- Department of Anatomy, All India Institute of Medical Sciences, Bhubaneswar, India
Pancreatic cancer continues to be a deadly disease because of its delayed diagnosis and aggressive tumor biology. Oncogenes and risk factors are being reported to influence the signaling pathways involved in pancreatic embryogenesis leading to pancreatic cancer genesis. Although studies using rodent models have yielded insightful information, the scarcity of human pancreatic tissue has made it difficult to comprehend how the human pancreas develops. Transcription factors like IPF1/PDX1, HLXB9, PBX1, MEIS, Islet-1, and signaling pathways, including Hedgehog, TGF-β, and Notch, are directing pancreatic organogenesis. Any derangements in the above pathways may lead to pancreatic cancer. TP53: and CDKN2A are tumor suppressor genes, and the mutations in TP53 and somatic loss of CDKN2A are the drivers of pancreatic cancer. This review clarifies the complex signaling mechanism involved in pancreatic cancer, the same signaling pathways in pancreas development, the current therapeutic approach targeting signaling molecules, and the mechanism of action of risk factors in promoting pancreatic cancer.
Introduction
The close association between the incidence and death highlights the terrible prognosis of pancreatic cancer (PC). Recent data indicates that the 5-year survival rate for pancreatic cancer worldwide is approximately 11% (Pancreatic Cancer Statistics, 2024). Adding to this low survival rate is late late-stage diagnosis of the disease (Kamisawa et al., 2016). Currently, PC is the seventh leading cause of cancer-related deaths worldwide (Rawla et al., 2019) and is assumed to become the second major cause of death globally by 2030. The European countries have the highest occurrence rates, and China leads the Asian nations in incidence and fatality, followed by Bhutan, Nepal, and India (Gaidhani and Balasubramaniam, 2021; Kamisawa et al., 2016; Raimondi et al., 2009).
PC is more common in men (5.5 per 100,000, 243,033 cases) than in women (4.0 per 100,000, 215,885 cases). PC is more commonly observed in elderly populations and rarely diagnosed before 55 years of age. The treatment strategies are complicated due to early metastasis, recurrence, and resistance to radiation and chemotherapy (Brunner et al., 2019). Signaling pathways play a significant role in disease progression and behavior. When signaling pathways malfunction, cancer develops. Cancerous cells can grow and metastasize due to mutations and dysregulation in the signaling pathways that link with pancreatic development (Kamisawa et al., 2016).
Understanding the signaling pathways in pancreatic development is crucial for comprehending PC. In recent years, research on transcription factors involved in the development, function, and disease process of the pancreas has expanded (Jennings et al., 2020). These include the signaling mechanisms that might regulate cell connections in the developing pancreas. Here, we will explore the factors and genes involved in the signaling pathways leading to PC while exploring how these pathways are involved in pancreatic embryogenesis.
Signaling pathways in pancreatic morphogenesis and cancer
The pancreas is a unique tissue with endocrine and exocrine components. Acinar and duct cells constitute the exocrine portion of the pancreas, and the islet of Langerhans comprises endocrine components (Rizk et al., 2023). Ghrelin, somatostatin, insulin, glucagon-producing cells, and pancreatic polypeptide comprise the endocrine part of the islet of Langerhans (Ornellas et al., 2020; Murtaugh and Melton, 2003). The development of endocrine and exocrine cells, along with acini formation, is influenced by signaling pathways (Dohrmann et al., 2000). Signaling pathways are complex networks of molecular interactions that allow cells to communicate with each other and respond to external signals (Azeloglu and Iyengar, 2015). Interaction between exocrine and endocrine components of Langerhans through signaling components results in controlled hormone secretion. The signaling component involves ligands, receptors, and intracellular Smads (Figure 1), which are present in the pancreatic epithelium and mesenchyme (El-Gohary et al., 2013).
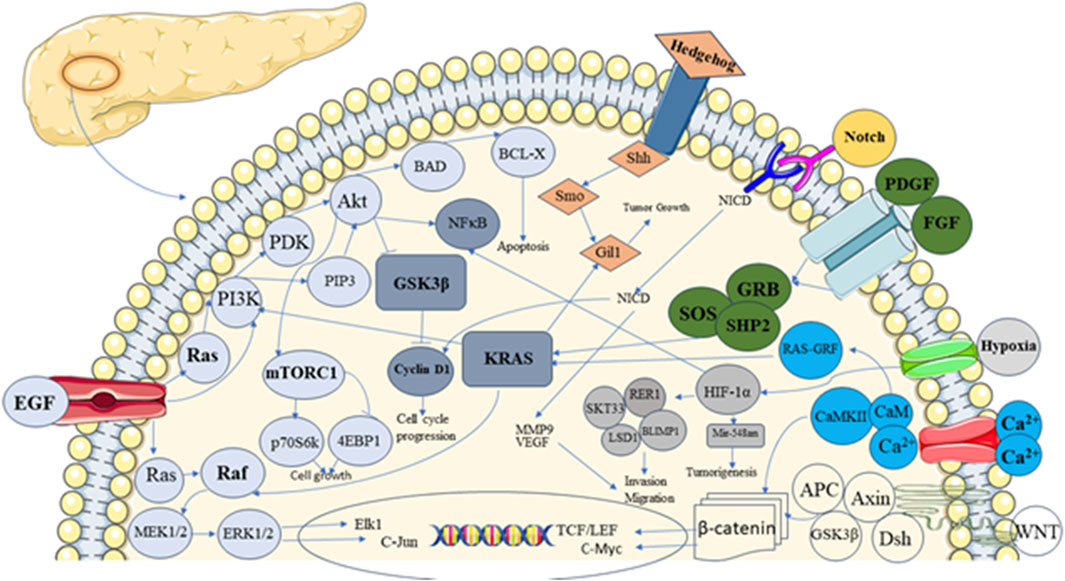
Figure 1. Showing different signalling pathways involved in pancreatic development and carcinogenesis.
Pancreatic embryogenesis requires complex signaling pathways; Insulin Promoter Factor 1/Pancreatic and Duodenal homeobox 1 (IPF1/PDX1) is the most significant transcription factor, the earliest expressed in the embryonic pancreas. IPF1/PDX1 is a ParaHox group homeodomain transcription factor essential for the development of the pancreas in humans and mice (Rosanas-Urgell et al., 2005). This signaling molecule is expressed in pancreatic cells of postnatal mice, and literature indicates that this signaling pathway is necessary for specific tasks in the mature cell (Ebrahim et al., 2022). It responds to growth signals from the mesenchyme (Kim and MacDonald, 2002) and is expressed in the endoderm. The expression is not limited to pancreatic tissue, as PDX1 mutant mice commence dorsal and ventral bud development (Offield et al., 1996), indicating that other components are needed to designate the pancreas anlage. The IPF1/PDX1 pathway is dysregulated in PC, leading to a loss of normal functions. The loss of IPF1/PDX1 function can lead to abnormal cell growth and decreased differentiation of pancreatic cells, creating an environment conducive to cancer development (Roy et al., 2016). IPF1/PDX1 regulates downstream molecules such as Neurogenin 3 (NGN3), Forkhead Box A2 (FOXA2), Hepatocyte Nuclear Factor 1 Beta (HNF1B), Fibroblast Growth Factor Receptor 2 (FGFR2IIIB), and Spondin 1, which are involved in pancreatic development, differentiation, and function in pancreatic cancer (Svensson et al., 2007; Oliver-Krasinski et al., 2009). IPF1/PDX1 expression levels are also being explored as potential diagnostic and prognostic markers for PC (Kim and MacDonald, 2002). The tumor’s aggressiveness can be determined based on IPF1/PDX1 expression changes. Changes in signaling pathways, epigenetic modifications, and genetic mutations might cause dysregulation in IPF1/PDX1 expression (Kim and MacDonald, 2002).
Pre-B-cell leukemia homeobox- 1 (PBX1) and Myeloid Ecotropic Integration Site (MEIS) regulate the DNA binding activity of other gene products like IPF1/PDX1. They control gene expression and coordinate pancreas development (Kim and Hebrok, 2001). PBX1 and MEIS interact with HOX genes and form complexes that regulate gene expression during the embryonic development of the pancreas. Dysregulation of these interactions can potentially affect the normal growth and maintenance of pancreatic tissue, indirectly contributing to the initiation or progression of PC (Girgin et al., 2020). MEIS1 mutant mice die during embryogenesis from hematological and vascular abnormalities, indicating that MEIS1 is crucial for embryonic development (Burstin et al., 2010). PBX1 contributes to cancer by affecting lineages within the hematopoietic system, such as B cells, HSCs, and Mk-Erythrocyte Progenitors (MEP) (Muggeo et al., 2021).
Similarly, Homeobox gene B9 (HLXB9) is a vital transcription factor required for dorsal pancreatic development and the formation of insulin-producing beta cells (Jensen J, 2004). HLXB9 altered expression contributes to the progression of the disease. Still, it is not a driver mutation in PC; HLXB9 expression can impact the cellular environment and promote cancerous growth (Chen et al., 2018). In order to maintain cancer cell proliferation, HLXB9 upregulates genes that are involved in the G1-S transition of the cell cycle, such as Cyclin E1 (CCNE1) and Cyclin E2 (CCNE2) (Chen et al., 2018). Understanding HLXB9’s role in pancreatic development could have implications for regenerative medicine and potential therapies that aim to restore or repair pancreatic tissue in patients with PC (Desai et al.,2015). Hence, a temporally prolonged expression of HLXB9 leads to severe impairment of pancreatic development, whereas a total loss of HLXB9 expression blocks the start of the dorsal pancreatic program (Li and Edlund, 2001).
Transforming growth factor - beta (TGF-β) signaling components, including ligands like activin and TGF-β and their corresponding receptors, as well as ligand antagonists like follistatin, noggin, and gremlin, are present in both mesenchyme and epithelium of embryonic and adult pancreas (Chang, 2016). The distinct functions TGF proteins play in regulating the endocrine and exocrine pancreas are suggested by the varied expression patterns for each TGF-β isoform. All of them are expressed persistently and become localized to the acinar cells later in gestation. They are all faintly present in the E12.5 epithelial cells early in the pancreas formation (Rane et al., 2006).
Additionally, TGF-β signaling regulates several cellular functions; dysregulation of this signaling results in the onset and spread of cancer (Baba et al., 2022). Upon binding of active TGF-β to a class of transmembrane serine-threonine kinases known as Type I and Type II TGF-β receptors (TβRI and TβRII, respectively), the TGF-β signaling cascade is initiated. (Aashaq et al., 2022). After binding to TβRII, the TGF-β ligand recruits TβRI to form a complex. TβRII can cross-phosphorylate TβRI, which activates it, thanks to this ligand-bound receptor complex (Smith et al., 2012). In advanced stages, TGF-β can promote tumor growth and metastasis. However, In the early stages of cancer, it acts as a tumor suppressor by inhibiting cell growth and promoting apoptosis (Liu et al., 2021). A similar trend is for PC; TGF-β signaling often becomes dysregulated, contributing to disease progression. TGF-β can function as a tumor suppressor by inhibiting the uncontrolled proliferation of cancer cells in early pancreatic carcinogenesis (Sabbadini et al., 2021). Early stages of PC development are due to the loss of the TGF-β receptor or mutations in downstream signaling components that disrupt this tumor suppressor role (Truty and Urrutia, 2007). The TGF-β signaling pathway is the current research interest because of its dual nature. TGF-β signaling inhibitors are being investigated as possible therapeutics to counteract TGF-β′s pro-tumorigenic activities and re-establish its tumor-suppressive properties (Javle et al., 2014).
Early on, it was discovered that follistatin was widely distributed in pancreatic mesenchyme and vanished at the E12.5 stage of pancreas development. Follistatin is still expressed in adult islets and reappears in E18.5 (Maldonado et al., 2000). Throughout the early stages of embryogenesis, mesenchyme-derived follistatin inhibits epithelium-derived activin, allowing for unopposed exocrine differentiation and a relative reduction in endocrine differentiation (Namwanje and Brown, 2016). Epithelial activin is released later in life due to a decrease in mesenchyme compared to epithelium and a drop in follistatin levels (Xia and Schneyer, 2009). This helps to differentiate endocrine cells into mature islets after birth (Maldonado et al., 2000). TGF-β antagonist follistatin is a glycoprotein primarily known for regulating the activity of TGF-β family members, such as activin and myostatin. The ability of follicular fluid to block follicle-stimulating hormone (FSH) led to the discovery of follistatin (FST) (Kappes et al., 2023; Welt et al., 2002). Activins are bound to FST, a monomeric glycosylated protein, with remarkable affinity, neutralizing their binding affinity and bioactivity. FST also exhibits a lower affinity for binding to myostatin (MST) and Bone Morphogenetic Proteins (BMPs) 2, 5, 7, and 8 (Sidis et al., 2006; Amthor et al., 2004; Hedger et al., 2011; Iemura et al., 1998) in addition to other TGF-β superfamily members. These studies demonstrate how FST may affect the biological activities of many members of the TGF-β superfamily, especially at greater doses (Pervin et al., 2021). Follistatin inhibits TGF-β signaling pathways by binding to ligands and preventing them from binding to their receptors (Harrington et al., 2006). It affects embryonic exocrine and endocrine cell differentiation. By controlling the TGF-β pathway, follistatin shapes cell development in the pancreas (Lee et al., 2021; Kahata et al., 2018).
Similarly, pancreas transcription factor 1A (PTF1A) plays a crucial role in controlling the proliferation of multipotent progenitor cells throughout pancreatic development and in the maintenance and specification of acinar cells. The inhibitory neuronal cell fate in neural tissues is determined by PTF1A, transiently produced in the post-mitotic cells, and mediated mostly by downstream genes such as transcription factor activation profiles (TFAP2A/B) and Positive Regulatory Domain (PRDM13). In humans and rodents, Mutations in the coding and non-coding regulatory spaces cause PTF1A gain or loss of function, which is linked to hereditary disorders such as pancreatic and cerebellar agenesis (Jin and Xiang, 2019).
The vital pancreatic transcription factor PTF1A is induced in the dorsal pancreatic endoderm by aortic endothelial cells; in contrast, ventral PTF1A induction and ventral pancreatic bud commencement, do not require the vitelline veins, which are often located next to the developing ventral pancreatic bud. We discover that PTF1A is induced in dorsal endoderm explants by the aorta cells, independent of the blood supply (Yoshitomi and Zaret, 2004).
The Sry-related HMG box (SOX) family of transcription factors, also known as the sex-determining region on the Y box, is involved in developing multiple tissues throughout embryogenesis and determining cell destiny. In PDX1+ ductal cells of the human pancreas, SOX9 is detected in the eighth week of embryonic development. It frequently co-localizes with Neurogenin-3 (NGN3) and other significant islet beta-cell progenitor markers (McDonald et al., 2012). SOX9 transcription factor plays a less important role in the development of the exocrine pancreas and regulates the adoption of an endocrine phenotype (Seymour et al., 2008).
Notch signaling in pancreatic embryogenesis and cancer
Tumor-suppressing and tumor-promoting effects in PC are mediated by Notch signaling. Cancer cell proliferation, metastasis, and cancer stem cell phenotype formation are also mediated by notch signaling. Notch signaling governs the development of pancreatic endocrine and exocrine cells. Notch signaling involves the activation of ligands by neurogenin genes, which leads to the transcription of Hairy and Enhancer-of-Split (HES) genes that influence cell fate (Kim et al., 2010; Li et al., 2016). Abnormalities in Notch signaling and PC are associated. The most common PC, pancreatic ductal adenocarcinoma (PDAC), has the notch pathway dysregulated. Several studies have shown that NGN3, a pro-endocrine factor, is negatively regulated by Notch signaling. Pro-endocrine factor activation or Notch processing inhibition dramatically promotes the development of insulin-producing β-cells. However, as of late, several scientists have disputed that the Notch pathway prevents the growth of endocrine cells. It has been suggested that either the inactivated Notch pathway favors acinar cell development or the Notch pathway determines the pancreatic progenitors developing towards endocrine lineage. Based on the current research, Notch signaling controls the quiescence, self-renewal, and differentiation of pancreatic progenitor cells during pancreatic development in a manner dependent on the Notch level (Li et al., 2016).
Hedgehog (Hh) signaling in pancreatic embryogenesis and cancer
Hedgehog signaling, in addition to notch signaling, is essential for PC. Hh ligands (Sonic, Indian, and Desert Hhs), Smoothened (SMO), Patched receptor (PTCH1), and transcription factors (GLI1, GLI2, and GLI3) are essential elements of the Hh signaling system. The Hh ligands bind to the transmembrane receptor, PTCH1, which usually inhibits another transmembrane protein, SMO. Active SMO triggers a downstream signaling cascade that involves a family of transcription factors known as GLI proteins (GLI1, GLI2, and GLI3). In the absence of Hh signaling, GLI proteins are inhibited through a complex formation involving other proteins, resulting in abnormal cell growth. Activin signaling suppresses the expression of Sonic Hedgehog (SHH), ensuring proper pancreatic development (Han et al., 2016). The differentiation of diverse cell types in the embryonic pancreas is then regulated by Hh signaling pathways (Carballo et al., 2018). The Hh pathway regulates insulin production in the adult pancreas, but it is also necessary to regenerate the exocrine pancreas in response to damage, Its activity is highly restricted to the beta-cells of the endocrine pancreas.
Two independent studies first reported aberrant activation of the Hh pathway in human PC. The normal pancreas does not produce Shh, whereas 70% of human PC samples exhibit overexpression of Shh in both pre-invasive and invasive epithelium; this overexpression can be observed as early as pancreatic intraepithelial neoplasia-1 (PanIN1) and persists throughout the disease (Gu et al., 2016). On the other hand, most PC cell lines have an abnormal expression of the Hh ligand. This finding in PDAC in humans was also validated in a genetically modified mice model. In PDAC, oncogenic Kirsten rat sarcoma (KRAS) expression is closely linked to abnormal SHH expression. Increased SHH transcript results from oncogenic KRASG12D ectopic expression in healthy human pancreatic ductal cells, suggesting that SHH functions as a downstream effector of oncogenic KRASG12D in developing PC. It has also been demonstrated that NF-κB targets the gene SHH and is constitutively activated in PC. In both cell-based and in-vivo scenarios, NF-κB activation can enhance SHH’s transcriptional activity. The putative NF-κB binding sites are present in the human SHH promoter region. Furthermore, oncogenic KRAS is recognised to activate the transcriptional activity of NF-κB. Thus, oncogenic KRAS may use NF-κB signaling to encourage SHH expression (Gu et al., 2016).
Oncogenes altering common signaling pathways
The literature presents an increasing number of oncogenes that cause PC (Hezel et al., 2006; Avila and Kissil, 2013; Wood, 2013). When oncogenes are mutated and activated, they contribute to cancer growth. PC was attributed to genetic alterations, germline mutations, and somatic mutations. KRAS, TP53, CDKNA2A, MLL3, ZIM2, MAP2K4, ARID1A, NALCN, SMAD4, EPC1, ARID2, ATM, TGFBR2, SLC16A4, SF3B1, and MAGEA6 are the sixteen mutant oncogenes that have been found majorly associated with this disease (Cicenas et al., 2017).
KRAS mutations, one of the earliest changes in PC, trigger signals promoting cancer cell survival and multiplication (Berrozpe et al.,1994). These mutations lead to the continuous activation of the KRAS protein, which acts as a molecular switch to activate several downstream signaling pathways, including the MAPK/ERK and PI3K/AKT pathways (Buscail et al., 2020). Tumor suppressor genes help control cell growth and prevent tumors from forming. In PC, these brakes often malfunction due to mutations (Bardeesy and DePinho, 2002). P16/CDKN2A, TP53, and SMAD4/DPC4 are critical players in this process. Loss of P16/CDKN2A function leads to unchecked cell cycle progression from the G1 to the S phase. Loss of SMAD4 function disrupts TGF-β signaling and promotes epithelial-mesenchymal transition (EMT), enhancing the invasiveness and metastatic potential of pancreatic cancer cells (Hu et al., 2021). Loss of these genes removes the brakes, giving cancer cells a growth advantage (Austen and Bronte, 2023).
Growth factor receptors work as antennas on the cell surface. These antennas receive signals from growth factors like epidermal growth factor (EGF), insulin-like growth factor (IGF), and vascular endothelial growth factor (VEGF). In PC, growth factor receptors can be proactive, leading to uncontrolled cell growth and spread. When activated, the receptor for advanced glycation end products (RAGE) acts as a magnifier of inflammation and promotes the progression of PC. Researchers are exploring ways to turn down the activity of RAGE to slow down cancer growth and enhance the effectiveness of treatments (Faruqui et al.,2022). EMT is crucial for cancer cells to spread and form new tumors in distant places (Zhou et al., 2017). The WNT signaling pathway acts as a control center for cell growth (Scheibner et al., 2019). This control center can go haywire in PC, leading to unregulated cell division and migration (Zeng et al., 2006).
Risk factors and their mechanism of action in altering signaling pathways
Risk factors like the combination of family history, obesity, smoking, diabetes, and chronic pancreatitis can contribute to the development of PC. A genetic predisposition to the disease is noticed by studying a family history of PC. Hereditary mutations in the BRCA1, BRCA2, and PALB2 genes are risk factors for PC. These genetic alterations may affect cellular signaling pathways, including KRAS oncogene activation. Similarly, Genetic mutation can also cause disruptions to cellular processes, potentially leading to the activation of WNT/β-catenin Pathway and an increased risk of developing PC (Zanini et al., 2021).
One of the most common risk factors that raises the possibility of developing PC is type 2 diabetes (Pannala et al., 2008). The complete mechanism is still a doubt for researchers. However, there is evidence that insulin resistance and abnormal signaling pathways, such as the serine/threonine kinase (AKT) pathway, may play a role. AKT (AKT1, AKT2, AKT3), previously known as protein kinase B (PKB), signaling pathway is currently driving the research as it plays a vital role in primary cellular functions, including regulation of glucose metabolism, cell size, and cell cycle progression (Hu et al., 2022; Permert et al., 1993). In type 2 diabetes Insulin resistance and hyperinsulinemia activate PI3K/AKT pathway, low grade inflammation activates the NF-κB pathway and high glucose levels activate the TGF-β1 pathway, leading to a decrease in E-cadherin levels and promoting a mesenchymal phenotype (Gong et al., 2014; Duan et al., 2021).
Another risk factor for PC is Chronic pancreatitis. Chronic inflammation leads to the activation of Pancreatic Stellate Cells (PSCs), which transform into myofibroblast-like cells. PSCs secrete chemokines, reactive oxygen species, and cytokines which activates TGF-β, MAPK, and NF-κB, promoting cancer cell proliferation, invasion (Jin et al., 2020).
There is an established connection between the risk of PC and obesity. Adipose tissue secretes leptin, and it is possible that leptin could influence Hh signaling (Zanini et al., 2021). Like in other cancers, smoking is a well-established risk factor in PC. nitrosamines and polycyclic aromatic hydrocarbons present in smoke, can cause mutations in the KRAS gene and activates NF-κB Pathway, Overall leads to mutation in key genes like KRAS, p53, and CDKN2A. Nicotine, a major component of tobacco smoke, can bind to nicotinic acetylcholine receptors (nAChRs) on pancreatic cells. This binding activates the EGFR signaling pathway, leading to increased cell proliferation and survival (Schaal and Chellappan, 2014; Weissman et al., 2020). Accumulation of mutations and epigenetic alterations in the DNA due to age-related changes can lead to the activation of oncogenes or the inactivation of tumor suppressor genes. Gender-related differences may influence oncogene activation by triggering hormonal and genetic factors. For instance, hormones, including estrogen, testosterone, and insulin-like growth factor-1 (IGF-1) in men, significantly contribute to a higher incidence of PC. Genetic variations between racial groups lead to oncogene activation differences (Zanini et al., 2021).
Therapeutic interventions targeting common pathways
The initial step in treating PC is to remove the tumor surgically, which is followed by gemcitabine-based chemotherapy. In cases where patients exhibit a favourable performance status and surgery is not feasible, a combination of gemcitabine, FOLFIRINOX, and nanoparticle-bound (nab) paclitaxel is used. However, the prognosis is still dismal, and chemotherapy medications have only been shown to be palliative in PC patients whose cancer has spread or is incurable (Lambert et al., 2019). However, there is hope due to the known molecular mechanisms underlying the onset and spread of PC and the availability of novel medications that can disrupt essential signaling pathways (Polireddy and Chen, 2016).
Another therapeutic target for PC can be the hypoxia-inducible factor (HIF1α), which is a downstream effector of PBX1-MEIS1. This target utilisation has been observed to influence Myeloproliferative neoplasm (MPN) cells via PBX1 (Crisafulli et al., 2024). As discussed, HLXB9 can play a significant role in tumor progression, the GSK-3β phosphorylates and stabilizes the HLXB9 protein and, therefore, can be targeted to control the development of insulinomas (Desai et al., 2014).
Many inhibitors have applications in treating PC since the PI3K/AKT pathway can be blocked at multiple places (Stanciu et al., 2022). The mTOR kinase inhibitors, such as everolimus, are among the medications used to slow the disease progression to an end-stage and can also improve the effectiveness of gemcitabine-based chemotherapy. Agents that have demonstrated enhanced efficacy and can inhibit mTORC1 and mTORC2 are preferable. By attaching to the PH domain of AKT, perifoxine (KRX041, NSC639966) functions as an allosteric AKT inhibitor (Glaviano et al., 2023). Numerous clinical trials with this alkyl phospholipid have been conducted following encouraging outcomes from studies conducted on animal models. In pancreatic cell cultures, erifosine suppresses S6K1–GLI1 signaling and prevents gemcitabine resistance (Ying et al., 2014). Prior research indicates that cancer stem cells play a significant role in patient relapse, maybe through the reactivation of the SHH signaling pathway and the PI3K/AKT/mTOR pathway. A combination of NVP-BEZ235 and NVP-LDE225 may provide new hope for treating PC (Sharma et al., 2015). An oral medication called NVP-LDE225 (Sonidegib, Novartis) inhibits the Hh pathway by acting as an antagonist for the SMO receptor (Pan et al., 2010; Ruiz-Borrego et al., 2019; Wang et al., 2019; Stanciu et al., 2022).
Anti-TGF-β treatments are effective in preclinical research, and several of these tactics are presently undergoing clinical trials. TGF-β neutralizing antibodies, which prevent TGF-β ligands from binding to their receptor, TGF-β receptor kinase inhibitors, and direct delivery of antisense oligonucleotides (ASO) into tumors or immune cells are the three main strategies for inhibiting TGF-β or its pathway components. (Smith et al., 2012).
Although anticancer drugs have historically focused on TGF-β pathway inhibitors, the efficacy of contemporary therapy has not kept pace with this focus (Tian et al., 2019). This emphasizes the need for a more thorough investigation of FST as a strong and efficient TGF-β antagonist that can target cancer cells specifically or stop them from developing resistance to TGF-β′s anti-proliferative effects. It can also be used as a biomarker to classify cancer patients and enhance their responses to treatment more accurately. Comprehending the role of FST in specific disorders holds the potential for creating innovative therapeutics, especially with the current focus on secreted molecules in drug development (Table 1) (Sosa et al., 2024).
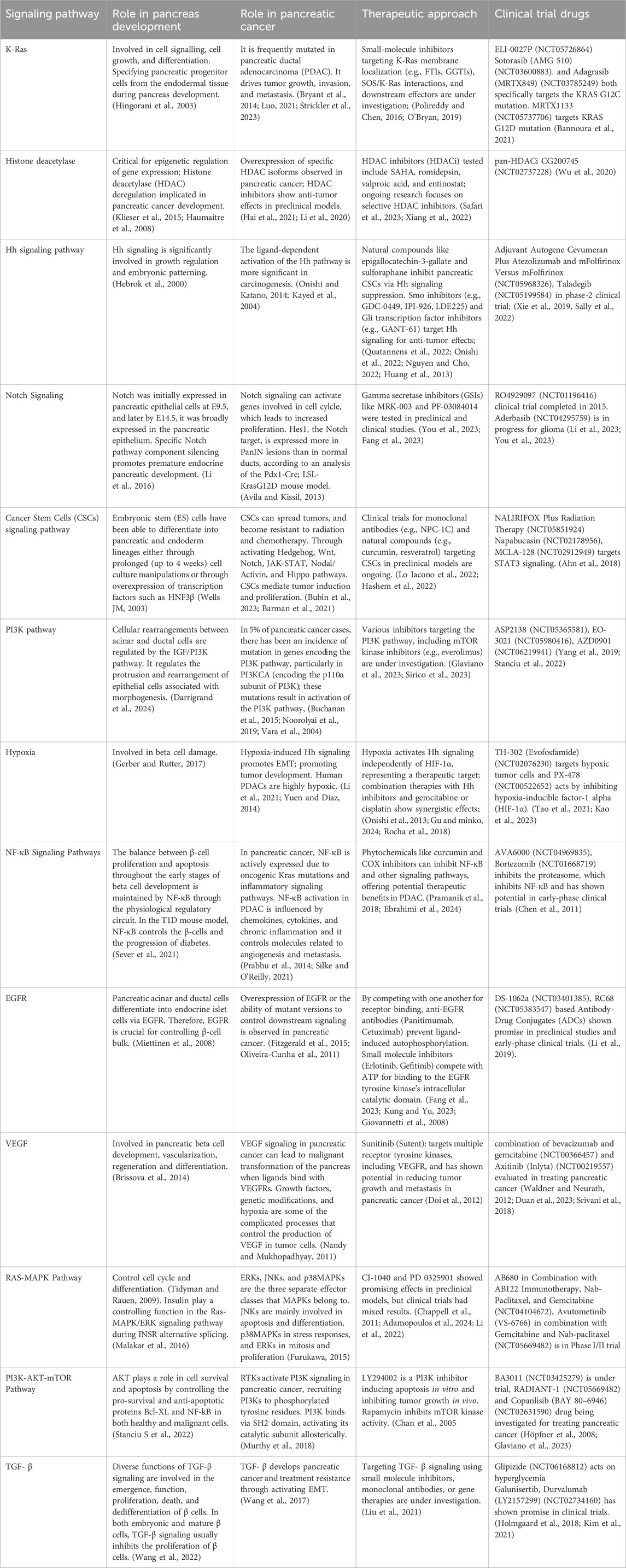
Table 1. Signaling pathways involved pancreas embryogenesis, pancreatic cancer and therapeutic development targeting the same signaling pathways.
Conclusion
PC poses a significant difficulty because of its late-stage diagnosis and aggressive nature. Our complete investigation exposed the intricate interaction of oncogenes and risk factors, essential signaling networks in the development of the pancreas, and modulation in the same signaling pathway in PC. Our understanding of how transcription factors and signaling pathways are regulated in PC has grown by comprehending pancreas development. Despite having a low occurrence, genetic abnormalities and alterations in tumor suppressor genes significantly influence PC. Future research on precise mechanisms by which signaling pathways are activated and further exploration of the specific transcription factor expression patterns associated with PC could enable more personalized treatment strategies.
Different care strategies are used according to the stage and resectability of the cancer, with surgical procedures being explored in some situations. Despite difficulties, continuous research on signaling pathways and biomarkers can give optimism for improvements in early identification, more efficient therapies, and eventually better results for people with PC. Clinical trials, including combination therapies and signaling pathway inhibitors, hold promise for improving outcomes for PC.
Author contributions
SS: Conceptualization, Formal Analysis, Investigation, Methodology, Writing–original draft. RN: Conceptualization, Project administration, Resources, Supervision, Validation, Writing–original draft. PM: Formal Analysis, Funding acquisition, Investigation, Resources, Supervision, Validation, Visualization, Writing–review and editing.
Funding
The author(s) declare that no financial support was received for the research, authorship, and/or publication of this article.
Conflict of interest
The authors declare that the research was conducted in the absence of any commercial or financial relationships that could be construed as a potential conflict of interest.
Publisher’s note
All claims expressed in this article are solely those of the authors and do not necessarily represent those of their affiliated organizations, or those of the publisher, the editors and the reviewers. Any product that may be evaluated in this article, or claim that may be made by its manufacturer, is not guaranteed or endorsed by the publisher.
References
Aashaq, S., Batool, A., Mir, S. A., Beigh, M. A., Andrabi, K. I., and Shah, Z. A. (2022). TGF β signaling: a recap of SMAD independent and SMAD-dependent pathways. J. Cell. physiology 237 (1), 59–85. doi:10.1002/jcp.30529
Adamopoulos, C., Cave, D. D., and Papavassiliou, A. G. (2024). Inhibition of the RAF/MEK/ERK signaling cascade in pancreatic cancer: recent advances and future perspectives. Int. J. Mol. Sci. 25 (3), 1631. doi:10.3390/ijms25031631
Ahn, D. H., Ramanathan, R. K., and Bekaii-Saab, T. (2018). Emerging therapies and future directions in targeting the tumor stroma and immune system in the treatment of pancreatic adenocarcinoma. Cancers (Basel) 10, 193. doi:10.3390/cancers10060193
Amthor, H., Nicholas, G., McKinnell, I., Kemp, C. F., Sharma, M., Kambadur, R., et al. (2004). Follistatin complexes Myostatin and antagonises Myostatin-mediated inhibition of myogenesis. Dev. Biol. 270 (1), 19–30. doi:10.1016/j.ydbio.2004.01.046
Austen, J., and Bronte, E. (2023). Oncogenic signaling pathways in carcinogenesis. J. Carcinog. 22 (1). doi:10.4103/jcar.jcar_22_01_16
Avila, J. L., and Kissil, J. L. (2013). Notch signaling in pancreatic cancer: oncogene or tumor suppressor? Trends Mol. Med. 19 (5), 320–327. doi:10.1016/j.molmed.2013.03.003
Azeloglu, E. U., and Iyengar, R. (2015). Signaling networks: information flow, computation, and decision making. Cold Spring Harb. Perspect. Biol. 7 (4), a005934. doi:10.1101/cshperspect.a005934
Baba, A. B., Rah, B., Bhat, G. R., Mushtaq, I., Parveen, S., Hassan, R., et al. (2022). Transforming growth factor-beta (TGF-β) signaling in cancer-A betrayal within. Front. Pharmacol. 13, 791272. doi:10.3389/fphar.2022.791272
Bannoura, S. F., Uddin, Md. H., Nagasaka, M., Fazili, F., Al-Hallak, M. N., Philip, P. A., et al. (2021). Targeting KRAS in pancreatic cancer: new drugs on the horizon. Cancer Metastasis Rev. 40, 819–835. doi:10.1007/s10555-021-09990-2
Bardeesy, N., and DePinho, R. A. (2002). Pancreatic cancer biology and genetics. Nat. Rev. Cancer 2 (12), 897–909. doi:10.1038/nrc949
Barman, S., Fatima, I., Singh, A. B., and Dhawan, P. (2021). Pancreatic cancer and therapy: role and regulation of cancer stem cells. Int. J. Mol. Sci. 22 (9), 4765. doi:10.3390/ijms22094765
Berrozpe, G., Schaeffer, J., Peinado, M. A., Real, F. X., and Perucho, M. (1994). Comparative analysis of mutations in the p53 and K-ras genes in pancreatic cancer. Int. J. cancer 58 (2), 185–191. doi:10.1002/ijc.2910580207
Brissova, M., Aamodt, K., Brahmachary, P., Prasad, N., Hong, J. Y., Dai, C., et al. (2014). Islet microenvironment, modulated by vascular endothelial growth factor-A signaling, promotes β cell regeneration. Cell. metab. 19 (3), 498–511. doi:10.1016/j.cmet.2014.02.001
Brunner, M., Wu, Z., Krautz, C., Pilarsky, C., Grützmann, R., and Weber, G. F. (2019). Current clinical strategies of pancreatic cancer treatment and open molecular questions. Int. J. Mol. Sci. 20 (18), 4543. PMID: 31540286; PMCID: PMC6770743. doi:10.3390/ijms20184543
Bryant, K. L., Mancias, J. D., Kimmelman, A. C., and Der, C. J. (2014). KRAS: feeding pancreatic cancer proliferation. Trends Biochem. Sci. 39 (2), 91–100. doi:10.1016/j.tibs.2013.12.004
Bubin, R., Uljanovs, R., and Strumfa, I. (2023). Cancer stem cells in pancreatic ductal adenocarcinoma. Int. J. Mol. Sci. 24 (8), 7030. PMID: 37108193; PMCID: PMC10138709. doi:10.3390/ijms24087030
Buchanan, A., Newton, P., Pehrsson, S., Inghardt, T., Antonsson, T., Svensson, P., et al. (2015). Structural and functional characterization of a specific antidote for ticagrelor. Blood 125, 3484–3490. doi:10.1182/blood-2015-01-622928
Burstin, J., Reichert, M., Wescott, M. P., and Rustgi, A. K. (2010). The pancreatic and duodenal homeobox protein PDX-1 regulates the ductal specific keratin 19 through the degradation of MEIS1 and DNA binding. PLoS One 5 (8), e12311. doi:10.1371/journal.pone.0012311
Buscail, L., Bournet, B., and Cordelier, P. (2020). Role of oncogenic KRAS in the diagnosis, prognosis and treatment of pancreatic cancer. Nat. Rev. Gastroenterol. Hepatol. 17, 153–168. doi:10.1038/s41575-019-0245-4
Carballo, G. B., Honorato, J. R., de Lopes, G. P., and Spohr, T. C. L. d. S. E. (2018). A highlight on Sonic hedgehog pathway. Cell. Commun. Signal 16, 11. doi:10.1186/s12964-018-0220-7
Chan, S., Scheulen, M. E., Johnston, S., Mross, K., Cardoso, F., Dittrich, C., et al. (2005). Phase II study of temsirolimus (CCI-779), a novel inhibitor of mTOR, in heavily pretreated patients with locally advanced or metastatic breast cancer. J. Clin. Oncol. 23, 5314–5322. doi:10.1200/JCO.2005.66.130
Chang, C. (2016). Agonists and antagonists of TGF-β family ligands. Cold Spring Harb. Perspect. Biol. 8 (8), a021923. PMID: 27413100; PMCID: PMC4968162. doi:10.1101/cshperspect.a021923
Chappell, W. H., Steelman, L. S., Long, J. M., Kempf, R. C., Abrams, S. L., Franklin, R. A., et al. (2011). Ras/Raf/MEK/ERK and PI3K/PTEN/Akt/mTOR inhibitors: rationale and importance to inhibiting these pathways in human health. Oncotarget 2 (3), 135–164. PMID: 21411864; PMCID: PMC3260807. doi:10.18632/oncotarget.240
Chen, D., Frezza, M., Schmitt, S., Kanwar, J., and P. Dou, Q. (2011). Bortezomib as the first proteasome inhibitor anticancer drug: current status and future perspectives. Curr. Cancer Drug Targets 11, 239–253. doi:10.2174/156800911794519752
Chen, M., Wu, R., Li, G., Liu, C., Tan, L., Xiao, K., et al. (2018). Motor neuron and pancreas homeobox 1/HLXB9 promotes sustained proliferation in bladder cancer by upregulating CCNE1/2. J. Exp. Clin. Cancer Res. 37, 154. doi:10.1186/s13046-018-0829-9
Cicenas, J., Kvederaviciute, K., Meskinyte, I., Meskinyte-Kausiliene, E., Skeberdyte, A., and Cicenas, J. (2017). KRAS, TP53, CDKN2A, SMAD4, BRCA1, and BRCA2 mutations in pancreatic cancer. Cancers 9 (5), 42. doi:10.3390/cancers9050042
Crisafulli, L., Brindisi, M., Liturri, M. G., Sobacchi, C., and Ficara, F. (2024). PBX1: a TALE of two seasons—key roles during development and in cancer. Front. Cell. Dev. Biol. 12, 1372873. doi:10.3389/fcell.2024.1372873
Darrigrand, J. F., Salowka, A., Torres-Cano, A., Tapia-Rojo, R., Zhu, T., Garcia-Manyes, S., et al. (2024). Acinar-ductal cell rearrangement drives branching morphogenesis of the murine pancreas in an IGF/PI3K-dependent manner. Dev. Cell. 59 (3), 326–338.e5. doi:10.1016/j.devcel.2023.12.011
Desai, S. S., Kharade, S. S., Parekh, V. I., Iyer, S., and Agarwal, S. K. (2015). Pro-oncogenic roles of HLXB9 protein in insulinoma cells through interaction with Nono protein and down-regulation of the c-Met inhibitor Cblb (Casitas B-lineage lymphoma b). J. Biol. Chem. 290 (42), 25595–25608. doi:10.1074/jbc.M115.661413
Desai, S. S., Modali, S. D., Parekh, V. I., Kebebew, E., and Agarwal, S. K. (2014). GSK-3β protein phosphorylates and stabilizes HLXB9 protein in insulinoma cells to form a targetable mechanism of controlling insulinoma cell proliferation. J. Biol. Chem. 289, 5386–5398. doi:10.1074/jbc.M113.533612
Dohrmann, C., Gruss, P., and Lemaire, L. (2000). Pax genes and the differentiation of hormone-producing endocrine cells in the pancreas. Mech. Dev. 92 (1), 47–54. doi:10.1016/s0925-4773(99)00324-x
Doi, Y., Yashiro, M., Yamada, N., Amano, R., Noda, S., and Hirakawa, K. (2012). VEGF-A/VEGFR-2 signaling plays an important role for the motility of pancreas cancer cells. Ann. Surg. Oncol. 19, 2733–2743. doi:10.1245/s10434-011-2181-6
Duan, H., Li, L., and He, S. (2023). Advances and prospects in the treatment of pancreatic cancer. Int. J. Nanomedicine 31, 3973–3988. doi:10.2147/IJN.S413496
Duan, X., Wang, W., Pan, Q., and Guo, L. (2021). Type 2 diabetes mellitus intersects with pancreatic cancer diagnosis and development. Front. Oncol. 11, 730038. doi:10.3389/fonc.2021.730038
Ebrahim, N., Shakirova, K., and Dashinimaev, E. (2022). PDX1 is the cornerstone of pancreatic β-cell functions and identity. Front. Mol. Biosci. 9, 1091757. doi:10.3389/fmolb.2022.1091757
Ebrahimi, N., Abdulwahid, A. H., Mansouri, A., Karimi, N., Bostani, R. J., Beiranvand, S., et al. (2024). Targeting the NF-κB pathway as a potential regulator of immune checkpoints in cancer immunotherapy. Cell. Mol. Life Sci. 81 (1), 106–122. doi:10.1007/s00018-023-05098-8
El-Gohary, Y., Tulachan, S., Guo, P., Welsh, C., Wiersch, J., Prasadan, K., et al. (2013). Smad signaling pathways regulate pancreatic endocrine development. Dev. Biol. 378 (2), 83–93. doi:10.1016/j.ydbio.2013.04.003
Fang, Y. T., Yang, W. W., Niu, Y. R., and Sun, Y. K. (2023). Recent advances in targeted therapy for pancreatic adenocarcinoma. World J. Gastrointest. Oncol. 15 (4), 571–595. doi:10.4251/wjgo.v15.i4.571
Faruqui, T., Khan, M. S., Akhter, Y., Khan, S., Rafi, Z., Saeed, M., et al. (2022). RAGE inhibitors for targeted therapy of cancer: a comprehensive review. Int. J. Mol. Sci. 24 (1), 266. doi:10.3390/ijms24010266
Fitzgerald, T. L., Lertpiriyapong, K., Cocco, L., Martelli, A. M., Libra, M., Candido, S., et al. (2015). Roles of EGFR and KRAS and their downstream signaling pathways in pancreatic cancer and pancreatic cancer stem cells. Adv. Biol. Regul. 59, 65–81. doi:10.1016/j.jbior.2015.06.003
Furukawa, T. (2015). Impacts of activation of the mitogen-activated protein kinase pathway in pancreatic cancer. Front. Oncol. 5, 23. PMID: 25699241; PMCID: PMC4316689. doi:10.3389/fonc.2015.00023
Gaidhani, R. H., and Balasubramaniam, G. (2021). An epidemiological review of pancreatic cancer with special reference to India. Indian J. Med. Sci. 73 (1), 99–109. doi:10.25259/ijms_92_2020
Gerber, P. A., and Rutter, G. A. (2017). The role of oxidative stress and hypoxia in pancreatic beta-cell dysfunction in diabetes mellitus. Antioxidants and redox Signal. 26 (10), 501–518. doi:10.1089/ars.2016.6755
Giovannetti, E., Lemos, C., Tekle, C., Smid, K., Nannizzi, S., Rodriguez, J. A., et al. (2008). Molecular mechanisms underlying the synergistic interaction of erlotinib, an epidermal growth factor receptor tyrosine kinase inhibitor, with the multitargeted antifolate pemetrexed in non-small-cell lung cancer cells. Mol. Pharmacol. 73 (4), 1290–1300. doi:10.1124/mol.107.042382
Girgin, B., Karadag-Alpaslan, M., and KocabaS, F. (2020). Oncogenic and tumor suppressor function of MEIS and associated factors. Turk J. Biol. 44 (6), 328–355. doi:10.3906/biy-2006-25
Glaviano, A., Foo, A. S. C., Lam, H. Y., Yap, K. C. H., Jacot, W., Jones, R. H., et al. (2023). PI3K/AKT/mTOR signaling transduction pathway and targeted therapies in cancer. Mol. Cancer 22, 138. doi:10.1186/s12943-023-01827-6
Gong, J., Robbins, L. A., Lugea, A., Waldron, R. T., Jeon, C. Y., and Pandol, S. J. (2014). Diabetes, pancreatic cancer, and metformin therapy. Front. physiology 5, 426. doi:10.3389/fphys.2014.00426
Gu, D., Schlotman, K. E., and Xie, J. (2016). Deciphering the role of hedgehog signaling in pancreatic cancer. J. Biomed. Res. 30 (5), 353–360. doi:10.7555/JBR.30.20150107
Gu, X., and Minko, T. (2024). Targeted nanoparticle-based diagnostic and treatment options for pancreatic cancer. Cancers 16 (8), 1589. doi:10.3390/cancers16081589
Hai, R., He, L., Shu, G., and Yin, G. (2021). Characterization of histone deacetylase mechanisms in cancer development. Front. Oncol. 11, 700947. doi:10.3389/fonc.2021.700947
Han, L., Ma, J., Duan, W., Zhang, L., Yu, S., Xu, Q., et al. (2016). Pancreatic stellate cells contribute pancreatic cancer pain via activation of sHH signaling pathway. Oncotarget 7 (14), 18146–18158. doi:10.18632/oncotarget.7776
Harrington, A. E., Morris Triggs, S. A., Ruotolo, B. T., Robinson, C. V., Ohnuma, S., and Hyvönen, M. (2006). Structural basis for the inhibition of activin signalling by follistatin. EMBO J. 25 (5), 1035–1045. doi:10.1038/sj.emboj.7601000
Hashem, S., Ali, T. A., Akhtar, S., Nisar, S., Sageena, G., Ali, S., et al. (2022). Targeting cancer signaling pathways by natural products: exploring promising anti-cancer agents. Biomed. and Pharmacother. 150, 113054. doi:10.1016/j.biopha.2022.113054
Haumaitre, C., Lenoir, O., and Scharfmann, R. (2008). Histone deacetylase inhibitors modify pancreatic cell fate determination and amplify endocrine progenitors. Mol. Cell. Biol. 28 (20), 6373–6383. doi:10.1128/MCB.00413-08
Hebrok, M., Kim, S. K., St-Jacques, B., McMahon, A. P., and Melton, D. A. (2000). Regulation of pancreas development by hedgehog signaling. Development 127 (22), 4905–4913. doi:10.1242/dev.127.22.4905
Hedger, M. P., Winnall, W. R., Phillips, D. J., and de Kretser, D. M. (2011). The regulation and functions of activin and follistatin in inflammation and immunity. Vitam. Horm. 85, 255–297. doi:10.1016/B978-0-12-385961-7.00013-5
Hezel, A. F., Kimmelman, A. C., Stanger, B. Z., Bardeesy, N., and DePinho, R. A. (2006). Genetics and biology of pancreatic ductal adenocarcinoma. Genes. and Dev. 20 (10), 1218–1249. doi:10.1101/gad.1415606
Hingorani, S. R., Petricoin, E. F., Maitra, A., Rajapakse, V., King, C., Jacobetz, M. A., et al. (2003). Preinvasive and invasive ductal pancreatic cancer and its early detection in the mouse. Cancer Cell. 4 (6), 437–450. doi:10.1016/s1535-6108(03)00309-x
Holmgaard, R. B., Schaer, D. A., Li, Y., Castaneda, S. P., Murphy, M. Y., Xu, X., et al. (2018). Targeting the TGFβ pathway with galunisertib, a TGFβRI small molecule inhibitor, promotes anti-tumor immunity leading to durable, complete responses, as monotherapy and in combination with checkpoint blockade. J. Immunother. Cancer 6, 47. doi:10.1186/s40425-018-0356-4
Höpfner, M., Schuppan, D., and Scherübl, H. (2008). Treatment of gastrointestinal neuroendocrine tumors with inhibitors of growth factor receptors and their signaling pathways: recent advances and future perspectives. World J. Gastroenterol. 14, 2461–2473. doi:10.3748/wjg.14.2461
Hu, H., Ye, Z., Qin, Y., Xu, X., Yu, X., Zhuo, Q., et al. (2021). Mutations in key driver genes of pancreatic cancer: molecularly targeted therapies and other clinical implications. Acta Pharmacol. Sin. 42, 1725–1741. doi:10.1038/s41401-020-00584-2
Hu, Y., Zeng, N., Ge, Y., Wang, D., Qin, X., Zhang, W., et al. (2022). Identification of the shared gene signatures and biological mechanism in type 2 diabetes and pancreatic cancer. Front. Endocrinol. 13, 847760. doi:10.3389/fendo.2022.847760
Huang, Y. C., Chao, K. S., Liao, H. F., and Chen, Y. J. (2013). Targeting sonic hedgehog signaling by compounds and derivatives from natural products. Evid. Based Complement. Altern. Med. 2013, 748587. Epub 2013 May 20. PMID: 23762158; PMCID: PMC3671665. doi:10.1155/2013/748587
Iemura, S., Yamamoto, T. S., Takagi, C., Uchiyama, H., Natsume, T., Shimasaki, S., et al. (1998). Direct binding of follistatin to a complex of bone-morphogenetic protein and its receptor inhibits ventral and epidermal cell fates in early Xenopus embryo. Proc. Natl. Acad. Sci. U. S. A. 95 (16), 9337–9342. doi:10.1073/pnas.95.16.9337
Javle, M., Li, Y., Tan, D., Dong, X., Chang, P., Kar, S., et al. (2014). Biomarkers of TGF-β signaling pathway and prognosis of pancreatic cancer. PloS one 9 (1), e85942. doi:10.1371/journal.pone.0085942
Jennings, R. E., Scharfmann, R., and Staels, W. (2020). Transcription factors that shape the mammalian pancreas. Diabetologia 63 (10), 1974–1980. Epub 2020 Sep 7. PMID: 32894307; PMCID: PMC7476910. doi:10.1007/s00125-020-05161-0
Jensen, J. (2004). Gene regulatory factors in pancreatic development. Dev. Dyn. 229 (1), 176–200. doi:10.1002/dvdy.10460
Jin, G., Hong, W., Guo, Y., Bai, Y., and Chen, B. (2020). Molecular mechanism of pancreatic stellate cells activation in chronic pancreatitis and pancreatic cancer. J. Cancer 11, 1505–1515. doi:10.7150/jca.38616
Jin, K., and Xiang, M. (2019). Transcription factor Ptf1a in development, diseases and reprogramming. Cell. Mol. Life Sci. 76 (5), 921–940. doi:10.1007/s00018-018-2972-z
Kahata, K., Maturi, V., and Moustakas, A. (2018). TGF-Β family signaling in ductal differentiation and branching morphogenesis. Cold Spring Harb. Perspect. Biol. 10 (3), a031997. doi:10.1101/cshperspect.a031997
Kamisawa, T., Wood, L. D., Itoi, T., and Takaori, K. (2016). Pancreatic cancer. Lancet 388 (10039), 73–85. doi:10.1016/S0140-6736(16)00141-0
Kao, T.-W., Bai, G.-H., Wang, T.-L., Shih, I.-M., Chuang, C.-M., Lo, C.-L., et al. (2023). Novel cancer treatment paradigm targeting hypoxia-induced factor in conjunction with current therapies to overcome resistance. J. Exp. and Clin. Cancer Res. 42, 171. doi:10.1186/s13046-023-02724-y
Kappes, E. C., Kattamuri, C., Czepnik, M., Yarawsky, A. E., Brûlé, E., Wang, Y., et al. (2023). Follistatin forms a stable complex with inhibin A that does not interfere with activin A antagonism. Endocrinology 164 (3), bqad017. doi:10.1210/endocr/bqad017
Kayed, H., Kleeff, J., Keleg, S., Guo, J., Ketterer, K., Berberat, P. O., et al. (2004). Indian hedgehog signaling pathway: expression and regulation in pancreatic cancer. Int. J. cancer 110 (5), 668–676. doi:10.1002/ijc.20194
Kim, B.-G., Malek, E., Choi, S. H., Ignatz-Hoover, J. J., and Driscoll, J. J. (2021). Novel therapies emerging in oncology to target the TGF-β pathway. J. Hematol. Oncol. 14, 55. doi:10.1186/s13045-021-01053-x
Kim, S. K., and Hebrok, M. (2001). Intercellular signals regulating pancreas development and function. Genes. and Dev. 15 (2), 111–127. doi:10.1101/gad.859401
Kim, S. K., and MacDonald, R. J. (2002). Signaling and transcriptional control of pancreatic organogenesis. Curr. Opin. Genet. and Dev. 12 (5), 540–547. doi:10.1016/s0959-437x(02)00338-6
Kim, W., Shin, Y. K., Kim, B. J., and Egan, J. M. (2010). Notch signaling in pancreatic endocrine cell and diabetes. Biochem. Biophys. Res. Commun. 392 (3), 247–251. doi:10.1016/j.bbrc.2009.12.115
Klieser, E., Swierczynski, S., Mayr, C., Schmidt, J., Neureiter, D., Kiesslich, T., et al. (2015). Role of histone deacetylases in pancreas: implications for pathogenesis and therapy. World J. Gastrointest. Oncol. 7 (12), 473–483. doi:10.4251/wjgo.v7.i12.473
Kung, H. C., and Yu, J. (2023). Targeted therapy for pancreatic ductal adenocarcinoma: mechanisms and clinical study. MedComm 4 (2), e216. doi:10.1002/mco2.216
Lambert, A., Schwarz, L., Borbath, I., Henry, A., Van Laethem, J.-L., Malka, D., et al. (2019). An update on treatment options for pancreatic adenocarcinoma. Ther. Adv. Med. Oncol. 11, 1758835919875568. doi:10.1177/1758835919875568
Lee, J. H., Lee, J. H., and Rane, S. G. (2021). TGF-Β signaling in pancreatic islet β cell development and function. Endocrinology 162 (3), bqaa233. doi:10.1210/endocr/bqaa233
Li, G., Tian, Y., and Zhu, W. G. (2020). The roles of histone deacetylases and their inhibitors in cancer therapy. Front. Cell. Dev. Biol. 8, 576946. doi:10.3389/fcell.2020.576946
Li, H., and Edlund, H. (2001). Persistent expression of Hlxb9 in the pancreatic epithelium impairs pancreatic development. Dev. Biol. 240 (1), 247–253. doi:10.1006/dbio.2001.0440
Li, H., Peng, C., Zhu, C., Nie, S., Qian, X., Shi, Z., et al. (2021). Hypoxia promotes the metastasis of pancreatic cancer through regulating NOX4/KDM5A-mediated histone methylation modification changes in a HIF1A-independent manner. Clin. Epigenet 13, 18. doi:10.1186/s13148-021-01016-6
Li, Q., Li, Z., Luo, T., and Shi, H. (2022). Targeting the PI3K/AKT/mTOR and RAF/MEK/ERK pathways for cancer therapy. Mol. Biomed. 3, 47. doi:10.1186/s43556-022-00110-2
Li, X., Yan, X., Wang, Y., Kaur, B., Han, H., and Yu, J. (2023). The Notch signaling pathway: a potential target for cancer immunotherapy. J. Hematol. Oncol. 16, 45. doi:10.1186/s13045-023-01439-z
Li, X.-Y., Zhai, W.-J., and Teng, C.-Bo (2016). Notch signaling in pancreatic development. Int. J. Mol. Sci. 17 (1), 48. doi:10.3390/ijms17010048
Li, Z., Wang, M., Yao, X., Luo, W., Qu, Y., Yu, D., et al. (2019). Development of a novel EGFR-targeting antibody-drug conjugate for pancreatic cancer therapy. Target Oncol. 14, 93–105. doi:10.1007/s11523-018-0616-8
Liu, S., Ren, J., and Ten Dijke, P. (2021). Targeting TGFβ signal transduction for cancer therapy. Signal Transduct. Target. Ther. 6 (1), 8. doi:10.1038/s41392-020-00436-9
Lo Iacono, M., Gaggianesi, M., Bianca, P., Brancato, O. R., Muratore, G., Modica, C., et al. (2022). Destroying the shield of cancer stem cells: natural compounds as promising players in cancer therapy. J. Clin. Med. 11 (23), 6996. doi:10.3390/jcm11236996
Luo, J. (2021). KRAS mutation in pancreatic cancer. Seminars Oncol. 2021 Feb 1 48 (1), 10–18. doi:10.1053/j.seminoncol.2021.02.003
Malakar, P., Chartarifsky, L., Hija, A., Leibowitz, G., Glaser, B., Dor, Y., et al. (2016). Insulin receptor alternative splicing is regulated by insulin signaling and modulates beta cell survival. Sci. Rep. 6, 31222. doi:10.1038/srep31222
Maldonado, T. S., Kadison, A. S., Crisera, C. A., Grau, J. B., Alkasab, S. L., Longaker, M. T., et al. (2000). Ontogeny of activin B and follistatin in developing embryonic mouse pancreas: implications for lineage selection. J. Gastrointest. Surg. 4 (3), 269–275. doi:10.1016/s1091-255x(00)80075-x
McDonald, E., Li, J., Krishnamurthy, M., Fellows, G. F., Goodyer, C. G., and Wang, R. (2012). SOX9 regulates endocrine cell differentiation during human fetal pancreas development. Int. J. Biochem. and Cell. Biol. 44 (1), 72–83. doi:10.1016/j.biocel.2011.09.008
Miettinen, P., Ormio, P., Hakonen, E., Banerjee, M., and Otonkoski, T. (2008). EGF receptor in pancreatic beta-cell mass regulation. Biochem. Soc. Trans. 36 (3), 280–285. doi:10.1042/BST0360280
Muggeo, S., Crisafulli, L., Uva, P., Fontana, E., Ubezio, M., Morenghi, E., et al. (2021). PBX1-directed stem cell transcriptional program drives tumor progression in myeloproliferative neoplasm. Stem Cell. Rep. 16 (11), 2607–2616. doi:10.1016/j.stemcr.2021.09.016
Murtaugh, L. C., and Melton, D. A. (2003). Genes, signals, and lineages in pancreas development. Annu. Rev. Cell. Dev. Biol. 19 (1), 71–89. doi:10.1146/annurev.cellbio.19.111301.144752
Murthy, D., Attri, K. S., and Singh, P. K. (2018). Phosphoinositide 3-kinase signaling pathway in pancreatic ductal adenocarcinoma progression, pathogenesis, and therapeutics. Front. physiology 9, 335. doi:10.3389/fphys.2018.00335
Namwanje, M., and Brown, C. W. (2016). Activins and inhibins: roles in development, physiology, and disease. Cold Spring Harb. Perspect. Biol. 8 (7), a021881. PMID: 27328872; PMCID: PMC4930927. doi:10.1101/cshperspect.a021881
Nandy, D., and Mukhopadhyay, D. (2011). Growth factor mediated signaling in pancreatic pathogenesis. Cancers (Basel) 3 (1), 841–871. doi:10.3390/cancers3010841
Nguyen, N. M., and Cho, J. (2022). Hedgehog pathway inhibitors as targeted cancer therapy and strategies to overcome drug resistance. Int. J. Mol. Sci. 23 (3), 1733. doi:10.3390/ijms23031733
Noorolyai, S., Shajari, N., Baghbani, E., Sadreddini, S., and Baradaran, B. (2019). The relation between PI3K/AKT signalling pathway and cancer. Gene 698, 120–128. doi:10.1016/j.gene.2019.02.076
O'Bryan, J. P. (2019). Pharmacological targeting of RAS: recent success with direct inhibitors. Pharmacol. Res. 139, 503–511. doi:10.1016/j.phrs.2018.10.021
Offield, M. F., Jetton, T. L., Labosky, P. A., Ray, M., Stein, R. W., Magnuson, M. A., et al. (1996). PDX-1 is required for pancreatic outgrowth and differentiation of the rostral duodenum. Development 122 (3), 983–995. doi:10.1242/dev.122.3.983
Oliveira-Cunha, M., Newman, W. G., and Siriwardena, A. K. (2011). Epidermal growth factor receptor in pancreatic cancer. Cancers 3 (2), 1513–1526. doi:10.3390/cancers3021513
Oliver-Krasinski, J. M., Kasner, M. T., Yang, J., Crutchlow, M. F., Rustgi, A. K., Kaestner, K. H., et al. (2009). The diabetes gene Pdx1 regulates the transcriptional network of pancreatic endocrine progenitor cells in mice. J. Clin. Investigation 119, 1888–1898. doi:10.1172/JCI37028
Onishi, H., and Katano, M. (2014). Hedgehog signaling pathway as a new therapeutic target in pancreatic cancer. World J. gastroenterology WJG 20 (9), 2335–2342. doi:10.3748/wjg.v20.i9.2335
Onishi, H., Morisaki, T., Nakao, F., Odate, S., Morisaki, T., and Katano, M. (2013). Protein-bound polysaccharide decreases invasiveness and proliferation in pancreatic cancer by inhibition of hedgehog signaling and HIF-1α pathways under hypoxia. Cancer Lett. 335 (2), 289–298. doi:10.1016/j.canlet.2013.02.041
Onishi, H., Nakamura, K., Yanai, K., Nagai, S., Nakayama, K., Oyama, Y., et al. (2022). Cancer therapy that targets the Hedgehog signaling pathway considering the cancer microenvironment. Oncol. Rep. 47 (5), 1–0. doi:10.3892/or.2022.8304
Ornellas, F., Karise, I., Aguila, M. B., and Mandarim-de-Lacerda, C. A. (2020) “Pancreatic islets of langerhans: adapting cell and molecular biology to changes of metabolism,” in Obesity and diabetes: scientific advances and best practice, 175–190.
Pan, S., Wu, X., Jiang, J., Gao, W., Wan, Y., Cheng, D., et al. (2010). Discovery of NVP-LDE225, a potent and selective smoothened antagonist. ACS Med. Chem. Lett. 1, 130–134. doi:10.1021/ml1000307
Pancreatic cancer statistics (2024). Available at: https://www.wcrf.org/cancer-trends/pancreatic-cancer-statistics/(Accessed August 5, 2024).
Pannala, R., Leirness, J. B., Bamlet, W. R., Basu, A., Petersen, G. M., and Chari, S. T. (2008). Prevalence and clinical profile of pancreatic cancer-associated diabetes mellitus. Gastroenterology 134, 981–987. doi:10.1053/j.gastro.2008.01.039
Permert, J., Ihse, I., Jorfeldt, L., von Schenck, H., Arnqvist, H. J., and Larsson, J. (1993). Pancreatic cancer is associated with impaired glucose metabolism. Eur. J. Surg. 159, 101–107.
Pervin, S., Reddy, S. T., and Singh, R. (2021). Novel roles of follistatin/myostatin in transforming growth factor-β signaling and adipose browning: potential for therapeutic intervention in obesity related metabolic disorders. Front. Endocrinol. 12, 653179. doi:10.3389/fendo.2021.653179
Polireddy, K., and Chen, Q. (2016). Cancer of the pancreas: molecular pathways and current advancement in treatment. J. Cancer 7, 1497–1514. doi:10.7150/jca.14922
Prabhu, L., Mundade, R., Korc, M., Loehrer, P. J., and Lu, T. (2014). Critical role of NF-κB in pancreatic cancer. Oncotarget 5 (22), 10969–10975. doi:10.18632/oncotarget.2624
Pramanik, K. C., Makena, M. R., Bhowmick, K., and Pandey, M. K. (2018). Advancement of NF-κB signaling pathway: a novel target in pancreatic cancer. Int. J. Mol. Sci. 19 (12), 3890. doi:10.3390/ijms19123890
Quatannens, D., Verhoeven, Y., Van Dam, P., Lardon, F., Prenen, H., Roeyen, G., et al. (2022). Targeting hedgehog signaling in pancreatic ductal adenocarcinoma. Pharmacol. and Ther. 236, 108107. doi:10.1016/j.pharmthera.2022.108107
Raimondi, S., Maisonneuve, P., and Lowenfels, A. B. (2009). Epidemiology of pancreatic cancer: an overview. Nat. Rev. Gastroenterology and Hepatology 6 (12), 699–708. doi:10.1038/nrgastro.2009.177
Rane, S. G., Lee, J. H., and Lin, H. M. (2006). Transforming growth factor-beta pathway: role in pancreas development and pancreatic disease. Cytokine Growth Factor Rev. 17 (1-2), 107–119. doi:10.1016/j.cytogfr.2005.09.003
Rawla, P., Sunkara, T., and Gaduputi, V. (2019). Epidemiology of pancreatic cancer: global trends, etiology and risk factors. World J. Oncol. 10, 10–27. doi:10.14740/wjon1166
Rizk, A. A., Dybala, M. P., Rodriguez, K. C., Slak Rupnik, M., and Hara, M. (2023). Pancreatic regional blood flow links the endocrine and exocrine diseases. J. Clin. Investig. 133 (15), e166185. doi:10.1172/JCI166185
Rocha, C. R. R., Silva, M. M., Quinet, A., Cabral-Neto, J. B., and Menck, C. F. M. (2018). DNA repair pathways and cisplatin resistance: an intimate relationship. Clinics 73, e478s. doi:10.6061/clinics/2018/e478s
Rosanas-Urgell, A., Marfany, G., and Garcia-Fernandez, J. (2005). Pdx1-related homeodomain transcription factors are distinctly expressed in mouse adult pancreatic islets. Mol. Cell. Endocrinol. 237 (1-2), 59–66. doi:10.1016/j.mce.2005.03.008
Roy, N., Takeuchi, K. K., Ruggeri, J. M., Bailey, P., Chang, D., Li, J., et al. (2016). PDX1 dynamically regulates pancreatic ductal adenocarcinoma initiation and maintenance. Genes. Dev. 30 (24), 2669–2683. doi:10.1101/gad.291021.116
Ruiz-Borrego, M., Jimenez, B., Antolín, S., García-Saenz, J. A., Corral, J., Jerez, Y., et al. (2019). A phase Ib study of sonidegib (LDE225), an oral small molecule inhibitor of smoothened or Hedgehog pathway, in combination with docetaxel in triple negative advanced breast cancer patients: GEICAM/2012–12 (EDALINE) study. Investig. New Drugs 37, 98–108. doi:10.1007/s10637-018-0614-9
Sabbadini, F., Bertolini, M., De Matteis, S., Mangiameli, D., Contarelli, S., Pietrobono, S., et al. (2021). The multifaceted role of TGF-β in gastrointestinal tumors. Cancers 13 (16), 3960. doi:10.3390/cancers13163960
Safari, M., Scotto, L., Basseville, A., Litman, T., Xue, H., Petrukhin, L., et al. (2023). Abstract LB_C03: protein translation inhibition enforces histone deacetylase inhibitor activity resulting in synergistic pancreatic cancer cell death. Mol. Cancer Ther. 22 (12_Suppl. ment), LB_C03-. doi:10.1158/1535-7163.targ-23-lb_c03
Sally, Á., McGowan, R., Finn, K., and Moran, B. M. (2022). Current and future therapies for pancreatic ductal adenocarcinoma. Cancers 14 (10), 2417. doi:10.3390/cancers14102417
Schaal, C., and Chellappan, S. P. (2014). Nicotine-mediated cell proliferation and tumor progression in smoking-related cancers. Mol. Cancer Res. 12, 14–23. doi:10.1158/1541-7786.MCR-13-0541
Scheibner, K., Bakhti, M., Bastidas-Ponce, A., and Lickert, H. (2019). Wnt signaling: implications in endoderm development and pancreas organogenesis. Curr. Opin. Cell. Biol. 61, 48–55. doi:10.1016/j.ceb.2019.07.002
Sever, D., Hershko-Moshe, A., Srivastava, R., Eldor, R., Hibsher, D., Keren-Shaul, H., et al. (2021). NF-κB activity during pancreas development regulates adult β-cell mass by modulating neonatal β-cell proliferation and apoptosis. Cell. Death Discov. 7, 2. doi:10.1038/s41420-020-00386-9
Seymour, P. A., Freude, K. K., Dubois, C. L., Shih, H. P., Patel, N. A., and Sander, M. (2008). A dosage-dependent requirement for Sox9 in pancreatic endocrine cell formation. Dev. Biol. 323 (1), 19–30. doi:10.1016/j.ydbio.2008.07.034
Sharma, N., Nanta, R., Sharma, J., Gunewardena, S., Singh, K. P., Shankar, S., et al. (2015). PI3K/AKT/mTOR and sonic hedgehog pathways cooperate together to inhibit human pancreatic cancer stem cell characteristics and tumor growth. Oncotarget 6, 32039–32060. doi:10.18632/oncotarget.5055
Sidis, Y., Mukherjee, A., Keutmann, H., Delbaere, A., Sadatsuki, M., and Schneyer, A. (2006). Biological activity of follistatin isoforms and follistatin-like-3 is dependent on differential cell surface binding and specificity for activin, myostatin, and bone morphogenetic proteins. Endocrinology 147, 3586–3597. doi:10.1210/en.2006-0089
Silke, J., and O’Reilly, L. A. (2021). NF-κB and pancreatic cancer; chapter and verse. Cancers 13 (18), 4510. doi:10.3390/cancers13184510
Sirico, M., D’Angelo, A., Gianni, C., Casadei, C., Merloni, F., and De Giorgi, U. (2023). Current state and future challenges for PI3K inhibitors in cancer therapy. Cancers 15 (3), 703. doi:10.3390/cancers15030703
Smith, A. L., Robin, T. P., and Ford, H. L. (2012). Molecular pathways: targeting the TGF-β pathway for cancer therapy. Clin. Cancer Res. 18 (17), 4514–4521. doi:10.1158/1078-0432.CCR-11-3224
Sosa, J., Oyelakin, A., and Sinha, S. (2024). The reign of follistatin in tumors and their microenvironment: implications for drug resistance. Biology 13 (2), 130. doi:10.3390/biology13020130
Srivani, G., Bethi, S. R., Aliya, S., Alam, A., and Nagaraju, G. P. (2018). “VEGFR and PDGFR targeting in pancreatic cancer,” in Role of tyrosine kinases in gastrointestinal malignancies (Singapore: Springer Singapore), 83–96. doi:10.1007/978-981-13-1486-5_7
Stanciu, S., Ionita-Radu, F., Stefani, C., Miricescu, D., Stanescu-Spinu, I.-I., Greabu, M., et al. (2022). Targeting PI3K/AKT/mTOR signaling pathway in pancreatic cancer: from molecular to clinical aspects. Int. J. Mol. Sci. 23, 10132. doi:10.3390/ijms231710132
Strickler, J. H., Satake, H., George, T. J., Yaeger, R., Hollebecque, A., Garrido-Laguna, I., et al. (2023). Sotorasib in KRAS p. G12C–mutated advanced pancreatic cancer. N. Engl. J. Med. 388 (1), 33–43. doi:10.1056/NEJMoa2208470
Svensson, P., Williams, C., Lundeberg, J., Rydén, P., Bergqvist, I., and Edlund, H. (2007). Gene array identification of Ipf1/Pdx1-/-regulated genes in pancreatic progenitor cells. BMC Dev. Biol. 7, 129. doi:10.1186/1471-213X-7-129
Tao, J., Yang, G., Zhou, W., Qiu, J., Chen, G., Luo, W., et al. (2021). Targeting hypoxic tumor microenvironment in pancreatic cancer. J. Hematol. Oncol. 14, 14. doi:10.1186/s13045-020-01030-w
Tian, T., Li, X., and Zhang, J. (2019). mTOR signaling in cancer and mTOR inhibitors in solid tumor targeting therapy. Int. J. Mol. Sci. 20, 755. doi:10.3390/ijms20030755
Tidyman, W. E., and Rauen, K. A. (2009). The RASopathies: developmental syndromes of Ras/MAPK pathway dysregulation. Curr. Opin. Genet. and Dev. 19 (3), 230–236. doi:10.1016/j.gde.2009.04.001
Truty, M. J., and Urrutia, R. (2007). Basics of TGF-beta and pancreatic cancer. Pancreatology 7 (5-6), 423–435. doi:10.1159/000108959
Vara, J. Á., Casado, E., de Castro, J., Cejas, P., Belda-Iniesta, C., and González-Barón, M. (2004). PI3K/Akt signalling pathway and cancer. Cancer Treat. Rev. 30 (2), 193–204. doi:10.1016/j.ctrv.2003.07.007
Waldner, M. J., and Neurath, M. F. (2012). Targeting the VEGF signaling pathway in cancer therapy. Expert Opin. Ther. targets 16 (1), 5–13. doi:10.1517/14728222.2011.641951
Wang, H.-L., Wang, L., Zhao, C.-Y., and Lan, H.-Y. (2022). Role of TGF-beta signaling in beta cell proliferation and function in diabetes. Biomolecules 12, 373. doi:10.3390/biom12030373
Wang, J., Chan, D. K. W., Sen, A., Ma, W. W., and Straubinger, R. M. (2019). Tumor priming by SMO inhibition enhances antibody delivery and efficacy in a pancreatic ductal adenocarcinoma model. Mol. Cancer Ther. 18, 2074–2084. doi:10.1158/1535-7163.MCT-18-0354
Wang, S., Huang, S., and Sun, Y. L. (2017). Epithelial-mesenchymal transition in pancreatic cancer: a review. Biomed. Res. Int. 2017, 2646148–2646210. doi:10.1155/2017/2646148
Weissman, S., Takakura, K., Eibl, G., Pandol, S. J., and Saruta, M. (2020). The diverse involvement of cigarette smoking in pancreatic cancer development and prognosis. Pancreas 49, 612–620. doi:10.1097/MPA.0000000000001550
Wells, J. M. (2003). Genes expressed in the developing endocrine pancreas and their importance for stem cell and diabetes research. Diabetes/metabolism Res. Rev. 19 (3), 191–201. doi:10.1002/dmrr.364
Welt, C., Sidis, Y., Keutmann, H. T., and Schneyer, A. (2002). Activins, inhibins, and follistatins: from endocrinology to signaling. A paradigm for the new millennium. Exp. Biol. Med. 227, 724–752. doi:10.1177/153537020222700905
Wood, L. D. (2013). Pancreatic cancer genomes: toward molecular subtyping and novel approaches to diagnosis and therapy. Mol. Diagn Ther. 17, 287–297. doi:10.1007/s40291-013-0043-6
Wu, D., Qiu, Y., Jiao, Y., Qiu, Z., and Liu, D. (2020). Small molecules targeting HATs, HDACs, and BRDs in cancer therapy. Front. Oncol. 10, 560487. doi:10.3389/fonc.2020.560487
Xia, Y., and Schneyer, A. L. (2009). The biology of activin: recent advances in structure, regulation and function. J. Endocrinol. 202 (1), 1–12. Epub 2009 Mar 9. PMID: 19273500; PMCID: PMC2704481. doi:10.1677/JOE-08-0549
Xiang, X. S., Li, P. C., Wang, W. Q., and Liu, L. (2022). Histone deacetylases: a novel class of therapeutic targets for pancreatic cancer. Biochimica Biophysica Acta (BBA)-Reviews Cancer 1877 (1), 188676. doi:10.1016/j.bbcan.2022.188676
Xie, H., Paradise, B. D., Ma, W. W., and Fernandez-Zapico, M. E. (2019). Recent advances in the clinical targeting of hedgehog/GLI signaling in cancer. Cells 8, 394. doi:10.3390/cells8050394
Yang, J., Nie, J., Ma, X., Wei, Y., Peng, Y., and Wei, X. (2019). Targeting PI3K in cancer: mechanisms and advances in clinical trials. Mol. Cancer 18, 26. doi:10.1186/s12943-019-0954-x
Ying, X., Shen, X., Cheng, L., Hong, D., and Chen, B. (2014). Perifosine inhibits S6K1–Gli1 signaling and enhances gemcitabine-induced anti-pancreatic cancer efficiency. Cancer Chemother. Pharmacol. 73, 711–719. doi:10.1007/s00280-014-2397-9
Yoshitomi, H., and Zaret, K. S. (2004). Endothelial cell interactions initiate dorsal pancreas development by selectively inducing the transcription factor Ptf1a. Development 131 (4), 807–817. doi:10.1242/dev.00960
You, W. K., Schuetz, T. J., and Lee, S. H. (2023). Targeting the DLL/Notch signaling pathway in cancer: challenges and advances in clinical development. Mol. cancer Ther. 22 (1), 3–11. doi:10.1158/1535-7163.MCT-22-0243
Yuen, A., and Díaz, B. (2014). The impact of hypoxia in pancreatic cancer invasion and metastasis. Hypoxia 7, 91–106. doi:10.2147/HP.S52636
Zanini, S., Renzi, S., Limongi, A. R., Bellavite, P., Giovinazzo, F., and Bermano, G. (2021). A review of lifestyle and environment risk factors for pancreatic cancer. Eur. J. Cancer 145, 53–70. doi:10.1016/j.ejca.2020.11.040
Zeng, G., Germinaro, M., Micsenyi, A., Monga, N. K., Bell, A., Sood, A., et al. (2006). Aberrant Wnt/beta-catenin signaling in pancreatic adenocarcinoma. Neoplasia 8 (4), 279–289. doi:10.1593/neo.05607
Keywords: pancreatic cancer, transcription factor, signaling pathway, therapeutic approach, embryogenesis
Citation: Swain S, Narayan RK and Mishra PR (2024) Unraveling the interplay: exploring signaling pathways in pancreatic cancer in the context of pancreatic embryogenesis. Front. Cell Dev. Biol. 12:1461278. doi: 10.3389/fcell.2024.1461278
Received: 08 July 2024; Accepted: 13 August 2024;
Published: 22 August 2024.
Edited by:
Shashi Anand, University of Mississippi Medical Center, United StatesReviewed by:
Harit Panda, Washington University in St. Louis, United StatesSanthosh Shanthi Bhupathi, West Virginia University, United States
Copyright © 2024 Swain, Narayan and Mishra. This is an open-access article distributed under the terms of the Creative Commons Attribution License (CC BY). The use, distribution or reproduction in other forums is permitted, provided the original author(s) and the copyright owner(s) are credited and that the original publication in this journal is cited, in accordance with accepted academic practice. No use, distribution or reproduction is permitted which does not comply with these terms.
*Correspondence: Pravash Ranjan Mishra, YW5hdF9wcmF2YXNoQGFpaW1zYmh1YmFuZXN3YXIuZWR1Lmlu
†These authors have contributed equally to this work