- 1Cancer Institute, The Affiliated Hospital of Qingdao University, Qingdao University, Qingdao Cancer Institute, Qingdao, China
- 2School of Physical Education, Qingdao University, Qingdao, China
Nicotinamide adenine dinucleotide (NAD+) is crucial for cellular energy production, serving as a coenzyme in oxidation-reduction reactions. It also supports enzymes involved in processes such as DNA repair, aging, and immune responses. Lower NAD+ levels have been associated with various diseases, highlighting the importance of replenishing NAD+. Nicotinamide phosphoribosyltransferase (NAMPT) plays a critical role in the NAD+ salvage pathway, which helps sustain NAD+ levels, particularly in high-energy tissues like skeletal muscle.This review explores how the NAMPT-driven NAD+ salvage pathway influences skeletal muscle health and functionality in aging, type 2 diabetes mellitus (T2DM), and skeletal muscle injury. The review offers insights into enhancing the salvage pathway through exercise and NAD+ boosters as strategies to improve muscle performance. The findings suggest significant potential for using this pathway in the diagnosis, monitoring, and treatment of skeletal muscle conditions.
1 Introduction
Nicotinamide phosphoribosyltransferase (NAMPT) was first identified in B cells, where it helps mature B cells, earning it the initial name Pre-B cell colony-enhancing factor (PBEF) (Samal et al., 1994). Additionally, NAMPT, known as “Visfatin” (Fukuhara et al., 2005) for its insulin-like effects, is secreted by visceral fat. Analysis of the tissue origins of 719 cDNA clones revealed that NAMPT is expressed in nearly all organs, tissues, and cells (Friebe et al., 2011), indicating that this protein may have multiple regulatory functions in human physiological processes. NAD+ is a coenzyme essential for redox reactions in metabolic processes, including glycolysis, the citric acid cycle, and oxidative phosphorylation (Houtkooper et al., 2010). It also acts as a substrate for various NAD+-dependent enzymes, influencing processes regulated by enzymes like silent information regulators (Sirtuins), poly (ADP-ribose)polymerases (PARPs), cyclic ADP-ribose (cADPR) and sterile alpha and TIR motif-containing 1(SARM1)synthases (Cantó et al., 2013; Essuman et al., 2017; Malavasi et al., 2008), thereby impacting cellular functions like DNA repair and signaling. NAD+ is replenished through the De novo biosynthesis pathway, Preiss-Handler pathway and salvage pathway, with 85% of total NAD+ being produced by the salvage pathway. Revollo et al. (2007). In the salvage pathway, NAMPT plays a central role by converting nicotinamide (NAM) into nicotinamide mononucleotide (NMN), which is then converted into NAD+ by enzymes NMNAT1-3, completing the cycle of NAD+ synthesis and breakdown (Revollo et al., 2004) which is depicted in Figure 1.
During intense physical activity, skeletal muscles rely heavily on aerobic oxidation in mitochondria for energy. The tricarboxylic acid cycle, integral to this process, involves NAD+ in three out of four dehydrogenation reactions. Reduced NAD+ levels can impair muscle contractions and disrupt mitochondrial energy metabolism, potentially triggering an energy crisis in skeletal muscles. NAMPT-driven NAD+ salvage pathway is critical for maintaining NAD+ levels in skeletal muscles. Eliminating NAMPT in the skeletal muscles of adult mice led to an 85% reduction in NAD+ content (Frederick et al., 2016). Basse et al. (2021) specifically knocked out NAMPT in the skeletal muscle of fetal mice, the mice began to exhibit abnormal muscle development, impaired function, and decreased motor ability from the age of 4 weeks, gradually progressing to severe myopathy. Despite a normal muscle stem cell pool, it did not alleviate the progression of the myopathy. Further research by Frederick and team shows that mice lacking NAMPT are smaller and exhibit reduced muscle mass as they age, a condition linked to increased expression of genes related to muscle damage and inflammation, as well as decreased metabolic activity (Frederick et al., 2016). Six weeks after knocking out NAMPT, three-month-old male mice exhibited significant neurite loss in both motor and hippocampal neurons (Wang et al., 2017). This disruption of neural regulatory mechanisms, essential for governing muscular activity, led to abnormal movement, muscle atrophy, and ultimately, the death of the mice (Shen et al., 2023); NAD+ supplementation has been shown to improve pathological changes in rats with muscular dystrophy through several mechanisms: enhancing mitochondrial function, increasing the expression of structural proteins like α-dystrobrevin, decreasing poly (ADP-ribosyl)ation, and reducing inflammation and fibrosis, underscoring its importance in muscle health and function (Ryu et al., 2016). Disruptions in NAMPT homeostasis impair the ion transport function of the mitochondrial permeability transition pore (mPTP) (Briston et al., 2019). When NAMPT levels are deficient or insufficient, the mPTP stays persistently open, making mitochondria more sensitive to Ca2+ (Basse et al., 2021). This sensitivity causes mitochondrial Ca2+ overload, triggering oxidative stress in skeletal muscle and further opening the mPTP. This vicious cycle leads to mitochondrial swelling and a decrease in membrane potential. NAMPT deficiencies in skeletal muscle significantly reduce mitochondrial fusion proteins (Mfns) expression and increase mitochondrial fission protein (Drp1) levels and protein acetylation. This imbalance leads to excessive mitochondrial fission and altered post-translational modifications, disrupting mitochondrial structure (Kim et al., 2014). As a result, vital components such as cardiolipin, phosphatidylglycerol, enzymes of the electron transport chain, and citrate are markedly reduced, impairing mitochondrial function and biogenesis (Agerholm et al., 2018). These structural and functional mitochondrial abnormalities lead to oxidative stress and inflammation, which accelerate protein degradation, impair regenerative capacity, and exacerbate skeletal muscle dysfunction (Lee et al., 1998; Wanagat et al., 2001). In conclusion, NAMPT plays a vital role in regulating skeletal muscle growth, development, and contraction by influencing mitochondrial structure and function via its involvement in NAD⁺ synthesis. Under pathological conditions, the enhancement of NAD⁺-centered metabolic pathways has been shown to significantly enhance skeletal muscle function. Subsequent sections will explore how the NAD⁺ salvage pathway mitigates issues such as aging, type 2 diabetes mellitus (T2DM), and skeletal muscle injuries.
2 NAD+ salvage pathway improves age-related decline in skeletal muscle function
2.1 Possible physiological mechanisms underlying the development of sarcopenia
The Asian Working Group for Sarcopenia defines sarcopenia as an age-related condition marked by a decline in muscle mass, strength, and physical function (Chen et al., 2014). Oxidative stress occurs when an imbalance between oxidants and antioxidants leads to excessive oxidative activity, damaging vital molecules such as sugars, lipids, proteins, and DNA (Sies et al., 2017). Aging is associated with a decrease in the quantity and activity of antioxidant enzymes, fostering an overproduction of reactive oxygen species (ROS), which disrupt cellular redox balance (Sullivan-Gunn and Lewandowski, 2013). Research has conclusively demonstrated that oxidative stress significantly contributes to sarcopenia (Coto-Montes et al., 2017) by accelerating muscle protein degradation through hyperactivation of the ubiquitin-proteasome and autophagy-lysosome pathways, while simultaneously impeding protein synthesis (Jang et al., 2020). Furthermore, oxidative stress impairs mitochondrial biogenesis, diminishes ATP production, and disrupts mitochondrial autophagy, leading to energy metabolism disorders in skeletal muscle fibers and exacerbating muscle wasting (Jang et al., 2010). In addition, oxidative stress stimulates the accumulation of inflammatory cytokines and enhances the phagocytic activity of macrophages and neutrophils, triggering a chronic inflammatory response that intensifies oxidative stress (Bartnik et al., 2000.). This vicious cycle further damages muscle fiber structure and function (Chen et al., 2022). Mitochondrial dysfunction, central to aging (López-Otín et al., 2023), involves the accumulation of mtDNA mutations, imbalances in protein synthesis and degradation, instability of respiratory chain complexes, and altered mitochondrial dynamics. These changes undermine mitochondrial integrity and function, contributing to muscle fiber degeneration. Deterioration in mitochondrial function increases ROS production and mitochondrial membrane permeability, precipitating inflammation and cellular destruction (Hepple, 2014).
In the muscles of aged mice and elderly humans, levels of autophagy markers, including autophagy-related protein7 (ATG7)and Light Chain 3 (LC3) lipidation, are reduced, indicating impaired autophagic function (Carnio et al., 2014). Skeletal muscles in ATG7-specific knockout mice exhibit muscle atrophy, reduced strength, and pathological features characteristic of myopathy, with the decline in muscle strength becoming more pronounced with aging (Masiero et al., 2009). Impaired autophagy disrupts the protein quality control system, leading to increased protein aggregates that further exacerbate sarcopenia (Jiao and Demontis, 2017). Aging is often accompanied by chronic low-grade inflammation, primarily associated with senescent cells and their senescence-associated secretory phenotype (SASP). The SASP increases with age, releasing pro-inflammatory cytokines that contribute to both inflammation and aging (Fulop et al., 2018; Fulop et al., 2021). Previous studies have shown that elevated IL-6 levels can predict disability in the elderly, likely due to the direct effects of IL-6 on muscle atrophy (Ferrucci et al., 1999). Additionally, IL-1β levels are negatively correlated with walking speed, further linking inflammation to the decline in skeletal muscle function (Morawin et al., 2023). Inflammatory cytokines activate protein degradation and inhibit protein synthesis, leading to sarcopenia (Jo et al., 2012).
Age-related structural and functional decline of the neuromuscular junction (NMJ) significantly contributes to muscular dystrophy. Aging leads to the thinning and degradation of motor neuron axons, which exhibit disordered terminal branching (Padilla et al., 2021). Decreased reactivity of terminal Schwann cells and a reduction in the quantity and density of acetylcholine receptors on muscle fibers contribute to neural innervation failure and disrupted muscle contractions (Alice et al., 2016).Skeletal muscle stem cells (MuSCs) are crucial for the regenerative and repair functions of skeletal muscles due to their quantity and functionality (Jiang et al., 2024). In male rats between 15 and 18 months of age, the number of MuSCs declines with advancing age, and they undergo morphological changes, including flattening, enlargement, and a loss of three-dimensional structure. Consequently, these alterations weaken the proliferation and self-renewal potential of MuSCs, causing a shift from a reversible dormant state to a permanently quiescent state, which compromises the regenerative capacity of skeletal muscles (Pedro et al., 2021).
2.2 The NAD+ salvage pathway has been shown to ameliorate sarcopenia and its underlying mechanisms
NAD+ was one of the most prominent metabolites that decreased in older adults, and this decline was even more pronounced in impaired older adults (Janssens et al., 2022). This outcome is a consequence of both reduced synthesis efficiency and increased consumption. Across various tissues in humans and mice, the level of NAMPT in the NAD+ salvage pathway declines with age (De Guia et al., 2019; Stein and Imai, 2014; Xing et al., 2019), resulting in decreased synthesis of NAD+. Furthermore, as the aging process progresses, the accumulation of DNA damage, inflammation, and oxidative stress intensifies, leading to an enhancement in the activity of NAD+ consuming enzymes such as CD38 and PARPs (Covarrubias et al., 2020; Goody and Henry, 2018; Khaidizar et al., 2021; Schultz and Sinclair, 2016; Silva et al., 2023). Enhancing NAD+ metabolism has emerged as a viable therapeutic approach to mitigate age-related conditions and extend lifespan, as supported by numerous studies (Gerdts et al., 2015; Krafczyk and Klotz, 2022; Mouchiroud et al., 2013; Tzameli, 2012). Lifelong overexpression of NAMPT can maintain NAD+ content and functionality in the skeletal muscle of aged mice (Frederick et al., 2016). We summarize the mechanisms by which the NAMPT salvage pathway improves aging skeletal muscle, including alleviating oxidative stress, maintaining the stability of the NAD+ pool, promoting autophagy, reducing chronic low-grade inflammation, enhancing the quantity and function of MuSCs, and improving the formation of neuromuscular junctions.
Increasing NAD+ enhances the function of silent information regulator factor (SIRT1) (Khaidizar et al., 2017), which activates the forkhead box proteins (FoxOs)pathway (Olmos et al., 2013), increases levels of antioxidant enzymes like Superoxide Dismutase 2 (SOD2), strengthens antioxidant defense mechanisms, reduces ROS (Zhang et al., 2023), mitigates oxidative stress damage, and therefore retards the aging process in skeletal muscle. Activated SIRT1 acts as an antioxidant by suppressing NF-κB expression while boosting levels of peroxisome proliferator-activated receptor γ coactivator 1α(PGC-1α) and nuclear factor erythroid 2-related factor 2 (Nrf2), which are key regulators of cellular defense against oxidative stress (Tzameli, 2012). The malate-aspartate shuttle (MAS), essential for the exchange between mitochondrial NAD+ and cytoplasmic NADH (Yang et al., 2007), stabilizes mitochondrial NAD+ during increased energy demands, facilitated by PGC-1α activation in skeletal muscle (Kang and Li Ji, 2012). The mitochondrial unfolded protein response (UPRmt) is crucial for maintaining mitochondrial function and homeostasis. Enhancing NAD+ in skeletal muscle models activates UPRmt, balancing mitochondrial protein systems and improving function (Mouchiroud et al., 2013). The NAD+-dependent enzyme SIRT1 deacetylates LC3 at K49 and K51 sites, enabling LC3 to interact with nucleoproteins and translocate to the cytoplasm, where it binds with autophagy factors such as ATG7, thereby promoting autophagy in skeletal muscle (Huang et al., 2015). Adenosine 5‘-monophosphate (AMP)-activated protein kinase (AMPK) is a key initiator of autophagy (Ge et al., 2022), and SIRT1 can activate AMPK (Gao et al., 2020; Ruderman et al., 2010). AMPK, in turn, activates Unc-51-like autophagy activating kinase 1 (ULK1) through phosphorylation. ULK1 then activates downstream molecules such as Bcl-2-interacting coiled-coil protein 1 (Beclin1) and ATGs, thereby promoting the autophagy process (Holczer et al., 2020). Studies have also shown that AMPK protects cells from oxidative stress-induced aging by increasing autophagic flux and enhancing NAD+ levels (Han et al., 2016). The NLR family pyrin domain containing 3(NLRP3) inflammasome is an intracellular signaling complex that produces pro-inflammatory cytokines, such as IL-1β. Reducing NLRP3 inflammasome expression can enhance muscle fiber size and contractility (McBride et al., 2017). SIRT2 inhibits NLRP3 inflammasome activity by deacetylating NLRP3, thereby reducing aging-associated chronic inflammation (He et al., 2020). Oral administration of nicotinamide riboside (NR) can increase skeletal muscle NAD+ levels and reduce circulating inflammatory cytokines in skeletal muscle (Elhassan et al., 2019). The regulation of other inflammatory factors by NAD+ to improve skeletal muscle function is discussed in the section on T2DM in this paper.
Research shows that the NAD+ salvage pathway enhances neuromuscular junctions (NMJ). Administering NMN to increase NAD+ levels improves vesicle count in axons, restores neuronal function, and maintains synaptic connectivity and transmission at NMJs (Oki et al., 2015; Samuel et al., 2020). Supplementing with NAMPT to increase NAD+ levels can boost MuSCs counts (Hongbo et al., 2016), as NAD+ promotes SIRT1 overexpression, which prevents aging of these cells (Ma et al., 2017), aiding in repair and maintaining the integrity of NMJs (Larouche et al., 2021). The TIR domain of SARM1 can cause axon damage, but overexpressing NAMPT in dorsal root ganglion neurons counters this effect, preventing axon degeneration and cell death (Gerdts et al., 2015). Overexpression of NAMPT also protects against glutamate excitotoxicity and neuronal apoptosis due to oxygen-glucose deprivation, thereby enhancing neuroprotection (Wang et al., 2016). Restoring the number and function of quiescent muscle stem cells in aging skeletal muscle helps improve sarcopenia. The NAD+-dependent SIRT1 deacetylates substrates such as histone H4K16ac, regulating gene expression and maintaining the quiescent state of stem cells (Ryall et al., 2015). NR supplementation can increase the number of MuSCs and enhance their self-renewal capacity (Hongbo et al., 2016). Paired Box 7(PAX7)is essential for maintaining the regenerative function of adult muscle stem cells (Sambasivan et al., 2011). SIRT2 deacetylates PAX7, promoting muscle stem cell self-renewal, inhibiting differentiation, and maintaining the undifferentiated state of MuSCs (Sincennes et al., 2021). Studies in twins have shown that NR promotes the activation of MuSCs from differentiation and fusion to formation of muscle fibers (Lapatto et al., 2023). In conclusion, the NAD+ salvage pathway holds therapeutic potential for treating sarcopenia by reversing pathogenic processes, as depicted in Figure 2.
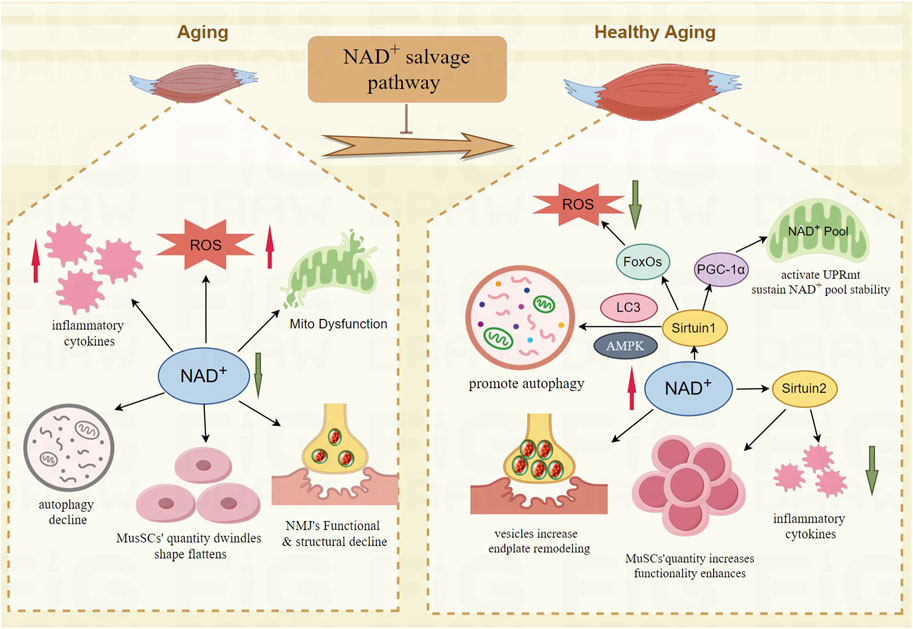
Figure 2. The mechanism of NAD+ salvage pathway improve the function of skeletal muscle in aging. NAD+-Sirtuin1- FoxOs mitigates oxidative stress damage; NAD+-Sirtuin1- PGC-1α improves the mitochondrial function; NAD+-Sirtuin1-AMPK promotes autophagy; NAD+-Sirtuin2 reduces chronic low-grade inflammation and enhances the quantity and functionality of MuSCs; NAD+ increases vesicles, remodels endplate of NMJ.
3 NAD+ salvage pathway in improving T2DM skeletal muscle pathology
3.1 Decreased skeletal muscle contractile function in T2DM
Current epidemiological data shows approximately 387 million adults worldwide are affected by diabetes, with projections suggesting this number will increase to 693 million by 2045 (Cole and Florez, 2020). Type 2 diabetes (T2DM) is characterized by elevated blood sugar levels, primarily due to impaired β-cell function, leading to inadequate insulin secretion or reduced insulin sensitivity. T2DM affects skeletal muscles through insulin resistance, chronic inflammation, advanced glycation end products, and increased oxidative stress, all of which undermine muscle structure, function, and mass (Chen et al., 2023). This results in muscle loss, decreased quality of life, and a higher risk of disability or death (Kawada, 2021), with diabetics having a 1.5–2 times greater risk of muscle loss than non-diabetics (Izzo et al., 2021). GLUT4, a glucose transporter, manages about 80% of glucose uptake, mainly in skeletal muscles (Son et al., 2017). In T2DM, impaired insulin signaling prevents GLUT4 from effectively transporting glucose, reducing muscle glycogen synthesis and contributing to insulin resistance in muscle tissues. High glucose levels lead to fat accumulation in skeletal muscles, activating protein kinase C-epsilon (PKCε) and worsening insulin resistance (Meex et al., 2019). Increased fat in muscles also releases fatty and inflammatory molecules (Donath and Shoelson, 2011), promoting chronic inflammation, which impairs muscle formation, accelerates muscle breakdown, and causes muscle wasting (Cole and Florez, 2020). Hyperglycemia affects neurons by decreasing their responsiveness to electrical stimuli and altering action potential conduction at the neuromuscular junctions. Additionally, it reduces sarco/endoplasmic reticulum Ca2+-ATPase (SERCA) levels (Bayley et al., 2016), impairing skeletal muscle contraction.
3.2 NAD+ salvage pathways improve the mechanism of skeletal muscle energy metabolism in T2DM
Nicotinamide phosphoribosyltransferase (NAMPT), an adipokine resembling insulin, plays a vital role in the NAD+ salvage pathway, which is crucial for the function of pancreatic islet β-cells and is closely linked to glucose and insulin metabolism (Revollo et al., 2007; Yoshino et al., 2011). Research indicates that NMN supplementation can enhance insulin secretion and sensitivity in diabetic mice by improving the NAD+ synthesis pathway (Yoshino et al., 2011). Additionally, elevating SIRT1 specifically in the pancreatic islet β-cells of C57BL/6 mice enhances insulin secretion, improves glucose tolerance, and increases insulin sensitivity (Moynihan et al., 2005).NMN supplementation significantly enhances muscle insulin signaling in prediabetic women, improving skeletal muscle insulin sensitivity and increasing glucose uptake and metabolism in skeletal muscle (Yoshino et al., 2021). NR ameliorates insulin resistance in the skeletal muscle of high-fat diet (HFD) mice by activating the AMPK signaling pathway, which inhibits oxidative stress and enhances mitochondrial function (Li et al., 2023). The NAD+-dependent enzyme SIRT1 regulates AMPK activity (Imi et al., 2023; Liu and Chang, 2018), which phosphorylates Histone Deacetylase 5 (HDAC5) at Ser259 and Ser498, reducing HDAC5 binding to the GLUT4 gene promoter. This increases GLUT4 gene expression and improves skeletal muscle insulin sensitivity (McGee et al., 2008). AMPK also reduces inflammation and insulin resistance in the skeletal muscle of HFD-fed mice, thereby improving skeletal muscle function in T2DM (Zhao et al., 2018). In the skeletal muscle-specific NAMPT knockout mouse model, k-means clustering analysis was conducted on all detected genes, revealing a distinct cluster associated with inflammation and immune response-related genes (Frederick et al., 2016). The activation of the nuclear factor kappa-B (NF-κB) pathway, a key player in inflammation (van der Heiden et al., 2010), triggers the upregulation of muscle-specific E3 ubiquitin ligase (MuRF1) and cytokines such as TNF, IL-6, and IL-1β. This increases muscle protein breakdown and inhibits protein synthesis, disrupting protein balance and leading to muscle atrophy (Cai et al., 2004). The activity of NF-κB in skeletal muscle of T2DM patients is 2.7 times higher than that of normal glucose tolerant subjects (Tantiwong et al., 2010). Inhibiting NF-κB can significantly reduce muscle loss. Supplementation with NAMPT enhances NAD+ synthesis, thereby increasing SIRT1 activity (Stein and Imai, 2012). This activity includes the deacetylation of RelA AcK310 by SIRT1, which inhibits NF-κB transcriptional activity (Yeung et al., 2004), decreases inflammation in skeletal muscles, and improves muscle function in diabetes.
Patients with type 2 diabetes and obesity typically exhibit increased fat accumulation around organs, especially in skeletal muscles and liver. Fat accumulation in the skeletal muscle of T2DM patients leads to decreased muscle strength and function (Almurdhi et al., 2016). Adiponectin, a key gene in glucose metabolism (Banks et al., 2015), acts as an insulin sensitizer, increasing insulin sensitivity in skeletal muscle, thereby enhancing glucose uptake and metabolism (Ceddia et al., 2005; Liu et al., 2009). Targeted deletion of NAMPT in mouse adipocytes results in systemic insulin resistance, reduced fat metabolism, lower adiponectin levels, and decreased metabolic efficiency (Stromsdorfer et al., 2016). PPARγ is crucial for fat synthesis, and its complete deletion in mice leads to the loss of both white and brown adipose tissues, causing severe metabolic issues (Gilardi et al., 2019). Additionally, PPARγ suppresses NF-κB activation, which otherwise enhances muscle inflammation (Huang and Glass, 2010). Supplementing PPARγ helps maintain insulin responsiveness in peripheral tissues, increases glucose uptake and utilization in muscles, improves lipid metabolism, and lowers triglyceride levels (Ji et al., 2022). Specific overexpression of NAM in adipose tissue results in a 32-fold increase in NAD+ (Luo et al., 2022), levels and a corresponding rise in carnitine content. This enhancement promotes the transport of fatty acids to mitochondria for oxidative breakdown (Rebouche and Seim, 1998). Reduced NAD+ levels cause phosphorylation of PPARγ at Ser273 in adipose tissue, which disrupts PPARγ's role in lipid metabolism and inhibits adiponectin function, thereby affecting glucose metabolism (Stromsdorfer et al., 2016). Deacetylating PPARγ at K268 and K293 promotes the recruitment of coactivators that facilitate the browning of white adipose tissue, thereby increasing insulin sensitivity, enhancing energy expenditure, and reducing obesity (Ji et al., 2022). P7C3, an orally active neuroprotective agent, targets the NAMPT enzyme, enhancing the NAD+ salvage pathway. This increases insulin sensitivity, boosts mitochondrial fatty acid β-oxidation in skeletal muscle, and improves energy metabolism in T2DM mice (Ravikumar et al., 2022) which is depicted in Figure 3.
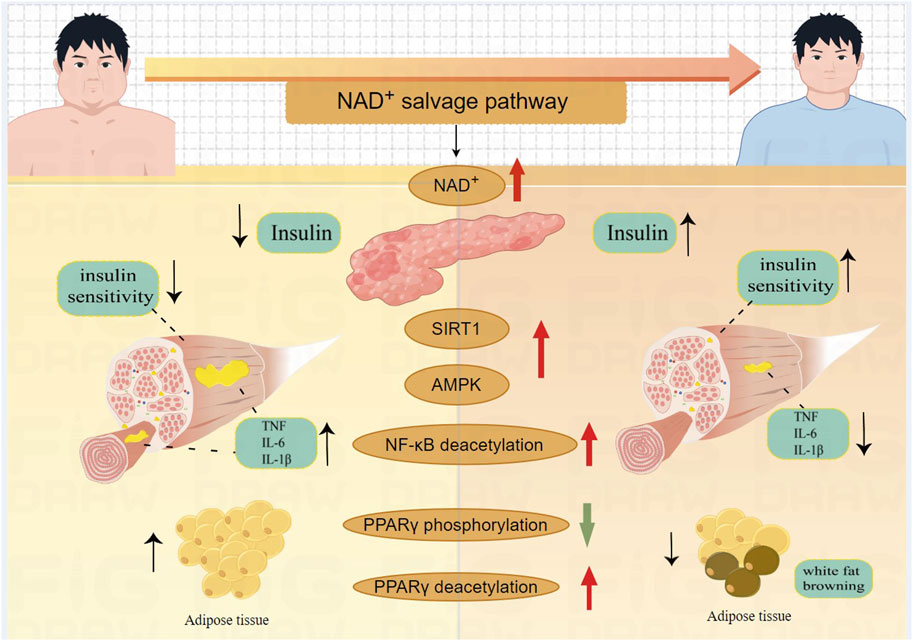
Figure 3. The mechanism of NAD+ salvage pathway improve the function of skeletal muscle in T2DM. NAD+ enhances insulin secretion; NAD+-Sirtuin1- NF-κB decreases inflammation in skeletal muscles; NAD+-Sirtuin1-AMPK improves skeletal muscle insulin sensitivity; NAD+ decreases PPARγ phosphorylation, promotes fat metabolism; NAD+-Sirtuin1 deacetylates PPARγ to induce white fat browning.
4 The application of the NAD+ salvage pathway in promoting skeletal muscle injury recovery
Rehabilitation and treatment of skeletal muscle injuries remain significant challenges in sports physiology, medicine, and rehabilitation. There is substantial evidence that the NAMPT-mediated NAD+ salvage pathway plays a crucial role in the structural and functional recovery of injured skeletal muscles. Studies suggest that extracellular vesicles from young individual’s adipose-derived mesenchymal stem cells (ADMSC young-EVs) are promising for treating tendinopathy by potentially increasing NAMPT expression in tendon cells and macrophages (Wu et al., 2023). Increasing NAMPT expression activates pathways such as NAMPT-SIRT1-PPARγ-PGC-1α, improving mitochondrial functions, reducing cellular damage, and slowing tendon deterioration. Additionally, the activation of the NAMPT-SIRT1-NF-κB/NLRP3 pathway rejuvenates macrophage phagocytic capabilities, lowers pro-inflammatory factor levels, and alleviates tendinopathy. NAMPT enhances M2 macrophage polarization, increasing their proportion, which helps reduce inflammation and promote tendon repair in tendinopathy (Wu et al., 2023). Disuse atrophy occurs when skeletal muscle atrophies due to reduced activity following injury. In models of acute supraspinatus and extensor digitorum longus muscle tears, injecting exogenous NAMPT improves citrate synthase activity and mitochondrial function, thereby enhancing muscle regeneration capabilities (Yao et al., 2023). Dhanushika et al. (2021) discovered in a transgenic zebrafish model that following skeletal muscle injury, about 34% of macrophages quickly migrated to the injury site. A particular subset secretes NAMPT that binds to CCR5 on muscle stem cells (MuSCs), enhancing their repair and proliferation. Research has shown that knocking down SIRT1 disrupts sarcolemma repair and vesicle dynamics at the injury site, increasing vulnerability to further mechanical damage (Fujiwara et al., 2019). Thus, enhancing the NAD+ salvage pathway and boosting SIRT1 expression are believed to protect against skeletal muscle damage and hasten sarcolemma recovery.
Exercise is essential for stimulating the regeneration and repair of muscle fibers, and the combination of exercise with NAD+ boosters holds promising potential for restoring the function of injured skeletal muscle. Exercise and NAD+ boosters may simultaneously enhance the recovery of injured skeletal muscle by improving MuSCs and mitochondrial function. In rodents and humans, exercise and muscle contraction activate AMPK in skeletal muscle (Jørgensen et al., 2006; Winder and Hardie, 1996). Studies have shown that the absence of AMPKα1 leads to a reduction in satellite cell numbers and decreased expression of myogenic factors such as Myf5 and Myogenin (Fu et al., 2015; Thomson, 2018). The AMPKα1-LDH pathway regulates the activation, proliferation, differentiation, and self-renewal of MuSCs (Theret et al., 2017). AMPK enhances sirtuin activity (Cantó et al., 2009; Cantó et al., 2010), and NAD+ boosters can also activate sirtuins. High expression of SIRT1 facilitates the recovery of injured muscle function (Myers et al., 2019), while SIRT2 deacetylates PAX7, promoting the self-renewal of MuSCs (Sincennes et al., 2021). AMPK and Sirtuins together can increase PGC-1α activity, which improves mitochondrial function and enhances skeletal muscle regeneration (Wu et al., 2023). The crosstalk between AMPK and Sirtuins offers additional theoretical possibilities for the use of exercise and NAD+ boosters in the recovery of injured skeletal muscle. However, the practicality of this approach requires further experimental validation. Furthermore, different types and intensities of exercise also promote NAMPT and NAD+ levels, which will be discussed in the next section.
5 Exercise regulate NAD+ salvage pathway to improve skeletal muscle function strategy
The benefits of regular exercise on skeletal muscles are well-documented. While studies on exercise’s impact on skeletal muscle NAMPT levels are limited, available research indicates that elderly individuals experience a more pronounced increase in NAMPT and NAD+ levels from exercise than younger people. Different types of exercise positively affect NAMPT levels, though the specific molecular mechanisms driving these increases are not yet fully understood. Exercise enhances skeletal muscle glycolysis and aerobic metabolism, leading to ATP breakdown into ADP and Pi, releasing energy and producing AMP. This increase in AMP and ADP levels stimulates AMPK phosphorylation at the Thr172 site, enhancing AMPK activity (Hardie et al., 2012). Administering an AMPK activator raises skeletal muscle NAMPT protein levels (Brandauer et al., 2013; Ratia et al., 2023). Furthermore, the level of AMPK activity correlates with exercise intensity, with greater intensity causing more AMPK activation and increased phosphorylation of AMPK (Rothschild et al., 2022). Research suggests that more intense exercise might more effectively elevate NAMPT levels. It is theorized that exercise boosts NAMPT levels primarily through the AMPK-NAMPT pathway, as indicated in Table 1.
6 NAD+ boosters
For individuals unable to perform physical exercise due to injury or chronic disease, NAD+ boosters might serve as exercise mimetics, activating similar biochemical pathways that are engaged during physical exercise, thus maintaining muscle function and promoting metabolic health. Modulators of enzymes of the NAD+ biosynthesis pathway, NAD+ precursors and NAD+ consuming enzyme inhibitors are main NAD+ boosters. We will discuss the prospects, side effects, and current status of clinical transformation of NAMPT agonists, NAD+ precursors and NAD+-consuming enzyme inhibitors to provide a more balanced and unbiased perspective on NAD+ metabolism.
6.1 NAMPT agonists
NAMPT agonists can enhance the efficiency of the body’s salvage synthesis pathway to produce NMN, and even with small or intermittent supplementation of NMN or NR, they can maintain high levels of NAD+ in the long term. Therefore, many studies have attempted to explore the types and potential roles of NAMPT agonists. P7C3 is the first discovered activator of NAMPT. The active variants of P7C3 can enhance the activity of purified NAMPT, and their promotion of NMN production is dose-dependent (Wang et al., 2014). Research has shown that P7C3-mediated NAMPT activation improves insulin sensitivity and muscle function in obese mice, reducing diabetic symptoms (Ravikumar et al., 2022). The exact mechanisms of how P7C3 activates NAMPT or competes with its inhibitors are still under investigation. The synthesis of SBI-797812, an NAMPT activator, involved modifying the GNI-50 molecule by moving the pyridine group from the third to the fourth position. This change enhances NAMPT’s catalytic efficiency, increasing NMN synthesis by 2.1 times (Gardell et al., 2019). SBI-797812 also counteracts the inhibitory effects of NAD+ on NAMPT by binding to its rear channel, boosting NAMPT activity and increasing NAD+ levels (Ratia et al., 2023). NAT and its active variant NAT-5r, potent NAMPT activators, interact through a hydrogen bond with NAMPT’s K189 residue, enhancing its catalysis and countering FK866-induced cell death (Hong et al., 2022). High-throughput screening has identified several compounds that enhance NAMPT function, including NAMPT positive allosteric modulators A1 (NP-A1) (Zhengnan et al., 2023). These modulators increase NAMPT activity by 1.6–2.6 times, binding to its back channel (Ratia et al., 2023). N-PAMs reduce NAD+'s inhibitory effect on NAMPT, enhancing its function. Natural activators like notoginseng leaf triterpenes (PNGL) also stimulate NAMPT (Xie et al., 2020), activating NAMPT-NAD+-SIRT1/2/3-Foxo3a-MnSOD/PGC-1α pathways that improve mitochondrial function and prevent mitochondrial damage. The hypertension peptide IRW increases muscle cell NAMPT content significantly (Bhullar et al., 2021). Additionally, substances like low-dose nicotine enhance NAMPT activity by facilitating its interaction with SIRT1, boosting NAD+ and β-NMN levels, and improving metabolic function in aging tissues (Liang et al., 2023).
Although NAMPT agonists have shown therapeutic potential in laboratory studies, they have revealed certain safety concerns in clinical trials, their therapeutic effects do not yet meet clinical needs, and the progress of clinical trials has been slow (Wen et al., 2024). Moreover, NAMPT regulates macrophage survival and pro-inflammatory activity, contributing to the modulation of the tumor inflammatory microenvironment and promoting tumor cell metastasis (Lucena-Cacace et al., 2018; Piacente et al., 2017). NAMPT activity appears to be tightly controlled by NMN and NAD+ through strict feedback regulation (Burgos and Schramm, 2008; Takahashi et al., 2010). Developing NAMPT agonists that can specifically enhance NAD+ production without affecting other functions, such as immune response regulation and cancer cell metabolism, remains a significant challenge.
6.2 NAD+ precursors
NAD+ precursors, such as NA, NMN, NR, and NAM, have gained significant attention for NAD+ supplementation and have been shown in both animal and human studies to extend lifespan, enhance muscle regeneration, improve mitochondrial and stem cell function, boost glucose metabolism, and enhance cardiovascular function. The specific effects of these precursors on the body are detailed in several excellent reviews (Iqbal and Nakagawa, 2024; Keisuke and Takashi, 2023; Lautrup et al., 2024; Montllor-Albalate et al., 2021; Reiten et al., 2021). However, different NAD+ precursors exhibit varying effects depending on the experimental subjects. For instance, postmenopausal overweight or obese women with prediabetes who took NMN(250 mg/day) for 10 weeks showed significant improvements in muscle insulin sensitivity (Yoshino et al., 2021). In contrast, healthy, obese, sedentary men aged 40–70 who received NR (2000 mg/day) for 12 weeks did not experience improvements in insulin sensitivity, endogenous glucose production, glucose handling, or oxidation (Dollerup et al., 2018). Older men with diabetes and impaired physical function did not see improvements in muscle strength after taking NMN 250 (mg/day) for 24 weeks (Akasaka et al., 2023). Patients with mitochondrial myopathy who took NA 750–1,000 (mg/day) for four months showed improvements in muscle NAD+ levels, disease symptoms, and muscle metabolism (Pirinen et al., 2020). These variations in outcomes may be associated with factors such as gut microbiota, dose dependency, and individual differences. Currently, NAD+ precursors such as NA, NAM, NR, and NMN are used clinically and have demonstrated certain levels of safety and bioavailability (Reiten et al., 2021). However, more comprehensive research is needed to evaluate their potential side effects and therapeutic efficacy. The interactions of gut microbiota with NAD+ precursors add complexity to NAD+ metabolism. Optimizing precursor formulations and administration methods could enhance their stability and conversion efficiency in the body. Furthermore, exploring targeted delivery methods of NAD+ precursors to specific organs or tissues could improve clinical trial outcomes.
6.3 NAD+-consuming enzyme inhibitors
NAD+ boosters also include inhibitors of NAD+-consuming enzymes. CD38 inhibitors improve age-related metabolic disorders by reversing tissue NAD+ decline, increasing muscle fiber size, and reducing fibrosis (Tarragó et al., 2018). CD38-deficient mice prevent high-fat diet-induced obesity by activating the SIRT-PGC1α axis through elevated NAD+ levels (Barbosa et al., 2007). The deletion of the PARP gene increases NAD+ content and SIRT1 activity in brown adipose tissue and muscle, enhancing mitochondrial content and oxidative metabolism (Bai et al., 2011). However, using PARP inhibitors could have adverse effects, as PARP is involved in essential cellular processes, and its inhibition may lead to genomic instability (Beneke et al., 2004). The development of NAD+ consumption enzyme inhibitors requires comprehensive consideration of drug safety, its impact on tumor development, the role of inflammation and metabolic regulation, biological rhythms and adaptive responses, individual differences, and optimization of supplementation strategies. These challenges need to be fully addressed in future research.
NAMPT agonists accelerate NAD+ synthesis, NAD+ precursors provide direct supplementation, and consumption inhibitors reduce NAD+ degradation, forming a multifaceted synthesis and protection network that significantly enhances intracellular NAD+ levels. This synergistic approach holds promise as a target for treating diseases and disorders associated with disrupted NAD+ homeostasis.
7 Conclusion
In skeletal muscle, efficient energy metabolism is crucial, and disruptions in NAMPT and NAD+ levels or synthesis significantly impact muscle function. These disturbances are particularly evident in conditions like aging, type 2 diabetes (T2DM), and muscle injuries, where NAMPT-mediated NAD+ salvage pathways are compromised. The NAD+ salvage pathway improves function in aging skeletal muscles by reducing oxidative stress, stabilizing mitochondrial NAD+ pools, promoting autophagy, reducing chronic low-grade inflammation, restoring neuromuscular junction (NMJ) functionality, and enhancing MuSCs quantity and function. Similarly in T2DM, it enhances muscle function by improving insulin secretion, enhancing skeletal muscle insulin sensitivity, reducing inflammation, increasing fatty acid oxidation, and promoting the browning of white adipose tissue. In muscle injury scenarios, the NAD+ salvage pathway facilitates MuSCs proliferation and mitigates mitochondrial dysfunction, thereby enhancing muscle contractility. Exercise similarly exploits this pathway to bolster skeletal muscle functionality. Therefore, manipulating the NAD+ salvage pathway holds significant potential for enhancing skeletal muscle function. NAD+ boosters, in particular, could be effective in treating various muscle dysfunctions and enhancing overall muscle health.
Author contributions
MS: Writing–original draft. FQ: Writing–review and editing. YL: Writing–review and editing. TC: Writing–review and editing. NL: Writing–review and editing. SZ: Writing–original draft, Writing–review and editing.
Funding
The author(s) declare that financial support was received for the research, authorship, and/or publication of this article. This research related to NAD+ and skeletal muscle was funded by Qingdao Postdoctoral Project of China to SZ, grant numbers QDBSH20230102096.
Acknowledgments
The authors would like to acknowledge all the literatures contributed to this field. The authors certify that they comply with the ethical guidelines for authorship and publishing of the Frontiers in Cell and Development Biology.
Conflict of interest
The authors declare that the research was conducted in the absence of any commercial or financial relationships that could be construed as a potential conflict of interest.
Publisher’s note
All claims expressed in this article are solely those of the authors and do not necessarily represent those of their affiliated organizations, or those of the publisher, the editors and the reviewers. Any product that may be evaluated in this article, or claim that may be made by its manufacturer, is not guaranteed or endorsed by the publisher.
References
Agerholm, M., Dall, M., Jensen, B. A. H., Prats, C., Madsen, S., Basse, A. L., et al. (2018). Perturbations of NAD + salvage systems impact mitochondrial function and energy homeostasis in mouse myoblasts and intact skeletal muscle. Am. J. Physiol.-Endocrinol. Metab. 314 (4), E377–E395. doi:10.1152/ajpendo.00213.2017
Alice, P., Margherita, S., Eugenia, M., Alex, I., Mathew, P., Sonia, K., et al. (2016). A robust neuromuscular system protects rat and human skeletal muscle from sarcopenia. Aging 8 (4), 712–729. doi:10.18632/aging.100926
Akasaka, H., Nakagami, H., Sugimoto, K., Yasunobe, Y., Minami, T., Fujimoto, T., et al. (2023). Effects of nicotinamide mononucleotide on older patients with diabetes and impaired physical performance: A prospective, placebo-controlled, double-blind study. Geriatr Gerontol Int. 23 (1), 38–43. doi:10.1111/ggi.14513
Almurdhi, M. M., Reeves, N. D., Bowling, F. L., Boulton, A. J. M., Jeziorska, M., and Malik, R. A. (2016). Reduced lower-limb muscle strength and volume in patients with type 2 diabetes in relation to neuropathy, intramuscular fat, and vitamin D levels. Diabetes Care 39 (3), 441–447. doi:10.2337/dc15-0995
Bai, P., Cantó, C., Oudart, H., Brunyánszki, A., Cen, Y., Thomas, C., et al. (2011). PARP-1 inhibition increases mitochondrial metabolism through SIRT1 activation. Cell Metab. 13 (4), 461–468. doi:10.1016/j.cmet.2011.03.004
Banks, A. S., McAllister, F. E., Camporez, J. P. G., Zushin, P.-J. H., Jurczak, M. J., Laznik-Bogoslavski, D., et al. (2015). An ERK/Cdk5 axis controls the diabetogenic actions of PPARγ. Nature 517 (7534), 391–395. doi:10.1038/nature13887
Barbosa, M. T. P., Soares, S. M., Novak, C. M., Sinclair, D., Levine, J. A., Aksoy, P., et al. (2007). The enzyme CD38 (a NAD glycohydrolase, EC 3.2.2.5) is necessary for the development of diet-induced obesity. FASEB J. 21 (13), 3629–3639. doi:10.1096/fj.07-8290com
Bartnik, B., Juurlink, B., and Devon, R. (2000). Macrophages: their myelinotrophic or neurotoxic actions depend upon tissue oxidative stress. Mult. Scler. 6, 37–42. doi:10.1177/135245850000600108
Basse, A. L., Agerholm, M., Farup, J., Dalbram, E., Nielsen, J., Ørtenblad, N., et al. (2021). Nampt controls skeletal muscle development by maintaining Ca2+ homeostasis and mitochondrial integrity. Mol. Metab. 53, 101271. doi:10.1016/j.molmet.2021.101271
Bayley, J. S., Pedersen, T. H., and Nielsen, O. B. (2016). Skeletal muscle dysfunction inthe db/db mouse model of type 2 diabetes. Muscle Nerve 54 (3), 460–468. doi:10.1002/mus.25064
Beneke, S., Diefenbach, J., and Bürkle, A. (2004). Poly(ADP-ribosyl)ation inhibitors: promising drug candidates for a wide variety of pathophysiologic conditions. Int. J. Cancer 111 (6), 813–818. doi:10.1002/ijc.20342
Bhullar, K. S., Son, M., Kerek, E., Cromwell, C. R., Wingert, B. M., Wu, K., et al. (2021). Tripeptide IRW upregulates NAMPT protein levels in cells and obese C57bl/6J mice. J. Agric. Food Chem. 69 (5), 1555–1566. doi:10.1021/acs.jafc.0c07831
Brandauer, J., Vienberg, S. G., Andersen, M. A., Ringholm, S., Risis, S., Larsen, P. S., et al. (2013). AMP-activated protein kinase regulates nicotinamide phosphoribosyl transferase expression in skeletal muscle. J. Physiol. 591 (20), 5207–5220. doi:10.1113/jphysiol.2013.259515
Briston, T., Selwood, D. L., Szabadkai, G., and Duchen, M. R. (2019). Mitochondrial permeability transition: a molecular lesion with multiple drug targets. Trends Pharmacol. Sci. 40 (1), 50–70. doi:10.1016/j.tips.2018.11.004
Burgos, E. S., and Schramm, V. L. (2008). Weak coupling of ATP hydrolysis to the chemical equilibrium of human nicotinamide phosphoribosyltransferase. Biochemistry 47 (42), 11086–11096. doi:10.1021/bi801198m
Cai, D., Frantz, J. D., Tawa, N. E., Melendez, P. A., Oh, B.-C., Lidov, H. G. W., et al. (2004). IKKbeta/NF-kappaB activation causes severe muscle wasting in mice. Cell 119, 285–298. doi:10.1016/j.cell.2004.09.027
Cantó, C., Gerhart-Hines, Z., Feige, J. N., Lagouge, M., Noriega, L., Milne, J. C., et al. (2009). AMPK regulates energy expenditure by modulating NAD+ metabolism and SIRT1 activity. Nature 458 (7241), 1056–1060. doi:10.1038/nature07813
Cantó, C., Jiang, L. Q., Deshmukh, A. S., Mataki, C., Coste, A., Lagouge, M., et al. (2010). Interdependence of AMPK and SIRT1 for metabolic adaptation to fasting and exercise in skeletal muscle. Cell Metab. 11 (3), 213–219. doi:10.1016/j.cmet.2010.02.006
Cantó, C., Sauve, A. A., and Bai, P. (2013). Crosstalk between poly(ADP-ribose) polymerase and sirtuin enzymes. Mol. Asp. Med. 34 (6), 1168–1201. doi:10.1016/j.mam.2013.01.004
Carnio, S., LoVerso, F., Baraibar, M. A., Longa, E., Khan, M. M., Maffei, M., et al. (2014). Autophagy impairment in muscle induces neuromuscular junction degeneration and precocious aging. Cell Rep. 8 (5), 1509–1521. doi:10.1016/j.celrep.2014.07.061
Ceddia, R. B., Somwar, R., Maida, A., Fang, X., Bikopoulos, G., and Sweeney, G. (2005). Globular adiponectin increases GLUT4 translocation and glucose uptake but reduces glycogen synthesis in rat skeletal muscle cells. Diabetologia 48 (1), 132–139. doi:10.1007/s00125-004-1609-y
Chee, C. M., Anabel, S., F, J. P., Xuan, W. S. S., and Jason, H. (2022). Exercise increases the release of NAMPT in extracellular vesicles and alters NAD+ activity in recipient cells. Aging Cell 21 (7), 13647–13652. doi:10.1111/acel.13647
Chen, H., Huang, X., Dong, M., Wen, S., Zhou, L., and Yuan, X. (2023). The association between sarcopenia and diabetes: from pathophysiology mechanism to therapeutic strategy. Diabetes Metab. Syndr. Obes. 16, 1541–1554. doi:10.2147/DMSO.S410834
Chen, L.-K., Liu, L.-K., Woo, J., Assantachai, P., Auyeung, T.-W., Bahyah, K. S., et al. (2014). Sarcopenia in asia: consensus report of the asian working group for sarcopenia. J. Am. Med. Dir. Assoc. 15 (2), 95–101. doi:10.1016/j.jamda.2013.11.025
Chen, M., Wang, Y., Deng, S., Lian, Z., and Yu, K. (2022). Skeletal muscle oxidative stress and inflammation in aging: focus on antioxidant and anti-inflammatory therapy. Front. Cell Dev. Biol. 10, 964130. doi:10.3389/fcell.2022.964130
Sincennes, M.-C., Brun, C. E., Lin, A. Y. T., Rosembert, T., Datzkiw, D., and Saber, J. (2021). Acetylation of PAX7 controls muscle stem cell self-renewal and differentiation potential in mice. Nat. Commun. 12 (1), 3253–3267. doi:10.1038/s41467-021-23577-z
Cole, J. B., and Florez, J. C. (2020). Genetics of diabetes mellitus and diabetes complications. Nat. Rev. Nephrol. 16 (7), 377–390. doi:10.1038/s41581-020-0278-5
Costford, S. R., Brouwers, B., Hopf, M. E., Sparks, L. M., Dispagna, M., Gomes, A. P., et al. (2018). Skeletal muscle overexpression of nicotinamide phosphoribosyl transferase in mice coupled with voluntaryexercise augments exercise endurance. Mol. Metab. 7, 1–11. doi:10.1016/j.molmet.2017.10.012
Coto Montes, A., Boga, J. A., Bermejo Millo, C., Rubio González, A., Potes Ochoa, Y., Vega Naredo, I., et al. (2017). Potential early biomarkers of sarcopenia among independent older adults. Maturitas 104, 117–122. doi:10.1016/j.maturitas.2017.08.009
Covarrubias, A. J., Kale, A., Perrone, R., Lopez-Dominguez, J. A., Pisco, A. O., Kasler, H. G., et al. (2020). Senescent cells promote tissue NAD+ decline during ageing via the activation of CD38+ macrophages. Nat. Metab. 2 (11), 1265–1283. doi:10.1038/s42255-020-00305-3
De Guia, R. M., Agerholm, M., Nielsen, T. S., Consitt, L. A., Søgaard, D., Helge, J. W., et al. (2019). Aerobic and resistanceexercise training reverses age-dependent decline in NAD + salvage capacity in human skeletal muscle. Physiol. Rep. 7 (12), e14139. doi:10.14814/phy2.14139
Dhanushika, R. (2021). Macrophages provide a transient muscle stem cell niche via NAMPT secretion. Nature 591 (7849), 281–314. doi:10.1038/s41586-021-03199-7
Dollerup, O. L., Christensen, B., Svart, M., Schmidt, M. S., Sulek, K., Ringgaard, S., et al. (2018). A randomized placebo-controlled clinical trial of nicotinamide riboside in obese men: safety, insulin-sensitivity, and lipid-mobilizing effects. Am. J. Clin. Nutr. 108 (2), 343–353. doi:10.1093/ajcn/nqy132
Donath, M. Y., and Shoelson, S. E. (2011). Type 2 diabetes as an inflammatory disease. Nat. Rev. Immunol. 11 (2), 98–107. doi:10.1038/nri2925
Elhassan, Y. S., Kluckova, K., Fletcher, R. S., Schmidt, M. S., Garten, A., Doig, C. L., et al. (2019). Nicotinamide riboside augments the aged human skeletal muscle NAD+ metabolome and induces transcriptomic and anti-inflammatory signatures. Cell Rep. 28 (7), 1717–1728. doi:10.1016/j.celrep.2019.07.043
Essuman, K., Summers, D. W., Sasaki, Y., Mao, X., DiAntonio, A., and Milbrandt, J. (2017). The SARM1 toll/interleukin-1 receptor domain possesses intrinsic NAD + cleavage activity that promotes pathological axonal degeneration. Neuron 93 (6), 1334–1343. doi:10.1016/j.neuron.2017.02.022
Ferrucci, L., Harris, T. B., Guralnik, J. M., Tracy, R. P., Corti, M., Cohen, H. J., et al. (1999). Serum IL-6 level and the development of disability in older persons. J. Am. Geriatr. Soc. 47 (6), 639–646. doi:10.1111/j.1532-5415.1999.tb01583.x
Frederick, D. W., Loro, E., Liu, L., Davila, A., Chellappa, K., Silverman, I. M., et al. (2016). Loss of NAD homeostasis leads to progressive and reversible degeneration of skeletal muscle. Cell Metab. 24 (2), 269–282. doi:10.1016/j.cmet.2016.07.005
Friebe, D., Neef, M., Kratzsch, J., Erbs, S., Dittrich, K., Garten, A., et al. (2011). Leucocytesare a major source of circulating nicotinamide phosphoribosyltransferase (NAMPT)/pre-B cell colony (PBEF)/visfatin linking obesity and inflammation in humans. Diabetologia 54 (5), 1200–1211. doi:10.1007/s00125-010-2042-z
Fu, X., Zhu, M.-J., Dodson, M. V., and Du, M. (2015). AMP-Activated protein kinase stimulates warburg-like glycolysis and activation of satellite cells during muscle regeneration. J. Bio. Chem. 290 (44), 26445–26456. doi:10.1074/jbc.M115.665232
Fujiwara, D., Iwahara, N., Sebori, R., Hosoda, R., Shimohama, S., Kuno, A., et al. (2019). SIRT1 deficiency interferes with membrane resealing after cell membrane injury. PLOS ONE 14 (6), e0218329. doi:10.1371/journal.pone.0218329
Fukuhara, A., Matsuda, M., Nishizawa, M., Segawa, K., Tanaka, M., Kishimoto, K., et al. (2005). Visfatin: a protein secreted by visceral fat that mimics the effects of insulin. Science 307 (5708), 426–430. doi:10.1126/science.1097243
Fulop, T., Larbi, A., Dupuis, G., Le Page, A., Frost, E. H., Cohen, A. A., et al. (2018). Immunosenescence and inflamm-aging as two sides of the same coin: friends or foes? Front. Immunol. 8, 1960–1971. doi:10.3389/fimmu.2017.01960
Fulop, T., Larbi, A., Pawelec, G., Khalil, A., Cohen, A. A., Hirokawa, K., et al. (2021). Immunology of aging: the birth of inflammaging. Clin. Rev. Allerg. Immu 64 (2), 109–122. doi:10.1007/s12016-021-08899-6
Gao, K., Niu, J., and Dang, X. (2020). Neuroprotection of melatonin on spinal cord injury by activating autophagy and inhibiting apoptosis via SIRT1/AMPK signaling pathway. Biotechnol. Lett. 42 (10), 2059–2069. doi:10.1007/s10529-020-02939-5
Gardell, S. J., Hopf, M., Khan, A., Dispagna, M., Hampton Sessions, E., Falter, R., et al. (2019). Boosting NAD+ witha small molecule that activates NAMPT. Nat. Commun. 10 (1), 3241. doi:10.1038/s41467-019-11078-z
Ge, Y., Zhou, M., Chen, C., Wu, X., and Wang, X. (2022). Role of AMPK mediated pathways in autophagy and aging. Biochimie 195, 100–113. doi:10.1016/j.biochi.2021.11.008
Gerdts, J., Brace, E. J., Sasaki, Y., DiAntonio, A., and Milbrandt, J. (2015). SARM1 activation triggers axon degeneration locally via NAD + destruction. Science 348 (6233), 453–457. doi:10.1126/science.1258366
Gilardi, F., Winkler, C., Quignodon, L., Diserens, J.-G., Toffoli, B., Schiffrin, M., et al. (2019). Systemic PPARγ deletion in mice provokes lipoatrophy, organomegaly, severe type 2 diabetes and metabolic inflexibility. Metabolism 95, 8–20. doi:10.1016/j.metabol.2019.03.003
Goody, M. F., and Henry, C. A. (2018). A need for NAD+ in muscle development, homeostasis, and aging. Skelet. Muscle 8 (1), 9–22. doi:10.1186/s13395-018-0154-1
Han, X., Tai, H., Wang, X., Wang, Z., Zhou, J., Wei, X., et al. (2016). AMPK activation protects cells from oxidative stress-induced senescence via autophagic flux restoration and intracellular NAD+ elevation. Aging Cell 15, 416–427. doi:10.1111/acel.12446
Hardie, D. G., Ross, F. A., and Hawley, S. A. (2012). AMPK: a nutrient and energy sensor that maintains energy homeostasis. Nat. Rev. Mol. Cell Biol. 13 (4), 251–262. doi:10.1038/nrm3311
He, M., Chiang, H.-H., Luo, H., Zheng, Z., Qiao, Q., Wang, L., et al. (2020). An acetylation switch of the NLRP3 inflammasome regulates aging-associated chronic inflammation and insulin resistance. Cell Metab. 31 (3), 580–591. doi:10.1016/j.cmet.2020.01.009
Hepple, R. T. (2014). Mitochondrial involvement and impact in aging skeletal muscle. Front. Aging Neurosci. 6, 211–233. doi:10.3389/fnagi.2014.00211
Holczer, M., Hajdú, B., Lőrincz, T., Szarka, A., Bánhegyi, G., and Kapuy, O. (2020). Fine-tuning of AMPK–ULK1–mTORC1 regulatory triangle is crucial for autophagy oscillation. Sci. Rep. 10 (1), 17803. doi:10.1038/s41598-020-75030-8
Hong, Y., Minghui, L., Leibo, W., Yumeng, Z., Chou, W., Chenyu, L., et al. (2022). Discovery of small-molecule activators of nicotinamide phosphoribosyltransferase (NAMPT) and their preclinical neuroprotective activity. Cell Res. 32 (6), 570–584. doi:10.1038/s41422-022-00651-9
Hongbo, Z., Dongryeol, R., Yibo, W., Karim, G., Xu, W., Peiling, L., et al. (2016). NAD+ repletion improves mitochondrial and stem cell function and enhances life span in mice. Science 352 (6292), 1436–1443. doi:10.1126/science.aaf2693
Houtkooper, R. H., Cantó, C., Wanders, R. J., and Auwerx, J. (2010). The secret life ofNAD+: an old metabolite controlling new metabolic signaling pathways. Endocr. Rev. 31 (2), 194–223. doi:10.1210/er.2009-0026
Huang, R., Xu, Y., Wan, W., Shou, X., Qian, J., You, Z., et al. (2015). Deacetylation of nuclear LC3 drives autophagy initiation under starvation. Mol. Cell 57 (3), 456–466. doi:10.1016/j.molcel.2014.12.013
Huang, W., and Glass, C. K. (2010). Nuclear receptors and inflammation control: molecular mechanisms and pathophysiological relevance. Arterioscler. Thromb. Vasc. Biol. 30 (8), 1542–1549. doi:10.1161/ATVBAHA.109.191189
Imi, Y., Amano, R., Kasahara, N., Obana, Y., and Hosooka, T. (2023). Nicotinamide mononucleotide induces lipolysis by regulating ATGL expression via the SIRT1-AMPK axis in adipocytes. Biochem. Biophys. Rep. 34, 101476. doi:10.1016/j.bbrep.2023.101476
Iqbal, T., and Nakagawa, T. (2024). The therapeutic perspective of NAD+ precursors in age-related diseases. Biochem. Bioph Res. Co. 702, 149590. doi:10.1016/j.bbrc.2024.149590
Izzo, A., Massimino, E., Riccardi, G., and Della Pepa, G. (2021). A narrative review onSarcopenia in type 2 diabetes mellitus: prevalence and associated factors. Nutrients 13 (1), 183. doi:10.3390/nu13010183
Jang, Y. C., Lustgarten, M. S., Liu, Y., Muller, F. L., Bhattacharya, A., Liang, H., et al. (2010). Increased superoxide in vivo accelerates age-associated muscle atrophy through mitochondrial dysfunction and neuromuscular junction degeneration. FASEB J. 24 (5), 1376–1390. doi:10.1096/fj.09-146308
Jang, Y. C., Rodriguez, K., Lustgarten, M. S., Muller, F. L., Bhattacharya, A., Pierce, A., et al. (2020). Superoxide-mediated oxidative stress accelerates skeletal muscle atrophy by synchronous activation of proteolytic systems. GeroScience 42 (6), 1579–1591. doi:10.1007/s11357-020-00200-5
Janssens, G. E., Grevendonk, L., Perez, R. Z., Schomakers, B. V., De Vogel-van Den Bosch, J., Geurts, J. M. W., et al. (2022). Healthy aging and muscle function are positively associated with NAD+ abundancein humans. Nat. Aging 2 (3), 254–263. doi:10.1038/s43587-022-00174-3
Ji, X., Zhang, W., Yin, L., Shi, Z., Luan, J., Chen, L., et al. (2022). The potential roles of post-translational modifications of PPARγ in treating diabetes. Biomolecules 12 (12), 1832–1843. doi:10.3390/biom12121832
Jiang, H., Liu, B., Lin, J., Xue, T., Han, Y., Lu, C., et al. (2024). MuSCs and IPCs roles in skeletal muscle homeostasis, aging and injury. Cell Mol. Life Sci. 81, 67–94. doi:10.1007/s00018-023-05096-w
Jiao, J., and Demontis, F. (2017). Skeletal muscle autophagy and its role in sarcopenia and organismal aging. Curr. Opin. Pharmacol. 34, 1–6. doi:10.1016/j.coph.2017.03.009
Jo, E., Lee, S.-R., Park, B.-S., and Kim, J.-S. (2012). Potential mechanisms underlying the role of chronic inflammation in age-related muscle wasting. Aging Clin. Exp. Res. 24 (5), 412–422. doi:10.3275/8464
Jørgensen, S. B., Richter, E. A., and Wojtaszewski, J. F. P. (2006). Role of AMPK in skeletal muscle metabolic regulation and adaptation in relation to exercise. J. Physiol. 574 (1), 17–31. doi:10.1113/jphysiol.2006.109942
Kang, C., and Li Ji, L. (2012). Role of PGC-1α signaling in skeletal muscle health and disease. Ann. N. Y. Acad. Sci. 1271 (1), 110–117. doi:10.1111/j.1749-6632.2012.06738.x
Kawada, T. (2021). Mortality risk of sarcopenia in older subjects. J. Am. Med. Dir. Assoc. 22 (9), 1883. doi:10.1016/j.jamda.2021.04.011
Keisuke, Y., and Takashi, N. (2023). NAD+ precursors in human health and disease: current status and future prospects. Antioxid. Redox Sign. 39 (16), 1133–1149. doi:10.1089/ars.2023.0354
Khaidizar, F. D., Bessho, Y., and Nakahata, Y. (2021). Nicotinamide phosphoribosyltransferase as a key molecule of the aging/senescence process. I Int. J. Mol. Sci. 22 (7), 3709. doi:10.3390/ijms22073709
Khaidizar, F. D., Nakahata, Y., Kume, A., Sumizawa, K., Kohno, K., Matsui, T., et al. (2017). Nicotinamide phosphoribosyltransferase delays cellular senescence by upregulating SIRT 1 activity and antioxidant gene expression in mouse cells. Genes cells. 22 (12), 982–992. doi:10.1111/gtc.12542
Kim, J. S., Yoon, C.-S., and Park, D. R. (2014). NAMPT regulates mitochondria biogenesis via NAD metabolism and calcium binding proteins during skeletal muscle contraction. J. Exerc. Nutr. Biochem. 18 (3), 259–266. doi:10.5717/jenb.2014.18.3.259
Koltai, E., Szabo, Z., Atalay, M., Boldogh, I., Naito, H., Goto, S., et al. (2010). Exercise alters SIRT1, SIRT6, NAD and NAMPT levels in skeletal muscle of aged rats. Mech. Ageing Dev. 131 (1), 21–28. doi:10.1016/j.mad.2009.11.002
Krafczyk, N., and Klotz, L. (2022). FOXO transcription factors in antioxidant defense. IUBMB Life 74 (1), 53–61. doi:10.1002/iub.2542
Lamb, D. A., Moore, J. H., Mesquita, P. H. C., Smith, M. A., Vann, C. G., Osburn, S. C., et al. (2020). Resistance training increases muscle NAD+ and NADH concentrations as well as NAMPT protein levels and global sirtuin activity in middle-aged, overweight, untrained individuals. Aging 12 (10), 9447–9460. doi:10.18632/aging.103218
Lapatto, H. A. K., Kuusela, M., Heikkinen, A., Muniandy, M., van der Kolk, B. W., Gopalakrishnan, S., et al. (2023). Nicotinamide riboside improves muscle mitochondrial biogenesis, satellite cell differentiation, and gut microbiota in a twin study. Sci. Adv. 9 (2), eadd5163–23. doi:10.1126/sciadv.add5163
Larouche, J. A., Mohiuddin, M., Choi, J. J., Ulintz, P. J., Fraczek, P., Sabin, K., et al. (2021). Murine muscle stem cell response to perturbations of the neuromuscular junction are attenuated with aging. eLife 10, e66749. doi:10.7554/eLife.66749
Lautrup, S., Hou, Y., Fang, E. F., and Bohr, V. A. (2024). Roles of NAD + in health and aging. CSH Perspect. Med. 14 (1), a041193. doi:10.1101/cshperspect.a041193
Lee, C. M., Lopez, M. E., Weindruch, R., and Aiken, J. M. (1998). Association of age-related mitochondrial abnormalities with skeletal muscle fiber atrophy. Free Radic. Biol. Med. 25 (8), 964–972. doi:10.1016/S0891-5849(98)00185-3
Li, Q., Jiang, X., Zhou, Y., Gu, Y., Ding, Y., Luo, J., et al. (2023). Improving mitochondrial function in skeletal muscle contributes to the amelioration of insulin resistance by nicotinamide riboside. Int. J. Mol. Sci. 24 (12), 10015. doi:10.3390/ijms241210015
Liang, Y., Junfeng, S., Chunhua, L., Zhonghua, K., Yong, T., Zhengjiang, Q., et al. (2023). Nicotine rebalances NAD+ homeostasis and improves aging-related symptoms in male mice by enhancing NAMPT activity. Nat. Commun. 14 (1), 900–916. doi:10.1038/s41467-023-36543-8
Liu, H.-W., and Chang, S.-J. (2018). Moderate exercise suppresses NF-κB signaling and activates the SIRT1-AMPK-pgc1α Axis to attenuate muscle loss in diabetic db/db mice. Front. Physiol. 9, 636–644. doi:10.3389/fphys.2018.00636
Liu, Y., Chewchuk, S., Lavigne, C., Brûlé, S., Pilon, G., Houde, V., et al. (2009). Functional significance of skeletal muscle adiponectin production, changes in animal models of obesity and diabetes, and regulation by rosiglitazone treatment. Am. J. Physiol.-Endocrinol. Metab. 297 (3), E657–E664. doi:10.1152/ajpendo.00186.2009
López-Otín, C., Blasco, M. A., Partridge, L., Serrano, M., and Kroemer, G. (2023). Hallmarks of aging: an expanding universe. Cell 186 (2), 243–278. doi:10.1016/j.cell.2022.11.001
Lucena-Cacace, A., Otero-Albiol, D., Jiménez-García, M. P., Muñoz-Galvan, S., and Carnero, A. (2018). NAMPT is a potent oncogene in colon cancer progression that modulates cancer stem cell properties and resistance to therapy through Sirt1 and PARP. Clin. Cancer Res. 24 (5), 1202–1215. doi:10.1158/1078-0432.CCR-17-2575
Luo, C., Yang, C., Wang, X., Chen, Y., Liu, X., and Deng, H. (2022). Nicotinamide reprograms adipose cellular metabolism and increases mitochondrial biogenesis to ameliorate obesity. J. Nutr. Biochem. 107, 109056. doi:10.1016/j.jnutbio.2022.109056
Ma, C., Pi, C., Yang, Y., Lin, L., Shi, Y., Li, Y., et al. (2017). Nampt expression decreases age-related senescence in rat bone marrow mesenchymal stem cells byTargeting Sirt1. PLOS ONE 12 (1), e0170930. doi:10.1371/journal.pone.0170930
Malavasi, F., Deaglio, S., Funaro, A., Ferrero, E., Horenstein, A. L., Ortolan, E., et al. (2008). Evolution and function of the ADP ribosyl cyclase/CD38 gene family in physiology and pathology. Physiol. Rev. 88 (3), 841–886. doi:10.1152/physrev.00035.2007
Masiero, E., Agatea, L., Mammucari, C., Blaauw, B., Loro, E., Komatsu, M., et al. (2009). Autophagy is required to maintain muscle mass. Cell Metab. 10 (6), 507–515. doi:10.1016/j.cmet.2009.10.008
McBride, M. J., Foley, K. P., D’Souza, D. M., Li, Y. E., Lau, T. C., Hawke, T. J., et al. (2017). The NLRP3 inflammasome contributes to sarcopenia and lower muscle glycolytic potential in old mice. Am. J. Physiol.-Endocrinol. Metab. 313 (2), E222–E232. doi:10.1152/ajpendo.00060.2017
McGee, S. L., Van Denderen, B. J. W., Howlett, K. F., Mollica, J., Schertzer, J. D., Kemp, B. E., et al. (2008). AMP-activated protein kinase regulates GLUT4 transcription by phosphorylating histone deacetylase 5. Diabetes 57 (4), 860–867. doi:10.2337/db07-0843
Meex, R. C. R., Blaak, E. E., and Van Loon, L. J. C. (2019). Lipotoxicity plays a key role in the development of both insulin resistance and muscle atrophy in patients with type2 diabetes. Obes. Rev. Off. J. Int. Assoc. Study Obes. 20 (9), 1205–1217. doi:10.1111/obr.12862
Montllor-Albalate, C., Song, Z., and Chen, D. (2021). The therapeutic promises of NAD+ boosters. Cell Metab. 33 (7), 1274–1275. doi:10.1016/j.cmet.2021.06.008
Morawin, B., Tylutka, A., Bielewicz, F., and Zembron-Lacny, A. (2023). Diagnostics of inflammaging in relation to sarcopenia. Front. Public Health 11, 1162385. doi:10.3389/fpubh.2023.1162385
Mouchiroud, L., Houtkooper, R. H., Moullan, N., Katsyuba, E., Ryu, D., Cantó, C., et al. (2013). The NAD +/sirtuin pathway modulates longevity through activation of mitochondrial UPR and FOXO signaling. Cell 154 (2), 430–441. doi:10.1016/j.cell.2013.06.016
Moynihan, K. A., Grimm, A. A., Plueger, M. M., Bernal-Mizrachi, E., Ford, E., Cras-Méneur, C., et al. (2005). Increased dosage of mammalian Sir2 in pancreatic beta cells enhances glucose-stimulated insulin secretion in mice. Cell Metab. 2 (2), 105–117. doi:10.1016/j.cmet.2005.07.001
Myers, M. J., Shepherd, D. L., Durr, A. J., Stanton, D. S., Mohamed, J. S., Hollander, J. M., et al. (2019). The role of SIRT1 in skeletal muscle function and repair of older mice. J. Cachexia Sarcopenia Muscle 10 (4), 929–949. doi:10.1002/jcsm.12437
Oki, K., Halievski, K., Vicente, L., Xu, Y., Zeolla, D., Poort, J., et al. (2015). Contractile dysfunction in muscle may underlie androgen-dependent motor dysfunction in spinal bulbar muscular atrophy. J. Appl. Physiol. 118 (7), 941–952. doi:10.1152/japplphysiol.00886.2014
Olmos, Y., Sánchez-Gómez, F. J., Wild, B., García-Quintans, N., Cabezudo, S., Lamas, S., et al. (2013). SirT1 regulation of antioxidant genes is dependent on the formation of a FoxO3a/PGC-1α complex. Antioxid. Redox Signal. 19 (13), 1507–1521. doi:10.1089/ars.2012.4713
Padilla, C. J., Harrigan, M. E., Harris, H., Schwab, J. M., Rutkove, S. B., Rich, M. M., et al. (2021). Profiling age-related muscle weakness and wasting: neuromuscular junction transmission as a driver of age-related physical decline. GeroScience 43 (3), 1265–1281. doi:10.1007/s11357-021-00369-3
Pedro, S., Laura, G., and Pura, M. (2021). Control of satellite cell function in muscle regeneration and its disruption in ageing. Nat. Rev. Mol. Cell Biol. 23 (3), 204–226. doi:10.1038/s41580-021-00421-2
Piacente, F., Caffa, I., Ravera, S., Sociali, G., Passalacqua, M., Vellone, V. G., et al. (2017). Nicotinic acid phosphoribosyltransferase regulates cancer cell metabolism, susceptibility to NAMPT inhibitors, and DNA repair. Cancer Res. 77 (14), 3857–3869. doi:10.1158/0008-5472.CAN-16-3079
Pirinen, E., Auranen, M., Khan, N. A., Brilhante, V., Urho, N., Pessia, A., et al. (2020). Niacin cures systemic NAD+ deficiency and improves muscle performance in adult-onset mitochondrial myopathy. Cell Metab. 31 (6), 1078–1090. doi:10.1016/j.cmet.2020.04.008
Ratia, K. M., Zhengnan, S., Jesse, G., Hyun, L., M. S, L., S, K. I., et al. (2023). Mechanism of allosteric modulation of nicotinamide phosphoribosyltransferase to elevate cellular NAD. Biochemistry 62 (4), 16704–16727. doi:10.1021/acs.biochem.2c00655
Ravikumar, M., and Jared, T. (2022). Nampt activator P7C3 ameliorates diabetes and improves skeletal muscle function modulating cell metabolism and lipid mediators. J. Cachexia Sarcopenia Muscle 13 (2), 1177–1196. doi:10.1002/jcsm.12887
Rebouche, C. J., and Seim, H. (1998). Carnitine metabolism and its regulation in microorganisms and mammals. Annu. Rev. Nutr. 18 (1), 39–61. doi:10.1146/annurev.nutr.18.1.39
Reiten, O. K., Wilvang, M. A., Mitchell, S. J., Hu, Z., and Fang, E. F. (2021). Preclinical and clinical evidence of NAD+ precursors in health, disease, and ageing. Mech. Ageing Dev. 199, 111567. doi:10.1016/j.mad.2021.111567
Revollo, J. R., Körner, A., Mills, K. F., Satoh, A., Wang, T., Garten, A., et al. (2007). Nampt/PBEF/Visfatin regulates insulin secretion in beta cells as a systemic NAD biosynthetic enzyme. Cell Metab. 6 (5), 363–375. doi:10.1016/j.cmet.2007.09.003
Rothschild, J. A., Islam, H., Bishop, D. J., Kilding, A. E., Stewart, T., and Plews, D. J. (2022). Factors influencing AMPK activation during cycling exercise: a pooled analysis and meta-regression. Sports Med. 52 (6), 1273–1294. doi:10.1007/s40279-021-01610-x
Ruderman, N. B., Julia Xu, X., Nelson, L., Cacicedo, J. M., Saha, A. K., Lan, F., et al. (2010). AMPK and SIRT1: a long-standing partnership? Am. J. Physiol.-Endocrinol. Metab. 298 (4), E751–E760. doi:10.1152/ajpendo.00745.2009
Ryall, J. G., Dell’Orso, S., Derfoul, A., Juan, A., Zare, H., Feng, X., et al. (2015). The NAD+-Dependent SIRT1 deacetylase translates a metabolic switch into regulatory epigenetics in skeletal muscle stem cells. Cell Stem Cell 16 (2), 171–183. doi:10.1016/j.stem.2014.12.004
Ryu, D., Zhang, H., Ropelle, E. R., Sorrentino, V., Mázala, D. A. G., Mouchiroud, L., et al. (2016). NAD + repletion improves muscle function in muscular dystrophy and counters global PARylation. Sci. Transl. Med. 8 (361), 361ra139–14. doi:10.1126/scitranslmed.aaf5504
Revollo, J. R., Grimm, A. A., and Imai, S. (2004). The NAD Biosynthesis Pathway Mediated by Nicotinamide Phosphoribosyltransferase Regulates Sir2 Activity in Mammalian Cells. J. Biol. Chem. 279 (49), 50754–50763. doi:10.1074/jbc.M408388200
Samal, B., Sun, Y., Stearns, G., Xie, C., Suggs, S., and McNIECE, I. (1994). Cloning and characterization of the cDNA encoding a novel human pre-B-cell colony-enhancing factor. Mol. Cell Biol. 114, 1431–1437. doi:10.1128/mcb.14.2.1431
Sambasivan, R., Yao, R., Kissenpfennig, A., Van Wittenberghe, L., Paldi, A., Gayraud-Morel, B., et al. (2011). Pax7-expressing satellite cells are indispensable for adult skeletal muscle regeneration. Development 138 (19), 3647–3656. doi:10.1242/dev.067587
Samuel, L., Nannan, Z., Xiaowan, W., Luis, P.-P., and Shinghua, D. (2020). The effect of NAMPT deletion in projection neurons on the function and structure of neuromuscular junction (NMJ) in mice. Sci. Rep. 10 (1), 99–113. doi:10.1038/s41598-019-57085-4
Schultz, M. B., and Sinclair, D. A. (2016). Why NAD+ declines during aging: it’s destroyed. Cell Metab. 23 (6), 965–966. doi:10.1016/j.cmet.2016.05.022
Shen, C., Chen, C., Wang, T., Gao, T.-Y., Zeng, M., Lu, Y.-B., et al. (2023). The depletion of NAMPT disturbs mitochondrial homeostasis and causes neuronal degeneration in mouse Hippocampus. Mol. Neurobiol. 60 (3), 1267–1280. doi:10.1007/s12035-022-03142-5
Sies, H., Berndt, C., and Jones, D. P. (2017). Oxidative stress. Annu. Rev. Biochem. 86, 715–748. doi:10.1146/annurev-biochem-061516-045037
Silva, C. C. C., Soares, C. H., Kim, T. N. L., and Nunes, C. E. (2023). NAD metabolism: role in senescence regulation and aging. Aging Cell 23 (1), 1–23. doi:10.1111/acel.13920
Son, J. W., Lee, S. S., Kim, S. R., Yoo, S. J., Cha, B. Y., Son, H. Y., et al. (2017). Low muscle mass and risk of type 2 diabetes in middle-aged and older adults: findings from the KoGES. Diabetologia 60 (5), 865–872. doi:10.1007/s00125-016-4196-9
Stein, L. R., and Imai, S. (2012). The dynamic regulation of NAD metabolism in mitochondria. Trends Endocrinol. Metab. 23 (9), 420–428. doi:10.1016/j.tem.2012.06.005
Stein, L. R., and Imai, S. (2014). Specific ablation of Nampt in adult neural stem cells recapitulates their functional defects during aging. EMBO J. 33 (12), 1321–1340. doi:10.1002/embj.201386917
Stromsdorfer, K. L., Yamaguchi, S., Yoon, M. J., Moseley, A. C., Franczyk, M. P., Kelly, S. C., et al. (2016). NAMPT-mediated NAD+ biosynthesis in adipocytes regulates adipose tissue function and multi-organ insulin sensitivity in mice. Cell Rep. 16 (7), 1851–1860. doi:10.1016/j.celrep.2016.07.027
Sullivan-Gunn, M. J., and Lewandowski, P. A. (2013). Elevated hydrogen peroxide and decreased catalase and glutathione peroxidase protection are associated with aging sarcopenia. BMC Geriatr. 13 (1), 104. doi:10.1186/1471-2318-13-104
Takahashi, R., Nakamura, S., Nakazawa, T., Minoura, K., Yoshida, T., Nishi, Y., et al. (2010). Structure and reaction mechanism of human nicotinamide phosphoribosyltransferase. J. Biochem. 147 (1), 95–107. doi:10.1093/jb/mvp152
Tantiwong, P., Shanmugasundaram, K., Monroy, A., Ghosh, S., Li, M., DeFronzo, R. A., et al. (2010). NF-□B activity in muscle from obese and type 2 diabetic subjects under basal and exercise-stimulated conditions. Am. J. Physiol. Endocrinol. Metab. 299, E794–E801. doi:10.1152/ajpendo.00776.2009
Tarragó, M. G., Chini, C. C. S., Kanamori, K. S., Warner, G. M., Caride, A., De Oliveira, G. C., et al. (2018). A potent and specific CD38 inhibitor ameliorates age-related metabolic dysfunction by reversing tissue NAD+ decline. Cell Metab. 27 (5), 1081–1095. doi:10.1016/j.cmet.2018.03.016
Theret, M., Gsaier, L., Schaffer, B., Juban, G., Ben Larbi, S., Weiss-Gayet, M., et al. (2017). AMPK α1- LDH pathway regulates muscle stem cell self-renewal by controlling metabolic homeostasis. EMBO J. 36 (13), 1946–1962. doi:10.15252/embj.201695273
Thomson, D. M. (2018). The role of AMPK in the regulation of skeletal muscle size, hypertrophy, and regeneration. Int. J. Mol. Sci. 19 (10), 3125. doi:10.3390/ijms19103125
Tzameli, I. (2012). The evolving role of mitochondria in metabolism. Trends Endocrinol. Metab. 23 (9), 417–419. doi:10.1016/j.tem.2012.07.008
van der Heiden, K., Cuhlmann, S., Luong, L. A., Zakkar, M., and Evans, P. C. (2010). Role of nuclear factor kappaB in cardiovascular health and disease. Clin. Sci. 118 (10), 593–605. doi:10.1042/CS20090557
Wanagat, J., Cao, Z., Pathare, P., and Aiken, J. M. (2001). Mitochondrial DNA deletion mutations colocalize with segmental electron transport system abnormalities, muscle fiber atrophy, fiber splitting, and oxidative damage in sarcopenia. FASEB J. 15 (2), 322–332. doi:10.1096/fj.00-0320com
Wang, G., Han, T., Nijhawan, D., Theodoropoulos, P., Naidoo, J., Yadavalli, S., et al. (2014). P7C3 neuroprotective chemicals function by activating the rate-limiting enzyme in NAD salvage. Cell 158 (6), 1324–1334. doi:10.1016/j.cell.2014.07.040
Wang, X., Li, H., and Ding, S. (2016). Pre-B-cell colony-enhancing factor protects against apoptotic neuronal death and mitochondrial damage in ischemia. Sci. Rep. 6 (1), 32416. doi:10.1038/srep32416
Wang, X., Zhang, Q., Bao, R., Zhang, N., Wang, Y., Polo-Parada, L., et al. (2017). Deletion of nampt in projection neurons of adult mice leads to motor dysfunction, neurodegeneration, and death. Cell Rep. 20 (9), 2184–2200. doi:10.1016/j.celrep.2017.08.022
Wen, F., Gui, G., Wang, X., Ye, L., Qin, A., Zhou, C., et al. (2024). Drug discovery targeting nicotinamide phosphoribosyltransferase (NAMPT): updated progress and perspectives. Bioorgan Med. Chem. 99, 117595–117617. doi:10.1016/j.bmc.2024.117595
Winder, W. W., and Hardie, D. G. (1996). Inactivation of acetyl-CoA carboxylase and activation of AMP-activated protein kinase in muscle during exercise. Am. J. Physiol.-Endocrinol. Metab. 270 (2), E299–E304. doi:10.1152/ajpendo.1996.270.2.E299
Wu, G., Su, Q., Li, J., Xue, C., Zhu, J., Cai, Q., et al. (2023). NAMPT encapsulated by extracellular vesicles from young adipose-derived mesenchymal stem cells treated tendinopathy in a ‘One-Stone-Two-Birds’ manner. J. Nanobiotechnology 21 (1), 7. doi:10.1186/s12951-022-01763-5
Xie, W., Zhu, T., Zhou, P., Xu, H., Meng, X., Ding, T., et al. (2020). Notoginseng leaf triterpenes ameliorates OGD/R-Induced neuronal injury via SIRT1/2/3-foxo3a-MnSOD/PGC-1α signaling pathways mediated by the NAMPT-NAD pathway. Oxid. Med. Cell. Longev. 2020, 7308386–7308415. doi:10.1155/2020/7308386
Xing, S., Hu, Y., Huang, X., Shen, D., and Chen, C. (2019). Nicotinamide phosphoribosyltransferase-related signaling pathway in early Alzheimer’s disease mouse models. Mol. Med. Rep. 20 (6), 5163–5171. doi:10.3892/mmr.2019.10782
Yang, H., Yang, T., Baur, J. A., Perez, E., Matsui, T., Carmona, J. J., et al. (2007). Nutrient-sensitive mitochondrial NAD+ levels dictate cell survival. Cell 130 (6), 1095–1107. doi:10.1016/j.cell.2007.07.035
Yao, Z., Yingming, W., Shuai, L., Rui, Z., Zhilin, L., Qichun, Z., et al. (2023). Nicotinamide Phosphoribosyltransferase-elevated NAD+ biosynthesis prevents muscle disuse atrophy by reversing mitochondrial dysfunction. J. Cachexia Sarcopenia Muscle 14 (2), 1003–1018. doi:10.1002/jcsm.13182
Yeung, F., Hoberg, J. E., Ramsey, C. S., Keller, M. D., Jones, D. R., Frye, R. A., et al. (2004). Modulation of NF-kappaB-dependent transcription and cell survival by the SIRT1 deacetylase. EMBO J. 23 (12), 2369–2380. doi:10.1038/sj.emboj.7600244
Yoshino, J., Mills, K. F., Yoon, M. J., and Imai, S. (2011). Nicotinamide mononucleotide, a key NAD(+) intermediate, treats the pathophysiology of diet- and age-induced diabetes in mice. Cell Metab. 14, 528–536. doi:10.1016/j.cmet.2011.08.014
Yoshino, M., Yoshino, J., Kayser, B. D., Patti, G. J., Franczyk, M. P., Mills, K. F., et al. (2021). Nicotinamide mononucleotide increases muscle insulin sensitivity in prediabetic women. Science 372 (6547), 1224–1229. doi:10.1126/science.abe9985
Zhang, L., Zhang, X., Zhang, T., Guo, Y., Pei, W., Liu, R., et al. (2023). Linolenic acid ameliorates sarcopenia in C. elegans by promoting mitophagy and fighting oxidative stress. Food Funct. 14 (3), 1498–1509. doi:10.1039/D2FO02974J
Zhao, L., Zou, T., Gomez, N. A., Wang, B., Zhu, M.-J., and Du, M. (2018). Raspberry alleviates obesity-induced inflammation and insulin resistance in skeletal muscle through activation of AMP-activated protein kinase (AMPK) α1. Nutr. Diabetes 8 (1), 39. doi:10.1038/s41387-018-0049-6
Zhengnan, S., Kiira, R., Isabella, K., Martha, A., Christopher, P., Reddy, M. S., et al. (2023). Synthesis, optimization, and structure-activity relationships of nicotinamide phosphoribosyltransferase (NAMPT) positive allosteric modulators (N-PAMs). J. Med. Chem. 66 (24), 16704–16727. doi:10.1021/acs.jmedchem.3c01406
Keywords: NAD+, NAMPT, skelelal muscle, aging, T2DM
Citation: Su M, Qiu F, Li Y, Che T, Li N and Zhang S (2024) Mechanisms of the NAD+ salvage pathway in enhancing skeletal muscle function. Front. Cell Dev. Biol. 12:1464815. doi: 10.3389/fcell.2024.1464815
Received: 15 July 2024; Accepted: 09 September 2024;
Published: 20 September 2024.
Edited by:
Venkaiah Betapudi, United States Department of Health and Human Services, United StatesReviewed by:
John Joseph McCarthy, University of Kentucky, United StatesCarlos Escande, Pasteur Institute of Montevideo, Uruguay
Ye Cao, German Institute of Human Nutrition Potsdam-Rehbruecke (DIfE), Germany
Copyright © 2024 Su, Qiu, Li, Che, Li and Zhang. This is an open-access article distributed under the terms of the Creative Commons Attribution License (CC BY). The use, distribution or reproduction in other forums is permitted, provided the original author(s) and the copyright owner(s) are credited and that the original publication in this journal is cited, in accordance with accepted academic practice. No use, distribution or reproduction is permitted which does not comply with these terms.
*Correspondence: Shuangshuang Zhang, emhhbmdzaHVhbmdzaHVhbmdAcWR1LmVkdS5jbg==