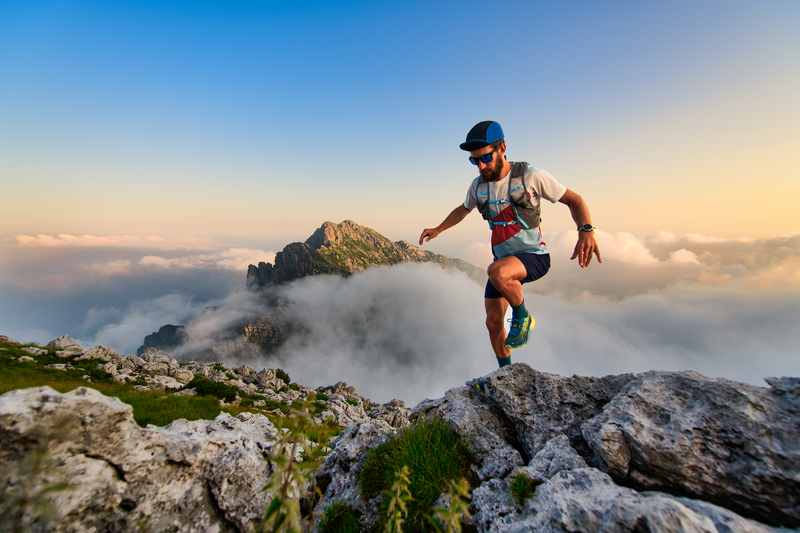
95% of researchers rate our articles as excellent or good
Learn more about the work of our research integrity team to safeguard the quality of each article we publish.
Find out more
REVIEW article
Front. Cell Dev. Biol. , 18 December 2024
Sec. Molecular and Cellular Pathology
Volume 12 - 2024 | https://doi.org/10.3389/fcell.2024.1466997
Human lungs consist of a distinctive array of cell types, which are subjected to persistent challenges from chemical, mechanical, biological, immunological, and xenobiotic stress throughout life. The disruption of endoplasmic reticulum (ER) homeostatic function, triggered by various factors, can induce ER stress. To overcome the elevated ER stress, an adaptive mechanism known as the unfolded protein response (UPR) is activated in cells. However, persistent ER stress and maladaptive UPR can lead to defects in proteostasis at the cellular level and are typical features of the lung aging. The aging lung and associated lung diseases exhibit signs of ER stress-related disruption in cellular homeostasis. Dysfunction resulting from ER stress and maladaptive UPR can compromise various cellular and molecular processes associated with aging. Hence, comprehending the mechanisms of ER stress and UPR components implicated in aging and associated lung diseases could enable to develop appropriate therapeutic strategies for the vulnerable population.
The process of aging is intricate, involving the gradual decline of tissues and organs throughout the body, representing a standalone risk factor for various age-related illnesses (Zhang W. et al., 2020). As life expectancy rises and the aging demographic expands globally, it is crucial from a public health standpoint to comprehend the mechanisms by which aging contributes to an escalating vulnerability to chronic illnesses and disabilities over time (Ferrucci and Fabbri, 2018).
The human lungs, constituting most of the body’s surface area, serve as a distinctive interface for engaging with the external environment and continually face biological, chemical, mechanical, and immunological stress throughout an individual’s lifespan (Schneider et al., 2021). As age progresses, the lungs undergo a gradual weakening characterized by structural changes that hinder gas exchange and weaken defensive mechanisms. Consequently, this susceptibility heightens the risk of lung injuries induced by environmental exposures (Sharma and Goodwin, 2006; Bowdish, 2019) and contributing to increased susceptibility to several lung diseases. Notably, conditions like chronic obstructive pulmonary disease (COPD) and idiopathic pulmonary fibrosis (IPF), prevalent in the elderly, not only align with accelerated aging but also replicate the aged lung’s structural and physiological traits (Ito and Barnes, 2009; Pardo and Selman, 2016). The COVID-19 pandemic has highlighted the heightened vulnerability of the elderly to acute respiratory distress syndrome (ARDS) (Chen et al., 2021). Additionally, while asthma is traditionally viewed as a childhood ailment, severe forms with elevated morbidity and mortality rates are more prevalent in the elderly population (Gillman and Douglass, 2012).
Recent findings suggest that intervening in age-related degenerative biological processes can mitigate or postpone the emergence of various age-related diseases. Experimental trials in model animals have further established a fact regarding aging that it is a manageable as well as alterable condition (de Cabo and Mattson, 2019; Abbasi, 2017).
The endoplasmic reticulum (ER) is a membranous network consisting of branching tubules and flattened sacs. It plays a crucial role in overseeing the synthesis, folding, and processing of over one-third of all cellular proteins (Walter and Ron, 2011). Within cells equipped with an endoplasmic reticulum (ER), such as those found in the lungs, the secretory capacity undergoes constant influence from both physiological demands and pathological disturbances. This results in a disruption of ER homeostasis, recognized as ER stress (Dastghaib et al., 2021). In response to heightened ER stress, adaptive mechanisms, collectively termed the unfolded protein response (UPR), come into play to restore equilibrium (Wiseman et al., 2022).
The aging process has a significant impact on cellular functions, mainly affecting the chaperone system, leading to an essential role in heightened protein misfolding and aggregation (Macario and Conway de Macario, 2002). Contemporary findings propose an association between disturbances in proteome homeostasis (proteostasis) and the standard aging process, contributing to age-related disroders in lungs (Schneider et al., 2021; Balch et al., 2014). Consequently, it is unsurprising that the aging lung exhibits various indications of disruption in cellular homeostasis due to ER stress. Additionally, malfunctioning of cellular homeostasis due to ER stress can impair other metabolic functions responsible for aging. Therefore, understanding these inter-relationships is important because interventions targeting these relationships should have a beneficial effect on delaying aging and preventing aging-related disorders. In this context, we examined ER stress dynamics for aging and associated lung disorders and discussed attempts to target ER stress in the lungs to achieve an antiaging effect; this research could enable to develop therapeutic strategies for the vulnerable population.
The ER constitutes a complex structure of branching tubules and flattened sacs, overseeing the synthesis, folding, and processing of majority of cellular proteins (Hetz and Papa, 2018). The ER is also responsible for Ca2+ storage and biosynthesis of lipids and steroids (Hetz et al., 2020). In the ER, chaperones and enzymes ensure proper folding and modification of secretory proteins, maintaining biosynthetic function (Walter and Ron, 2011). Upon attaining the correct conformation, chaperones within the ER disengage from proteins, enabling their exit. Subsequently, cells efficiently remove improperly folded proteins through stringent quality control mechanisms such as ER-associated degradation (ERAD) and ER-phagy.
While the endoplasmic reticulum (ER) is resilient, cells frequently operate near their secretory capacity limits. Various factors, including environmental, genetic, disease-related, or aging-related influences, can perturb protein folding efficiency, resulting in the buildup of misfolded or unfolded proteins in the ER lumen, causing “ER stress” (Walter and Ron, 2011). In response to ER stress, the UPR signal transduction pathway is activated within the cell to safeguard the genuineness and integrity of proteins. The UPR modifies cellular transcription and translation programs, impacting diverse parameters related to protein metabolism, redox homeostasis, and apoptosis (Walter and Ron, 2011; Wang and Kaufman, 2012).
Briefly, the UPR signal comprises three major stress-related proteins situated on the ER membrane: protein kinase R-like ER kinase (PERK), activating transcription factor 6 (ATF6), and inositol-requiring enzyme 1 (IRE1) (Wiseman et al., 2022) (Figure 1). PERK and IRE1 are type I transmembrane proteins that have a luminal domain for sensing unfolded proteins and a cytoplasmic kinase domain. In addition, IRE1 has a cytoplasmic endoribonuclease activity domain (Karagöz et al., 2017). In mammals IRE1 has two isoforms, namely the IRE1α and IRE1β. IRE1α is primarily expressed in almost all tissues. whereas IRE1β is found exclusively in intestinal epithelial and airway mucous cells (Akhter et al., 2020). IRE1β functions at the interface of epithelial cells with the external environment, maintaining mucosal homeostasis. ATF6 is a type II transmembrane protein that resides in the ER membrane. It has a luminal domain that senses ER stress and a cytoplasmic transcription factor domain (Lei et al., 2024).
Figure 1. ER stress and activation of the UPR signaling pathways. The UPR is triggered by ER stress sensors, namely IRE1, PERK, and ATF6 upon UPR target genes related dissociation of BiP. The activation of PERK through its dimerization and autophosphorylation suppresses the overall protein synthesis by eukaryotic translation initiation factor 2 subunit α (eIF2α) phosphorylation. PERK activation also increases the formation of the transcription factor ATF4 that regulates several components involved in amino acid transport and protein folding. IRE1α is activated following its dissociation from BiP and spontaneously undergoes homodimerization and autophosphorylation; the modified IRE1α induces splicing of the X-box binding protein 1 (XBP1) mRNA to generate sXBP1 that regulates several UPR target genes responsible for protein folding, lipid synthesis, and quality control of cellular proteins. The activated ATF6 is processed in the Golgi apparatus by site 1 protease (S1P) and site 2 protease (S2P) to release the cytosolic domain ATF6f that regulates some UPR target genes related to XBP1, protein folding, and quality control of cellular proteins.
The initial phase of the UPR commences with the activation of PERK. When unfolded proteins accumulate in the ER, they bind to BiP, causing BiP to dissociate from PERK. This dissociation enables PERK to oligomerize and autophosphorylate (Brown et al., 2014). This process inhibits overall protein synthesis by phosphorylating eIF2α, reducing mRNA translation and preventing protein buildup in the ER. Notably, it enhances the translation of specific mRNAs, including ATF4, which activates CHOP, illustrating the UPR’s cascade to maintain cellular homeostasis under ER stress (Han et al., 2013; Tabas and Ron, 2011). CHOP undergoes nuclear translocation, regulating elements of the B cell lymphoma protein 2 (BCL-2) protein family to initiate apoptosis mediated by ER stress. During ER stress, IRE1α activates after dissociating from GRP78/BiP, homodimerizes, and autophosphorylates. This leads to the activation of the cytosolic RNase domain, which splices the mRNA encoding XBP1 (X-box-binding protein 1), removing a 26-nucleotide intron. This splicing event converts unspliced XBP1 (uXBP1) into spliced XBP1 (sXBP1), a potent transcription factor (Calfon et al., 2002; Yoshida et al., 2001). sXBP1 functions as an active transcription factor, overseeing the regulation of various genes associated with protein functions as well as lipid, ER, and Golgi biogenesis (Acosta-Alvear et al., 2007; He et al., 2010). Under normal conditions, IRE1β restricts the activity of IRE1α and UPR signaling (Grey et al., 2020). During stressful situations, IRE1β can still play a role in XBP1 splicing, thereby helping to adjust the protein folding capacity of epithelial cells and restore mucosal homeostasis. Additionally, IRE1β has been identified as a negative regulator of IRE1α, as its overexpression is able to decrease IRE1α-dependent XBP1 splicing. As such, IRE1β is believed to alleviate ER stress in the mucosal epithelium during inflammation-induced diseases (Le Goupil et al., 2024). Upon encountering ER stress, ATF6 is activated through a mechanism distinct from both IRE1 and PERK. BiP separates from ATF6α, revealing the Golgi localization signal of ATF6α. ATF6 transitions to its monomeric form and moves to the Golgi apparatus. Processing in the Golgi via COP II vesicles, where S1P and S2P cleave ATF6 in the transmembrane domain, produces the active ATF6 fragment and releases the cytosolic domain of ATF6 (Ye et al., 2004; Chen et al., 2002). Similar to sXBP1, the ATF6 fragment actively medicates the gene regulation related to XBP1 and protein functions (Janssens et al., 2014).
Initially deemed protective, the UPR serves to decrease protein load and alleviate ER stress. Nevertheless, if the UPR persists in an extended or overly activated state, it can transition into a maladaptive mode, posing the risk of irreversible cell injury and, in severe cases, cell death. (Hetz and Papa, 2018).
The aging of the lungs can ensue from the cumulative alterations in the cellular systems, reflecting the interplay between injury and repair within the lung (Abadie et al., 2005). These changes disrupt lung cell homeostasis by affecting proteostasis, cellular senescence, stem cell reservoirs, oxidative stress, and mitochondrial function.
The biological function of the lungs is compromised in advanced age, irrespective of the presence of specific diseases. The major lung components, including respiratory epithelium, lung progenitor cells, the interstitium, and pulmonary immune cells, are altered in the aged lung (Schneider et al., 2021), eventually leading to a reduced surface for gas exchange (Lalley, 2013), decreased mucociliary clearance in both upper and lower airways (Proença de Oliveira-Maul et al., 2013; Svartengren et al., 2005), and impaired repair and regenerative ability, subsequently resulting in the development of emphysema and pulmonary fibrosis (Kotton and Morrisey, 2014). Interactions between aging, senescence, and environmental factors lead to the breakdown of stress response pathways, contributing to the development of various lung diseases (Luppi et al., 2021).
The structural and functional changes in the aging lung collectively lead to decreased lung function, diminished compensatory mechanism, and enhanced susceptibility to pulmonary diseases. Consequently, lung diseases disproportionately affect elderly individuals. Therefore, to delay these aging-related lung diseases, it is important to modify the biological processes that deteriorate with age.
Aging can influence the structure of the ER inside the cell (Erickson et al., 2006; Moltedo et al., 2019). In individuals with COPD, lung fibroblasts exhibit a less organized and patchy ER structure compared to the reticulated ER structure observed in the lung fibroblasts of both never-smokers and ever-smokers (Weidner et al., 2018).
The functionality of the UPR is compromised as a result of the age-related gradual deterioration in the apparatus essential for the proper folding of proteins; consequently, in aged individuals, UPR activation cannot rescue ER stress (Naidoo, 2009). For example, salient chaperones and enzymes such as GRP78, PDI, calnexin, and GRP94, in the ER are impaired during the aging process (Nuss et al., 2008). This functional decline in chaperones and enzymes may be caused by progressive oxidation with advancing age (Nuss et al., 2008). Normally, the protective UPR and apoptotic signals are in a balanced state in a cell; however, this equilibrium might incline towards the proapoptotic condition as age progresses, attributable to the gradual deterioration in the UPR. Aging diminishes PERK expression and kinase activity, inhibiting the cytoprotective phosphorylation of eIF2α. This condition promotes protein translation and the expression of proapoptotic proteins in the ER during aging (Hussain and Ramaiah, 2007; Paz Gavilán et al., 2006). Similarly, CHOP and caspase-12 expression was induced in aged, stressed rats, but not in young, stressed ones (Paz Gavilán et al., 2006), suggesting more vulnerability of aged-animals to apoptosis.
The ubiquitin-proteasome system (UPS) plays an important role in the cellular processes for protein quality control. UPS maintains the homeostasis of proteins within the cell, ensuring correct protein folding, function, and degradation (Park et al., 2020). The UPS is responsible for the degradation of most short-lived intracellular proteins in eukaryotes by removing over 90% of improperly folded and damaged proteins. Additionally, the UPS actively participates in the regulation of numerous signaling pathways, including mTOR, UPR, as well as both innate and adaptive immune responses. Regulating protein degradation is an integral part of the UPR to alleviate ER stress (Xia et al., 2020). However, this system is impaired with age (Sun-Wang et al., 2020). Age-related UPS dysfunction involves reduced proteasome subunit expression, altered composition, and changes in ubiquitin enzyme activity. Effects may vary by tissue due to differing proteasome activities (Sun-Wang et al., 2020). Proteasome activity remains intact in healthy aged mouse lungs, but caspase-like activity significantly declines compared to young mice (Caniard et al., 2015). Another study confirmed a decline in the proteasome activity in the lungs of 2-year-old aged male rats as compared to that in the lungs of 2-week-old male rats (Keller et al., 2000). When the functions of UPS are compromised, the degradation of ERAD substrates is prevented, causing the accumulation of unfolded or misfolded proteins in the ER lumen. This accumulation triggers the UPR (Ebstein et al., 2019).
In summary, the decline in ER mechanisms for maintaining protein quality and the attenuation of degradation pathways overseeing protein quality control lead to a significant rise in misfolded proteins within the ER, ultimately triggering prolonged ER stress in aging lung tissue.
Hormesis, characterized by a dose–response dynamic where low doses stimulate and high doses inhibit the reaction, (Calabrese, 2008), signifies the adaptive responses of cells and organisms to mild or moderate stressors, such as heat, hypoxia, caloric restriction, and oxidative stress. This phenomenon has played a pivotal role in the evolutionary process (Salminen and Kaarniranta, 2010).
ER stress is a paradigm of this hormetic regulation. The buildup of unfolded and incorrectly folded proteins in the ER cavity swiftly initiates ER stress. To overcome this ER stress, cells use a dynamic intracellular UPR process. This mechanism activates adaptive initiatives to adjust and enhance crucial elements of the entire secretory pathway (Hetz and Papa, 2018). If this reaction proves effective, the response restores cellular balance and promotes survival under ER stress. However, persistent ER stress can lead to oxidative stress, inflammation, and apoptosis via UPR pathways (Wang and Kaufman, 2016; Zhang and Kaufman, 2008).
Hormesis of ER stress can also involve the regulation of cell lifespan in a dose-dependent manner. Aging weakens the adaptive UPR-based defense, heightening vulnerability to ER stress and reducing stress resistance. This increases susceptibility to pathological changes, including protein issues, mitochondrial impairments, and disruptions in Ca2+ homeostasis, leading to apoptotic cell death.
COPD is marked by partially reversible airflow limitation, chronic inflammation, and emphysematous lung destruction, resembling a condition of accelerated lung aging (Barnes, 2017).
ER stress plays a key role in COPD development, leading to alveolar epithelial cells (AECs) apoptosis (Yu et al., 2022). Elevated GRP78 levels in lung tissues, lavage fluid, and serum are associated with decreased lung function and severe emphysema in smokers and COPD patients (Aksoy et al., 2017; Merali et al., 2014). Cigarette smoke (CS) exposure is the primary driver of COPD pathogenesis and progression (Vogelmeier et al., 2017). CS-induced pathogenic effects involve ER stress and UPR, elevating misfolded protein levels, including impaired PDI, a crucial ER foldase (Kenche et al., 2013; van Rijt et al., 2012). Additionally, IRE1α exacerbates airway inflammation caused by CS through NF-κB signaling (Wang et al., 2017), and it plays a crucial role in nicotine-induced epithelial-mesenchymal transition, contributing to airway remodeling in COPD and impeding cell migration in human bronchial epithelial (HBE) cells (Lin et al., 2022). Cigarette smoke extract (CSE) induces apoptosis by triggering the PERK/eIF2α/CHOP pathway through the superoxide anion (Tagawa et al., 2011).
IPF, a progressive lung dysfunction, exhibits symptoms like dyspnea and respiratory failure, and its incidence rises with the aging population (Wolters et al., 2014). While the exact pathogenic mechanism is unclear, evidence links ER stress to IPF, observed in lung samples from patients with familial and sporadic IPF (Ghavami et al., 2018; Korfei et al., 2008; Lawson et al., 2008). The profibrotic impacts of ER stress can be communicated across different lung cell types. In AECs of IPF patients, there was a notable rise in the levels of various ER stress markers, including ATF4, ATF6, CHOP BiP, EDEM, and XBP1, particularly observed in type II AECs (Korfei et al., 2008; Lawson et al., 2008). Mice with asbestos-induced lung fibrosis and individuals with asbestosis displayed increased expression of GRP78 in their respective macrophages (Ryan et al., 2014). Additionally, ER stress plays a role in collagen and fibronectin production induced by transforming growth factor β1 (TGFβ1) in fibroblasts (Zimmerman et al., 2013). ER stress has the capacity to influence critical elements of lung fibrosis, including the AECs, polarization of M2 macrophages, and differentiation of myofibroblasts (Burman et al., 2018). CHOP may participate in ER stress-dependent AEC apoptosis (Korfei et al., 2008). In CHOP-deficient mice treated with bleomycin, decreased AEC apoptosis and lung fibrosis were observed (Tanaka et al., 2015). Furthermore, CHOP has been identified as an inducer of M2 polarization in bleomycin-induced pulmonary fibrosis (Yao et al., 2016). The inhibition of ER stress in fibroblasts from patients with IPF significantly reduces TGFβ1-induced myofibroblast differentiation, αSMA expression, and collagen production (Baek et al., 2012). This indicates the potential therapeutic impact of targeting ER stress pathways in pulmonary fibrosis.
Asthma, a complex chronic airway disease, results from intricate interactions between genetic, environmental factors, and aging (Nagasaki and Matsumoto, 2013). Elderly patients with asthma are more susceptible to sensitization by allergens than age-matched controls (Burrows et al., 1991). Elderly individuals with asthma experience increased morbidity and mortality along with various comorbidities compared to adults; moreover, it is clinically characterized by neutrophilic inflammation rather than by eosinophilic inflammation and less frequent atopy, with a reduced response to treatment (Boulet, 2016).
The pathogenesis of asthma involves the activation of ER stress and the unfolded protein response (UPR). Genetic factors related to ER stress, such as orosomucoid-like 3 (ORMDL3), contribute to asthma development by influencing calcium homeostasis, ER stress response, and inflammatory processes (Schmiedel et al., 2016; Breslow et al., 2010; Luthers et al., 2020). Previous studies have shown that genetic variation affecting ORMDL3 expression is an important determinant of asthma susceptibility and predisposition to other autoimmune or inflammatory diseases (Moffatt et al., 2007; James et al., 2019). Stimuli such as aeroallergens, microorganisms, smoking, and pollutants are critical factors in the development of allergic asthma; these stimulants can disrupt ER integrity and induce ER stress (Pathinayake et al., 2018; Osorio et al., 2013). According to previous studies, ER stress is activated in AECs and immune cells in the presence of asthma (Pathinayake et al., 2018; Kim and Lee, 2015). The immediate and sustained activation of the UPR signaling pathway initiates allergic airway inflammation (Osorio et al., 2013; Makhija et al., 2014), particularly in severe or asthma unresponsive to steroids through NF-κB modulation (Kim S. R. et al., 2013). Experimental observations in a mice asthma model indicated that the application of chemical chaperones to mitigate the ER stress response displayed promise in alleviating airway hyperresponsiveness (Kim and Lee, 2015; Makhija et al., 2014; Siddesha et al., 2016).
Elderly individuals show a reduced capacity to resist infections, including influenza (Gross et al., 1995) and COVID-19 (Chen et al., 2021). Thus, elderly persons are more susceptible to acute lung injury (ALI) that culminates into ARDS and show high mortality or prolonged morbidity due to ARDS-related complications and fibrosis (Meduri and Eltorky, 2015). ARDS encompasses a sudden inflammatory reaction triggered by the impairment of the alveolar capillary barrier, stemming from pulmonary or extra-pulmonary origins (Meduri and Eltorky, 2015; Confalonieri et al., 2017), leading to the impairment of pulmonary vascular permeability, alveolar flooding, and diminished respiratory capacity.
Numerous investigations have demonstrated elevated levels of ER stress markers and UPR mediators, including GRP78, in patients with ALI/ARDS and rodent models of lipopolysaccharide-induced ALI (Kim H. J. et al., 2013; Kim et al., 2015; Zeng et al., 2017). Similarly, suppressing ER stress mitigates endotoxin-triggered acute lung injury (ALI) in both in vivo and in vitro settings. Additionally, restraining the UPR diminishes ALI induced by the Middle East respiratory syndrome coronavirus, acting on the apoptotic pathway downstream of UPR (Sims et al., 2021).
The ER stress signaling components are associated with the cellular signaling network that regulates longevity, thus suggesting a functional relationship between ER stress and aging-related pathways.
Inflammation is a fundamental protective mechanism of the innate immune system to combat both invading pathogens and endogenously produced toxic substances (Mogensen, 2009), and it is tightly regulated under normal conditions. However, prolonged and sustained inflammation can be detrimental to normal health. Inflammatory pathogenesis is a common mechanism for the development of most age-related diseases (Franceschi and Campisi, 2014). The term inflammaging, a low-grade, chronic, sterile inflammation, is widely accepted as a critical risk factor for aging and major diseases (Santoro et al., 2021). The theory of antagonistic pleiotropy provides a better explanation for the role of inflammation in aging. An evolutionary process, inflammation, manifests significant impacts during early life and adulthood; however, it turns detrimental in old age when the impact of natural selection wanes (Franceschi et al., 2017).
The susceptibility of the lungs to aging increases with age, and inflammaging contributes to a proinflammatory environment with diminished resilience against challenges (Faniyi et al., 2022). Inflammation in airway epithelia increases protein synthesis demand, causing an overload of unfolded polypeptide chains in the ER, leading to ER stress and UPR activation (Ribeiro and O'Neal, 2012; Ribeiro and Boucher, 2010) (Figure 2). ER stress also amplifies the cytokine-mediated inflammatory response, leading to the pathogenesis of inflammatory diseases (Zhang and Kaufman, 2008). Thus, a reciprocal relationship appears to be present between ER stress and inflammation; this loop might aggravate the pathological condition of the lung, given that the respiratory tract is an important immune interface with continuous exposure to environmental stress (Schneider et al., 2021).
Figure 2. The insults to airway epithelial cells, their impact on UPR and the consequences of UPR activation. Cigarette smoke, pollution, aging, and pathogens invade airway epithelial cells, causing oxidative stress, inflammatory responses, and cellular damage. These insults lead to the accumulation of misfolded or unfolded proteins within the ER lumen.The three signaling pathways of UPR are activated. PERK activation increases the formation of the transcription factor ATF4 that reduces protein synthesis. IRE1 activation results in the production of sXBP1, enhancing the protein folding capacity of the endoplasmic reticulum. Activated ATF6 stimulates the expression of UPR-related genes. Normally, the protective UPR and apoptotic signals are in a balanced state in a cell; however, this equilibrium might incline towards the proapoptotic condition as the damage worsens. If the UPR can successfully alleviate ER stress, the cell can restore protein homeostasis and thus survive. If ER stress persists, the UPR signal can activate the apoptotic pathway, leading to cell death.
The induction of inflammatory reactions by ER stress involves the activation of all three branches of the UPR. These ER stress sensors activate diverse inflammatory pathways and trigger the expression of inflammatory mediators. IRE1α-dependent sXBP1 activation during the inflammation of human bronchial epithelia can trigger increased storage of Ca2+ in the ER (Martino et al., 2009), thereby inducing Ca2+-dependent cytokine generation and release (Martino et al., 2009; Ribeiro et al., 2005). In the lung, IRE1α activation is required for the production of cytokines such as IL-8, IL-6, and TNF-α by the inflamed human airway epithelia and human alveolar macrophages (Martino et al., 2009; Hull-Ryde et al., 2021; Lubamba et al., 2015). PERK signaling can activate the signaling of TXNIP, leading to the upregulation of inflammasomes such as NLRP3 (Oslowski et al., 2012). The involvement of NLRP3 activation is crucial in the pathogenesis of several lung diseases, mediating the release of proinflammatory cytokines IL-1β and IL-18, initiating pyroptosis (inflammatory cell death), and playing a key role in the development of inflammaging (Meyers and Zhu, 2020; Liu et al., 2022; Chen et al., 2023). The NF-κB family of transcription factors plays a key role in inflammation and innate immunity, and persistent activation is associated with age-related lung disorders (Korhonen et al., 1997; Zaynagetdinov et al., 2016; Zhang et al., 2013). ER stress serves as the trigger for UPR signaling, intricately linked with the initiation of proinflammatory pathways mediated by NF-κB. The activation of IRE1α recruits TRAF2 and ASK-1, setting off the cascade that leads to the activation of JNK and NF-κB. Simultaneously, PERK plays a pivotal role in influencing NF-κB activation and apoptosis through orchestrating the eIF2α-ATF4-CHOP axis109 (Belali et al., 2022). Furthermore, ATF6 emerges as a positive contributor to NF-κB activation, operating through the mTOR/AKT signaling pathway. This intricate network of interactions emphasizes the complexity of the crosstalk between ER stress, UPR signaling, and the proinflammatory machinery orchestrated by NF-κB (Nakajima et al., 2011) (Figure 3).
Figure 3. The inflammatory signaling pathway regulated by UPR. IRE1α-dependent sXBP1 activation during the inflammation of human bronchial epithelia can trigger the storage of Ca2+ in the ER and subsequently amplify Ca2+-dependent cytokine generation and release. IRE1α activation leads to the recruitment of TRAF2 and ASK-1, which activate JNK and NF-κB, leading to the production of inflammatory cytokines. The IRE1/TRAF2/ASK1 complex activates inhibitory κ B kinase, which induces the phosphorylation of IκB, leading to the translocation of NF-κB into the nucleus and induction of expression of inflammatory cytokine genes. PERK signaling can activate the signaling of TXNIP, leading to the upregulation of inflammasomes such as NLRP3. PERK modulates NF-κB activation and apoptosis by activating the eIF2α-ATF4-CHOP axis. Additionally, the activation of ATF6 can cause transient phosphorylation of AKT, leading to the activation of NF-κB. ATF6 also positively affects NF-κB activation by inhibiting AKT/GSK3β.
Proteostasis is a highly conserved biological process and includes an array of mechanisms governing protein functions to maintain cell and tissue homeostasis (Balch et al., 2014). Aging reduces proteostasis, evidenced by declining control over protein quality independent of occurring diseases (Schneider et al., 2021). Persistent biochemical strain in the lungs leads to the buildup of misfolded proteins, detrimental post-translational alterations, and disturbed protein interactions, fostering the development of lung diseases (Balch et al., 2014). Thus, a robust proteostasis network is crucial for lung function and cellular metabolism.
ER stress and UPR are conserved cellular pathways and are important to maintain cellular proteostasis. Dysfunctions of the proteostasis network, particularly disturbances in ER function, are thought to contribute to abnormal protein aggregation (Wang and Kaufman, 2016; Kaushik and Cuervo, 2015). Aging alters ER chaperone and folding enzyme expression, creating an imbalance with heightened ER stress and diminished UPR, leading to chronic ER stress and eventual proteostasis loss (Brown and Naidoo, 2012). This imbalance is also implicated in aging-related disorders through a reduction in global proteostasis at both lung and whole organism levels, which induces senescence in the lungs (Katzen et al., 2022; Wei et al., 2013).
As stated earlier, ER stress was initially associated with IPF when mutations in surfactant protein C (SPC) secreted by type II AECs were identified, leading to misfolding. Type II AECs are secretory cells, and mutations in SPC can further elevate ER stress in these cells (Nakada et al., 2021). In a bleomycin-induced fibrosis mouse model, Increased ER stress triggers the activation of UPR-related receptors in both the entire lung and pulmonary fibroblasts; this led to the proliferation of fibroblasts and excessive collagen deposition (Baek et al., 2012; Hsu et al., 2017). Inhibiting IRE1α was observed to enhance collagen induced by TGFβ1and fibronectin synthesis by fibroblasts in individuals experiencing IPF, along with production of mucus in murine fibrosis models (Ghavami et al., 2018; Chen et al., 2019). Accumulating evidence suggests that damaged proteins are present in the lungs of patients with COPD; enhanced oxidative stress in the lungs, induced by CS, leads to oxidative damage in various lung macromolecules, including proteins, even among individuals who have quit smoking (MacNee, 2005; Pryor and Stone, 1993). CS-induced oxidative load facilitates the buildup of insoluble, polyubiquitinated proteins, observed both in laboratory settings (in vitro) and living organisms (in vivo) (Kenche et al., 2013; van Rijt et al., 2012). Additionally, the ER-resident foldase PDI experiences oxidation and misfolding due to CS exposure, evident in vivo and in vitro conditions (Kenche et al., 2013). Hence, CS could compromise ER folding capabilities, resulting in an elevated burden of misfolded proteins within the ER.
Future investigations should focus on specific contributions of UPR pathways to proteostasis in various lung cells and diseases associated with aging.
Cellular senescence is characterized by a persistent cessation of the cell cycle, accompanied by distinctive changes in cellular structure and gene expression. These senescent cells exhibit resistance to programmed cell death through antiapoptotic signaling, maintaining metabolic activity and releasing a combination of inflammatory molecules known as the senescence-associated secretory phenotype (SASP) (Aghali et al., 2022). Cellular senescence exerts a protective effect against cellular stress and tumorigenesis, and it is a crucial biological process frequently observed in embryonic development and wound healing (Storer et al., 2013; Jun and Lau, 2010; Collado and Serrano, 2010). Over time, the buildup of senescent cells becomes a contributing factor to the onset of aging-related diseases (Song et al., 2020; Borghesan et al., 2020), and is considered one of the hallmarks of aging (López-Otín et al., 2013; López-Otín et al., 2023).
Senescent cell accumulation is evident in the lung in response to various stimuli. Lung tissues from individuals with COPD and IPF exhibit senescent cell characteristics, including heightened levels of specific markers (p53, p21CIP1, and p16ink4a) and an upregulated antiapoptotic pathway (Aghali et al., 2022). Moreover, lung homogenates from old mice show a significant increase in SASP components and the inflammatory index compared to those in young mice (Shivshankar et al., 2011).
Senescence, viewed as a stress response to halt the cell cycle of altered cells, is strongly linked with ER stress (Pluquet et al., 2015; Druelle et al., 2016). Research showed that ER stress and UPR is involved in the generation of cellular senescence, and all arms of the UPR may be involved in senescence. For example, PERK activation induces eIF2α phosphorylation and thereafter induce the GADD45α expression (Lee et al., 2019), which is involved in cellular senescence. PERK and IRE1 activation is required to generate SASP components (Matos et al., 2015). The inhibition of IRE1 suppressed H2O2-induced senescence of nucleus pulposus cells (Kang et al., 2022). The ATF6α pathway is involved in cell senescence through its target gene product calreticulin (Shoulders et al., 2013). The secretory state of senescent cells, however, triggers an ER stress response. In senescence, ER and secretory pathway organelles become more active to produce SASP components, which are upregulated and oversecreted. During cellular senescence, changes in morphology occur, marked by expanded and vacuolized cell shapes, along with occasional presence of numerous or enlarged nuclei. The association of vacuolation with uncontrolled activation of UPR has been noted (Peng et al., 2011).
Presently, there is limited direct evidence showing the relationship of ER stress response with cellular senescence in the aging lung. In response to bleomycin injury, IRE1-α signaling has been shown to induce senescence in type II alveolar epithelial cells (AECs), leading to the accumulation of pre-alveolar type 1 transitional cells (PATS) and subsequent fibrosis. Blocking IRE1-α signaling alleviates bleomycin-induced lung fibrosis by reducing senescence and promoting the differentiation of PATS cells to AT1 cells (Auyeung et al., 2022). ER stress may promote cellular senescence in the lung. The lung relies on a strong proteostasis network to handle continuous mechanical stress and various environmental challenges, preventing protein misfolding or recycling damaged proteins. Senescent cells in the lung are most likely to experience ER stress because of the increasing demand for protein folding; moreover, the production of SASP components induces a high burden on the secretory pathway of ER, thereby requiring increased maintenance of proteostasis.
Adult stem cells play a crucial role in maintaining tissue balance and regenerating cells. Stem cell exhaustion, a decline in both quantity and quality over time, is proposed as a key aging factor (López-Otín et al., 2013; López-Otín et al., 2023).
The intricate structure of the human lung’s epithelium, vital for its functions, depends on the proper functioning of airway epithelial stem cells for maintenance and repair (Basil et al., 2020). The major stem cells in the conducting airways are basal and secretory club cells (Rawlins et al., 2009; Rock et al., 2009). Type II AECs proliferate in the alveoli and generate type I AECs to restore the alveolar epithelium; thus, these cells are considered alveolar stem/progenitor cells (Barkauskas et al., 2013). During the aging process, the number of basal and club cells decrease (Schneider et al., 2021); additionally, airway basal cells exhibit restricted regenerative potential, unable to fully restore a differentiated epithelium, particularly in pathological conditions like COPD (Staudt et al., 2014). With the progress in age, type II AECs remain intact in number but exhibit a decline in their self-renewal and differentiation capacity (Schneider et al., 2021).
Stress affects stem cells, especially hematopoietic stem cells (HSCs) in the physiological hierarchy. The UPR in HSCs regulates self-renewal, resolving stress or initiating apoptosis (Kharabi Masouleh et al., 2015). UFBP1 deficiency elevates ER stress and UPR activation, causing the death of hematopoietic stem/progenitor cells (van Galen et al., 2014). UFBP1 plays various roles in hematopoietic cell survival and differentiation by modulating ER homeostasis and regulating gene expression specific to the erythroid lineage (Cai et al., 2015). The targeted removal of the vital chaperone GRP78/BiP from type II AECs in the lung led to a decline in the regenerative ability of type II AECs. This deletion also triggered activated TGF-β signaling, contributing to spontaneous age-dependent fibrotic lung remodeling characterized by interstitial pneumonia-like features. An ER stress inhibitor alleviated fibrosis in mice lacking Grp78 in epithelial cells and IPF lung slices, highlighting a potential aging-IPF connection through ER stress (Borok et al., 2020). An in vitro study in type II AECs revealed that CSE could activate ER stress-induced apoptosis by activating JNK and caspase-12 in a dose- and time-dependent manner; however, this activation of the ER stress signaling pathway can also initiate a protective mechanism by inducing HRD1, which is a specific ubiquitin E3 ligase on the ER membrane, to counteract CSE-induced UPR for protecting type II AECs (Tan et al., 2017).
The mitochondrial UPR (UPRmt), a regulatory mechanism for preserving adult stem cells, is conserved across tissues. Dysregulation of this protective mechanism contributes to the functional decline of stem cells and tissue degeneration over time (Mohrin et al., 2018). Alternatively, the constant UPRmt activation, along with tissue-specific HSP60 loss, can detrimentally impact stemness (Berger et al., 2016). In AECs of the lung, by inducing ATF4-dependent UPRmt and mitochondrial dysfunction, ER stress is implicated in the development of diverse pulmonary disorders, and type II AECs may play a role in this process (Jiang et al., 2020).
Mitochondria, essential cellular organelles, produce energy and reactive oxygen species (ROS), influencing physiological functions, while also playing a crucial role in governing inflammatory responses, innate immunity, cell death, and aging (Schafer et al., 2017; Miwa et al., 2022). As the cell ages, its mitochondria accumulate abnormalities, but increased mutations in mtDNA, thereby leading to declined mitochondrial function (Mora et al., 2017). Mitochondrial dysfunction contributes to impaired lung functions; affects the trachea, bronchi, bronchioles, alveoli, and interstitium; hinders lung recovery after an injury (Caldeira et al., 2021).
The ER serves as a central hub establishing physical connections with mitochondria. MAMs are specialized subdomains facilitating close proximity between the ER and mitochondria, fostering intricate cross-talk (Phillips and Voeltz, 2016) (Figure 4). This juxtaposition enhances mitochondrial Ca2+ uptake and improves mitochondrial dynamics and redox balance; it also triggers apoptosis under excessive ER stress condition (van Vliet and Agostinis, 2018). Various mitochondrial and ER stress-associated proteins such as Drp1, Mfn2, and PERK are involved in the structure and functions of MAMs (Mao et al., 2022). Disturbance in the MAM during ER stress is implicated in the aging process and age-related diseases (Moltedo et al., 2019; Volgyi et al., 2015). In an in vitro study using HBE cells, ER stress induced by titanium dioxide nanoparticles mediated autophagic cell death through MAM disruption (Yu et al., 2015). The exposure of human airway smooth muscle (hASM) cells to the proinflammatory cytokine TNF-α triggers ER stress pathways, leading to the disruption of ER–mitochondria interaction, inhibition of Mfn2 expression, and impairment of mitochondrial mobility (Yap et al., 2020; Delmotte et al., 2017).
Figure 4. Mitochondria-associated membrane modulates mitochondrial Ca2+ uptake and improves mitochondrial dynamics and redox balance. Grp75 interacts with transglutaminase type 2 in mitochondria-associated membranes (MAMs) and then stabilizes the interaction between Grp75 and IP3R to enhance ER–mitochondria association. The enhanced ER–mitochondria association promotes the ER-mitochondrial Ca2+ flux. Dynamic-related protein 1 (Drp1) is recruited to the outer mitochondrial membrane by the fission receptor mitochondrial fission factor, which increases ER–mitochondria interactions by promoting the formation of tubules in the ER. Mitofusin 2 (Mfn2) on the ER interacts with Mfn2 on the mitochondria to form homo-complexes. These homo-complexes can tether the ER and mitochondria to enhance MAM formation. PERK is also a crucial component of MAMs. PERK plays a role in establishing a physical and functional association between the ER and mitochondria. The knockout of the PERK gene affects the ER–mitochondria association, thereby disturbing ER morphology, decreasing Ca2+ transfer from the ER to the mitochondria, and reducing the transmission of ROS signaling from the ER to the mitochondria. mtROS-mediated MAM dysfunction is involved in the mechanism of arsenic-induced ferroptosis and ALI.
In aged mice and IPF patients, ATF4 and UPRmt were induced in lung alveolar epithelial cells. Inducing ATF4 in mouse AECs worsened pulmonary UPRmt, inflammation, and death post-bleomycin injury (Jiang et al., 2020). Thus, it can be inferred that the UPRmt generally slows mitochondrial activity for organelle recovery but may raise the risk of declining oxidative phosphorylation, membrane potential loss, and increased ROS production in AECs.
ROS, which are continuously produced in all aerobic organisms, are derived from oxygen metabolism as byproducts of cell respiration (Lenaz, 2012) and include superoxide anions (O2·-), hydroxyl radicals (·OH), and hydrogen peroxide (H2O2). Oxidative stress arises due to an imbalance between the production of ROS and antioxidant mechanisms. The causal relationship among ROS, aging, age-related pathologies, and senescence has been studied intensively based on the free radical theory of aging (Harman, 1956) and the mitochondrial theory of aging (Alexeyev, 2009). A recent study investigated whether ROS is beneficial or detrimental to extend lifespan; this is because the basal level of ROS is essential to regulate biological processes and metabolic homeostasis (Zhang et al., 2019). The hormetic response of ROS is not advantageous for the mammalian lung. Pulmonary disorders linked to oxidative stress involve increased ROS production and decreased antioxidants. Asthma patients, for instance, show elevated ROS in macrophages and diminished lung antioxidants (Comhair and Erzurum, 2010). Exposure to oxidative toxicants in the lungs causes tissue damage, inflammation, and the accumulation of phagocytic leukocytes, further enhancing oxidant production (van der Toorn et al., 2009).
Prior research indicates a close interconnection between ER stress and oxidative stress. These two stressors mutually reinforce each other, playing roles in several chronic conditions associated with aging (Kim et al., 2008; Lee and Lee, 2022). The ER serves as an optimal setting for protein oxidation and folding. Oxygen is essential for the redox coupling between ER oxidoreductase 1 (ERO1) and the final enzyme PDI, enabling the formation of protein disulfide bonds unique to the ER (Cao and Kaufman, 2014). This ER redox system converts oxygen to H2O2, thereby contributing to the total cellular ROS, although the amount of ROS thus generated is very small as compared to that produced in the mitochondria (Tu and Weissman, 2004). During ER stress, ROS production is elevated through NADPH oxidases, mainly through Nox2 and Nox4 (Santos et al., 2014). Moreover, a substantial quantity of activated JNK interacts with the MAM-linking protein Sab, leading to the release of ROS (Win et al., 2014). Global transcriptome studies revealed that ER stress did not alter the expression of UPR-associated genes in aging lungs (Misra et al., 2007); however, the expression of Grp78 was decreased, and the expression of Grp94 and proapoptotic CHOP was increased in type II AECs (Borok et al., 2020). CHOP is involved in excessive ROS production by increased expression of ERO1 (Marciniak et al., 2004). Oxidative stress enhances protein oxidation, disturbs ER protein folding, and leads to the buildup of misfolded oxidized protein substrates. This is exacerbated by the compromised function of the chaperone system and other cellular “quality control” mechanisms due to proteins modified by ROS (Muller et al., 2013). Consequently, the simultaneous occurrence of oxidative stress and ER stress constitutes the initial response to pathological challenges. The inhibition of these cellular stress factors can stop the formation of a vicious loop and thus prevent pathological developments in the lung. Moreover, it possesses the ability to protect against acute lung injury (ALI) induced by sepsis. This protection is achieved by suppressing ER stress and mitochondrial dysfunction mediated by oxidative stress through the activation of the SIRT1/AMPK pathways specifically within the lung (Sang et al., 2022).
Genome integrity is constantly threatened by damage due to exogenous agents and intrinsic biological processes that can induce DNA damage (Lindahl and Barnes, 2000). A common phenomenon in the aging process is the accumulation of genetic damage throughout the life (López-Otín et al., 2013; López-Otín et al., 2023). Accordingly, cells have evolved an intricate and specific DNA damage response (DDR) cascade responsible for the recognition and repair process (Sancar et al., 2004). The DDR cascade mainly involves the phosphoinositide 3-kinase proteins (PI3Ks) (ATM, ATR, and DNA-PK) and poly (ADP-ribose) polymerase (PARP).
DNA damage, which is mostly oxidative in nature, plays a key role in the development of aging-related chronic diseases. In asthma, exposure to environmental allergens induces oxidative DNA damage in airway epithelial cells, with the repair of this damage linked to Th2 cytokine secretion and the initiation of allergic inflammation (Zahiruddin et al., 2018).
UPR and DDR are the two important mechanisms to maintain cell homeostasis. Although these adaptive mechanisms occur in the ER and nucleus, respectively, they are involved in the mechanisms and signaling pathways that modulate genome integrity. The DDR-related protein ATM might be associated with the overlap between the DDR and ER. As discussed earlier, oxidative stress is a natural biological process that occurs in all cells and can also occur in aging and aging-related lung disorders. The mutated versions of ATM exhibit resistance to oxidative stress and a minimal impact on the DNA damage response (DDR). Yet, they assist in the removal of toxic protein buildup (Lee et al., 2018). Thus, ATM may not only act as an oxidative stress sensor but can also sense alterations in other cellular compartments, including the ER (Hotokezaka et al., 2020; He et al., 2009). The nonhomologous DNA end-joining double-strand break (DSB) repair pathway in Saccharomyces cerevisiae is influenced by the exogenous expression of mammalian XBP1, which regulates H4 acetylation (Tao et al., 2011). Additionally, sXBP1, under ER stress, controls the transcription of a cluster of DNA repair genes (Acosta-Alvear et al., 2007). SRC is a protein tyrosine kinase and can be activated by the UPR; following its activation, it creates a complex with IRE1α, leading to the relocation of ER chaperones to the cell surface (Tsai et al., 2018). SRC is also involved in the regulation of the DDR by terminating the ATR-Chk1-dependent G2 DNA damage checkpoint (Fukumoto et al., 2014).
To date, few studies have provided evidence of an association between DNA damage and ER stress in the lungs. The obtained results indicate that the DDR and UPR can direct a coordinated response to manage cellular stress, which may be relevant in aging and lung diseases. Additional experimental evidence is needed to understand the interaction between this UPR branch and the cellular machinery involved in genomic stability, particularly pertaining to aging and related lung diseases.
Autophagy is a conserved multistep catabolic process that degrades unfolded proteins, cytoplasmic contents, and damaged organelles by forming autophagosomes. It is currently viewed as an association between metabolic and proteostatic signaling that can determine key physiological decisions ranging from cell fate to organismal lifespan (Kaushik et al., 2021).
ER stress and autophagy are two closely interconnected response pathways to cellular stress (Senft and Ronai, 2015; Bhardwaj et al., 2020). In eukaryotic cells, ER stress can activate lysosomes through the p38 MAPK pathway, thereby activating Chaperone-Mediated Autophagy (CMA) (Li et al., 2017). Autophagy degrades unfolded proteins when the canonical, proteasome-dependent pathway is impaired or overwhelmed by an excessive amount of ubiquitinated proteins. Therefore, autophagy is considered the last approach to restore the homeostasis of the ER. Autophagy can be triggered following UPR-inducing stimuli (Nijholt et al., 2011) and serves as a pro-survival mechanism under ER stress conditions (Figure 5). However, ER stress can also disrupt lysosomal homeostasis, leading to an obstruction of autophagic flux. For instance, the level of serum lysosomal-associated membrane protein 1 (LAMP1) was significantly decreased in pre-eclampsia patients compared to normal pregnant women, suggesting potential lysosomal dysfunction due to ER stress in pre-eclamptic placentas (Nakashima et al., 2019).
Figure 5. ER stress-mediated autophagy and the possible mechanisms. ER stress can induce autophagy through the IRE1α, PERK, and ATF6 signaling pathways. IRE1 forms a complex with TRAF-2, which subsequently binds to ASK1, leading to the phosphorylation of JNK and the promotion of Bcl-2 activation, thereby activating autophagy. sXBP1 also triggers transcriptional activation of Beclin-1. The activated PERK induces autophagy through ATF4-driven transcriptional regulation of Atg12, whereas ATF4-mediated CHOP activation transcriptionally induces Atg5 expression. Atg5, Atg12, and Atg16L form the Atg5-Atg12-Atg16L complex, which is involved in the elongation process. ATF6 indirectly regulates autophagy through CHOP; it upregulates the expression of DAPK1, which phosphorylates Beclin-1 and mediates autophagy initiation. ER-phagy is initiated to remove damaged ER organelles. ER-phagy receptors on the ER membrane mediate the interaction between LC3 and its target ER sites through the LC3-interacting region (LIR).
Beyond autophagy, the interplay between the ER and lysosomes manifests in various other ways. Research has demonstrated that SNX19 restricts endolysosomal motility via its interaction with the endoplasmic reticulum, a process crucial for the concentration of endolysosomes in the perinuclear region (Saric et al., 2021). These interactions not only contribute to the stability of the intracellular environment but also play a pivotal role in a range of pathological conditions, including neurodegenerative diseases, metabolic disorders, and cancer.
ER stress and UPR pathways share mechanisms that control autophagy, collectively influencing responses to diverse stimuli. This interplay is implicated in the development of lung diseases. For example, during respiratory viral infection, the deficiency of the autophagy protein Map1-LC3b can increase ER stress and associated IL-1 secretion and lead to IL-17-dependent lung pathology (Reed et al., 2015). CS induces the expression of lysosome-associated membrane protein 2A (LAMP2A) and chaperone-mediated autophagy (CMA) in an Nrf2-dependent manner in HBE cells. The suppression of LAMP2A and CMA inhibition enhances UPR and apoptosis; this effect can be reversed by the induction of LAMP2A expression (Hosaka et al., 2020). Thus, careful manipulation of autophagy in the context of UPR may enable to develop new therapeutic strategies for aging-related lung diseases.
Sirtuins, the family of NAD + -dependent protein deacetylases, have been studied extensively as a potential antiaging factor (Bonkowski and Sinclair, 2016). Decreased sirtuin activity, leading to elevated histone acetylation, causes aging phenotype in certain organisms (Wątroba et al., 2017).
Initially recognized for their deacetylase function, sirtuins are now recognized for their involvement in various biological activities and are a focal point in research on ER stress and the UPR. Sirtuins deacetylate sXBP1, potentially preventing ER stress-mediated release of proinflammatory cytokines and apoptosis (Wang et al., 2011). XBP1 induces SIRT7 expression, reducing ER stress-related protein expression (Shin et al., 2013). SIRT1 plays a crucial role in protecting against ER stress-related lung damage, including in sepsis-induced lung injury and hyperoxic ALI (Wang et al., 2019; Sun et al., 2017). Lower serum SIRT1 levels are observed in COPD patients, while SIRT1 upregulation protects against COPD by reducing apoptosis and ER stress (He et al., 2019). SIRT1 elevation promotes autophagy, but using SIRT1 inhibitors reverses this effect, worsening COPD by intensifying ER stress (Tang and Ling, 2019).
Given the significance of ER stress and the UPR signaling pathway in the process of aging and the pathogenesis of pulmonary diseases, strategies to target these two factors are crucial for the intervention of lung diseases. As an alternative strategy, the activation of the adaptive UPR or the suppression of the maladaptive UPR by using appropriate compounds has considerable potential.
Metformin, a pharmaceutical agent commonly prescribed for the management of type 2 diabetes mellitus, demonstrates additional therapeutic benefits beyond glycemic control. In mouse lungs, it mitigates cigarette smoke-induced emphysematous COPD pathologies through the AMPK pathway, concurrently suppressing the unfolded protein response (UPR) and endoplasmic reticulum (ER) stress (Polverino et al., 2021).
Melatonin, a neuroendocrine hormone primarily produced in the pineal gland, serves various biological roles, including sleep modulation, circadian rhythm regulation, immune system enhancement, antioxidant properties, antiaging influence, and antitumor activity (Luo et al., 2020). Additionally, it can impede the initiation of NLRP3 inflammation and ER stress in COPD (Mahalanobish et al., 2020).
Resveratrol is a natural phenolic compound found in many foods. Many studies have highlighted its role in longevity and prevention of aging-related disorders (Harikumar and Aggarwal, 2008). Resveratrol diminishes inflammation and apoptosis by easing ER stress via the Akt/mTOR pathway in cases of allergic airway inflammation induced by fungi. This provides potential therapeutic approaches for lung diseases triggered by allergic reactions to fungi (Wang S. et al., 2022).
SIRT1 attenuates ER stress and apoptosis in the rat models of COPD and septic associated-lung injury (Zhang L. et al., 2020; Wang F. et al., 2022). Interestingly, melatonin protects against COPD through the attenuation of apoptosis and ER stress by upregulating SIRT1 expression in rats (He et al., 2019).
While compounds directed at ER stress or UPR elements exhibit promise in averting and treating age-related lung diseases, clinical trials are necessary to comprehensively assess their impact on ER stress and UPR (Figure 6).
Figure 6. The roles of metformin, melatonin and resveratrol in ER stress and pulmonary dysfunction. Metformin mitigates cigarette smoke-induced emphysematous COPD pathologies through the AMPK pathway, concurrently suppressing the unfolded protein response (UPR) and endoplasmic reticulum (ER) stress. Melatonin protects against COPD through the attenuation of apoptosis and ER stress by upregulating SIRT1 expression in rats. Additionally, it can impede the initiation of NLRP3 inflammation and ER stress in COPD. Resveratrol diminishes inflammation and apoptosis by easing ER stress via the Akt/mTOR pathway in cases of allergic airway inflammation induced by fungi.
Regular exercise is known to promote healthy aging and mitigate aging-related diseases (Booth et al., 2012; Viña et al., 2016). Exercise also shows protective effects against COPD (Rochester et al., 2015), IPF, and COVID-19 (Dwyer et al., 2020; Yu et al., 2019). The mechanisms underlying the effects of exercise on longevity are yet to be fully clarified; however, regular exercise can reverse ER dysfunctions (Estébanez et al., 2018).
In obese individuals, physical exercise alleviates ER stress by reducing the expression and release of the GRP78 chaperone (Khadir et al., 2016). Exercise influences the adaptive Unfolded Protein Response (UPR) for the purpose of muscle remodeling, as evidenced by the analysis of biopsy samples from human skeletal muscle. Nevertheless, this capability is compromised with the progression of aging (Khadir et al., 2016).
Based on the findings and hypotheses presented in this review, ER homeostasis, ER stress, and UPR have been extensively implicated in aging and aging-related lung disorders. Regulating ER stress shows potential as a strategy to mitigate age-related health decline and avert lung disorders.
Aging itself is, however, a highly complicated process, and ER stress has a complex role in the biology of aging. Investigations on the role of ER stress in aging and aging-related lung diseases thus remain a challenge. The bulk of research on ER stress relies on simplistic models or undifferentiated cells, potentially limiting its applicability to understanding the pathophysiology of well-differentiated pulmonary cell types, especially in the context of aging. The efficacy of manipulating ER stress could be contingent on its collective influence on each of these cellular categories. Therefore, more detailed mechanistic studies are required on the interaction between ER stress and disease progression in the aging lung and on the modulation of this association for clinical translation. Nonetheless, the investigations reviewed here provide substantial insights into the role of ER stress and UPR in aging-related lung diseases, and these insights could prove to be valuable for developing effective therapeutic prevention and intervention strategies.
ZF: Conceptualization, Investigation, Writing–review and editing. WW: Conceptualization, Writing–original draft. YG: Writing–original draft.
The author(s) declare that no financial support was received for the research, authorship, and/or publication of this article.
The authors declare that the research was conducted in the absence of any commercial or financial relationships that could be construed as a potential conflict of interest.
All claims expressed in this article are solely those of the authors and do not necessarily represent those of their affiliated organizations, or those of the publisher, the editors and the reviewers. Any product that may be evaluated in this article, or claim that may be made by its manufacturer, is not guaranteed or endorsed by the publisher.
Abadie, Y., Bregeon, F., Papazian, L., Lange, F., Chailley-Heu, B., Thomas, P., et al. (2005). Decreased VEGF concentration in lung tissue and vascular injury during ARDS. Eur. Respir. J. 25, 139–146. doi:10.1183/09031936.04.00065504
Abbasi, J. (2017). Can a diet that mimics fasting turn back the clock? Jama 318, 227–229. doi:10.1001/jama.2017.6648
Acosta-Alvear, D., Zhou, Y., Blais, A., Tsikitis, M., Lents, N. H., Arias, C., et al. (2007). XBP1 controls diverse cell type- and condition-specific transcriptional regulatory networks. Mol. Cell 27, 53–66. doi:10.1016/j.molcel.2007.06.011
Aghali, A., Koloko Ngassie, M. L., Pabelick, C. M., and Prakash, Y. S. (2022). Cellular senescence in aging lungs and diseases. Cells 11, 1781. doi:10.3390/cells11111781
Akhter, M. S., Uddin, M. A., Kubra, K. T., and Barabutis, N. (2020). Autophagy, unfolded protein response and lung disease. Curr. Res. Cell Biol. 1, 100003. doi:10.1016/j.crcbio.2020.100003
Aksoy, M. O., Kim, V., Cornwell, W. D., Rogers, T. J., Kosmider, B., Bahmed, K., et al. (2017). Secretion of the endoplasmic reticulum stress protein, GRP78, into the BALF is increased in cigarette smokers. Respir. Res. 18, 78. doi:10.1186/s12931-017-0561-6
Alexeyev, M. F. (2009). Is there more to aging than mitochondrial DNA and reactive oxygen species? Febs J. 276, 5768–5787. doi:10.1111/j.1742-4658.2009.07269.x
Auyeung, V. C., Downey, M. S., Thamsen, M., Wenger, T. A., Backes, B. J., Sheppard, D., et al. (2022). IRE1α drives lung epithelial progenitor dysfunction to establish a niche for pulmonary fibrosis. Am. J. Physiol. Lung Cell Mol. Physiol. 322, L564–l580. doi:10.1152/ajplung.00408.2021
Baek, H. A., Kim, D. S., Park, H. S., Jang, K. Y., Kang, M. J., Lee, D. G., et al. (2012). Involvement of endoplasmic reticulum stress in myofibroblastic differentiation of lung fibroblasts. Am. J. Respir. Cell Mol. Biol. 46, 731–739. doi:10.1165/rcmb.2011-0121OC
Balch, W. E., Sznajder, J. I., Budinger, S., Finley, D., Laposky, A. D., Cuervo, A. M., et al. (2014). Malfolded protein structure and proteostasis in lung diseases. Am. J. Respir. Crit. Care Med. 189, 96–103. doi:10.1164/rccm.201306-1164WS
Barkauskas, C. E., Cronce, M. J., Rackley, C. R., Bowie, E. J., Keene, D. R., Stripp, B. R., et al. (2013). Type 2 alveolar cells are stem cells in adult lung. J. Clin. Invest 123, 3025–3036. doi:10.1172/JCI68782
Barnes, P. J. (2017). Senescence in COPD and its comorbidities. Annu. Rev. Physiol. 79, 517–539. doi:10.1146/annurev-physiol-022516-034314
Basil, M. C., Katzen, J., Engler, A. E., Guo, M., Herriges, M. J., Kathiriya, J. J., et al. (2020). The cellular and physiological basis for lung repair and regeneration: past, present, and future. Cell Stem Cell 26, 482–502. doi:10.1016/j.stem.2020.03.009
Belali, O. M., Ahmed, M. M., Mohany, M., Belali, T. M., Alotaibi, M. M., Al-Hoshani, A., et al. (2022). LCZ696 protects against diabetic cardiomyopathy-induced myocardial inflammation, ER stress, and apoptosis through inhibiting AGEs/NF-κB and PERK/CHOP signaling pathways. Int. J. Mol. Sci. 23, 1288. doi:10.3390/ijms23031288
Berger, E., Rath, E., Yuan, D., Waldschmitt, N., Khaloian, S., Allgäuer, M., et al. (2016). Mitochondrial function controls intestinal epithelial stemness and proliferation. Nat. Commun. 7, 13171. doi:10.1038/ncomms13171
Bhardwaj, M., Leli, N. M., Koumenis, C., and Amaravadi, R. K. (2020). Regulation of autophagy by canonical and non-canonical ER stress responses. Semin. Cancer Biol. 66, 116–128. doi:10.1016/j.semcancer.2019.11.007
Bonkowski, M. S., and Sinclair, D. A. (2016). Slowing ageing by design: the rise of NAD(+) and sirtuin-activating compounds. Nat. Rev. Mol. Cell Biol. 17, 679–690. doi:10.1038/nrm.2016.93
Booth, F. W., Roberts, C. K., and Laye, M. J. (2012). Lack of exercise is a major cause of chronic diseases. Compr. Physiol. 2, 1143–1211. doi:10.1002/cphy.c110025
Borghesan, M., Hoogaars, W. M. H., Varela-Eirin, M., Talma, N., and Demaria, M. (2020). A senescence-centric view of aging: implications for longevity and disease. Trends Cell Biol. 30, 777–791. doi:10.1016/j.tcb.2020.07.002
Borok, Z., Horie, M., Flodby, P., Wang, H., Liu, Y., Ganesh, S., et al. (2020). Grp78 loss in epithelial progenitors reveals an age-linked role for endoplasmic reticulum stress in pulmonary fibrosis. Am. J. Respir. Crit. Care Med. 201, 198–211. doi:10.1164/rccm.201902-0451OC
Boulet, L. P. (2016). Asthma in the elderly patient. Asthma Res. Pract. 2, 3. doi:10.1186/s40733-015-0015-1
Bowdish, D. M. E. (2019). The aging lung: is lung health good health for older adults? Chest 155, 391–400. doi:10.1016/j.chest.2018.09.003
Breslow, D. K., Collins, S. R., Bodenmiller, B., Aebersold, R., Simons, K., Shevchenko, A., et al. (2010). Orm family proteins mediate sphingolipid homeostasis. Nature 463, 1048–1053. doi:10.1038/nature08787
Brown, M. K., Chan, M. T., Zimmerman, J. E., Pack, A. I., Jackson, N. E., and Naidoo, N. (2014). Aging induced endoplasmic reticulum stress alters sleep and sleep homeostasis. Neurobiol. Aging 35, 1431–1441. doi:10.1016/j.neurobiolaging.2013.12.005
Brown, M. K., and Naidoo, N. (2012). The endoplasmic reticulum stress response in aging and age-related diseases. Front. Physiol. 3, 263. doi:10.3389/fphys.2012.00263
Burman, A., Tanjore, H., and Blackwell, T. S. (2018). Endoplasmic reticulum stress in pulmonary fibrosis. Matrix Biol. 68-69, 355–365. doi:10.1016/j.matbio.2018.03.015
Burrows, B., Barbee, R. A., Cline, M. G., Knudson, R. J., and Lebowitz, M. D. (1991). Characteristics of asthma among elderly adults in a sample of the general population. Chest 100, 935–942. doi:10.1378/chest.100.4.935
Cai, Y., Pi, W., Sivaprakasam, S., Zhu, X., Zhang, M., Chen, J., et al. (2015). UFBP1, a key component of the Ufm1 conjugation system, is essential for ufmylation-mediated regulation of erythroid development. PLoS Genet. 11, e1005643. doi:10.1371/journal.pgen.1005643
Calabrese, E. J. (2008). Hormesis: why it is important to toxicology and toxicologists. Environ. Toxicol. Chem. 27, 1451–1474. doi:10.1897/07-541
Caldeira, D. A. F., Weiss, D. J., Rocco, P. R. M., Silva, P. L., and Cruz, F. F. (2021). Mitochondria in focus: from function to therapeutic strategies in chronic lung diseases. Front. Immunol. 12, 782074. doi:10.3389/fimmu.2021.782074
Calfon, M., Zeng, H., Urano, F., Till, J. H., Hubbard, S. R., Harding, H. P., et al. (2002). IRE1 couples endoplasmic reticulum load to secretory capacity by processing the XBP-1 mRNA. Nature 415, 92–96. doi:10.1038/415092a
Caniard, A., Ballweg, K., Lukas, C., Yildirim, A., Eickelberg, O., and Meiners, S. (2015). Proteasome function is not impaired in healthy aging of the lung. Aging (Albany NY) 7, 776–792. doi:10.18632/aging.100820
Cao, S. S., and Kaufman, R. J. (2014). Endoplasmic reticulum stress and oxidative stress in cell fate decision and human disease. Antioxid. Redox Signal 21, 396–413. doi:10.1089/ars.2014.5851
Chen, G., Ribeiro, C. M. P., Sun, L., Okuda, K., Kato, T., Gilmore, R. C., et al. (2019). XBP1S regulates MUC5B in a promoter variant-dependent pathway in idiopathic pulmonary fibrosis airway epithelia. Am. J. Respir. Crit. Care Med. 200, 220–234. doi:10.1164/rccm.201810-1972oc
Chen, X., Shen, J., and Prywes, R. (2002). The luminal domain of ATF6 senses endoplasmic reticulum (ER) stress and causes translocation of ATF6 from the ER to the Golgi. J. Biol. Chem. 277, 13045–13052. doi:10.1074/jbc.M110636200
Chen, Y., Klein, S. L., Garibaldi, B. T., Li, H., Wu, C., Osevala, N. M., et al. (2021). Aging in COVID-19: vulnerability, immunity and intervention. Ageing Res. Rev. 65, 101205. doi:10.1016/j.arr.2020.101205
Chen, Y., Zhang, Y., Li, N., Jiang, Z., and Li, X. (2023). Role of mitochondrial stress and the NLRP3 inflammasome in lung diseases. Inflamm. Res. 72, 829–846. doi:10.1007/s00011-023-01712-4
Collado, M., and Serrano, M. (2010). Senescence in tumours: evidence from mice and humans. Nat. Rev. Cancer 10, 51–57. doi:10.1038/nrc2772
Comhair, S. A., and Erzurum, S. C. (2010). Redox control of asthma: molecular mechanisms and therapeutic opportunities. Antioxid. Redox Signal 12, 93–124. doi:10.1089/ars.2008.2425
Confalonieri, M., Salton, F., and Fabiano, F. (2017). Acute respiratory distress syndrome. Eur. Respir. Rev. 26, 160116. doi:10.1183/16000617.0116-2016
Dastghaib, S., Kumar, P. S., Aftabi, S., Damera, G., Dalvand, A., Sepanjnia, A., et al. (2021). Mechanisms targeting the unfolded protein response in asthma. Am. J. Respir. Cell Mol. Biol. 64, 29–38. doi:10.1165/rcmb.2019-0235TR
de Cabo, R., and Mattson, M. P. (2019). Effects of intermittent fasting on health, aging, and disease. N. Engl. J. Med. 381, 2541–2551. doi:10.1056/nejmra1905136
Delmotte, P., Zavaletta, V. A., Thompson, M. A., Prakash, Y. S., and Sieck, G. C. (2017). TNFα decreases mitochondrial movement in human airway smooth muscle. Am. J. Physiol. Lung Cell Mol. Physiol. 313, L166–l176. doi:10.1152/ajplung.00538.2016
Druelle, C., Drullion, C., Deslé, J., Martin, N., Saas, L., Cormenier, J., et al. (2016). ATF6α regulates morphological changes associated with senescence in human fibroblasts. Oncotarget 7, 67699–67715. doi:10.18632/oncotarget.11505
Dwyer, M. J., Pasini, M., De Dominicis, S., and Righi, E. (2020). Physical activity: benefits and challenges during the COVID-19 pandemic. Scand. J. Med. Sci. Sports 30, 1291–1294. doi:10.1111/sms.13710
Ebstein, F., Poli Harlowe, M. C., Studencka-Turski, M., and Krüger, E. (2019). Contribution of the unfolded protein response (UPR) to the pathogenesis of proteasome-associated autoinflammatory syndromes (PRAAS). Front. Immunol. 10, 2756. doi:10.3389/fimmu.2019.02756
Erickson, R. R., Dunning, L. M., and Holtzman, J. L. (2006). The effect of aging on the chaperone concentrations in the hepatic, endoplasmic reticulum of male rats: the possible role of protein misfolding due to the loss of chaperones in the decline in physiological function seen with age. J. Gerontol. A Biol. Sci. Med. Sci. 61, 435–443. doi:10.1093/gerona/61.5.435
Estébanez, B., de Paz, J. A., Cuevas, M. J., and González-Gallego, J. (2018). Endoplasmic reticulum unfolded protein response, aging and exercise: an update. Front. Physiol. 9, 1744. doi:10.3389/fphys.2018.01744
Faniyi, A. A., Hughes, M. J., Scott, A., Belchamber, K. B. R., and Sapey, E. (2022). Inflammation, ageing and diseases of the lung: potential therapeutic strategies from shared biological pathways. Br. J. Pharmacol. 179, 1790–1807. doi:10.1111/bph.15759
Ferrucci, L., and Fabbri, E. (2018). Inflammageing: chronic inflammation in ageing, cardiovascular disease, and frailty. Nat. Rev. Cardiol. 15, 505–522. doi:10.1038/s41569-018-0064-2
Franceschi, C., and Campisi, J. (2014). Chronic inflammation (inflammaging) and its potential contribution to age-associated diseases. J. Gerontol. A Biol. Sci. Med. Sci. 69 (Suppl. 1), S4–S9. doi:10.1093/gerona/glu057
Franceschi, C., Garagnani, P., Vitale, G., Capri, M., and Salvioli, S. (2017). Inflammaging and 'garb-aging. Trends Endocrinol. Metab. 28, 199–212. doi:10.1016/j.tem.2016.09.005
Fukumoto, Y., Morii, M., Miura, T., Kubota, S., Ishibashi, K., Honda, T., et al. (2014). Src family kinases promote silencing of ATR-Chk1 signaling in termination of DNA damage checkpoint. J. Biol. Chem. 289, 12313–12329. doi:10.1074/jbc.M113.533752
Ghavami, S., Yeganeh, B., Zeki, A. A., Shojaei, S., Kenyon, N. J., Ott, S., et al. (2018). Autophagy and the unfolded protein response promote profibrotic effects of TGF-β1 in human lung fibroblasts. Am. J. Physiol. Lung Cell Mol. Physiol. 314, L493–l504. doi:10.1152/ajplung.00372.2017
Gillman, A., and Douglass, J. A. (2012). Asthma in the elderly. Asia Pac Allergy 2, 101–108. doi:10.5415/apallergy.2012.2.2.101
Grey, M. J., Cloots, E., Simpson, M. S., LeDuc, N., Serebrenik, Y. V., De Luca, H., et al. (2020). IRE1β negatively regulates IRE1α signaling in response to endoplasmic reticulum stress. J. Cell Biol. 219, e201904048. doi:10.1083/jcb.201904048
Gross, P. A., Hermogenes, A. W., Sacks, H. S., Lau, J., and Levandowski, R. A. (1995). The efficacy of influenza vaccine in elderly persons. A meta-analysis and review of the literature. Ann. Intern Med. 123, 518–527. doi:10.7326/0003-4819-123-7-199510010-00008
Han, J., Back, S. H., Hur, J., Lin, Y. H., Gildersleeve, R., Shan, J., et al. (2013). ER-stress-induced transcriptional regulation increases protein synthesis leading to cell death. Nat. Cell Biol. 15, 481–490. doi:10.1038/ncb2738
Harikumar, K. B., and Aggarwal, B. B. (2008). Resveratrol: a multitargeted agent for age-associated chronic diseases. Cell Cycle 7, 1020–1035. doi:10.4161/cc.7.8.5740
Harman, D. (1956). Aging: a theory based on free radical and radiation chemistry. J. Gerontol. 11, 298–300. doi:10.1093/geronj/11.3.298
He, B., Zhang, W., Qiao, J., Peng, Z., and Chai, X. (2019). Melatonin protects against COPD by attenuating apoptosis and endoplasmic reticulum stress via upregulating SIRT1 expression in rats. Can. J. Physiol. Pharmacol. 97, 386–391. doi:10.1139/cjpp-2018-0529
He, L., Kim, S. O., Kwon, O., Jeong, S. J., Kim, M. S., Lee, H. G., et al. (2009). ATM blocks tunicamycin-induced endoplasmic reticulum stress. FEBS Lett. 583, 903–908. doi:10.1016/j.febslet.2009.02.002
He, Y., Sun, S., Sha, H., Liu, Z., Yang, L., Xue, Z., et al. (2010). Emerging roles for XBP1, a sUPeR transcription factor. Gene Expr. 15, 13–25. doi:10.3727/105221610x12819686555051
Hetz, C., and Papa, F. R. (2018). The unfolded protein response and cell fate control. Mol. Cell 69, 169–181. doi:10.1016/j.molcel.2017.06.017
Hetz, C., Zhang, K., and Kaufman, R. J. (2020). Mechanisms, regulation and functions of the unfolded protein response. Nat. Rev. Mol. Cell Biol. 21, 421–438. doi:10.1038/s41580-020-0250-z
Hosaka, Y., Araya, J., Fujita, Y., Kadota, T., Tsubouchi, K., Yoshida, M., et al. (2020). Chaperone-mediated autophagy suppresses apoptosis via regulation of the unfolded protein response during chronic obstructive pulmonary disease pathogenesis. J. Immunol. 205, 1256–1267. doi:10.4049/jimmunol.2000132
Hotokezaka, Y., Katayama, I., and Nakamura, T. (2020). ATM-associated signalling triggers the unfolded protein response and cell death in response to stress. Commun. Biol. 3, 378. doi:10.1038/s42003-020-1102-2
Hsu, H. S., Liu, C. C., Lin, J. H., Hsu, T. W., Hsu, J. W., Su, K., et al. (2017). Involvement of ER stress, PI3K/AKT activation, and lung fibroblast proliferation in bleomycin-induced pulmonary fibrosis. Sci. Rep. 7, 14272. doi:10.1038/s41598-017-14612-5
Hull-Ryde, E. A., Minges, J. T., Martino, M. E. B., Kato, T., Norris-Drouin, J. L., and Ribeiro, C. M. P. (2021). IRE1α is a therapeutic target for cystic fibrosis airway inflammation. Int. J. Mol. Sci. 22, 3063. doi:10.3390/ijms22063063
Hussain, S. G., and Ramaiah, K. V. (2007). Reduced eIF2alpha phosphorylation and increased proapoptotic proteins in aging. Biochem. Biophys. Res. Commun. 355, 365–370. doi:10.1016/j.bbrc.2007.01.156
Ito, K., and Barnes, P. J. (2009). COPD as a disease of accelerated lung aging. Chest 135, 173–180. doi:10.1378/chest.08-1419
James, B., Milstien, S., and Spiegel, S. (2019). ORMDL3 and allergic asthma: from physiology to pathology. J. Allergy Clin. Immunol. 144, 634–640. doi:10.1016/j.jaci.2019.07.023
Janssens, S., Pulendran, B., and Lambrecht, B. N. (2014). Emerging functions of the unfolded protein response in immunity. Nat. Immunol. 15, 910–919. doi:10.1038/ni.2991
Jiang, D., Cui, H., Xie, N., Banerjee, S., Liu, R. M., Dai, H., et al. (2020). ATF4 mediates mitochondrial unfolded protein response in alveolar epithelial cells. Am. J. Respir. Cell Mol. Biol. 63, 478–489. doi:10.1165/rcmb.2020-0107OC
Jun, J. I., and Lau, L. F. (2010). The matricellular protein CCN1 induces fibroblast senescence and restricts fibrosis in cutaneous wound healing. Nat. Cell Biol. 12, 676–685. doi:10.1038/ncb2070
Kang, H., Dong, Y., Peng, R., Liu, H., Guo, Q., Song, K., et al. (2022). Inhibition of IRE1 suppresses the catabolic effect of IL-1β on nucleus pulposus cell and prevents intervertebral disc degeneration in vivo. Biochem. Pharmacol. 197, 114932. doi:10.1016/j.bcp.2022.114932
Karagöz, G. E., Acosta-Alvear, D., Nguyen, H. T., Lee, C. P., Chu, F., and Walter, P. (2017). An unfolded protein-induced conformational switch activates mammalian IRE1. Elife 6, e30700. doi:10.7554/eLife.30700
Katzen, J., Rodriguez, L., Tomer, Y., Babu, A., Zhao, M., Murthy, A., et al. (2022). Disruption of proteostasis causes IRE1 mediated reprogramming of alveolar epithelial cells. Proc. Natl. Acad. Sci. U. S. A. 119, e2123187119. doi:10.1073/pnas.2123187119
Kaushik, S., and Cuervo, A. M. (2015). Proteostasis and aging. Nat. Med. 21, 1406–1415. doi:10.1038/nm.4001
Kaushik, S., Tasset, I., Arias, E., Pampliega, O., Wong, E., Martinez-Vicente, M., et al. (2021). Autophagy and the hallmarks of aging. Ageing Res. Rev. 72, 101468. doi:10.1016/j.arr.2021.101468
Keller, J. N., Hanni, K. B., and Markesbery, W. R. (2000). Possible involvement of proteasome inhibition in aging: implications for oxidative stress. Mech. Ageing Dev. 113, 61–70. doi:10.1016/s0047-6374(99)00101-3
Kenche, H., Baty, C. J., Vedagiri, K., Shapiro, S. D., and Blumental-Perry, A. (2013). Cigarette smoking affects oxidative protein folding in endoplasmic reticulum by modifying protein disulfide isomerase. Faseb J. 27, 965–977. doi:10.1096/fj.12-216234
Khadir, A., Kavalakatt, S., Abubaker, J., Cherian, P., Madhu, D., Al-Khairi, I., et al. (2016). Physical exercise alleviates ER stress in obese humans through reduction in the expression and release of GRP78 chaperone. Metabolism 65, 1409–1420. doi:10.1016/j.metabol.2016.06.004
Kharabi Masouleh, B., Chevet, E., Panse, J., Jost, E., O'Dwyer, M., Bruemmendorf, T. H., et al. (2015). Drugging the unfolded protein response in acute leukemias. J. Hematol. Oncol. 8, 87. doi:10.1186/s13045-015-0184-7
Kim, H. J., Jeong, J. S., Kim, S. R., Park, S. Y., Chae, H. J., and Lee, Y. C. (2013b). Inhibition of endoplasmic reticulum stress alleviates lipopolysaccharide-induced lung inflammation through modulation of NF-κB/HIF-1α signaling pathway. Sci. Rep. 3, 1142. doi:10.1038/srep01142
Kim, I., Xu, W., and Reed, J. C. (2008). Cell death and endoplasmic reticulum stress: disease relevance and therapeutic opportunities. Nat. Rev. Drug Discov. 7, 1013–1030. doi:10.1038/nrd2755
Kim, S. R., Kim, D. I., Kang, M. R., Lee, K. S., Park, S. Y., Jeong, J. S., et al. (2013a). Endoplasmic reticulum stress influences bronchial asthma pathogenesis by modulating nuclear factor κB activation. J. Allergy Clin. Immunol. 132, 1397–1408. doi:10.1016/j.jaci.2013.08.041
Kim, S. R., Kim, H. J., Kim, D. I., Lee, K. B., Park, H. J., Jeong, J. S., et al. (2015). Blockade of interplay between IL-17a and endoplasmic reticulum stress attenuates LPS-induced lung injury. Theranostics 5, 1343–1362. doi:10.7150/thno.11685
Kim, S. R., and Lee, Y. C. (2015). Endoplasmic reticulum stress and the related signaling networks in severe asthma. Allergy Asthma Immunol. Res. 7, 106–117. doi:10.4168/aair.2015.7.2.106
Korfei, M., Ruppert, C., Mahavadi, P., Henneke, I., Markart, P., Koch, M., et al. (2008). Epithelial endoplasmic reticulum stress and apoptosis in sporadic idiopathic pulmonary fibrosis. Am. J. Respir. Crit. Care Med. 178, 838–846. doi:10.1164/rccm.200802-313OC
Korhonen, P., Helenius, M., and Salminen, A. (1997). Age-related changes in the regulation of transcription factor NF-kappa B in rat brain. Neurosci. Lett. 225, 61–64. doi:10.1016/s0304-3940(97)00190-0
Kotton, D. N., and Morrisey, E. E. (2014). Lung regeneration: mechanisms, applications and emerging stem cell populations. Nat. Med. 20, 822–832. doi:10.1038/nm.3642
Lalley, P. M. (2013). The aging respiratory system--pulmonary structure, function and neural control. Respir. Physiol. Neurobiol. 187, 199–210. doi:10.1016/j.resp.2013.03.012
Lawson, W. E., Crossno, P. F., Polosukhin, V. V., Roldan, J., Cheng, D. S., Lane, K. B., et al. (2008). Endoplasmic reticulum stress in alveolar epithelial cells is prominent in IPF: association with altered surfactant protein processing and herpesvirus infection. Am. J. Physiol. Lung Cell Mol. Physiol. 294, L1119–L1126. doi:10.1152/ajplung.00382.2007
Lee, D., Hokinson, D., Park, S., Elvira, R., Kusuma, F., Lee, J. M., et al. (2019). ER stress induces cell cycle arrest at the G2/M phase through eIF2α phosphorylation and GADD45α. Int. J. Mol. Sci. 20, 6309. doi:10.3390/ijms20246309
Lee, J. H., and Lee, J. (2022). Endoplasmic reticulum (ER) stress and its role in pancreatic β-cell dysfunction and senescence in type 2 diabetes. Int. J. Mol. Sci. 23, 4843. doi:10.3390/ijms23094843
Lee, J. H., Mand, M. R., Kao, C. H., Zhou, Y., Ryu, S. W., Richards, A. L., et al. (2018). ATM directs DNA damage responses and proteostasis via genetically separable pathways. Sci. Signal 11, eaan5598. doi:10.1126/scisignal.aan5598
Le Goupil, S., Laprade, H., Aubry, M., and Chevet, E. (2024). Exploring the IRE1 interactome: from canonical signaling functions to unexpected roles. J. Biol. Chem. 300, 107169. doi:10.1016/j.jbc.2024.107169
Lei, Y., Yu, H., Ding, S., Liu, H., Liu, C., and Fu, R. (2024). Molecular mechanism of ATF6 in unfolded protein response and its role in disease. Heliyon 10, e25937. doi:10.1016/j.heliyon.2024.e25937
Lenaz, G. (2012). Mitochondria and reactive oxygen species. Which role in physiology and pathology? Adv. Exp. Med. Biol. 942, 93–136. doi:10.1007/978-94-007-2869-1_5
Li, W., Zhu, J., Dou, J., She, H., Tao, K., Xu, H., et al. (2017). Phosphorylation of LAMP2A by p38 MAPK couples ER stress to chaperone-mediated autophagy. Nat. Commun. 8, 1763. doi:10.1038/s41467-017-01609-x
Lin, F., Liao, C., Zhang, J., Sun, Y., Lu, W., Bai, Y., et al. (2022). Hydrogen sulfide inhibits bronchial epithelial cell epithelial mesenchymal transition through regulating endoplasm reticulum stress. Front. Mol. Biosci. 9, 828766. doi:10.3389/fmolb.2022.828766
Lindahl, T., and Barnes, D. E. (2000). Repair of endogenous DNA damage. Cold Spring Harb. Symp. Quant. Biol. 65, 127–134. doi:10.1101/sqb.2000.65.127
Liu, Y., Xu, X., Lei, W., Hou, Y., Zhang, Y., Tang, R., et al. (2022). The NLRP3 inflammasome in fibrosis and aging: the known unknowns. Ageing Res. Rev. 79, 101638. doi:10.1016/j.arr.2022.101638
López-Otín, C., Blasco, M. A., Partridge, L., Serrano, M., and Kroemer, G. (2013). The hallmarks of aging. Cell 153, 1194–1217. doi:10.1016/j.cell.2013.05.039
López-Otín, C., Blasco, M. A., Partridge, L., Serrano, M., and Kroemer, G. (2023). Hallmarks of aging: an expanding universe. Cell 186, 243–278. doi:10.1016/j.cell.2022.11.001
Lubamba, B. A., Jones, L. C., O'Neal, W. K., Boucher, R. C., and Ribeiro, C. M. (2015). X-Box-Binding protein 1 and innate immune responses of human cystic fibrosis alveolar macrophages. Am. J. Respir. Crit. Care Med. 192, 1449–1461. doi:10.1164/rccm.201504-0657OC
Luo, F., Sandhu, A. F., Rungratanawanich, W., Williams, G. E., Akbar, M., Zhou, S., et al. (2020). Melatonin and autophagy in aging-related neurodegenerative diseases. Int. J. Mol. Sci. 21, 7174. doi:10.3390/ijms21197174
Luppi, F., Kalluri, M., Faverio, P., Kreuter, M., and Ferrara, G. (2021). Idiopathic pulmonary fibrosis beyond the lung: understanding disease mechanisms to improve diagnosis and management. Respir. Res. 22, 109. doi:10.1186/s12931-021-01711-1
Luthers, C. R., Dunn, T. M., and Snow, A. L. (2020). ORMDL3 and asthma: linking sphingolipid regulation to altered T cell function. Front. Immunol. 11, 597945. doi:10.3389/fimmu.2020.597945
Macario, A. J., and Conway de Macario, E. (2002). Sick chaperones and ageing: a perspective. Ageing Res. Rev. 1, 295–311. doi:10.1016/s1568-1637(01)00005-8
MacNee, W. (2005). Pulmonary and systemic oxidant/antioxidant imbalance in chronic obstructive pulmonary disease. Proc. Am. Thorac. Soc. 2, 50–60. doi:10.1513/pats.200411-056SF
Mahalanobish, S., Dutta, S., Saha, S., and Sil, P. C. (2020). Melatonin induced suppression of ER stress and mitochondrial dysfunction inhibited NLRP3 inflammasome activation in COPD mice. Food Chem. Toxicol. 144, 111588. doi:10.1016/j.fct.2020.111588
Makhija, L., Krishnan, V., Rehman, R., Chakraborty, S., Maity, S., Mabalirajan, U., et al. (2014). Chemical chaperones mitigate experimental asthma by attenuating endoplasmic reticulum stress. Am. J. Respir. Cell Mol. Biol. 50, 923–931. doi:10.1165/rcmb.2013-0320OC
Mao, H., Chen, W., Chen, L., and Li, L. (2022). Potential role of mitochondria-associated endoplasmic reticulum membrane proteins in diseases. Biochem. Pharmacol. 199, 115011. doi:10.1016/j.bcp.2022.115011
Marciniak, S. J., Yun, C. Y., Oyadomari, S., Novoa, I., Zhang, Y., Jungreis, R., et al. (2004). CHOP induces death by promoting protein synthesis and oxidation in the stressed endoplasmic reticulum. Genes Dev. 18, 3066–3077. doi:10.1101/gad.1250704
Martino, M. E., Olsen, J. C., Fulcher, N. B., Wolfgang, M. C., O'Neal, W. K., and Ribeiro, C. M. (2009). Airway epithelial inflammation-induced endoplasmic reticulum Ca2+ store expansion is mediated by X-box binding protein-1. J. Biol. Chem. 284, 14904–14913. doi:10.1074/jbc.M809180200
Matos, L., Gouveia, A. M., and Almeida, H. (2015). ER stress response in human cellular models of senescence. J. Gerontol. A Biol. Sci. Med. Sci. 70, 924–935. doi:10.1093/gerona/glu129
Meduri, G. U., and Eltorky, M. A. (2015). Understanding ARDS-associated fibroproliferation. Intensive Care Med. 41, 517–520. doi:10.1007/s00134-014-3613-0
Merali, S., Barrero, C. A., Bowler, R. P., Chen, D. E., Criner, G., Braverman, A., et al. (2014). Analysis of the plasma proteome in COPD: novel low abundance proteins reflect the severity of lung remodeling. Copd 11, 177–189. doi:10.3109/15412555.2013.831063
Meyers, A. K., and Zhu, X. (2020). The NLRP3 inflammasome: metabolic regulation and contribution to inflammaging. Cells 9, 1808. doi:10.3390/cells9081808
Misra, V., Lee, H., Singh, A., Huang, K., Thimmulappa, R. K., Mitzner, W., et al. (2007). Global expression profiles from C57BL/6J and DBA/2J mouse lungs to determine aging-related genes. Physiol. Genomics 31, 429–440. doi:10.1152/physiolgenomics.00060.2007
Miwa, S., Kashyap, S., Chini, E., and von Zglinicki, T. (2022). Mitochondrial dysfunction in cell senescence and aging. J. Clin. Invest 132, e158447. doi:10.1172/JCI158447
Moffatt, M. F., Kabesch, M., Liang, L., Dixon, A. L., Strachan, D., Heath, S., et al. (2007). Genetic variants regulating ORMDL3 expression contribute to the risk of childhood asthma. Nature 448, 470–473. doi:10.1038/nature06014
Mogensen, T. H. (2009). Pathogen recognition and inflammatory signaling in innate immune defenses. Clin. Microbiol. Rev. 22, 240–273. Table of Contents. doi:10.1128/CMR.00046-08
Mohrin, M., Widjaja, A., Liu, Y., Luo, H., and Chen, D. (2018). The mitochondrial unfolded protein response is activated upon hematopoietic stem cell exit from quiescence. Aging Cell 17, e12756. doi:10.1111/acel.12756
Moltedo, O., Remondelli, P., and Amodio, G. (2019). The mitochondria-endoplasmic reticulum contacts and their critical role in aging and age-associated diseases. Front. Cell Dev. Biol. 7, 172. doi:10.3389/fcell.2019.00172
Mora, A. L., Bueno, M., and Rojas, M. (2017). Mitochondria in the spotlight of aging and idiopathic pulmonary fibrosis. J. Clin. Invest 127, 405–414. doi:10.1172/JCI87440
Muller, C., Bandemer, J., Vindis, C., Camaré, C., Mucher, E., Guéraud, F., et al. (2013). Protein disulfide isomerase modification and inhibition contribute to ER stress and apoptosis induced by oxidized low density lipoproteins. Antioxid. Redox Signal 18, 731–742. doi:10.1089/ars.2012.4577
Nagasaki, T., and Matsumoto, H. (2013). Influences of smoking and aging on allergic airway inflammation in asthma. Allergol. Int. 62, 171–179. doi:10.2332/allergolint.12-RA-0523
Naidoo, N. (2009). ER and aging-Protein folding and the ER stress response. Ageing Res. Rev. 8, 150–159. doi:10.1016/j.arr.2009.03.001
Nakada, E. M., Sun, R., Fujii, U., and Martin, J. G. (2021). The impact of endoplasmic reticulum-associated protein modifications, folding and degradation on lung structure and function. Front. Physiol. 12, 665622. doi:10.3389/fphys.2021.665622
Nakajima, S., Hiramatsu, N., Hayakawa, K., Saito, Y., Kato, H., Huang, T., et al. (2011). Selective abrogation of BiP/GRP78 blunts activation of NF-κB through the ATF6 branch of the UPR: involvement of C/EBPβ and mTOR-dependent dephosphorylation of Akt. Mol. Cell Biol. 31, 1710–1718. doi:10.1128/MCB.00939-10
Nakashima, A., Cheng, S. B., Kusabiraki, T., Motomura, K., Aoki, A., Ushijima, A., et al. (2019). Endoplasmic reticulum stress disrupts lysosomal homeostasis and induces blockade of autophagic flux in human trophoblasts. Sci. Rep. 9, 11466. doi:10.1038/s41598-019-47607-5
Nijholt, D. A., de Graaf, T. R., van Haastert, E. S., Oliveira, A. O., Berkers, C. R., Zwart, R., et al. (2011). Endoplasmic reticulum stress activates autophagy but not the proteasome in neuronal cells: implications for Alzheimer's disease. Cell Death Differ. 18, 1071–1081. doi:10.1038/cdd.2010.176
Nuss, J. E., Choksi, K. B., DeFord, J. H., and Papaconstantinou, J. (2008). Decreased enzyme activities of chaperones PDI and BiP in aged mouse livers. Biochem. Biophys. Res. Commun. 365, 355–361. doi:10.1016/j.bbrc.2007.10.194
Oslowski, C. M., Hara, T., O'Sullivan-Murphy, B., Kanekura, K., Lu, S., Hara, M., et al. (2012). Thioredoxin-interacting protein mediates ER stress-induced β cell death through initiation of the inflammasome. Cell Metab. 16, 265–273. doi:10.1016/j.cmet.2012.07.005
Osorio, F., Lambrecht, B., and Janssens, S. (2013). The UPR and lung disease. Semin. Immunopathol. 35, 293–306. doi:10.1007/s00281-013-0368-6
Pardo, A., and Selman, M. (2016). Lung fibroblasts, aging, and idiopathic pulmonary fibrosis. Ann. Am. Thorac. Soc. 13 (Suppl. 5), S417–s421. doi:10.1513/AnnalsATS.201605-341AW
Park, J., Cho, J., and Song, E. J. (2020). Ubiquitin-proteasome system (UPS) as a target for anticancer treatment. Arch. Pharm. Res. 43, 1144–1161. doi:10.1007/s12272-020-01281-8
Pathinayake, P. S., Hsu, A. C., Waters, D. W., Hansbro, P. M., Wood, L. G., and Wark, P. A. B. (2018). Understanding the unfolded protein response in the pathogenesis of asthma. Front. Immunol. 9, 175. doi:10.3389/fimmu.2018.00175
Paz Gavilán, M., Vela, J., Castaño, A., Ramos, B., del Río, J. C., Vitorica, J., et al. (2006). Cellular environment facilitates protein accumulation in aged rat hippocampus. Neurobiol. Aging 27, 973–982. doi:10.1016/j.neurobiolaging.2005.05.010
Peng, G., Li, L., Liu, Y., Pu, J., Zhang, S., Yu, J., et al. (2011). Oleate blocks palmitate-induced abnormal lipid distribution, endoplasmic reticulum expansion and stress, and insulin resistance in skeletal muscle. Endocrinology 152, 2206–2218. doi:10.1210/en.2010-1369
Phillips, M. J., and Voeltz, G. K. (2016). Structure and function of ER membrane contact sites with other organelles. Nat. Rev. Mol. Cell Biol. 17, 69–82. doi:10.1038/nrm.2015.8
Pluquet, O., Pourtier, A., and Abbadie, C. (2015). The unfolded protein response and cellular senescence. A review in the theme: cellular mechanisms of endoplasmic reticulum stress signaling in health and disease. Am. J. Physiol. Cell Physiol. 308, C415–C425. doi:10.1152/ajpcell.00334.2014
Polverino, F., Wu, T. D., Rojas-Quintero, J., Wang, X., Mayo, J., Tomchaney, M., et al. (2021). Metformin: experimental and clinical evidence for a potential role in emphysema treatment. Am. J. Respir. Crit. Care Med. 204, 651–666. doi:10.1164/rccm.202012-4510OC
Proença de Oliveira-Maul, J., Barbosa de Carvalho, H., Goto, D. M., Maia, R. M., Fló, C., Barnabé, V., et al. (2013). Aging, diabetes, and hypertension are associated with decreased nasal mucociliary clearance. Chest 143, 1091–1097. doi:10.1378/chest.12-1183
Pryor, W. A., and Stone, K. (1993). Oxidants in cigarette smoke. Radicals, hydrogen peroxide, peroxynitrate, and peroxynitrite. Ann. N. Y. Acad. Sci. 686, 12–28. ; discussion 27-8. doi:10.1111/j.1749-6632.1993.tb39148.x
Rawlins, E. L., Okubo, T., Xue, Y., Brass, D. M., Auten, R. L., Hasegawa, H., et al. (2009). The role of Scgb1a1+ Clara cells in the long-term maintenance and repair of lung airway, but not alveolar, epithelium. Cell Stem Cell 4, 525–534. doi:10.1016/j.stem.2009.04.002
Reed, M., Morris, S. H., Owczarczyk, A. B., and Lukacs, N. W. (2015). Deficiency of autophagy protein Map1-LC3b mediates IL-17-dependent lung pathology during respiratory viral infection via ER stress-associated IL-1. Mucosal Immunol. 8, 1118–1130. doi:10.1038/mi.2015.3
Ribeiro, C. M., and Boucher, R. C. (2010). Role of endoplasmic reticulum stress in cystic fibrosis-related airway inflammatory responses. Proc. Am. Thorac. Soc. 7, 387–394. doi:10.1513/pats.201001-017AW
Ribeiro, C. M., and O'Neal, W. K. (2012). Endoplasmic reticulum stress in chronic obstructive lung diseases. Curr. Mol. Med. 12, 872–882. doi:10.2174/156652412801318791
Ribeiro, C. M., Paradiso, A. M., Schwab, U., Perez-Vilar, J., Jones, L., O'Neal, W., et al. (2005). Chronic airway infection/inflammation induces a Ca2+i-dependent hyperinflammatory response in human cystic fibrosis airway epithelia. J. Biol. Chem. 280, 17798–17806. doi:10.1074/jbc.M410618200
Rochester, C. L., Vogiatzis, I., Holland, A. E., Lareau, S. C., Marciniuk, D. D., Puhan, M. A., et al. (2015). An official American thoracic society/European respiratory society policy statement: enhancing implementation, use, and delivery of pulmonary rehabilitation. Am. J. Respir. Crit. Care Med. 192, 1373–1386. doi:10.1164/rccm.201510-1966ST
Rock, J. R., Onaitis, M. W., Rawlins, E. L., Lu, Y., Clark, C. P., Xue, Y., et al. (2009). Basal cells as stem cells of the mouse trachea and human airway epithelium. Proc. Natl. Acad. Sci. U. S. A. 106, 12771–12775. doi:10.1073/pnas.0906850106
Ryan, A. J., Larson-Casey, J. L., He, C., Murthy, S., and Carter, A. B. (2014). Asbestos-induced disruption of calcium homeostasis induces endoplasmic reticulum stress in macrophages. J. Biol. Chem. 289, 33391–33403. doi:10.1074/jbc.M114.579870
Salminen, A., and Kaarniranta, K. (2010). ER stress and hormetic regulation of the aging process. Ageing Res. Rev. 9, 211–217. doi:10.1016/j.arr.2010.04.003
Sancar, A., Lindsey-Boltz, L. A., Unsal-Kaçmaz, K., and Linn, S. (2004). Molecular mechanisms of mammalian DNA repair and the DNA damage checkpoints. Annu. Rev. Biochem. 73, 39–85. doi:10.1146/annurev.biochem.73.011303.073723
Sang, A., Wang, Y., Wang, S., Wang, Q., Wang, X., Li, X., et al. (2022). Quercetin attenuates sepsis-induced acute lung injury via suppressing oxidative stress-mediated ER stress through activation of SIRT1/AMPK pathways. Cell Signal 96, 110363. doi:10.1016/j.cellsig.2022.110363
Santoro, A., Bientinesi, E., and Monti, D. (2021). Immunosenescence and inflammaging in the aging process: age-related diseases or longevity? Ageing Res. Rev. 71, 101422. doi:10.1016/j.arr.2021.101422
Santos, C. X., Nabeebaccus, A. A., Shah, A. M., Camargo, L. L., Filho, S. V., and Lopes, L. R. (2014). Endoplasmic reticulum stress and Nox-mediated reactive oxygen species signaling in the peripheral vasculature: potential role in hypertension. Antioxid. Redox Signal 20, 121–134. doi:10.1089/ars.2013.5262
Saric, A., Freeman, S. A., Williamson, C. D., Jarnik, M., Guardia, C. M., Fernandopulle, M. S., et al. (2021). SNX19 restricts endolysosome motility through contacts with the endoplasmic reticulum. Nat. Commun. 12, 4552. doi:10.1038/s41467-021-24709-1
Schafer, M. J., White, T. A., Iijima, K., Haak, A. J., Ligresti, G., Atkinson, E. J., et al. (2017). Cellular senescence mediates fibrotic pulmonary disease. Nat. Commun. 8, 14532. doi:10.1038/ncomms14532
Schmiedel, B. J., Seumois, G., Samaniego-Castruita, D., Cayford, J., Schulten, V., Chavez, L., et al. (2016). 17q21 asthma-risk variants switch CTCF binding and regulate IL-2 production by T cells. Nat. Commun. 7, 13426. doi:10.1038/ncomms13426
Schneider, J. L., Rowe, J. H., Garcia-de-Alba, C., Kim, C. F., Sharpe, A. H., and Haigis, M. C. (2021). The aging lung: physiology, disease, and immunity. Cell 184, 1990–2019. doi:10.1016/j.cell.2021.03.005
Senft, D., and Ronai, Z. A. (2015). UPR, autophagy, and mitochondria crosstalk underlies the ER stress response. Trends Biochem. Sci. 40, 141–148. doi:10.1016/j.tibs.2015.01.002
Sharma, G., and Goodwin, J. (2006). Effect of aging on respiratory system physiology and immunology. Clin. Interv. Aging 1, 253–260. doi:10.2147/ciia.2006.1.3.253
Shin, J., He, M., Liu, Y., Paredes, S., Villanova, L., Brown, K., et al. (2013). SIRT7 represses Myc activity to suppress ER stress and prevent fatty liver disease. Cell Rep. 5, 654–665. doi:10.1016/j.celrep.2013.10.007
Shivshankar, P., Boyd, A. R., Le Saux, C. J., Yeh, I. T., and Orihuela, C. J. (2011). Cellular senescence increases expression of bacterial ligands in the lungs and is positively correlated with increased susceptibility to pneumococcal pneumonia. Aging Cell 10, 798–806. doi:10.1111/j.1474-9726.2011.00720.x
Shoulders, M. D., Ryno, L. M., Genereux, J. C., Moresco, J. J., Tu, P. G., Wu, C., et al. (2013). Stress-independent activation of XBP1s and/or ATF6 reveals three functionally diverse ER proteostasis environments. Cell Rep. 3, 1279–1292. doi:10.1016/j.celrep.2013.03.024
Siddesha, J. M., Nakada, E. M., Mihavics, B. R., Hoffman, S. M., Rattu, G. K., Chamberlain, N., et al. (2016). Effect of a chemical chaperone, tauroursodeoxycholic acid, on HDM-induced allergic airway disease. Am. J. Physiol. Lung Cell Mol. Physiol. 310, L1243–L1259. doi:10.1152/ajplung.00396.2015
Sims, A. C., Mitchell, H. D., Gralinski, L. E., Kyle, J. E., Burnum-Johnson, K. E., Lam, M., et al. (2021). Unfolded protein response inhibition reduces Middle East respiratory syndrome coronavirus-induced acute lung injury. mBio 12, e0157221. doi:10.1128/mBio.01572-21
Song, S., Lam, E. W., Tchkonia, T., Kirkland, J. L., and Sun, Y. (2020). Senescent cells: emerging targets for human aging and age-related diseases. Trends Biochem. Sci. 45, 578–592. doi:10.1016/j.tibs.2020.03.008
Staudt, M. R., Buro-Auriemma, L. J., Walters, M. S., Salit, J., Vincent, T., Shaykhiev, R., et al. (2014). Airway Basal stem/progenitor cells have diminished capacity to regenerate airway epithelium in chronic obstructive pulmonary disease. Am. J. Respir. Crit. Care Med. 190, 955–958. doi:10.1164/rccm.201406-1167LE
Storer, M., Mas, A., Robert-Moreno, A., Pecoraro, M., Ortells, M. C., Di Giacomo, V., et al. (2013). Senescence is a developmental mechanism that contributes to embryonic growth and patterning. Cell 155, 1119–1130. doi:10.1016/j.cell.2013.10.041
Sun, Q., Han, W., Hu, H., Fan, D., Li, Y., Zhang, Y., et al. (2017). Hydrogen alleviates hyperoxic acute lung injury related endoplasmic reticulum stress in rats through upregulation of SIRT1. Free Radic. Res. 51, 622–632. doi:10.1080/10715762.2017.1351027
Sun-Wang, J. L., Ivanova, S., and Zorzano, A. (2020). The dialogue between the ubiquitin-proteasome system and autophagy: implications in ageing. Ageing Res. Rev. 64, 101203. doi:10.1016/j.arr.2020.101203
Svartengren, M., Falk, R., and Philipson, K. (2005). Long-term clearance from small airways decreases with age. Eur. Respir. J. 26, 609–615. doi:10.1183/09031936.05.00002105
Tabas, I., and Ron, D. (2011). Integrating the mechanisms of apoptosis induced by endoplasmic reticulum stress. Nat. Cell Biol. 13, 184–190. doi:10.1038/ncb0311-184
Tagawa, Y., Hiramatsu, N., Kato, H., Sakoh, T., Nakajima, S., Hayakawa, K., et al. (2011). Induction of CCAAT/enhancer-binding protein-homologous protein by cigarette smoke through the superoxide anion-triggered PERK-eIF2α pathway. Toxicology 287, 105–112. doi:10.1016/j.tox.2011.06.005
Tan, S. X., Jiang, D. X., Hu, R. C., Dai, A. G., Gan, G. X., Fu, D. Y., et al. (2017). Endoplasmic reticulum stress induces HRD1 to protect alveolar type II epithelial cells from apoptosis induced by cigarette smoke extract. Cell Physiol. Biochem. 43, 1337–1345. doi:10.1159/000481845
Tanaka, Y., Ishitsuka, Y., Hayasaka, M., Yamada, Y., Miyata, K., Endo, M., et al. (2015). The exacerbating roles of CCAAT/enhancer-binding protein homologous protein (CHOP) in the development of bleomycin-induced pulmonary fibrosis and the preventive effects of tauroursodeoxycholic acid (TUDCA) against pulmonary fibrosis in mice. Pharmacol. Res. 99, 52–62. doi:10.1016/j.phrs.2015.05.004
Tang, F., and Ling, C. (2019). Curcumin ameliorates chronic obstructive pulmonary disease by modulating autophagy and endoplasmic reticulum stress through regulation of SIRT1 in a rat model. J. Int. Med. Res. 47, 4764–4774. doi:10.1177/0300060519869459
Tao, R., Chen, H., Gao, C., Xue, P., Yang, F., Han, J. D., et al. (2011). Xbp1-mediated histone H4 deacetylation contributes to DNA double-strand break repair in yeast. Cell Res. 21, 1619–1633. doi:10.1038/cr.2011.58
Tsai, Y. L., Ha, D. P., Zhao, H., Carlos, A. J., Wei, S., Pun, T. K., et al. (2018). Endoplasmic reticulum stress activates SRC, relocating chaperones to the cell surface where GRP78/CD109 blocks TGF-β signaling. Proc. Natl. Acad. Sci. U. S. A. 115, E4245–e4254. doi:10.1073/pnas.1714866115
Tu, B. P., and Weissman, J. S. (2004). Oxidative protein folding in eukaryotes: mechanisms and consequences. J. Cell Biol. 164, 341–346. doi:10.1083/jcb.200311055
van der Toorn, M., Rezayat, D., Kauffman, H. F., Bakker, S. J., Gans, R. O., Koëter, G. H., et al. (2009). Lipid-soluble components in cigarette smoke induce mitochondrial production of reactive oxygen species in lung epithelial cells. Am. J. Physiol. Lung Cell Mol. Physiol. 297, L109–L114. doi:10.1152/ajplung.90461.2008
van Galen, P., Kreso, A., Mbong, N., Kent, D. G., Fitzmaurice, T., Chambers, J. E., et al. (2014). The unfolded protein response governs integrity of the haematopoietic stem-cell pool during stress. Nature 510, 268–272. doi:10.1038/nature13228
van Rijt, S. H., Keller, I. E., John, G., Kohse, K., Yildirim, A., Eickelberg, O., et al. (2012). Acute cigarette smoke exposure impairs proteasome function in the lung. Am. J. Physiol. Lung Cell Mol. Physiol. 303, L814–L823. doi:10.1152/ajplung.00128.2012
van Vliet, A. R., and Agostinis, P. (2018). Mitochondria-associated membranes and ER stress. Curr. Top. Microbiol. Immunol. 414, 73–102. doi:10.1007/82_2017_2
Viña, J., Rodriguez-Mañas, L., Salvador-Pascual, A., Tarazona-Santabalbina, F. J., and Gomez-Cabrera, M. C. (2016). Exercise: the lifelong supplement for healthy ageing and slowing down the onset of frailty. J. Physiol. 594, 1989–1999. doi:10.1113/JP270536
Vogelmeier, C. F., Criner, G. J., Martinez, F. J., Anzueto, A., Barnes, P. J., Bourbeau, J., et al. (2017). Global strategy for the diagnosis, management, and prevention of chronic obstructive lung disease 2017 report. GOLD executive summary. Am. J. Respir. Crit. Care Med. 195, 557–582. doi:10.1164/rccm.201701-0218PP
Volgyi, K., Juhász, G., Kovacs, Z., and Penke, B. (2015). Dysfunction of endoplasmic reticulum (ER) and mitochondria (MT) in Alzheimer's disease: the role of the ER-MT cross-talk. Curr. Alzheimer Res. 12, 655–672. doi:10.2174/1567205012666150710095035
Walter, P., and Ron, D. (2011). The unfolded protein response: from stress pathway to homeostatic regulation. Science 334, 1081–1086. doi:10.1126/science.1209038
Wang, F., Ma, J., Wang, J., Chen, M., Xia, H., Yao, S., et al. (2022b). SIRT1 ameliorated septic associated-lung injury and macrophages apoptosis via inhibiting endoplasmic reticulum stress. Cell Signal 97, 110398. doi:10.1016/j.cellsig.2022.110398
Wang, F. M., Chen, Y. J., and Ouyang, H. J. (2011). Regulation of unfolded protein response modulator XBP1s by acetylation and deacetylation. Biochem. J. 433, 245–252. doi:10.1042/BJ20101293
Wang, M., and Kaufman, R. J. (2016). Protein misfolding in the endoplasmic reticulum as a conduit to human disease. Nature 529, 326–335. doi:10.1038/nature17041
Wang, Q. L., Yang, L., Peng, Y., Gao, M., Yang, M. S., Xing, W., et al. (2019). Ginsenoside Rg1 regulates SIRT1 to Ameliorate sepsis-induced lung inflammation and injury via inhibiting endoplasmic reticulum stress and inflammation. Mediat. Inflamm. 2019, 6453296. doi:10.1155/2019/6453296
Wang, S., Gong, L., Mo, Y., Zhang, J., Jiang, Z., Tian, Z., et al. (2022a). Resveratrol attenuates inflammation and apoptosis through alleviating endoplasmic reticulum stress via Akt/mTOR pathway in fungus-induced allergic airways inflammation. Int. Immunopharmacol. 103, 108489. doi:10.1016/j.intimp.2021.108489
Wang, S., and Kaufman, R. J. (2012). The impact of the unfolded protein response on human disease. J. Cell Biol. 197, 857–867. doi:10.1083/jcb.201110131
Wang, Y., Wu, Z. Z., and Wang, W. (2017). Inhibition of endoplasmic reticulum stress alleviates cigarette smoke-induced airway inflammation and emphysema. Oncotarget 8, 77685–77695. doi:10.18632/oncotarget.20768
Wątroba, M., Dudek, I., Skoda, M., Stangret, A., Rzodkiewicz, P., and Szukiewicz, D. (2017). Sirtuins, epigenetics and longevity. Ageing Res. Rev. 40, 11–19. doi:10.1016/j.arr.2017.08.001
Wei, J., Rahman, S., Ayaub, E. A., Dickhout, J. G., and Ask, K. (2013). Protein misfolding and endoplasmic reticulum stress in chronic lung disease. Chest 143, 1098–1105. doi:10.1378/chest.12-2133
Weidner, J., Jarenbäck, L., Åberg, I., Westergren-Thorsson, G., Ankerst, J., Bjermer, L., et al. (2018). Endoplasmic reticulum, Golgi, and lysosomes are disorganized in lung fibroblasts from chronic obstructive pulmonary disease patients. Physiol. Rep. 6, e13584. doi:10.14814/phy2.13584
Win, S., Than, T. A., Fernandez-Checa, J. C., and Kaplowitz, N. (2014). JNK interaction with Sab mediates ER stress induced inhibition of mitochondrial respiration and cell death. Cell Death Dis. 5, e989. doi:10.1038/cddis.2013.522
Wiseman, R. L., Mesgarzadeh, J. S., and Hendershot, L. M. (2022). Reshaping endoplasmic reticulum quality control through the unfolded protein response. Mol. Cell 82, 1477–1491. doi:10.1016/j.molcel.2022.03.025
Wolters, P. J., Collard, H. R., and Jones, K. D. (2014). Pathogenesis of idiopathic pulmonary fibrosis. Annu. Rev. Pathol. 9, 157–179. doi:10.1146/annurev-pathol-012513-104706
Xia, S. W., Wang, Z. M., Sun, S. M., Su, Y., Li, Z. H., Shao, J. J., et al. (2020). Endoplasmic reticulum stress and protein degradation in chronic liver disease. Pharmacol. Res. 161, 105218. doi:10.1016/j.phrs.2020.105218
Yao, Y., Wang, Y., Zhang, Z., He, L., Zhu, J., Zhang, M., et al. (2016). Chop deficiency protects mice against bleomycin-induced pulmonary fibrosis by attenuating M2 macrophage production. Mol. Ther. 24, 915–925. doi:10.1038/mt.2016.36
Yap, J., Chen, X., Delmotte, P., and Sieck, G. C. (2020). TNFα selectively activates the IRE1α/XBP1 endoplasmic reticulum stress pathway in human airway smooth muscle cells. Am. J. Physiol. Lung Cell Mol. Physiol. 318, L483–l493. doi:10.1152/ajplung.00212.2019
Ye, Y., Shibata, Y., Yun, C., Ron, D., and Rapoport, T. A. (2004). A membrane protein complex mediates retro-translocation from the ER lumen into the cytosol. Nature 429, 841–847. doi:10.1038/nature02656
Yoshida, H., Matsui, T., Yamamoto, A., Okada, T., and Mori, K. (2001). XBP1 mRNA is induced by ATF6 and spliced by IRE1 in response to ER stress to produce a highly active transcription factor. Cell 107, 881–891. doi:10.1016/s0092-8674(01)00611-0
Yu, K. N., Chang, S. H., Park, S. J., Lim, J., Lee, J., Yoon, T. J., et al. (2015). Titanium dioxide nanoparticles induce endoplasmic reticulum stress-mediated autophagic cell death via mitochondria-associated endoplasmic reticulum membrane disruption in normal lung cells. PLoS One 10, e0131208. doi:10.1371/journal.pone.0131208
Yu, X., Li, X., Wang, L., Liu, R., Xie, Y., Li, S., et al. (2019). Pulmonary rehabilitation for exercise tolerance and quality of life in IPF patients: a systematic review and meta-analysis. Biomed. Res. Int. 2019, 8498603. doi:10.1155/2019/8498603
Yu, Y., Yang, A., Yu, G., and Wang, H. (2022). Endoplasmic reticulum stress in chronic obstructive pulmonary disease: mechanisms and future perspectives. Biomolecules 12, 1637. doi:10.3390/biom12111637
Zahiruddin, A. S., Grant, J. A., and Sur, S. (2018). Role of epigenetics and DNA-damage in asthma. Curr. Opin. Allergy Clin. Immunol. 18, 32–37. doi:10.1097/ACI.0000000000000415
Zaynagetdinov, R., Sherrill, T. P., Gleaves, L. A., Hunt, P., Han, W., McLoed, A. G., et al. (2016). Chronic NF-κB activation links COPD and lung cancer through generation of an immunosuppressive microenvironment in the lungs. Oncotarget 7, 5470–5482. doi:10.18632/oncotarget.6562
Zeng, M., Sang, W., Chen, S., Chen, R., Zhang, H., Xue, F., et al. (2017). 4-PBA inhibits LPS-induced inflammation through regulating ER stress and autophagy in acute lung injury models. Toxicol. Lett. 271, 26–37. doi:10.1016/j.toxlet.2017.02.023
Zhang, G., Li, J., Purkayastha, S., Tang, Y., Zhang, H., Yin, Y., et al. (2013). Hypothalamic programming of systemic ageing involving IKK-β, NF-κB and GnRH. Nature 497, 211–216. doi:10.1038/nature12143
Zhang, K., and Kaufman, R. J. (2008). From endoplasmic-reticulum stress to the inflammatory response. Nature 454, 455–462. doi:10.1038/nature07203
Zhang, L., Luo, B., Ting, Y., He, S., Xie, L., and Sun, S. (2020b). SIRT1 attenuates endoplasmic reticulum stress and apoptosis in rat models of COPD. Growth factors. 38, 94–104. doi:10.1080/08977194.2020.1810029
Zhang, L., Wang, X., Cueto, R., Effi, C., Zhang, Y., Tan, H., et al. (2019). Biochemical basis and metabolic interplay of redox regulation. Redox Biol. 26, 101284. doi:10.1016/j.redox.2019.101284
Zhang, W., Qu, J., Liu, G. H., and Belmonte, J. C. I. (2020a). The ageing epigenome and its rejuvenation. Nat. Rev. Mol. Cell Biol. 21, 137–150. doi:10.1038/s41580-019-0204-5
Keywords: ERstress, upr, autophagy, aging, lung dysfunction
Citation: Fu Z, Wang W and Gao Y (2024) Understanding the impact of ER stress on lung physiology. Front. Cell Dev. Biol. 12:1466997. doi: 10.3389/fcell.2024.1466997
Received: 24 July 2024; Accepted: 22 November 2024;
Published: 18 December 2024.
Edited by:
Yan Chun Li, The University of Chicago, United StatesReviewed by:
Francisco Javier del Castillo, Ramón y Cajal University Hospital, SpainCopyright © 2024 Fu, Wang and Gao. This is an open-access article distributed under the terms of the Creative Commons Attribution License (CC BY). The use, distribution or reproduction in other forums is permitted, provided the original author(s) and the copyright owner(s) are credited and that the original publication in this journal is cited, in accordance with accepted academic practice. No use, distribution or reproduction is permitted which does not comply with these terms.
*Correspondence: Yuan Gao, c2p5dWFuZ2c2NTE1QDEyNi5jb20=
Disclaimer: All claims expressed in this article are solely those of the authors and do not necessarily represent those of their affiliated organizations, or those of the publisher, the editors and the reviewers. Any product that may be evaluated in this article or claim that may be made by its manufacturer is not guaranteed or endorsed by the publisher.
Research integrity at Frontiers
Learn more about the work of our research integrity team to safeguard the quality of each article we publish.