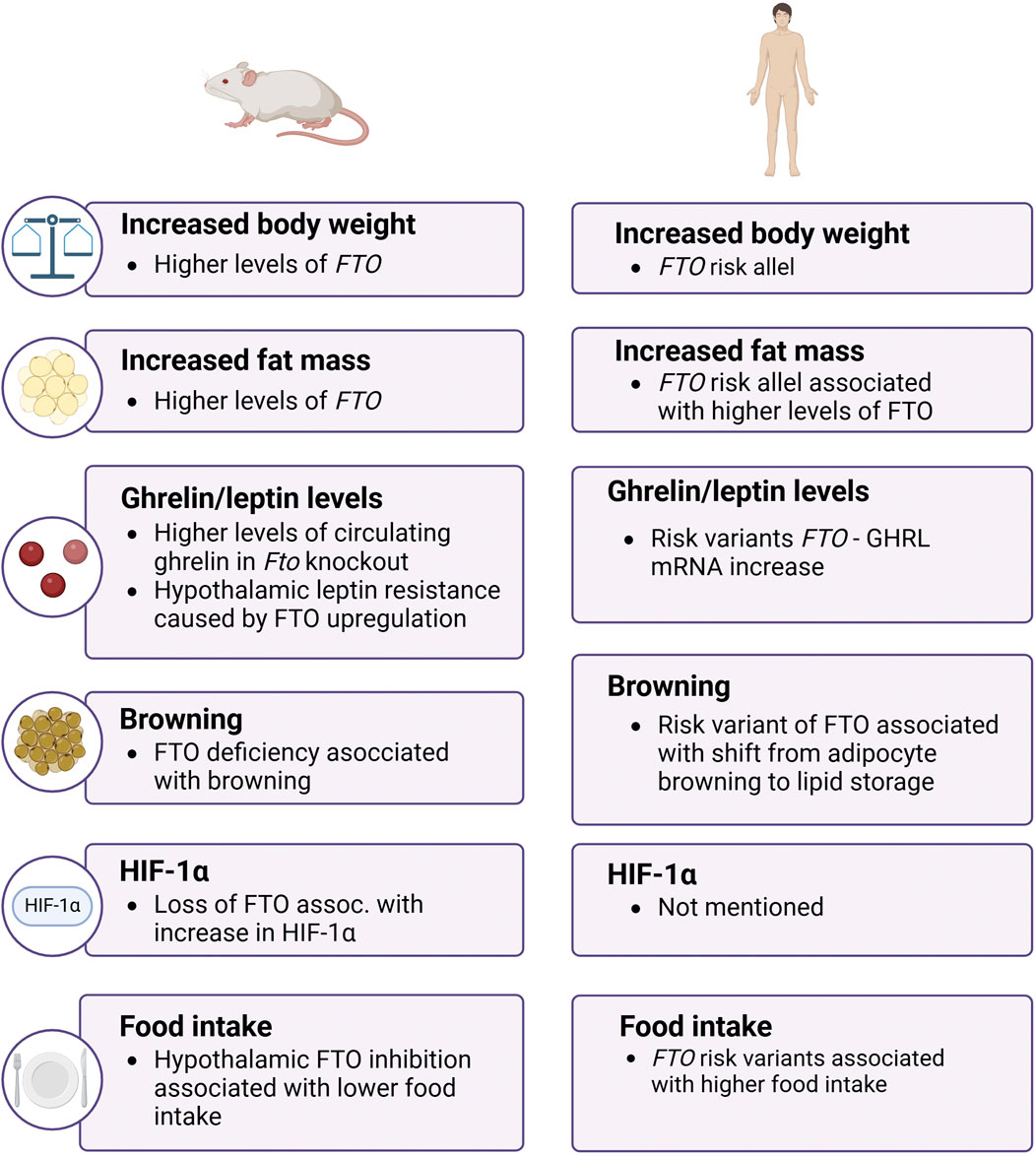
Figure 2. The role of FTO in obesity in mice and men. The image shows that FTO influences body weight, fat mass, and hormone levels in both mice and humans, with both species exhibiting increased obesity-related traits linked to FTO function. FTO – fat mass and obesity-associtated; GHRL – ghrelin; HIF-1α – hypoxia-inducible factor 1 α.
4 FTO in diabetes
Diabetes mellitus is a widespread chronic disease with an increasing number of cases worldwide (WHO, 2022). It is well-established that obesity is a major risk factor for type 2 diabetes mellitus (T2DM), as excess adipose tissue promotes insulin resistance through increased secretion of inflammatory cytokines, free fatty acids, and other metabolic byproducts that disrupt insulin signaling. Consequently, the risk of T2DM rises linearly with increases in body mass index (Klein et al., 2022). T2DM exceeds type 1 diabetes mellitus (T1DM) and gestational diabetes mellitus (GDM) in frequency and accounts for approximately 90% of all diabetes diagnoses (Goyal and Jialal, 2023; Babakhanian et al., 2022). This heterogeneous systemic disease is mainly characterized by two factors: insufficient insulin secretion by pancreatic β-cells and insulin resistance of insulin-sensitive tissues (Galicia-Garcia et al., 2020). Pancreatic β-cells are specialized cells within the islets of Langerhans that play a central role in regulating blood glucose levels by producing and secreting insulin. In response to rising blood glucose levels, β-cells release insulin, a hormone essential for glucose uptake by tissues and overall glucose homeostasis. This process is finely tuned by various cellular mechanisms that detect glucose and other metabolic cues to ensure timely insulin secretion. Disruptions in β-cell function can lead to insufficient insulin production, a key factor in the development of diabetes mellitus (Ashcroft and Rorsman, 2012). The subsequent chronic hyperglycemia (a characteristic feature of T2DM) damages glucose-sensitive organs and leads to subsequent impairment of vital functions (Malone and Hansen, 2019).
The progression from obesity to diabetes is linked to FTO’s dual influence on energy balance and adipose tissue, where its regulatory effects can contribute to systemic metabolic dysfunctions, such as insulin resistance, that characterize diabetes. FTO’s influence on adipogenesis, for example, may intensify lipid storage and impair insulin sensitivity in peripheral tissues, laying the groundwork for β-cell dysfunction and chronic hyperglycemia.
According to numerous studies, carriers of several SNPs in the human FTO gene are genetically predisposed to T2DM, T1DM, GDM, and chronic diabetic complications (Benak et al., 2023b; Hubacek et al., 2018a; Younus et al., 2017; Hubacek et al., 2023; Ghafarian-Alipour et al., 2018; Chaudhary et al., 2024; Mosaad et al., 2024; Zhang et al., 2023; Amin et al., 2023; Amine Ikhanjal et al., 2023; Zano and Baig, 2022). However, this association is still controversial with significant interethnic differences (Younus et al., 2017; Sabarneh et al., 2018; Sarkar et al., 2021; Bakhashab et al., 2020; Bazzi et al., 2014; Nasser et al., 2019; Chauhan et al., 2011; Bressler et al., 2010; Sanghera et al., 2008; Vasan et al., 2014; Yajnik et al., 2009; Yu H. et al., 2023). Moreover, some variants were found to be protective against diabetes (Bressler et al., 2010).
Gene expression analysis in pancreatic islets collected from healthy individuals and T2DM patients discovered downregulation of FTO in the diabetic group (De Jesus et al., 2019; Taneera et al., 2018; Kirkpatrick et al., 2010; Taneera et al., 2024). In contrast to these results, in vitro experiments revealed that high glucose concentrations in Min6 cells (mouse β-cell line) increased gene expression of Fto (Bornaque et al., 2022). Another study showed that increased production of this demethylase in Min6 cells promoted reactive oxygen species (ROS) generation and led to NF-κB (nuclear factor kappa-light-chain-enhancer of activated B cells) activation, which resulted in the inhibition of insulin secretion (Fan et al., 2015). Impaired insulin release was observed in INS-1 cells (rat insulinoma cell line) after Fto silencing, however, ROS production rate was not affected in this case (Taneera et al., 2024). On the contrary, overexpression of FTO in human islets promoted insulin secretion and increased protein levels of key β-cell proteins: INS, PDX1, MAFA, and GLUT1 (Taneera et al., 2024) The observed discrepancies between specific animal cell lines and diverse human islets could be attributed to variations between species or the inherent diversity within islet cell populations.
Besides the role of FTO in the diabetic islets of Langerhans, FTO has been also regulated in many other diabetic tissues. In db/db mice, which are used as a model of T2DM and diabetic cardiomyopathy, downregulation of cardiac FTO on both gene and protein levels was observed, resulting in elevated m6A levels (Ju et al., 2021). FTO was described to promote the progression of diabetic nephropathy (Sun et al., 2022), and several SNPs in the FTO gene were associated with a significantly lower risk of nephropathy in T2DM patients (Montesanto et al., 2018). FTO polymorphism was also linked to a higher risk of diabetic retinopathy (Hsiao et al., 2021). FTO expression was elevated in diabetic mice retinas and systemic administration of FTO inhibitor FB23-2 exhibited therapeutic efficacy in mice with diabetic retinopathy (Chen et al., 2024).
Importantly, increased FTO levels were detected in the peripheral blood of patients with T2DM (Shen et al., 2015; Onalan et al., 2022). Furthermore, a connection between high FTO levels and the severity of T2DM has been suggested (Masoud Abd El Gayed et al., 2021). In white blood cells of T2DM patients, a positive correlation between high FTO gene expression and fasting glucose concentration was found (Yang et al., 2019). Collectively, these findings suggest that FTO levels in peripheral blood could potentially serve as a novel biomarker for T2DM in the future.
In conclusion, FTO plays a critical role in the pathogenesis of diabetes, influencing insulin secretion, insulin sensitivity, and the development of diabetic complications through diverse mechanisms. The association between genetic variants of FTO and diabetes underscores the complexity of the disease and highlights the potential of FTO as a biomarker and therapeutic target in managing diabetes and its complications.
5 FTO in cardiovascular diseases
CVDs are the leading cause of death worldwide, with obesity significantly elevating risk through mechanisms like adipose tissue accumulation in the myocardium, as well as promoting a pro-inflammatory and prothrombotic state (Ashraf and Baweja, 2013). Globally, CVDs contribute to approximately 18 million deaths each year (WHO. Cardiovascular diseases, 2021). Current knowledge suggests that FTO plays a comprehensive role in cardiovascular health, impacting processes from the initial development of heart tissue to heart disease progression, making FTO an intriguing molecular target for potential clinical interventions.
The growth, proliferation, and differentiation of cardiomyocytes are critically dependent on the precise regulation of gene expression, with epitranscriptomic modifications increasingly recognized as vital contributors to these processes (Liu et al., 2023; Han et al., 2021; Yang C. et al., 2021). Levels of FTO are regulated throughout the development of the heart in a sex-dependent manner (Semenovykh et al., 2022; Krejčí et al., 2023). Loss-of-function mutation in the human FTO gene was associated with a range of heart defects (ventricular septal defect, atrioventricular defect, patent ductus arteriosus), as well as hypertrophic cardiomyopathy (Boissel et al., 2009). Moreover, variants of the FTO gene were linked with various CVDs, including hypertension, myocardial infarction (MI), acute coronary syndrome, and increased risk of rejection in heart transplant patients (Ahmad et al., 2010; He et al., 2014; Liu et al., 2013; Doney et al., 2009; Hubacek et al., 2016; Hubacek et al., 2010; Hubacek et al., 2018b). Regulation of FTO levels was observed in MI and heart failure (HF) patients and corresponding animal models (Mathiyalagan et al., 2019; Shi et al., 2021; Zhang et al., 2021a; Zhang et al., 2021b; Hinger et al., 2021; Wen et al., 2022; Wang X. et al., 2022; Vausort et al., 2022; Liu et al., 2022). However, it's important to note the possibility that the regulation of FTO in the heart could vary with age: downregulation of FTO levels was observed in response to acute myocardial ischemia-reperfusion injury in elderly murine hearts but remained unaffected in young hearts (Su et al., 2021).
FTO activity has been associated with cardiac hypertrophy. Global Fto KO in mice resulted in cardiac hypertrophy development (Carnevali et al., 2014). However, in vitro studies showed that cardiomyocyte hypertrophy can be triggered also by a leptin-induced increase in FTO levels, while FTO knockdown with siRNA abolished this effect (Gan et al., 2013). Transverse aortic constriction (TAC), an experimental model of pressure overload-induced cardiac hypertrophy and HF, was associated with a reduction of cardiac FTO levels, while FTO overexpression was shown to attenuate the cardiac dysfunction following TAC (Zhang et al., 2021a; Li W. et al., 2022), particularly by regulating glucose uptake and glycolysis (Zhang et al., 2021a). Mice with a cardiomyocyte-specific KO of Fto exhibited an impaired cardiac function manifesting with a more severe reduction in ejection fraction and a higher degree of left ventricular dilatation compared to wild-type animals upon TAC (Berulava et al., 2020). A recent study showed that the beneficial effects of cinnamic acid treatment of hypertrophy and HF in TAC mice are at least partially mediated by increasing FTO expression (Cui et al., 2023). In contrast to these data, the negative role of FTO has been documented in mice with HFpEF (heart failure with preserved ejection fraction), where upregulated FTO levels were reduced by exercise training. Overexpression of this demethylase then canceled the benefits of exercise and promoted myocyte hypertrophy, apoptosis, and myocardial fibrosis (Liu et al., 2022). The association of FTO and myocardial fibrosis was also documented by other studies, albeit with opposite results. In mouse models of MI, overexpression of Fto resulted in the reduction of fibrosis (Mathiyalagan et al., 2019). A recent study demonstrated that antifibrotic effects of leonurine in rat cardiac fibroblasts are also mediated through upregulation of FTO, which in turn leads to a reduction in m6A methylation levels (Meng et al., 2024).
Loss of FTO has been linked with a proarrhythmic remodeling of the heart. Global deficiency of this demethylase in mice resulted in a phenotype characterized by higher heart rate and heart rate variability, susceptibility to stress-induced tachyarrhythmias, and altered ventricular repolarization (Carnevali et al., 2014). According to a recent study, decreased FTO gene expression was an important predictor of atrial fibrillation in patients with metabolic syndrome (Rafaqat et al., 2024). Suppression of FTO was also linked to myocardial inflammation and dysfunction in mice during endotoxemia (Dubey et al., 2022). Furthermore, recent research has highlighted the role of FTO in mitigating septic cardiomyopathy through the suppression of ferroptosis, thereby alleviating heart inflammation and dysfunction (Zeng et al., 2024). However, another study demonstrated that inhibition of FTO using the LuHui Derivative (LHD) compound alleviated the inflammatory response and injury in hyperlipidemia-induced cardiomyopathy in rats (Yu et al., 2021).
Mice on HFD exhibited decreased cardiac FTO levels, which were reversed by long-lasting intermittent fasting, a well-known cardioprotective intervention (Xu et al., 2022). Similarly, a recent study demonstrated that FTO levels were elevated in the left ventricles of rats after a 3-day fasting period. Subsequent in vitro experiments revealed that cardiomyocytes isolated from fasted animals exhibited reduced hypoxic tolerance after FTO inhibition (Benak et al., 2024). Several other publications have described the positive impact of FTO on the tolerance of cardiomyocytes to hypoxia (Deng et al., 2021; Shen et al., 2021; Ke et al., 2022; Hlavackova et al., 2018). A recent study demonstrated that FTO targets sarcoplasmic/endoplasmic reticulum Ca2+ ATPase 2a (SERCA2a), leading to preservation of calcium homeostasis for myocardial contractile function in MI (Yang H. et al., 2024). FTO has also exhibited cardioprotective effects against the cardiotoxic effects of different drugs, such as sunitinib or doxorubicin (Ma et al., 2022; Yang Y. et al., 2024; Yu P. et al., 2023).
These data show that FTO exerts both beneficial and detrimental effects on the heart, depending on the underlying conditions. Thus, more studies are needed to elucidate the complex effects of FTO on the biology of the cardiovascular system.
6 FTO in cancer
Obesity is associated with an increased risk for a range of malignancies, largely due to altered fatty acid metabolism, extracellular matrix remodeling, secretion of adipokines and various hormones, immune dysregulation, and chronic inflammation. Although these mechanisms contribute to cancer development and recurrence, the exact relationship between obesity and cancer risk remains incompletely understood (Pati et al., 2023). According to the International Agency for Research on Cancer Working Group, there is convincing evidence that high body weight is linked to a higher risk for cancer of at least 13 anatomic sites, including endometrial, esophageal, renal and pancreatic adenocarcinomas, gastric cardia cancer, meningioma, multiple myeloma, colorectal, postmenopausal breast, ovarian, gallbladder, and thyroid cancers (Avgerinos et al., 2019; Lauby-Secretan et al., 2016). Given that FTO plays a crucial role in metabolism and obesity, it is not surprising that FTO dysregulation also significantly impacts tumorigenesis. Across a broad spectrum of cancer types, FTO is commonly found to be upregulated, serving as a crucial promoter of tumor progression (Li Y. et al., 2022). For instance, FTO has been reported as an oncogene in metastatic endometrial carcinoma, gastric cancer, bladder cancer, hepatocellular carcinoma, or acute myeloid leukemia; however, it also acts as an anti-oncogene in gastric cancer (An and Duan, 2022; Li et al., 2017).
Research into the relationship between FTO and cancer risk began shortly after SNPs in the human FTO gene were linked to obesity. However, establishing a straightforward link between polymorphic variants of FTO and cancer has proven difficult because many variables, such as ethnicity or the genetic origin of the tumors being compared (e.g., spontaneous vs hereditary tumors) (Azzam et al., 2022; Hernández-Caballero and Sierra-Ramírez, 2015). Despite this, several FTO SNPs were linked to higher or lower risk of cancers. For instance, already in 2009, Brennan et al. (Brennan et al., 2009) linked a variant of FTO gene to a lower risk of lung cancer and a slightly increased risk for kidney cancer. Later research associated FTO polymorphisms in some races with higher risks of pancreatic cancer (Tang et al., 2011; Pierce et al., 2011), endometrial and breast cancer (Delahanty et al., 2011; Lurie et al., 2011; Kaklamani et al., 2011), melanoma (Iles et al., 2013), or colorectal cancer (Gholamalizadeh et al., 2023).
The important hallmark of cancer is uncontrolled cell proliferation due to changes in metabolism and signaling pathways. These metabolic changes provide the energy and materials needed for cell growth and adaptation to tumor microenvironment. Recent studies showed that m6A modification is widely involved in the metabolic recombination of tumor cells (An and Duan, 2022). The mTOR (mammalian target of rapamycin) signaling pathway is a key regulator of tumor metabolism (Mirabilii et al., 2020). Several studies have already connected FTO to mTOR signaling (Li et al., 2018; Wang et al., 2017). For instance, stabilization of miR-139-5p by FTO supressed prostate cancer cell malignancies by inactivating the PI3/Akt/mTOR signal pathway (Azhati et al., 2023). Another recent study reported that FTO-induced upregulation of flotillin-2 contributed to cancer aggressivness in diffuse large B-cell lymphoma by activating PI3K/Akt/mTOR pathway (Zhang et al., 2024). Thus, the role of FTO in regulating the mTOR pathway is ambiguous, as it can lead to both activation and inactivation, resulting in negative and positive outcomes. FTO demethylates also the most important transcription factor and extensive nuclear oncogene MYC (MYC proto-oncogene, also named c-Myc). It has been reported that FTO can reduce the methylation of MYC in gastric cancer cells and stabilize its expression, ultimately resulting in augmented proliferation, migration and invasion of gastric cancer cells in vitro (Yang Z. et al., 2021). However, another study showed that MYC activated in Epstein-Barr virus-associated gastric cancer elevated FTO expression by binding to the FTO promoter. The increase in FTO levels was then associated with depressed cell metastasis, aggressiveness, and overall better clinical outcomes (Xu et al., 2023). Evidently, FTO’s role in gastric cancer can be both positive and negative, depending on the specific molecular and pathological context. This duality underscores the importance of context in understanding the function of molecular players in cancer biology.
Additionally, FTO has been implicated in resistance to chemo-radiotherapy. In cervical squamous cell carcinoma, FTO upregulates β-catenin by reducing m6A modification, leading to enhanced resistance to chemo-radiotherapy (Zhou et al., 2018). Similarly, in neuroblastoma, FTO has been shown to influence sensitivity to chemotherapeutic drugs, enhancing the response to paclitaxel while reducing sensitivity to etoposide, indicating a drug-specific role in chemotherapy resistance (Lin et al., 2024). This highlights the critical role of the FTO in modulating not just tumor growth but also drug resistance, making it a potential target for improving cancer therapy outcomes.
In conclusion, the role of FTO in cancer is multifaceted, influencing not only tumor growth and progression through metabolic reprogramming and m6A RNA modification but also impacting treatment outcomes by contributing to resistance mechanisms such as chemo-radiotherapy. Its involvement in key pathways like PI3K/Akt/mTOR and its regulation of oncogenes such as MYC and β-catenin further emphasize the importance of context when evaluating FTO’s function in various cancer types. As research continues to uncover the complexities of FTO’s role, it may offer novel therapeutic opportunities to target cancer growth and overcome treatment resistance.
7 FTO as a pharmacological target
Various inhibitors targeting FTO demethylase with a potential to increase m6A and m6Am methylation are currently available.
Rhein, an anthraquinone compound derived from the rhubarb plant (Rheum palmatum), was recognized as the first cell-active FTO inhibitor in 2012 (Chen et al., 2012). However, rhein is not a specific inhibitor as it acts also on other molecular targets (Henamayee et al., 2020), e.g., histone deacetylases (Barbosa et al., 2020). This lack of specificity exemplifies a central challenge in FTO inhibitor development: achieving selectivity to avoid unwanted effects on other enzymes and pathways.
Meclofenamic acid (MA), a well-recognized drug for its anti-inflammatory properties via cyclooxygenase inhibition, has been also described to inhibit the FTO enzyme (Huang et al., 2015). Entacapone, a reversible catechol-O-methyltransferase inhibitor used in the treatment of Parkinson’s disease, has been shown to bind FTO and inhibit its activity as well (Peng et al., 2019). While these compounds have shown efficacy in inhibiting FTO, their primary pharmacological targets lie elsewhere, highlighting the difficulty of repurposing drugs with established indications.
Besides these non-specific drugs, the compound MO-I-500 was introduced as a specific inhibitor of FTO (Zheng et al., 2014). Fluorescein and its derivatives were presented as bifunctional molecules for either inhibiting or labeling FTO (Wang et al., 2015). Additionally, selective inhibition of FTO was also achieved with other small molecule inhibitors, including FB23, FB23-2, CS1, CS2, Dac51, LHD, FTO-02, FTO-04, N-phenyl-1H-indol-2-amine, MU06, radicicol, CHTB, N-CDPCB, nafamostat mesilate, NOG, PDCA, or saikosaponin D (Yu et al., 2021; Toh et al., 2015; He et al., 2015; Svensen and Jaffrey, 2016; Huang et al., 2019; Su et al., 2020; Huff et al., 2021; Qin et al., 2022; Liu et al., 2021; Padariya and Kalathiya, 2016; Wang et al., 2018; Qiao et al., 2016; Han et al., 2019; Aik et al., 2013; Sun et al., 2021; Qiu et al., 2023). Each of these molecules showcases different approaches to enhancing selectivity through structural modifications, though issues such as off-target binding and stability have limited their progression beyond experimental stages. Developing compounds that specifically target FTO in relevant disease tissues without systemic side effects remains a critical goal for therapeutic applications.
Recent advances in structural biology have allowed for the design of more selective FTO inhibitors based on its catalytic domain, an approach that has improved specificity and facilitated the development of promising drug candidates. Challenges like ensuring high specificity without off-target effects, achieving effective tissue targeting, and managing toxicity–as well as optimizing bioavailability, half-life, and tissue localization–remain critical hurdles for the clinical translation of these compounds. Consequently, FTO inhibitors are mainly used in experimental settings and have not advanced to clinical application, primarily due to these specificity, pharmacokinetic, and localization limitations (Huff et al., 2021). Notably, STC-15, an inhibitor of the m6A writer METTL3, has recently become the first RNA-modifying enzyme inhibitor to enter clinical trials (NCT05584111) (Medicine, 2024). This milestone underscores the growing potential of RNA-modifying enzyme inhibitors, suggesting that the clinical advancement of FTO inhibitors may soon follow.
In summary, while the development of FTO inhibitors holds promise, the future identification of clinically effective and highly selective, tissue-targeted FTO-targeting agents is critical. Success in this area will be essential for creating novel treatments for various diseases, requiring close integration of structural biology insights, tissue-specific targeting strategies, and careful optimization to ensure drug-like properties amenable to clinical use.
8 Conclusion and perspectives
This review thoroughly discusses the critical role of the FTO protein in both health and disease, with a particular focus on its involvement in obesity and related non-communicable diseases such as diabetes, cardiovascular disease, and cancer. FTO, a key enzyme in the regulation of RNA modifications, influences a variety of physiological processes including RNA splicing, export, decay, and translation. Its dysregulation is linked to obesity through mechanisms affecting energy balance, appetite, and adipogenesis.
The relationship between FTO and various diseases is complex. In obesity, FTO is associated with increased food intake and reduced satiety, contributing to higher BMI. It also plays a significant role in adipogenesis and the regulation of thermogenesis, further emphasizing its importance in metabolic disorders.
In diabetes, FTO influences insulin secretion and sensitivity, with genetic variants linked to an increased risk of T2DM. Additionally, FTO’s role in diabetic complications, such as nephropathy and retinopathy, suggests it could serve as a potential biomarker or therapeutic target.
FTO’s involvement in CVDs is multifaceted, with evidence suggesting both protective and detrimental effects depending on the context. It is implicated in cardiac hypertrophy, heart failure, and myocardial ischemia-reperfusion injury, among other conditions.
Finally, FTO’s role in cancer is discussed, particularly its impact on tumor metabolism and proliferation. While FTO is generally upregulated in various cancers, contributing to tumor progression, its effects can vary depending on the type and context of the cancer. The role of FTO in CVDs and cancer exemplifies its broad influence on shared mechanisms, including cell proliferation and metabolic regulation, highlighting its specificity in disease manifestation while underscoring its universal function across diverse cell types, from cardiovascular to tumor cells.
Overall, this review underscores the importance of FTO as a central player in the epitranscriptomic regulation of health and disease (Figure 3). However, several challenges remain before these research findings can be implemented in clinical settings. A major obstacle in biomedical science is translating discoveries from laboratory animals, such as mice and rats, to human physiology. Substantial metabolic differences between rodents and humans–such as a 6.4-fold higher metabolic rate and a 9.6-fold faster protein turnover in rats (Agoston, 2017) – suggest species-specific variations in FTO function, given its role as a key metabolic regulator. These interspecies differences in FTO biology remain insufficiently understood, highlighting the need for further investigation to ensure the relevance of laboratory findings for clinical applications. Given FTO’s broad impact on physiology and pathophysiology, it remains a promising target for therapeutic interventions across a range of conditions. However, additional research is essential to fully elucidate its complex mechanisms and to develop effective, selective inhibitors that are suitable for clinical use. It is crucial that such therapeutic strategies are designed with precision to ensure that targeting FTO in one context does not disrupt its regulatory functions in other tissues or processes, thus avoiding potential adverse effects, such as treating CVDs at the risk of promoting cancer or other pathologies.
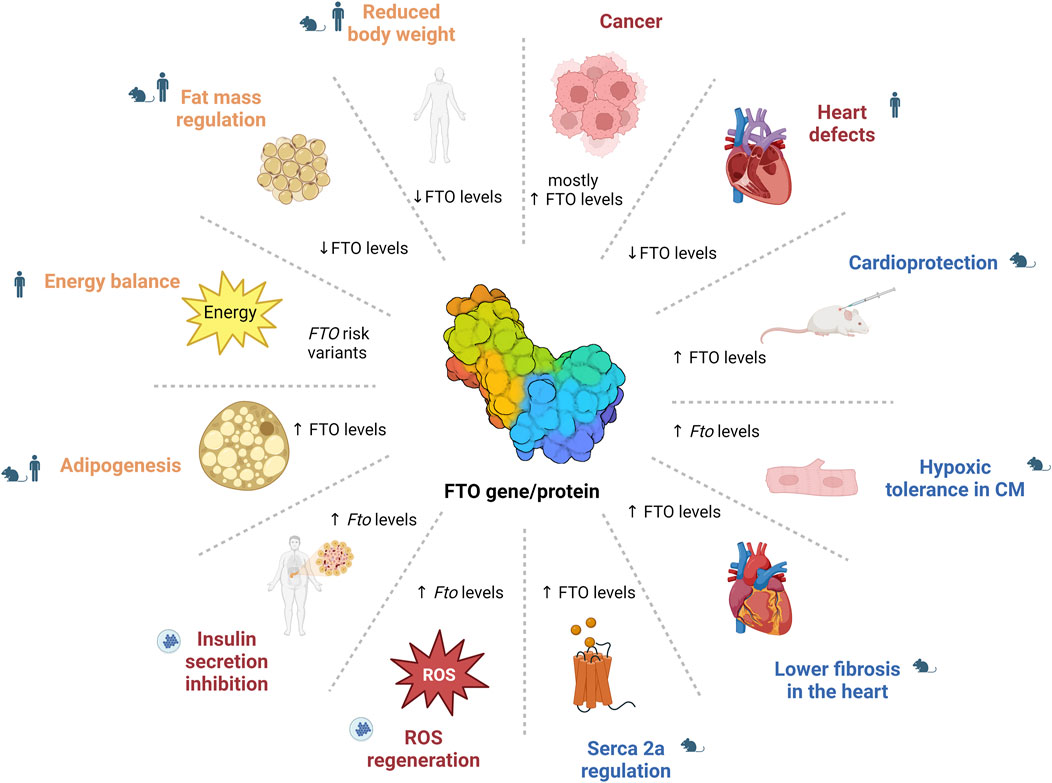
Figure 3. The role of FTO in health and disease. The image illustrates the diverse roles of FTO, showing that altered FTO levels affect various physiological and pathological processes, including energy balance and adipogenesis (e.g., through STAT3/ERK1/2 pathway or AMPK pathway regulation), diabetes (e.g., NF-κB pathway regulation), cardiovascular functions (e.g., SERCA2a regulation) and cancer (e.g., PI3K/Akt/mTOR pathway or MYC oncogene regulation), demonstrating its broad impact across the metabolic functions. CM – cardiomyocytes; FTO – fat mass and obesity-associated; ROS – reactive oxygen species.
Author contributions
BD: Visualization, Writing–original draft. SA: Visualization, Writing–original draft. HK: Writing–review and editing. HM: Writing–review and editing.
Funding
The author(s) declare that financial support was received for the research, authorship, and/or publication of this article. This work was supported by the Czech Science Foundation (grant number 24-10497S) to HM; the Ministry of Education, Youth and Sports of the CR (grant Inter-COST LUC24089), and the project National Institute for Research of Metabolic and Cardiovascular Diseases (Programme EXCELES, ID project No. LX22NPO5104) – Funded by the European Union – Next-Generation EU.
Acknowledgments
Figures were created with BioRender.com. ChatGPT 4.0, a large language model developed by OpenAI, was used for language corrections.
Conflict of interest
The authors declare that the research was conducted in the absence of any commercial or financial relationships that could be construed as a potential conflict of interest.
Publisher’s note
All claims expressed in this article are solely those of the authors and do not necessarily represent those of their affiliated organizations, or those of the publisher, the editors and the reviewers. Any product that may be evaluated in this article, or claim that may be made by its manufacturer, is not guaranteed or endorsed by the publisher.
References
Agoston, D. V. (2017). How to translate time? The temporal aspect of human and rodent biology. Front. Neurol. 8, 92. doi:10.3389/fneur.2017.00092
Ahmad, T., Chasman, D. I., Mora, S., Paré, G., Cook, N. R., Buring, J. E., et al. (2010). The fat-mass and obesity-associated (FTO) gene, physical activity, and risk of incident cardiovascular events in white women. Am. Heart J. 160 (6), 1163–1169. doi:10.1016/j.ahj.2010.08.002
Aik, W., Demetriades, M., Hamdan, M. K. K., Bagg, E. A. L., Yeoh, K. K., Lejeune, C., et al. (2013). Structural basis for inhibition of the fat mass and obesity associated protein (FTO). J. Med. Chem. 56 (9), 3680–3688. doi:10.1021/jm400193d
Amin, U. S. M., Rahman, T. A., Hasan, M., Tofail, T., Hasanat, M. A., Seraj, Z. I., et al. (2023). Type 2 diabetes linked FTO gene variant rs8050136 is significantly associated with gravidity in gestational diabetes in a sample of Bangladeshi women: meta-analysis and case-control study. PLoS One 18 (11), e0288318. doi:10.1371/journal.pone.0288318
Amine Ikhanjal, M., Ali Elouarid, M., Zouine, C., El Alami, H., Errafii, K., Ghazal, H., et al. (2023). FTO gene variants (rs9939609, rs8050136 and rs17817449) and type 2 diabetes mellitus risk: a Meta-Analysis. Gene 887, 147791. doi:10.1016/j.gene.2023.147791
An, Y., and Duan, H. (2022). The role of m6A RNA methylation in cancer metabolism. Mol. Cancer 21 (1), 14. doi:10.1186/s12943-022-01500-4
Ashcroft, F. M., and Rorsman, P. (2012). Diabetes mellitus and the β cell: the last ten years. Cell. 148 (6), 1160–1171. doi:10.1016/j.cell.2012.02.010
Ashraf, M. J., and Baweja, P. (2013). Obesity: the 'huge' problem in cardiovascular diseases. Mo Med. 110 (6), 499–504.
Avgerinos, K. I., Spyrou, N., Mantzoros, C. S., and Dalamaga, M. (2019). Obesity and cancer risk: emerging biological mechanisms and perspectives. Metabolism 92, 121–135. doi:10.1016/j.metabol.2018.11.001
Azhati, B., Reheman, A., Dilixiati, D., and Rexiati, M. (2023). FTO-stabilized miR-139-5p targets ZNF217 to suppress prostate cancer cell malignancies by inactivating the PI3K/Akt/mTOR signal pathway. Arch. Biochem. Biophys. 741, 109604. doi:10.1016/j.abb.2023.109604
Azzam, S. K., Alsafar, H., and Sajini, A. A. (2022). FTO m6A demethylase in obesity and cancer: implications and underlying molecular mechanisms. Int. J. Mol. Sci. 23 (7), 3800. doi:10.3390/ijms23073800
Babakhanian, M., Razavi, A., Rahimi Pordanjani, S., Hassanabadi, S., Mohammadi, G., and Fattah, A. (2022). High incidence of type 1 diabetes, type 2 diabetes and gestational diabetes in Central Iran: a six years results from Semnan health cohort. Ann. Med. Surg. (Lond) 82, 104749. doi:10.1016/j.amsu.2022.104749
Bakhashab, S., Filimban, N., Altall, R. M., Nassir, R., Qusti, S. Y., Alqahtani, M. H., et al. (2020). The effect sizes of PPARγ rs1801282, FTO rs9939609, and MC4R rs2229616 variants on type 2 diabetes mellitus risk among the western Saudi population: a cross-sectional prospective study. Genes. (Basel) 11 (1), 98. doi:10.3390/genes11010098
Barbosa, D. M., Fahlbusch, P., Herzfeld de Wiza, D., Jacob, S., Kettel, U., Al-Hasani, H., et al. (2020). Rhein, a novel Histone Deacetylase (HDAC) inhibitor with antifibrotic potency in human myocardial fibrosis. Sci. Rep. 10 (1), 4888. doi:10.1038/s41598-020-61886-3
Bazzi, M. D., Nasr, F. A., Alanazi, M. S., Alamri, A., Turjoman, A. A., Moustafa, A. S., et al. (2014). Association between FTO, MC4R, SLC30A8, and KCNQ1 gene variants and type 2 diabetes in Saudi population. Genet. Mol. Res. 13 (4), 10194–10203. doi:10.4238/2014.December.4.14
Benak, D., Benakova, S., Plecita-Hlavata, L., and Hlavackova, M. (2023b). The role of m6A and m6Am RNA modifications in the pathogenesis of diabetes mellitus. Front. Endocrinol. (Lausanne) 14, 1223583. doi:10.3389/fendo.2023.1223583
Benak, D., Holzerova, K., Hrdlicka, J., Kolar, F., Olsen, M., Karelson, M., et al. (2024). Epitranscriptomic regulation in fasting hearts: implications for cardiac health. RNA Biol. 21 (1), 1–14. doi:10.1080/15476286.2024.2307732
Benak, D., Kolar, F., Zhang, L., Devaux, Y., and Hlavackova, M. (2023a). RNA modification m(6)Am: the role in cardiac biology. Epigenetics 18 (1), 2218771. doi:10.1080/15592294.2023.2218771
Berulava, T., Buchholz, E., Elerdashvili, V., Pena, T., Islam, M. R., Lbik, D., et al. (2020). Changes in m6A RNA methylation contribute to heart failure progression by modulating translation. Eur. J. Heart Fail 22 (1), 54–66. doi:10.1002/ejhf.1672
Berulava, T., and Horsthemke, B. (2010). The obesity-associated SNPs in intron 1 of the FTO gene affect primary transcript levels. Eur. J. Hum. Genet. 18 (9), 1054–1056. doi:10.1038/ejhg.2010.71
Boissel, S., Reish, O., Proulx, K., Kawagoe-Takaki, H., Sedgwick, B., Yeo, G. S. H., et al. (2009). Loss-of-function mutation in the dioxygenase-encoding FTO gene causes severe growth retardation and multiple malformations. Am. J. Hum. Genet. 85 (1), 106–111. doi:10.1016/j.ajhg.2009.06.002
Bornaque, F., Delannoy, C. P., Courty, E., Rabhi, N., Carney, C., Rolland, L., et al. (2022). Glucose regulates m(6)A methylation of RNA in pancreatic islets. Cells 11 (2), 291. doi:10.3390/cells11020291
Brennan, P., McKay, J., Moore, L., Zaridze, D., Mukeria, A., Szeszenia-Dabrowska, N., et al. (2009). Obesity and cancer: mendelian randomization approach utilizing the FTO genotype. Int. J. Epidemiol. 38 (4), 971–975. doi:10.1093/ije/dyp162
Bressler, J., Kao, W. H. L., Pankow, J. S., and Boerwinkle, E. (2010). Risk of type 2 diabetes and obesity is differentially associated with variation in FTO in whites and African-Americans in the ARIC study. PLoS One 5 (5), e10521. doi:10.1371/journal.pone.0010521
Carnevali, L., Graiani, G., Rossi, S., Al Banchaabouchi, M., Macchi, E., Quaini, F., et al. (2014). Signs of cardiac autonomic imbalance and proarrhythmic remodeling in FTO deficient mice. PLoS One 9 (4), e95499. doi:10.1371/journal.pone.0095499
Cecil, J. E., Tavendale, R., Watt, P., Hetherington, M. M., and Palmer, C. N. A. (2008). An obesity-associated FTO gene variant and increased energy intake in children. N. Engl. J. Med. 359 (24), 2558–2566. doi:10.1056/NEJMoa0803839
Chaudhary, N., Alawadhi, F., Al-Serri, A., and Al-Temaimi, R. (2024). TCF7L2 and FTO polymorphisms are associated with type 2 diabetes mellitus risk in Kuwait. Med. Princ. Pract. 33, 157–163. doi:10.1159/000536229
Chauhan, G., Tabassum, R., Mahajan, A., Dwivedi, O. P., Mahendran, Y., Kaur, I., et al. (2011). Common variants of FTO and the risk of obesity and type 2 diabetes in Indians. J. Hum. Genet. 56 (10), 720–726. doi:10.1038/jhg.2011.87
Chen, B., Ye, F., Yu, L., Jia, G., Huang, X., Zhang, X., et al. (2012). Development of cell-active N6-methyladenosine RNA demethylase FTO inhibitor. J. Am. Chem. Soc. 134 (43), 17963–17971. doi:10.1021/ja3064149
Chen, X., Wang, Y., Wang, J. N., Zhang, Y. C., Zhang, Y. R., Sun, R. X., et al. (2024). Lactylation-driven FTO targets CDK2 to aggravate microvascular anomalies in diabetic retinopathy. EMBO Mol. Med. 16 (2), 294–318. doi:10.1038/s44321-024-00025-1
Church, C., Lee, S., Bagg, E. A. L., McTaggart, J. S., Deacon, R., Gerken, T., et al. (2009). A mouse model for the metabolic effects of the human fat mass and obesity associated FTO gene. PLoS Genet. 5 (8), e1000599. doi:10.1371/journal.pgen.1000599
Church, C., Moir, L., McMurray, F., Girard, C., Banks, G. T., Teboul, L., et al. (2010). Overexpression of Fto leads to increased food intake and results in obesity. Nat. Genet. 42 (12), 1086–1092. doi:10.1038/ng.713
Claussnitzer, M., Dankel, S. N., Kim, K. H., Quon, G., Meuleman, W., Haugen, C., et al. (2015). FTO obesity variant circuitry and adipocyte browning in humans. N. Engl. J. Med. 373 (10), 895–907. doi:10.1056/NEJMoa1502214
Cui, Y., Wang, P., Li, M., Wang, Y., Tang, X., Cui, J., et al. (2023). Cinnamic acid mitigates left ventricular hypertrophy and heart failure in part through modulating FTO-dependent N(6)-methyladenosine RNA modification in cardiomyocytes. Biomed. Pharmacother. 165, 115168. doi:10.1016/j.biopha.2023.115168
De Jesus, D. F., Zhang, Z., Kahraman, S., Brown, N. K., Chen, M., Hu, J., et al. (2019). m6A mRNA methylation regulates human β-cell biology in physiological states and in type 2 diabetes. Nat. Metab. 1 (8), 765–774. doi:10.1038/s42255-019-0089-9
Delahanty, R. J., Beeghly-Fadiel, A., Xiang, Y. B., Long, J., Cai, Q., Wen, W., et al. (2011). Association of obesity-related genetic variants with endometrial cancer risk: a report from the Shanghai Endometrial Cancer Genetics Study. Am. J. Epidemiol. 174 (10), 1115–1126. doi:10.1093/aje/kwr233
Deng, W., Jin, Q., and Li, L. (2021). Protective mechanism of demethylase fat mass and obesity-associated protein in energy metabolism disorder of hypoxia-reoxygenation-induced cardiomyocytes. Exp. Physiol. 106 (12), 2423–2433. doi:10.1113/EP089901
Desrosiers, R., Friderici, K., and Rottman, F. (1974). Identification of methylated nucleosides in messenger RNA from Novikoff hepatoma cells. Proc. Natl. Acad. Sci. U. S. A. 71 (10), 3971–3975. doi:10.1073/pnas.71.10.3971
Dhillo, W. S. (2007). Appetite regulation: an overview. Thyroid 17 (5), 433–445. doi:10.1089/thy.2007.0018
Dieterich, C., and Völkers, M. (2021). “Chapter 6 - RNA modifications in cardiovascular disease—an experimental and computational perspective,” in Epigenetics in cardiovascular disease. Editors Y. Devaux, and E. L. Robinson (Academic Press), 113–125.
Doney, A. S., Dannfald, J., Kimber, C. H., Donnelly, L. A., Pearson, E., Morris, A. D., et al. (2009). The FTO gene is associated with an atherogenic lipid profile and myocardial infarction in patients with type 2 diabetes: a Genetics of Diabetes Audit and Research Study in Tayside Scotland (Go-DARTS) study. Circ. Cardiovasc Genet. 2 (3), 255–259. doi:10.1161/CIRCGENETICS.108.822320
Dubey, P. K., Patil, M., Singh, S., Dubey, S., Ahuja, P., Verma, S. K., et al. (2022). Increased m6A-RNA methylation and FTO suppression is associated with myocardial inflammation and dysfunction during endotoxemia in mice. Mol. Cell. Biochem. 477 (1), 129–141. doi:10.1007/s11010-021-04267-2
Fan, H. Q., He, W., Xu, K. F., Wang, Z. X., Xu, X. Y., and Chen, H. (2015). FTO inhibits insulin secretion and promotes NF-κB activation through positively regulating ROS production in pancreatic β cells. PLoS One 10 (5), e0127705. doi:10.1371/journal.pone.0127705
Fischer, J., Koch, L., Emmerling, C., Vierkotten, J., Peters, T., Brüning, J. C., et al. (2009). Inactivation of the Fto gene protects from obesity. Nature 458 (7240), 894–898. doi:10.1038/nature07848
Frayling, T. M., Timpson, N. J., Weedon, M. N., Zeggini, E., Freathy, R. M., Lindgren, C. M., et al. (2007). A common variant in the FTO gene is associated with body mass index and predisposes to childhood and adult obesity. Science 316 (5826), 889–894. doi:10.1126/science.1141634
Galicia-Garcia, U., Benito-Vicente, A., Jebari, S., Larrea-Sebal, A., Siddiqi, H., Uribe, K. B., et al. (2020). Pathophysiology of type 2 diabetes mellitus. Int. J. Mol. Sci. 21 (17), 6275. doi:10.3390/ijms21176275
Gan, X. T., Zhao, G., Huang, C. X., Rowe, A. C., Purdham, D. M., and Karmazyn, M. (2013). Identification of fat mass and obesity associated (FTO) protein expression in cardiomyocytes: regulation by leptin and its contribution to leptin-induced hypertrophy. PLoS One 8 (9), e74235. doi:10.1371/journal.pone.0074235
Genis-Mendoza, A. D., Martínez-Magaña, J. J., Ruiz-Ramos, D., Gonzalez-Covarrubias, V., Tovilla-Zarate, C. A., Narvaez, M. L. L., et al. (2020). Interaction of FTO rs9939609 and the native American-origin ABCA1 p.Arg230Cys with circulating leptin levels in Mexican adolescents diagnosed with eating disorders: preliminary results. Psychiatry Res. 291, 113270. doi:10.1016/j.psychres.2020.113270
Gerken, T., Girard, C. A., Tung, Y. C. L., Webby, C. J., Saudek, V., Hewitson, K. S., et al. (2007). The obesity-associated FTO gene encodes a 2-oxoglutarate-dependent nucleic acid demethylase. Science 318 (5855), 1469–1472. doi:10.1126/science.1151710
Ghafarian-Alipour, F., Ziaee, S., Ashoori, M. R., Zakeri, M. S., Boroumand, M. A., Aghamohammadzadeh, N., et al. (2018). Association between FTO gene polymorphisms and type 2 diabetes mellitus, serum levels of apelin and androgen hormones among Iranian obese women. Gene 641, 361–366. doi:10.1016/j.gene.2017.10.082
Gholamalizadeh, M., Jonoush, M., Mobarakeh, K. A., Amjadi, A., Alami, F., Valisoltani, N., et al. (2023). The effects of FTO gene rs9939609 polymorphism on the association between colorectal cancer and dietary intake. Front. Nutr. 10, 1215559. doi:10.3389/fnut.2023.1215559
Gholami, M. (2024). FTO is a major genetic link between breast cancer, obesity, and diabetes. Breast Cancer Res. Treat. 204 (1), 159–169. doi:10.1007/s10549-023-07188-4
Goyal, R., and Jialal, I. (2023). “Diabetes mellitus type 2, in StatPearls,” in StatPearls publishing copyright © 2023. Treasure Island (FL): StatPearls Publishing LLC.
Han, X., Wang, N., Li, J., Wang, Y., Wang, R., and Chang, J. (2019). Identification of nafamostat mesilate as an inhibitor of the fat mass and obesity-associated protein (FTO) demethylase activity. Chem. Biol. Interact. 297, 80–84. doi:10.1016/j.cbi.2018.10.023
Han, Z., Niu, T., Chang, J., Lei, X., Zhao, M., Wang, Q., et al. (2010). Crystal structure of the FTO protein reveals basis for its substrate specificity. Nature 464 (7292), 1205–1209. doi:10.1038/nature08921
Han, Z., Wang, X., Xu, Z., Cao, Y., Gong, R., Yu, Y., et al. (2021). ALKBH5 regulates cardiomyocyte proliferation and heart regeneration by demethylating the mRNA of YTHDF1. Theranostics 11 (6), 3000–3016. doi:10.7150/thno.47354
Haupt, A., Thamer, C., Staiger, H., Tschritter, O., Kirchhoff, K., Machicao, F., et al. (2009). Variation in the FTO gene influences food intake but not energy expenditure. Exp. Clin. Endocrinol. Diabetes 117 (4), 194–197. doi:10.1055/s-0028-1087176
He, D., Fu, M., Miao, S., Hotta, K., Chandak, G. R., and Xi, B. (2014). FTO gene variant and risk of hypertension: a meta-analysis of 57,464 hypertensive cases and 41,256 controls. Metabolism 63 (5), 633–639. doi:10.1016/j.metabol.2014.02.008
He, W., Zhou, B., Liu, W., Zhang, M., Shen, Z., Han, Z., et al. (2015). Identification of A Novel small-molecule binding site of the fat mass and obesity associated protein (FTO). J. Med. Chem. 58 (18), 7341–7348. doi:10.1021/acs.jmedchem.5b00702
Henamayee, S., Banik, K., Sailo, B. L., Shabnam, B., Harsha, C., Srilakshmi, S., et al. (2020). Therapeutic emergence of rhein as a potential anticancer drug: a review of its molecular targets and anticancer properties. Molecules 25 (10), 2278. doi:10.3390/molecules25102278
Hernández-Caballero, M. E., and Sierra-Ramírez, J. A. (2015). Single nucleotide polymorphisms of the FTO gene and cancer risk: an overview. Mol. Biol. Rep. 42 (3), 699–704. doi:10.1007/s11033-014-3817-y
Hinger, S. A., Wei, J., Dorn, L. E., Whitson, B. A., Janssen, P. M. L., He, C., et al. (2021). Remodeling of the m(6)A landscape in the heart reveals few conserved post-transcriptional events underlying cardiomyocyte hypertrophy. J. Mol. Cell. Cardiol. 151, 46–55. doi:10.1016/j.yjmcc.2020.11.002
Hinney, A., Nguyen, T. T., Scherag, A., Friedel, S., Brönner, G., Müller, T. D., et al. (2007). Genome wide association (GWA) study for early onset extreme obesity supports the role of fat mass and obesity associated gene (FTO) variants. PLoS One 2 (12), e1361. doi:10.1371/journal.pone.0001361
Hlavackova, M. (2018). Fat mass and obesity-associated protein in chronically hypoxic myocardium. High Alt. Med. and Biol. 19 (4), A–443. doi:10.1089/ham.2018.29015.abstracts
Hsiao, Y. T., Shen, F. C., Weng, S. W., Wang, P. W., Chen, Y. J., and Lee, J. J. (2021). Multiple single nucleotide polymorphism testing improves the prediction of diabetic retinopathy risk with type 2 diabetes mellitus. J. Pers. Med. 11 (8), 689. doi:10.3390/jpm11080689
Hu, F., Yan, H. J., Gao, C. X., Sun, W. W., and Long, Y. S. (2023). Inhibition of hypothalamic FTO activates STAT3 signal through ERK1/2 associated with reductions in food intake and body weight. Neuroendocrinology 113 (1), 80–91. doi:10.1159/000526752
Huang, Y., Su, R., Sheng, Y., Dong, L., Dong, Z., Xu, H., et al. (2019). Small-molecule targeting of oncogenic FTO demethylase in acute myeloid leukemia. Cancer Cell. 35 (4), 677–691. doi:10.1016/j.ccell.2019.03.006
Huang, Y., Yan, J., Li, Q., Li, J., Gong, S., Zhou, H., et al. (2015). Meclofenamic acid selectively inhibits FTO demethylation of m6A over ALKBH5. Nucleic Acids Res. 43 (1), 373–384. doi:10.1093/nar/gku1276
Hubacek, J. A., Dlouha, D., Klementova, M., Lanska, V., Neskudla, T., and Pelikanova, T. (2018a). The FTO variant is associated with chronic complications of diabetes mellitus in Czech population. Gene 642, 220–224. doi:10.1016/j.gene.2017.11.040
Hubacek, J. A., Dlouha, L., Adamkova, V., Dlouha, D., Pacal, L., Kankova, K., et al. (2023). Genetic risk score is associated with T2DM and diabetes complications risks. Gene 849, 146921. doi:10.1016/j.gene.2022.146921
Hubacek, J. A., Stanek, V., Gebauerová, M., Pilipcincová, A., Dlouhá, D., Poledne, R., et al. (2010). A FTO variant and risk of acute coronary syndrome. Clin. Chim. Acta 411 (15-16), 1069–1072. doi:10.1016/j.cca.2010.03.037
Hubacek, J. A., Vrablik, M., Dlouha, D., Stanek, V., Gebauerova, M., Adamkova, V., et al. (2016). Gene variants at FTO, 9p21, and 2q36.3 are age-independently associated with myocardial infarction in Czech men. Clin. Chim. Acta 454, 119–123. doi:10.1016/j.cca.2016.01.005
Hubacek, J. A., Vymetalova, J., Lanska, V., and Dlouha, D. (2018b). The fat mass and obesity related gene polymorphism influences the risk of rejection in heart transplant patients. Clin. Transpl. 32 (12), e13443. doi:10.1111/ctr.13443
Huff, S., Tiwari, S. K., Gonzalez, G. M., Wang, Y., and Rana, T. M. (2021). m(6)A-RNA demethylase FTO inhibitors impair self-renewal in glioblastoma stem cells. ACS Chem. Biol. 16 (2), 324–333. doi:10.1021/acschembio.0c00841
Iles, M. M., Law, M. H., Stacey, S. N., Han, J., Fang, S., Pfeiffer, R., et al. (2013). A variant in FTO shows association with melanoma risk not due to BMI. Nat. Genet. 45 (4), 428–432e1. 432e1. doi:10.1038/ng.2571
Jia, G., Fu, Y., Zhao, X., Dai, Q., Zheng, G., Yang, Y., et al. (2011). N6-methyladenosine in nuclear RNA is a major substrate of the obesity-associated FTO. Nat. Chem. Biol. 7 (12), 885–887. doi:10.1038/nchembio.687
Jia, G., Yang, C. G., Yang, S., Jian, X., Yi, C., Zhou, Z., et al. (2008). Oxidative demethylation of 3-methylthymine and 3-methyluracil in single-stranded DNA and RNA by mouse and human FTO. FEBS Lett. 582 (23-24), 3313–3319. doi:10.1016/j.febslet.2008.08.019
Ju, W., Liu, K., Ouyang, S., Liu, Z., He, F., and Wu, J. (2021). Changes in N6-methyladenosine modification modulate diabetic cardiomyopathy by reducing myocardial fibrosis and myocyte hypertrophy. Front. Cell. Dev. Biol. 9, 702579. doi:10.3389/fcell.2021.702579
Kaklamani, V., Yi, N., Sadim, M., Siziopikou, K., Zhang, K., Xu, Y., et al. (2011). The role of the fat mass and obesity associated gene (FTO) in breast cancer risk. BMC Med. Genet. 12, 52. doi:10.1186/1471-2350-12-52
Karra, E., O'Daly, O. G., Choudhury, A. I., Yousseif, A., Millership, S., Neary, M. T., et al. (2013). A link between FTO, ghrelin, and impaired brain food-cue responsivity. J. Clin. Investig. 123 (8), 3539–3551. doi:10.1172/JCI44403
Ke, W. L., Huang, Z. W., Peng, C. L., and Ke, Y. P. (2022). m(6)A demethylase FTO regulates the apoptosis and inflammation of cardiomyocytes via YAP1 in ischemia-reperfusion injury. Bioengineered 13 (3), 5443–5452. doi:10.1080/21655979.2022.2030572
Khatiwada, B., Nguyen, T. T., Purslow, J. A., and Venditti, V. (2022). Solution structure ensemble of human obesity-associated protein FTO reveals druggable surface pockets at the interface between the N- and C-terminal domain. J. Biol. Chem. 298 (5), 101907. doi:10.1016/j.jbc.2022.101907
Kirkpatrick, C. L., Marchetti, P., Purrello, F., Piro, S., Bugliani, M., Bosco, D., et al. (2010). Type 2 diabetes susceptibility gene expression in normal or diabetic sorted human alpha and beta cells: correlations with age or BMI of islet donors. PLoS One 5 (6), e11053. doi:10.1371/journal.pone.0011053
Klein, S., Gastaldelli, A., Yki-Järvinen, H., and Scherer, P. E. (2022). Why does obesity cause diabetes? Cell. Metab. 34 (1), 11–20. doi:10.1016/j.cmet.2021.12.012
Krejčí, J., Arcidiacono, O. A., Čegan, R., Radaszkiewicz, K., Pacherník, J., Pirk, J., et al. (2023). Cell differentiation and aging lead to up-regulation of FTO, while the ALKBH5 protein level was stable during aging but up-regulated during in vitro-Induced cardiomyogenesis. Physiol. Res. 72 (4), 425–444. doi:10.33549/physiolres.935078
Lauby-Secretan, B., Scoccianti, C., Loomis, D., Grosse, Y., Bianchini, F., Straif, K., et al. (2016). Body fatness and cancer--viewpoint of the IARC working group. N. Engl. J. Med. 375 (8), 794–798. doi:10.1056/NEJMsr1606602
Li, H., Ren, Y., Mao, K., Hua, F., Yang, Y., Wei, N., et al. (2018). FTO is involved in Alzheimer's disease by targeting TSC1-mTOR-Tau signaling. Biochem. Biophys. Res. Commun. 498 (1), 234–239. doi:10.1016/j.bbrc.2018.02.201
Li, W., Xing, C., Bao, L., Han, S., Luo, T., Wang, Z., et al. (2022a). Comprehensive analysis of RNA m6A methylation in pressure overload-induced cardiac hypertrophy. BMC Genomics 23 (1), 576. doi:10.1186/s12864-022-08833-w
Li, Y., Su, R., Deng, X., Chen, Y., and Chen, J. (2022b). FTO in cancer: functions, molecular mechanisms, and therapeutic implications. Trends Cancer 8 (7), 598–614. doi:10.1016/j.trecan.2022.02.010
Li, Z., Weng, H., Su, R., Weng, X., Zuo, Z., Li, C., et al. (2017). FTO plays an oncogenic role in acute myeloid leukemia as a N(6)-methyladenosine RNA demethylase. Cancer Cell. 31 (1), 127–141. doi:10.1016/j.ccell.2016.11.017
Lin, M., Hua, Z., and Li, Z. (2024). FTO diversely influences sensitivity of neuroblastoma cells to various chemotherapeutic drugs. Front. Pharmacol. 15, 1384141. doi:10.3389/fphar.2024.1384141
Liu, C., Mou, S., and Pan, C. (2013). The FTO gene rs9939609 polymorphism predicts risk of cardiovascular disease: a systematic review and meta-analysis. PLoS One 8 (8), e71901. doi:10.1371/journal.pone.0071901
Liu, K., Ju, W., Ouyang, S., Liu, Z., He, F., Hao, J., et al. (2022). Exercise training ameliorates myocardial phenotypes in heart failure with preserved ejection fraction by changing N6-methyladenosine modification in mice model. Front. Cell. Dev. Biol. 10, 954769. doi:10.3389/fcell.2022.954769
Liu, S., Song, S., Wang, S., Cai, T., Qin, L., Wang, X., et al. (2024). Hypothalamic FTO promotes high-fat diet-induced leptin resistance in mice through increasing CX3CL1 expression. J. Nutr. Biochem. 123, 109512. doi:10.1016/j.jnutbio.2023.109512
Liu, X. H., Liu, Z., Ren, Z. H., Chen, H. X., Zhang, Y., Zhang, Z., et al. (2023). Co-effects of m6A and chromatin accessibility dynamics in the regulation of cardiomyocyte differentiation. Epigenetics Chromatin 16 (1), 32. doi:10.1186/s13072-023-00506-6
Liu, Y., Liang, G., Xu, H., Dong, W., Dong, Z., Qiu, Z., et al. (2021). Tumors exploit FTO-mediated regulation of glycolytic metabolism to evade immune surveillance. Cell. Metab. 33 (6), 1221–1233.e11. doi:10.1016/j.cmet.2021.04.001
Lurie, G., Gaudet, M. M., Spurdle, A. B., Carney, M. E., Wilkens, L. R., Yang, H. P., et al. (2011). The obesity-associated polymorphisms FTO rs9939609 and MC4R rs17782313 and endometrial cancer risk in non-Hispanic white women. PLoS One 6 (2), e16756. doi:10.1371/journal.pone.0016756
Ma, Y., Liu, X., Bi, Y., Wang, T., Chen, C., Wang, Y., et al. (2022). Alteration of N(6)-methyladenosine mRNA methylation in a human stem cell-derived cardiomyocyte model of tyrosine kinase inhibitor-induced cardiotoxicity. Front. Cardiovasc Med. 9, 849175. doi:10.3389/fcvm.2022.849175
Magno, F., Guaraná, H. C., Fonseca, A. C. P., Cabello, G. M. K., Carneiro, J. R. I., Pedrosa, A. P., et al. (2018). Influence of FTO rs9939609 polymorphism on appetite, ghrelin, leptin, IL6, TNFα levels, and food intake of women with morbid obesity. Diabetes Metab. Syndr. Obes. 11, 199–207. doi:10.2147/DMSO.S154978
Malone, J. I., and Hansen, B. C. (2019). Does obesity cause type 2 diabetes mellitus (T2DM)? Or is it the opposite? Pediatr. Diabetes 20 (1), 5–9. doi:10.1111/pedi.12787
Masoud Abd El Gayed, E., Kamal El Din Zewain, S., Ragheb, A., and ElNaidany, S. S. (2021). Fat mass and obesity-associated gene expression and disease severity in type 2 diabetes mellitus. Steroids 174, 108897. doi:10.1016/j.steroids.2021.108897
Mathiyalagan, P., Adamiak, M., Mayourian, J., Sassi, Y., Liang, Y., Agarwal, N., et al. (2019). FTO-dependent N(6)-methyladenosine regulates cardiac function during remodeling and repair. Circulation 139 (4), 518–532. doi:10.1161/CIRCULATIONAHA.118.033794
Mauer, J., and Jaffrey, S. R. (2018). FTO, m(6) A(m), and the hypothesis of reversible epitranscriptomic mRNA modifications. FEBS Lett. 592 (12), 2012–2022. doi:10.1002/1873-3468.13092
Mauer, J., Luo, X., Blanjoie, A., Jiao, X., Grozhik, A. V., Patil, D. P., et al. (2017). Reversible methylation of m(6)A(m) in the 5' cap controls mRNA stability. Nature 541 (7637), 371–375. doi:10.1038/nature21022
McMurray, F., Church, C. D., Larder, R., Nicholson, G., Wells, S., Teboul, L., et al. (2013). Adult onset global loss of the fto gene alters body composition and metabolism in the mouse. PLoS Genet. 9 (1), e1003166. doi:10.1371/journal.pgen.1003166
Medicine, N. L. o. (2024). Oral Administration of STC-15 in subjects with advanced malignancies (NCT05584111). 2022-2025 18. 11. Available at: https://clinicaltrials.gov/study/NCT05584111?term=NCT05584111&rank=1.
Mehrdad, M., Doaei, S., Gholamalizadeh, M., Fardaei, M., Fararouei, M., and Eftekhari, M. H. (2020). Association of FTO rs9939609 polymorphism with serum leptin, insulin, adiponectin, and lipid profile in overweight adults. Adipocyte 9 (1), 51–56. doi:10.1080/21623945.2020.1722550
Meng, Y., Xi, T., Fan, J., Yang, Q., Ouyang, J., and Yang, J. (2024). The inhibition of FTO attenuates the antifibrotic effect of leonurine in rat cardiac fibroblasts. Biochem. Biophys. Res. Commun. 693, 149375. doi:10.1016/j.bbrc.2023.149375
Merkestein, M., Laber, S., McMurray, F., Andrew, D., Sachse, G., Sanderson, J., et al. (2015). FTO influences adipogenesis by regulating mitotic clonal expansion. Nat. Commun. 6, 6792. doi:10.1038/ncomms7792
Mirabilii, S., Ricciardi, M. R., and Tafuri, A. (2020). mTOR regulation of metabolism in hematologic malignancies. Cells 9 (2), 404. doi:10.3390/cells9020404
Montesanto, A., Bonfigli, A. R., Crocco, P., Garagnani, P., De Luca, M., Boemi, M., et al. (2018). Genes associated with Type 2 Diabetes and vascular complications. Aging (Albany NY) 10 (2), 178–196. doi:10.18632/aging.101375
Mosaad, Y. M., Morzak, M., Abd El Aziz El Chennawi, F., Elsharkawy, A. A., and Abdelsalam, M. (2024). Evaluation of the role of FTO (rs9939609) and MC4R (rs17782313) gene polymorphisms in type 1 diabetes and their relation to obesity. J. Pediatr. Endocrinol. Metab. 37 (2), 110–122. doi:10.1515/jpem-2023-0372
Nabeel-Shah, S., Pu, S., Burke, G. L., Ahmed, N., Braunschweig, U., Farhangmehr, S., et al. (2024). Recruitment of the m(6)A/m6Am demethylase FTO to target RNAs by the telomeric zinc finger protein ZBTB48. Genome Biol. 25 (1), 246. doi:10.1186/s13059-024-03392-7
Nasser, F. A., Algenabi, A. A., Hadi, N. R., Hussein, M. K., Fatima, G., and Al-Aubaidy, H. A. (2019). The association of the common fat mass and obesity associated gene polymorphisms with type 2 diabetes in obese Iraqi population. Diabetes Metab. Syndr. 13 (4), 2451–2455. doi:10.1016/j.dsx.2019.06.024
Onalan, E., Yakar, B., Karakulak, K., Kaymaz, T., and Donder, E. (2022). m(6)A RNA, FTO, ALKBH5 expression in type 2 diabetic and obesity patients. J. Coll. Physicians Surg. Pak 32 (9), 1143–1148. doi:10.29271/jcpsp.2022.09.1143
Padariya, M., and Kalathiya, U. (2016). Structure-based design and evaluation of novel N-phenyl-1H-indol-2-amine derivatives for fat mass and obesity-associated (FTO) protein inhibition. Comput. Biol. Chem. 64, 414–425. doi:10.1016/j.compbiolchem.2016.09.008
Pati, S., Irfan, W., Jameel, A., Ahmed, S., and Shahid, R. K. (2023). Obesity and cancer: a current overview of epidemiology, pathogenesis, outcomes, and management. Cancers (Basel) 15 (2), 485. doi:10.3390/cancers15020485
Peng, S., Xiao, W., Ju, D., Sun, B., Hou, N., Liu, Q., et al. (2019). Identification of entacapone as a chemical inhibitor of FTO mediating metabolic regulation through FOXO1. Sci. Transl. Med. 11 (488), eaau7116. doi:10.1126/scitranslmed.aau7116
Peters, T., Ausmeier, K., and Rüther, U. (1999). Cloning of Fatso (Fto), a novel gene deleted by the Fused toes (Ft) mouse mutation. Mamm. Genome 10 (10), 983–986. doi:10.1007/s003359901144
Pierce, B. L., Austin, M. A., and Ahsan, H. (2011). Association study of type 2 diabetes genetic susceptibility variants and risk of pancreatic cancer: an analysis of PanScan-I data. Cancer Causes Control 22 (6), 877–883. doi:10.1007/s10552-011-9760-5
Piwonska, A. M., Cicha-Mikolajczyk, A., Sobczyk-Kopciol, A., Piwonski, J., Drygas, W., Kwasniewska, M., et al. (2022). Independent association of FTO rs9939609 polymorphism with overweight and obesity in Polish adults. Results from the representative population-based WOBASZ study. J. Physiol. Pharmacol. 73 (3). doi:10.26402/jpp.2022.3.07
Qiao, Y., Zhou, B., Zhang, M., Liu, W., Han, Z., Song, C., et al. (2016). A novel inhibitor of the obesity-related protein FTO. Biochemistry 55 (10), 1516–1522. doi:10.1021/acs.biochem.6b00023
Qin, B., Bai, Q., Yan, D., Yin, F., Zhu, Z., Xia, C., et al. (2022). Discovery of novel mRNA demethylase FTO inhibitors against esophageal cancer. J. Enzyme Inhib. Med. Chem. 37 (1), 1995–2003. doi:10.1080/14756366.2022.2098954
Qiu, L., Jing, Q., Li, Y., and Han, J. (2023). RNA modification: mechanisms and therapeutic targets. Mol. Biomed. 4 (1), 25. doi:10.1186/s43556-023-00139-x
Rafaqat, S., Sharif, S., Naz, S., Patoulias, D., and Klisic, A. (2024). Contributing role of metabolic genes APOE, FTO, and LPL in the development of atrial fibrillation: insights from a case-control study. Rev. Assoc. Med. Bras. 70 (8), e20240263. doi:10.1590/1806-9282.20240263
Relier, S., Ripoll, J., Guillorit, H., Amalric, A., Achour, C., Boissière, F., et al. (2021). FTO-mediated cytoplasmic m(6)A(m) demethylation adjusts stem-like properties in colorectal cancer cell. Nat. Commun. 12 (1), 1716. doi:10.1038/s41467-021-21758-4
Sabarneh, A., Ereqat, S., Cauchi, S., AbuShamma, O., Abdelhafez, M., Ibrahim, M., et al. (2018). Common FTO rs9939609 variant and risk of type 2 diabetes in Palestine. BMC Med. Genet. 19 (1), 156. doi:10.1186/s12881-018-0668-8
Sanghera, D. K., Ortega, L., Han, S., Singh, J., Ralhan, S. K., Wander, G. S., et al. (2008). Impact of nine common type 2 diabetes risk polymorphisms in Asian Indian Sikhs: PPARG2 (Pro12Ala), IGF2BP2, TCF7L2 and FTO variants confer a significant risk. BMC Med. Genet. 9, 59. doi:10.1186/1471-2350-9-59
Sarkar, P., Chatterjee, D., and Bandyopadhyay, A. R. (2021). Effect of MTHFR (rs1801133) and FTO (rs9939609) genetic polymorphisms and obesity in T2DM: a study among Bengalee Hindu caste population of West Bengal, India. Ann. Hum. Biol. 48 (1), 62–65. doi:10.1080/03014460.2021.1876920
Scuteri, A., Sanna, S., Chen, W. M., Uda, M., Albai, G., Strait, J., et al. (2007). Genome-wide association scan shows genetic variants in the FTO gene are associated with obesity-related traits. PLoS Genet. 3 (7), e115. doi:10.1371/journal.pgen.0030115
Semenovykh, D., Benak, D., Holzerova, K., Cerna, B., Telensky, P., Vavrikova, T., et al. (2022). Myocardial m6A regulators in postnatal development: effect of sex. Physiol. Res. 71 (6), 877–882. doi:10.33549/physiolres.934970
Shen, F., Huang, W., Huang, J. T., Xiong, J., Yang, Y., Wu, K., et al. (2015). Decreased N(6)-methyladenosine in peripheral blood RNA from diabetic patients is associated with FTO expression rather than ALKBH5. J. Clin. Endocrinol. Metab. 100 (1), E148–E154. doi:10.1210/jc.2014-1893
Shen, W., Li, H., Su, H., Chen, K., and Yan, J. (2021). FTO overexpression inhibits apoptosis of hypoxia/reoxygenation-treated myocardial cells by regulating m6A modification of Mhrt. Mol. Cell. Biochem. 476 (5), 2171–2179. doi:10.1007/s11010-021-04069-6
Shi, X., Cao, Y., Zhang, X., Gu, C., Liang, F., Xue, J., et al. (2021). Comprehensive analysis of N6-methyladenosine RNA methylation regulators expression identify distinct molecular subtypes of myocardial infarction. Front. Cell. Dev. Biol. 9, 756483. doi:10.3389/fcell.2021.756483
Smemo, S., Tena, J. J., Kim, K. H., Gamazon, E. R., Sakabe, N. J., Gómez-Marín, C., et al. (2014). Obesity-associated variants within FTO form long-range functional connections with IRX3. Nature 507 (7492), 371–375. doi:10.1038/nature13138
Speakman, J. R., Rance, K. A., and Johnstone, A. M. (2008). Polymorphisms of the FTO gene are associated with variation in energy intake, but not energy expenditure. Obes. (Silver Spring) 16 (8), 1961–1965. doi:10.1038/oby.2008.318
Stratigopoulos, G., Martin Carli, J. F., O'Day, D. R., Wang, L., Leduc, C. A., Lanzano, P., et al. (2014). Hypomorphism for RPGRIP1L, a ciliary gene vicinal to the FTO locus, causes increased adiposity in mice. Cell. Metab. 19 (5), 767–779. doi:10.1016/j.cmet.2014.04.009
Su, R., Dong, L., Li, Y., Gao, M., Han, L., Wunderlich, M., et al. (2020). Targeting FTO suppresses cancer stem cell maintenance and immune evasion. Cancer Cell. 38 (1), 79–96. doi:10.1016/j.ccell.2020.04.017
Su, X., Shen, Y., Jin, Y., Kim, I. M., Weintraub, N. L., and Tang, Y. (2021). Aging-associated differences in epitranscriptomic m6A regulation in response to acute cardiac ischemia/reperfusion injury in female mice. Front. Pharmacol. 12, 654316. doi:10.3389/fphar.2021.654316
Sun, K., Du, Y., Hou, Y., Zhao, M., Li, J., Du, Y., et al. (2021). Saikosaponin D exhibits anti-leukemic activity by targeting FTO/m(6)A signaling. Theranostics 11 (12), 5831–5846. doi:10.7150/thno.55574
Sun, Q., Geng, H., Zhao, M., Li, Y., Chen, X., Sha, Q., et al. (2022). FTO-mediated m(6) A modification of SOCS1 mRNA promotes the progression of diabetic kidney disease. Clin. Transl. Med. 12 (6), e942. doi:10.1002/ctm2.942
Svensen, N., and Jaffrey, S. R. (2016). Fluorescent RNA aptamers as a tool to study RNA-modifying enzymes. Cell. Chem. Biol. 23 (3), 415–425. doi:10.1016/j.chembiol.2015.11.018
Taneera, J., Khalique, A., Abdrabh, S., Mohammed, A. K., Bouzid, A., El-Huneidi, W., et al. (2024). Fat mass and obesity-associated (FTO) gene is essential for insulin secretion and β-cell function: in vitro studies using INS-1 cells and human pancreatic islets. Life Sci. 339, 122421. doi:10.1016/j.lfs.2024.122421
Taneera, J., Prasad, R. B., Dhaiban, S., Mohammed, A. K., Haataja, L., Arvan, P., et al. (2018). Silencing of the FTO gene inhibits insulin secretion: an in vitro study using GRINCH cells. Mol. Cell. Endocrinol. 472, 10–17. doi:10.1016/j.mce.2018.06.003
Tang, H., Dong, X., Hassan, M., Abbruzzese, J. L., and Li, D. (2011). Body mass index and obesity- and diabetes-associated genotypes and risk for pancreatic cancer. Cancer Epidemiol. Biomarkers Prev. 20 (5), 779–792. doi:10.1158/1055-9965.EPI-10-0845
Tews, D., Fischer-Posovszky, P., Fromme, T., Klingenspor, M., Fischer, J., Rüther, U., et al. (2013). FTO deficiency induces UCP-1 expression and mitochondrial uncoupling in adipocytes. Endocrinology 154 (9), 3141–3151. doi:10.1210/en.2012-1873
Toh, J. D. W., Sun, L., Lau, L. Z. M., Tan, J., Low, J. J. A., Tang, C. W. Q., et al. (2015). A strategy based on nucleotide specificity leads to a subfamily-selective and cell-active inhibitor of N(6)-methyladenosine demethylase FTO. Chem. Sci. 6 (1), 112–122. doi:10.1039/c4sc02554g
Tung, Y. C., Ayuso, E., Shan, X., Bosch, F., O'Rahilly, S., Coll, A. P., et al. (2010). Hypothalamic-specific manipulation of Fto, the ortholog of the human obesity gene FTO, affects food intake in rats. PLoS One 5 (1), e8771. doi:10.1371/journal.pone.0008771
Tung, Y. C., Gulati, P., Liu, C. H., Rimmington, D., Dennis, R., Ma, M., et al. (2015). FTO is necessary for the induction of leptin resistance by high-fat feeding. Mol. Metab. 4 (4), 287–298. doi:10.1016/j.molmet.2015.01.011
UniProt Consortium (2023). UniProt: the universal protein knowledgebase in 2023. Nucleic Acids Res. 51 (D1), D523–d531. doi:10.1093/nar/gkac1052
Vasan, S. K., Karpe, F., Gu, H. F., Brismar, K., Fall, C. H., Ingelsson, E., et al. (2014). FTO genetic variants and risk of obesity and type 2 diabetes: a meta-analysis of 28,394 Indians. Obes. (Silver Spring) 22 (3), 964–970. doi:10.1002/oby.20606
Vausort, M., Niedolistek, M., Lumley, A. I., Oknińska, M., Paterek, A., Mączewski, M., et al. (2022). Regulation of N6-methyladenosine after myocardial infarction. Cells 11 (15), 2271. doi:10.3390/cells11152271
Wang, L., Song, C., Wang, N., Li, S., Liu, Q., Sun, Z., et al. (2020b). NADP modulates RNA m(6)A methylation and adipogenesis via enhancing FTO activity. Nat. Chem. Biol. 16 (12), 1394–1402. doi:10.1038/s41589-020-0601-2
Wang, P., Yang, F. J., Du, H., Guan, Y. F., Xu, T. Y., Xu, X. W., et al. (2011). Involvement of leptin receptor long isoform (LepRb)-STAT3 signaling pathway in brain fat mass- and obesity-associated (FTO) downregulation during energy restriction. Mol. Med. 17 (5-6), 523–532. doi:10.2119/molmed.2010.00134
Wang, R., Han, Z., Liu, B., Zhou, B., Wang, N., Jiang, Q., et al. (2018). Identification of natural compound radicicol as a potent FTO inhibitor. Mol. Pharm. 15 (9), 4092–4098. doi:10.1021/acs.molpharmaceut.8b00522
Wang, T., Hong, T., Huang, Y., Su, H., Wu, F., Chen, Y., et al. (2015). Fluorescein derivatives as bifunctional molecules for the simultaneous inhibiting and labeling of FTO protein. J. Am. Chem. Soc. 137 (43), 13736–13739. doi:10.1021/jacs.5b06690
Wang, W., Yang, K., Wang, S., Zhang, J., Shi, Y., Zhang, H., et al. (2022a). The sex-specific influence of FTO genotype on exercise intervention for weight loss in adult with obesity. Eur. J. Sport Sci. 22 (12), 1926–1931. doi:10.1080/17461391.2021.1976843
Wang, X., Huang, N., Yang, M., Wei, D., Tai, H., Han, X., et al. (2017). FTO is required for myogenesis by positively regulating mTOR-PGC-1α pathway-mediated mitochondria biogenesis. Cell. Death Dis. 8 (3), e2702. doi:10.1038/cddis.2017.122
Wang, X., Wu, R., Liu, Y., Zhao, Y., Bi, Z., Yao, Y., et al. (2020a). m(6)A mRNA methylation controls autophagy and adipogenesis by targeting Atg5 and Atg7. Autophagy 16 (7), 1221–1235. doi:10.1080/15548627.2019.1659617
Wang, X., Wu, Y., Guo, R., Zhao, L., Yan, J., and Gao, C. (2022b). Comprehensive analysis of N6-methyladenosine RNA methylation regulators in the diagnosis and subtype classification of acute myocardial infarction. J. Immunol. Res. 2022, 5173761. doi:10.1155/2022/5173761
Wardle, J., Carnell, S., Haworth, C. M. A., Farooqi, I. S., O'Rahilly, S., and Plomin, R. (2008). Obesity associated genetic variation in FTO is associated with diminished satiety. J. Clin. Endocrinol. Metab. 93 (9), 3640–3643. doi:10.1210/jc.2008-0472
Wardle, J., Llewellyn, C., Sanderson, S., and Plomin, R. (2009). The FTO gene and measured food intake in children. Int. J. Obes. (Lond) 33 (1), 42–45. doi:10.1038/ijo.2008.174
Wei, J., Liu, F., Lu, Z., Fei, Q., Ai, Y., He, P. C., et al. (2018). Differential m(6)A, m(6)A(m), and m(1)A demethylation mediated by FTO in the cell nucleus and cytoplasm. Mol. Cell. 71 (6), 973–985. doi:10.1016/j.molcel.2018.08.011
Wen, C., Lan, M., Tan, X., Wang, X., Zheng, Z., Lv, M., et al. (2022). GSK3β exacerbates myocardial ischemia/reperfusion injury by inhibiting myc. Oxid. Med. Cell. Longev. 2022, 2588891. doi:10.1155/2022/2588891
WHO (2022). Diabetes. Available at: https://www.who.int/news-room/fact-sheets/detail/diabetes.
WHO (2021). Cardiovasc. Dis. (CVDs). (cvds). Available at: https://www.who.int/news-room/fact-sheets/detail/cardiovascular-diseases-.
WHO. Obes. overweight. 2021 Available at: https://www.who.int/news-room/fact-sheets/detail/obesity-and-overweight.
Wu, R., Chen, Y., Liu, Y., Zhuang, L., Chen, W., Zeng, B., et al. (2021). m6A methylation promotes white-to-beige fat transition by facilitating Hif1a translation. EMBO Rep. 22 (11), e52348. doi:10.15252/embr.202052348
Wu, R., Liu, Y., Yao, Y., Zhao, Y., Bi, Z., Jiang, Q., et al. (2018). FTO regulates adipogenesis by controlling cell cycle progression via m(6)A-YTHDF2 dependent mechanism. Biochim. Biophys. Acta Mol. Cell. Biol. Lipids 1863 (10), 1323–1330. doi:10.1016/j.bbalip.2018.08.008
Wu, W., Feng, J., Jiang, D., Zhou, X., Jiang, Q., Cai, M., et al. (2017). AMPK regulates lipid accumulation in skeletal muscle cells through FTO-dependent demethylation of N(6)-methyladenosine. Sci. Rep. 7, 41606. doi:10.1038/srep41606
Xu, Y. Y., Li, T., Shen, A., Bao, X. Q., Lin, J. F., Guo, L. Z., et al. (2023). FTO up-regulation induced by MYC suppresses tumour progression in Epstein-Barr virus-associated gastric cancer. Clin. Transl. Med. 13 (12), e1505. doi:10.1002/ctm2.1505
Xu, Z., Qin, Y., Lv, B., Tian, Z., and Zhang, B. (2022). Intermittent fasting improves high-fat diet-induced obesity cardiomyopathy via alleviating lipid deposition and apoptosis and decreasing m6A methylation in the heart. Nutrients 14 (2), 251. doi:10.3390/nu14020251
Yajnik, C. S., Janipalli, C. S., Bhaskar, S., Kulkarni, S. R., Freathy, R. M., Prakash, S., et al. (2009). FTO gene variants are strongly associated with type 2 diabetes in South Asian Indians. Diabetologia 52 (2), 247–252. doi:10.1007/s00125-008-1186-6
Yang, C., Zhao, K., Zhang, J., Wu, X., Sun, W., Kong, X., et al. (2021a). Comprehensive analysis of the transcriptome-wide m6A methylome of heart via MeRIP after birth: day 0 vs. Day 7. Front. Cardiovasc Med. 8, 633631. doi:10.3389/fcvm.2021.633631
Yang, H., Xuan, L., Wang, S., Luo, H., Duan, X., Guo, J., et al. (2024a). LncRNA CCRR maintains Ca(2+) homeostasis against myocardial infarction through the FTO-SERCA2a pathway. Sci. China Life Sci. 67, 1601–1619. doi:10.1007/s11427-023-2527-5
Yang, Y., Ren, J., Zhang, J., Shi, H., Wang, J., and Yan, Y. (2024b). FTO ameliorates doxorubicin-induced cardiotoxicity by inhibiting ferroptosis via P53-P21/Nrf2 activation in a HuR-dependent m6A manner. Redox Biol. 70, 103067. doi:10.1016/j.redox.2024.103067
Yang, Y., Shen, F., Huang, W., Qin, S., Huang, J. T., Sergi, C., et al. (2019). Glucose is involved in the dynamic regulation of m6A in patients with type 2 diabetes. J. Clin. Endocrinol. Metab. 104 (3), 665–673. doi:10.1210/jc.2018-00619
Yang, Z., Jiang, X., Zhang, Z., Zhao, Z., Xing, W., Liu, Y., et al. (2021b). HDAC3-dependent transcriptional repression of FOXA2 regulates FTO/m6A/MYC signaling to contribute to the development of gastric cancer. Cancer Gene Ther. 28 (1-2), 141–155. doi:10.1038/s41417-020-0193-8
Younus, L. A., Algenabi, A. H. A., Abdul-Zhara, M. S., and Hussein, M. K. (2017). FTO gene polymorphisms (rs9939609 and rs17817449) as predictors of Type 2 Diabetes Mellitus in obese Iraqi population. Gene 627, 79–84. doi:10.1016/j.gene.2017.06.005
Yu, H., Armstrong, N., Pavela, G., and Kaiser, K. (2023a). Sex and race differences in obesity-related genetic susceptibility and risk of cardiometabolic disease in older US adults. JAMA Netw. Open 6 (12), e2347171. doi:10.1001/jamanetworkopen.2023.47171
Yu, P., Wang, J., Xu, G. E., Zhao, X., Cui, X., Feng, J., et al. (2023b). RNA m(6)a-regulated circ-znf609 suppression ameliorates doxorubicin-induced cardiotoxicity by upregulating FTO. JACC Basic Transl. Sci. 8 (6), 677–698. doi:10.1016/j.jacbts.2022.12.005
Yu, Y., Pan, Y., Fan, Z., Xu, S., Gao, Z., Ren, Z., et al. (2021). LuHui derivative, A novel compound that inhibits the fat mass and obesity-associated (FTO), alleviates the inflammatory response and injury in hyperlipidemia-induced cardiomyopathy. Front. Cell. Dev. Biol. 9, 731365. doi:10.3389/fcell.2021.731365
Zano, S., and Baig, S. (2022). Association of FTO variant with parental history of type 2 diabetes mellitus in adults. J. Pak Med. Assoc. 72 (10), 2009–2013. doi:10.47391/JPMA.4299
Zeng, H., Xu, J., Wu, R., Wang, X., Jiang, Y., Wang, Q., et al. (2024). FTO alleviated ferroptosis in septic cardiomyopathy via mediating the m6A modification of BACH1. Biochim. Biophys. Acta Mol. Basis Dis. 1870, 167307. doi:10.1016/j.bbadis.2024.167307
Zhang, B., Jiang, H., Wu, J., Cai, Y., Dong, Z., Zhao, Y., et al. (2021a). m6A demethylase FTO attenuates cardiac dysfunction by regulating glucose uptake and glycolysis in mice with pressure overload-induced heart failure. Signal Transduct. Target Ther. 6 (1), 377. doi:10.1038/s41392-021-00699-w
Zhang, B., Xu, Y., Cui, X., Jiang, H., Luo, W., Weng, X., et al. (2021b). Alteration of m6A RNA methylation in heart failure with preserved ejection fraction. Front. Cardiovasc Med. 8, 647806. doi:10.3389/fcvm.2021.647806
Zhang, M., Zhang, Y., Ma, J., Guo, F., Cao, Q., Zhang, Y., et al. (2015). The demethylase activity of FTO (fat mass and obesity associated protein) is required for preadipocyte differentiation. PLoS One 10 (7), e0133788. doi:10.1371/journal.pone.0133788
Zhang, Y., Chen, L., Zhu, J., Liu, H., Xu, L., Wu, Y., et al. (2023). Minor alleles of FTO rs9939609 and rs17817449 polymorphisms confer a higher risk of type 2 diabetes mellitus and dyslipidemia, but not coronary artery disease in a Chinese Han population. Front. Endocrinol. (Lausanne) 14, 1249070. doi:10.3389/fendo.2023.1249070
Zhang, Y., Chen, Y., Guo, Q., and Liu, A. (2024). Fat mass and obesity-associated protein (FTO)-induced upregulation of flotillin-2 (FLOT2) contributes to cancer aggressiveness in diffuse large B-cell lymphoma (DLBCL) via activating the PI3K/Akt/mTOR signal pathway. Arch. Biochem. Biophys. 758, 110072. doi:10.1016/j.abb.2024.110072
Zhao, X., Yang, Y., Sun, B. F., Shi, Y., Yang, X., Xiao, W., et al. (2014). FTO-dependent demethylation of N6-methyladenosine regulates mRNA splicing and is required for adipogenesis. Cell. Res. 24 (12), 1403–1419. doi:10.1038/cr.2014.151
Zheng, G., Cox, T., Tribbey, L., Wang, G. Z., Iacoban, P., Booher, M. E., et al. (2014). Synthesis of a FTO inhibitor with anticonvulsant activity. ACS Chem. Neurosci. 5 (8), 658–665. doi:10.1021/cn500042t
Keywords: FTO, m6A, m6Am, obesity, diabetes, cardiovascular disease, cancer
Citation: Benak D, Sevcikova A, Holzerova K and Hlavackova M (2024) FTO in health and disease. Front. Cell Dev. Biol. 12:1500394. doi: 10.3389/fcell.2024.1500394
Received: 23 September 2024; Accepted: 05 December 2024;
Published: 18 December 2024.
Edited by:
Adelaide Fernandes, University of Lisbon, PortugalReviewed by:
Yongjie Xu, Xinyang Normal University, ChinaEmery Di Cicco, Harvard Medical School, United States
Copyright © 2024 Benak, Sevcikova, Holzerova and Hlavackova. This is an open-access article distributed under the terms of the Creative Commons Attribution License (CC BY). The use, distribution or reproduction in other forums is permitted, provided the original author(s) and the copyright owner(s) are credited and that the original publication in this journal is cited, in accordance with accepted academic practice. No use, distribution or reproduction is permitted which does not comply with these terms.
*Correspondence: Marketa Hlavackova, bWFya2V0YS5obGF2YWNrb3ZhQGZndS5jYXMuY3o=