- 1Graduate School of Computer Science and Systems Engineering, Kyushu Institute of Technology, Fukuoka, Japan
- 2Department of Physics and Information Technology, Faculty of Computer Science and Systems Engineering, Kyushu Institute of Technology, Fukuoka, Japan
- 3Japan Science and Technology Agency, PRESTO, Kawaguchi, Japan
The bacterial signaling molecule cyclic diguanosine monophosphate (c-di-GMP) is only synthesized and utilized by the cellular slime mold Dictyostelium discoideum among eukaryotes. Dictyostelium cells undergo a transition from a unicellular to a multicellular state, ultimately forming a stalk and spores. While Dictyostelium is known to employ c-di-GMP to induce differentiation into stalk cells, there have been no reports of direct observation of c-di-GMP using fluorescent probes. In this study, we used a fluorescent probe used in bacteria to visualize its localization within Dictyostelium multicellular bodies. Cytosolic c-di-GMP concentrations were significantly higher at the tip of the multicellular body during stalk formation.
1 Introduction
Cyclic diguanylate monophosphate (c-di-GMP) is a critical signaling molecule involved in diverse cellular processes including biofilm formation, motility, virulence factor production, and cell-cycle progression in prokaryotes (Romling et al., 2013; Jenal et al., 2017; Cheang et al., 2019; Homma and Kojima, 2022). In bacteria, intracellular c-di-GMP levels are tightly regulated to control the transition between the motile planktonic and the sessile biofilm states (Krasteva et al., 2012). Whereas c-di-GMP is a major signaling molecule in prokaryotes, it is rarely utilized in eukaryotes; it is an external stimulus in the innate immune response acting through the cyclic GMP–AMP synthase (cGAS)–stimulator of interferon genes (STING) pathway (Burdette et al., 2011).
On the other hand, the cellular slime mold Dictyostelium discoideum is the only eukaryote known to synthesize c-di-GMP and use it as an intercellular signal (Chen and Schaap, 2012; Kin and Schaap, 2021). It normally grows in a unicellular state and feeds on surrounding bacteria. However, when starved, its single cells aggregate by chemotaxis toward cAMP signals to form a multicellular body consisting of 104 to 105 cells. The anterior and posterior regions of this body (also called a slug) differentiate into prestalk and prespore cells, respectively; after a short migration, the slug go through a period of a culminant, eventually differentiates into a fruiting body composed of a stalk and spores (Chisholm and Firtel, 2004; Weijer, 2004; Loomis, 2015). During this process, c-di-GMP is produced by DgcA synthase, localized to the anterior region of the multicellular body. It contributes significantly to the differentiation of stalk cells by acting on adenylate cyclase A (AcaA) to stimulate the synthesis of cAMP, which in turn activates PKA and induces the expression of stalk-specific genes. While DgcA is widely expressed in the prestalk region, AcaA is expressed only in the tip region (Chen et al., 2017). It is unclear what mechanisms are responsible for this difference in spatial distribution.
D. discoideum is a model organism for the study of signal transduction, and sensitive fluorescence measurements of second messengers, including Ca2+, cAMP, and inositol phospholipids, have been reported (Arai et al., 2010; Gregor et al., 2010; Horikawa et al., 2010; Fukushima et al., 2019; Hashimura et al., 2019; Banerjee et al., 2022; Hashimura et al., 2022). However, c-di-GMP has never been observed using fluorescence techniques in Dictyostelium.
Several probes have been developed to detect intracellular c-di-GMP in living bacteria (Christen et al., 2010; Ho et al., 2013; Dippel et al., 2018; Halte et al., 2022). YcgR, a PilZ domain-containing protein, undergoes a conformational change upon c-di-GMP binding, functioning as a brake on the bacterial flagellar motor (Paul et al., 2010). A probe that changes FRET efficiency in a c-di-GMP concentration-dependent manner has been constructed by fusing yellow fluorescent protein (YFP) and cyan fluorescent protein (CFP) to the N- and C-termini of YcgR from Salmonella enterica serovar Typhimurium, respectively, to visualize asymmetric intracellular concentrations during cell divisions of Caulobacter crescentus (Christen et al., 2010). Fluorescent probes utilizing the PilZ protein MrkH, c-di-GMP-dependent dimerization of BldD, and a BRET probe fusing a YFP, Venus, and Split RLuc to YcgR have also been developed to visualize c-di-GMP in bacterial cells (Ho et al., 2013; Dippel et al., 2018; Halte et al., 2022). In this study, we have shown that the c-di-GMP probe YFP-YcgR-CFP also works in Dictyostelium cells to allow live imaging of c-di-GMP signaling in multicellular stages.
2 Materials and methods
2.1 Cell strains and culture conditions
D. discoideum strains used in this study are listed in Supplementary Table S1. Cells were grown axenically in HL5 medium including glucose (Formedium, United Kingdom) in 90-mm culture dishes at 21°C. Cells for fluorescence imaging were grown in low-fluorescence axenic medium containing 1 mL 1000 × FM salts 1, 1 mL 1000 × FM salts 2, 0.1 mL 10000 × FM trace elements, 11 g glucose·1H2O, 5 mL 1 M K2HPO4 and 5 g casein peptone per liter, and the pH was adjusted to 6.5 as described in DictyBase (http://dictybase.org/). 1000 × FM salts 1 contains 500 mM NH4Cl, 200 mM MgCl2 and 10 mM CaCl2. 1000 × FM salts 2 is 50 mM FeCl3. 10000 × FM trace elements is 100 mM Na2-EDTA·2H2O, 130 mM ZnSO4·H2O, 140 mM H3BO4, 25 mM MnCl2·4H2O, 7 mM CoCl2·6H2O, 6 mM CuSO4·5H2O, and 0.18 mM (NH4)6Mo7O24·4H2O. Transformants were maintained in medium containing 20 μg/mL G418 (Fujifilm Wako, Japan).
2.2 Plasmid construction and genetic manipulation
Plasmids used in this study are listed in Supplementary Table S2. pDM304/mYpet-YcgR-mCypet was constructed by insertion of a mYpet-YcgR-mCypet fragment from addgene plasmid #90102 into the BglII and SpeI sites of pDM304 (Veltman et al., 2009). The R118A point mutation was introduced to pDM304/mYpet-YcgR-mCypet plasmid by site-directed mutagenesis method (Kunkel, 1985) using DNA polymerase (KOD One, TOYOBO) and primers. The wild-type AX2 strain was transformed with ∼1.5 μg plasmid using a electroporator (MicroPulser, Bio-Rad); transformants were selected with 20 μg/mL G418.
2.3 Fluorescence spectrophotometry
Cells expressing the FRET sensor were starved in developmental buffer (DB: 5 mM Na2HPO4, 5 mM KH2PO4, 2 mM MgCl2, 0.2 mM CaCl2, pH 6.5) at a density of 1.0 × 107/mL for 3 h and lysed in ice cold lysis buffer containing 10 mM Tris-HCl, pH7.5, 0.2 mM EGTA, 200 mM sucrose as described by Bagorda et al. (Bagorda et al., 2009). The fluorescence intensities with or without 0.1, 0.5, 1.0, or 10 µM c-di-GMP (SML1228, Sigma-Aldrich) were measured with excitation 430 nm and emission 460 and 520 nm using fluorescence spectrophotometer (FP-8550, Jasco). The ratio of CFP/FRET values were calculated the emission value at 460 nm divided by the emission value at 520 nm.
2.4 Image acquisition and analysis
In all experiments, cells were observed at 21°C. Microscopic images were taken using an inverted microscope (IX83, Evident) equipped with an sCMOS camera (Prime 95B, Photometrics) and objectives (UPLSAPO 20X/0.75 NA or UPLXAPO 40X/0.95 NA, Evident). The c-di-GMP sensor was excited by a 130 W mercury light source system (U-HGLGPS, Evident) with a fluorescence mirror unit CFP-2432C-OFF (Excitation BP 438/24; Emission BP 483/32, Semrock) for CFP images and a customized mirror unit (Excitation BP 438/24; Emission BP 542/27, Semrock) for FRET images. All images were processed and analyzed using Fiji (Schindelin et al., 2012). Background intensity was defined as the mean pixel intensity of the ROI without cells. For ratiometric imaging, the fluorescence intensity of the CFP image was divided by the intensity of the FRET image using the CFP and FRET images subtracted the background intensity, respectively.
2.5 FRET imaging
To induce development, cells at the exponential phase (1.5–3 × 106/mL) were harvested and washed three times in reverse osmosis (RO) water. Multicellular bodies were allowed to form on agar plates and observed as described (Hashimura et al., 2019). Multicellular bodies were imaged using inverted fluorescence microscopy.
3 Results
3.1 Expression of the c-di-GMP fluorescent sensor
The FRET-based c-di-GMP sensor utilized in bacteria (Christen et al., 2010) was expressed under the constitutive act15 promoter in D. discoideum AX2. It consists of mYpet and mCypet fused to the N- and C-termini of Salmonella YcgR, respectively. Prediction of the sensor protein structure was performed using a machine-learning model AlphaFold2 (Jumper et al., 2021) (Figure 1A). Since the FRET efficiency of the sensor decreases when bound to c-di-GMP, an increase in c-di-GMP concentration decreases FRET efficiency (Figure 1B). To confirm that the FRET sensor is functional, cells expressing it were lysed and the dependence of the FRET efficiency on c-di-GMP concentration for the sensor produced in Dictyostelium cells was examined. Changes in fluorescence intensity with or without 0.1, 0.5, 1.0 or 10 µM c-di-GMP were measured using spectrophotometry. Increasing concentrations of c-di-GMP added to the lysate decreased FRET efficiency and increased the CFP/FRET ratio (p < 0.001, one-way ANOVA), confirming its utility as a sensor (Figure 1C). Since the 118th arginine residue of YcgR is conserved and known to be critical for c-di-GMP binding (Ryjenkov et al., 2006; Hou et al., 2020), the single-residue substitution R118A was made to YcgR, yielding mYpet-YcgR(R118A)-mCypet, and the lysate of cells expressing it were tested, but no significant FRET changes for c-di-GMP were observed (one-way ANOVA) (Figure 1D). Considering that Dictyostelium responds to more than 1 µM external c-di-GMP stimulation for gene expression (Chen et al., 2017) and that c-di-GMP functions at concentrations ranging from tens of nM to tens of µM in bacterial cells (Christen et al., 2010; Pultz et al., 2012) that utilize c-di-GMP, this sensor is suitable for intracellular c-di-GMP monitoring.
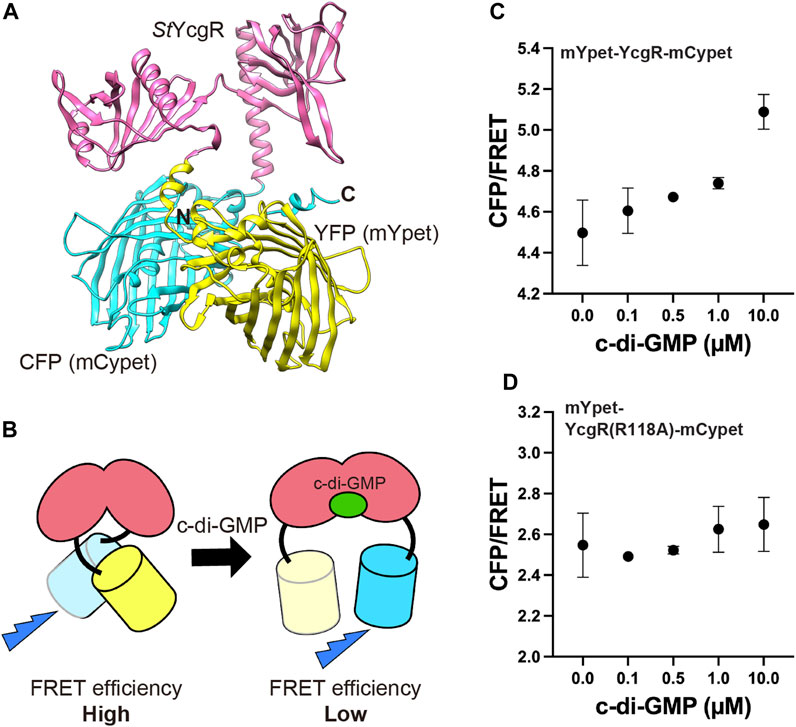
FIGURE 1. FRET c-di-GMP sensor. (A) Structure of mYpet-YcgR-mCypet (Christen et al., 2010) predicted by AlphaFold2. The sensor is a fusion of mYpet (yellow), Salmonella YcgR (magenta) and mCypet (cyan). (B) c-di-GMP sensor have decreased FRET efficiency when c-di-GMP binds to the YcgR part. FRET efficiencies of the mYpet-YcgR-mCypet (C) and mYpet-YcgR(R118A)-mCypet (D) from Dictyostelium cell lysates with or without 0.1, 0.5, 1.0, or 10 µM c-di-GMP at 23°C, respectively. CFP/FRET values were calculated CFP intensity divided by FRET intensity. Measurements were taken four times for each concentration. Error bars show standard deviations.
3.2 Fluorescent imaging of c-di-GMP in multicellular bodies
The fluorescence of c-di-GMP in single cells could be measured, but its brightness was not high; moreover, the FRET changes were not very large (Figure 1C). To better capture subtle changes in intracellular FRET, it was necessary to suppress autofluorescence. Utilizing a medium that has been shown to reduce autofluorescence described in DictyBase (Basu et al., 2015), the autofluorescence of multicellular bodies could be reduced at the emission wavelength (Supplementary Figure S1). Cells expressing the c-di-GMP sensor were cultured in this low-fluorescence medium and then starved to initiate development. In culminant stages, the c-di-GMP signal was observed to be greater in the tip region (Figure 2), where the boundary between the prestalk and prespore regions can be distinguished by a neck (Bonner, 1998; Gaudet et al., 2008). However, we observed no difference in signal between the prespore region and the prestalk regions, except for the tip (Figure 2E). This high concentration in the tip is not consistent with the reported distribution of its synthetic enzyme DgcA (Chen and Schaap, 2012), but is consistent with the distribution of the induced adenylyl cyclase AcaA, thought to be activated by c-di-GMP (Chen et al., 2017). Since the Kd of the c-di-GMP sensor is 195 nM (Christen et al., 2010), we infer that the tip area has a concentration of at least several hundred nM.
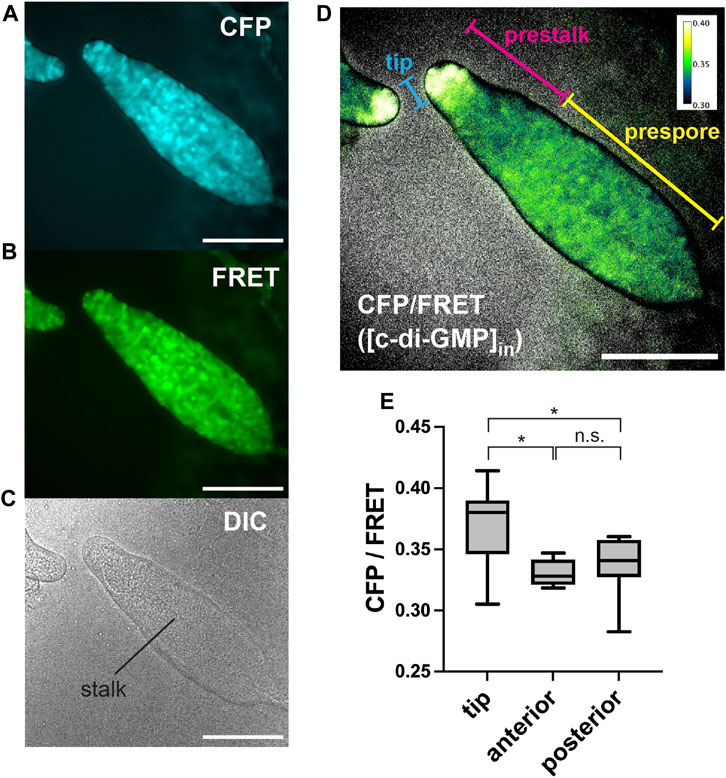
FIGURE 2. Representative images of c-di-GMP in Dictyostelium multicellular bodies of CFP (A), FRET (B), and DIC (C). (D) Ratiometric FRET image created by dividing the CFP image by the FRET image. Ratios are shown in pseudocolor (inset). Scale bars, 100 µm. (E) CFP/FRET ratios for multicellular body regions (n = 9). Anterior is defined as the prestalk region minus the tip region. Posterior is the prespore region. *p < 0.05 (ANOVA with Tukey’s multiple comparisons test); n.s., no significant difference.
3.3 Time-lapse measurement of c-di-GMP during development
To determine at what point during development the concentration of c-di-GMP in the tip begins, its signal was monitored during development from initial multicellularity (13 h after starvation) to the formation of fruiting bodies (Figure 3). Immediately after slug formation, there was no clear localization of c-di-GMP, but a strong signal at the tip was observed when stalk formation was confirmed inside the multicellular body (Figures 3A, B). Figure 2, showing c-di-GMP localization, also shows stalk formation (Figure 2C). Although cellular autofluorescence increases with fruiting body formation, we observed no significant difference between the tip and other regions in AX2 multicellular bodies not expressing fluorescent sensors (Supplementary Figure S2). The mYpet-YcgR(R118A)-mCypet does not respond significantly to c-di-GMP (Figure 1D). Time-lapse measurements of the development of cells expressing this probe showed no significant change in FRET efficiency (Figure 4A). The faint but non-significant difference in the tip region after 18.5 h may be due to the weak affinity of the YcgR(R118A) mutant, which has been reported to be Kd of 14 µM (Hou et al., 2020) (Figure 4). These results suggest that a large amount of c-di-GMP is synthesized during stalk formation at the tip of the culminant.
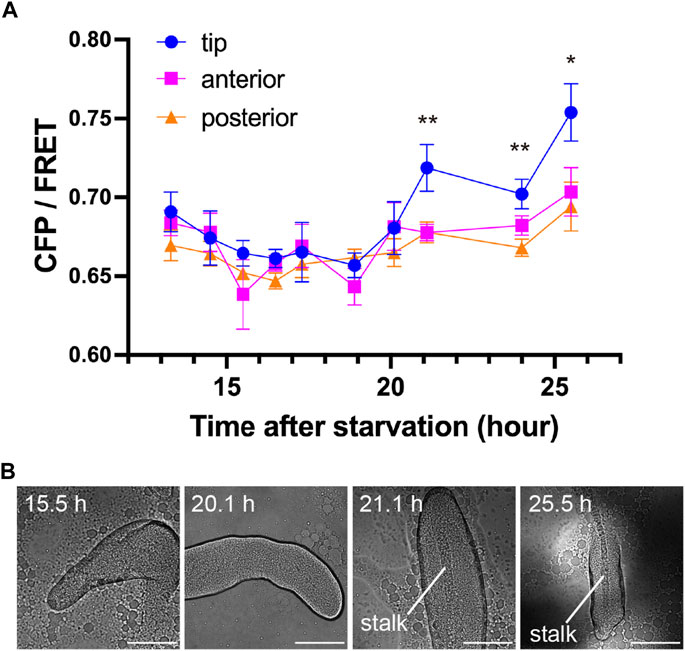
FIGURE 3. Transition of c-di-GMP synthesis during development. (A) CFP/FRET ratios for each region within the multicellular body at each time point. Means ± standard errors are shown (n = 19); *p < 0.05; **p < 0.01 (ANOVA with Tukey’s multiple comparisons test). The absence of asterisks indicates no significant difference. (B) Representative DIC micrographs of Dictyostelium multicellular bodies at four time points. Scale bars, 100 µm.
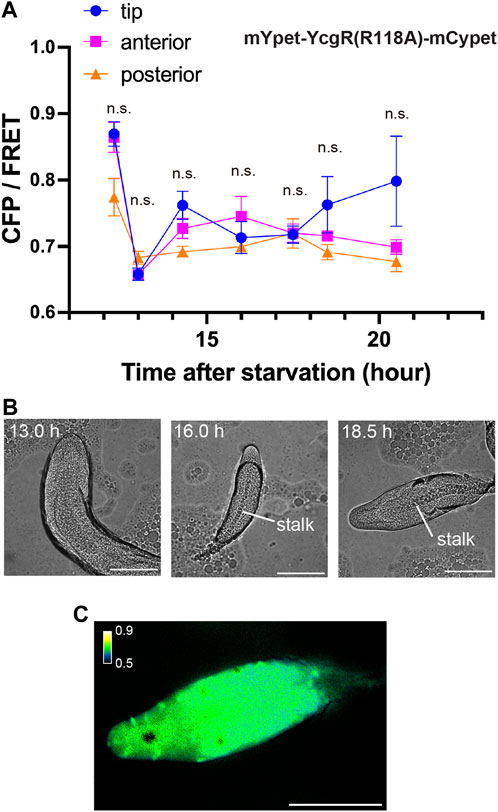
FIGURE 4. Measurement of mutant mYpet-YcgR(R118A)-mCypet during development. (A) CFP/FRET ratios of mYpet-YcgR(R118A)-mCypet for regions of the multicellular body at each time point. Means ± standard deviations are shown (n = 19). Data were assessed using ANOVA with Tukey’s multiple comparisons test; n.s., no significant difference. (B) Representative DIC micrographs of Dictyostelium multicellular bodies at three time points in development. (C) A representative ratiometric FRET image of the multicellular body at 18.5 h. Scale bars, 100 µm.
3.4 c-di-GMP in the dgcA mutant
D. discoideum, DgcA synthase is primarily responsible for the synthesis of c-di-GMP (Chen and Schaap, 2012; Song et al., 2015; Chen et al., 2017). The dgcA mutant does not form fruiting bodies because c-di-GMP does not induce stalk-cell differentiation, but they do progress to the slug stage (Chen and Schaap, 2012). FRET in the dgcA mutant was performed after multicellular body formation, with no significant difference between the regions (Figure 5). This indicates that the wild-type c-di-GMP signal (Figures 2D, 3A) depends on the c-di-GMP synthesized by DgcA synthase.
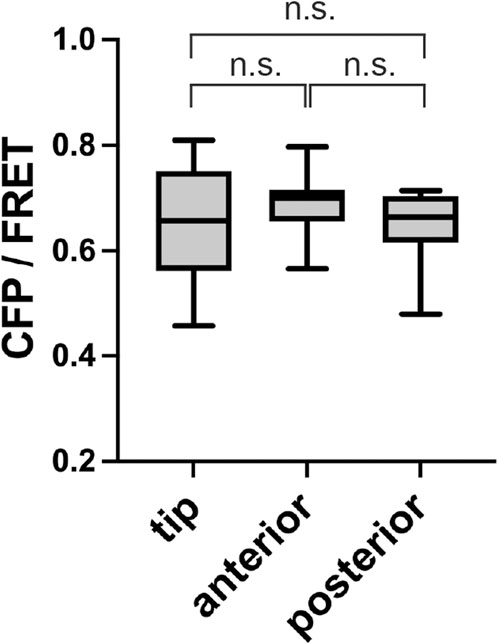
FIGURE 5. No localization of c-di-GMP in a dgcA mutant. CFP/FRET ratios for each slug region are shown as box plots (n = 10). Anterior is defined as the prestalk region minus the tip region. Posterior is the prespore region. Data were assessed with ANOVA with Tukey’s multiple comparisons test; n.s., no significant difference.
4 Discussion
In D. discoideum, stalk cell differentiation is induced by c-di-GMP and DIF-1 (Morris et al., 1987; Thompson and Kay, 2000; Saito et al., 2008; Chen and Schaap, 2012; Kin and Schaap, 2021). Since this differentiation also induces cell death, it provides information on cell-death mechanisms (Song et al., 2015). The presence of c-di-GMP activates AcaA in tip cells and cAMP synthesized by AcaA in turn activates PKA to induce the differentiation of prestalk cells into stalk (Chen et al., 2017). Conversely, ammonia acts on RegA via DhkC, which suppresses cAMP signaling, thereby inhibiting stalk differentiation (Schindler and Sussman, 1977; Singleton et al., 1998). Our observation of c-di-GMP accumulation in the tip during stalk formation (Figures 2, 3) is consistent with the localization of AcaA (Verkerke-van Wijk et al., 2001), supporting the previous spatiotemporal model (Chen et al., 2017). On the other hand, the fact that the distribution of the synthetic enzyme DgcA did not match the distribution of c-di-GMP may indicate that DgcA is activated only in tip cells, or that c-di-GMP synthesis is suppressed in prestalk cells except for the tip. The activity of some bacterial diguanylates are known to be regulated by partner proteins (Chen et al., 2021). There are proteins that are expressed only at the tip or prestalk region of Dictyostelium multicellular bodies (Williams, 2006); it is possible that they include regulatory partners for DgcA activity. Alternatively, since acidification is known to act on stalk differentiation (Gross et al., 1983), differences in the cellular environment, such as intracellular pH, may regulate DgcA activity spatially and temporally.
Cells not expressing the sensor, or expressing a probe that did not bind c-di-GMP, had normal stalk development at 16 h or more after starvation; however, in cells expressing the c-di-GMP sensor, stalk formation was delayed to 21 h or more after starvation (Figures 3, 4). This is likely due to the binding of c-di-GMP to the sensor reducing intracellular free c-di-GMP. This is also an indication that c-di-GMP is important for stalk formation.
This study visualized c-di-GMP signaling in eukaryotes for the first time (Figure 2). To elucidate more of the spatiotemporal dynamics of c-di-GMP at the single-cell or single-local levels, it is necessary to increase sensor sensitivity. The Kd of the sensor used in this study is approximately 195 nM (Christen et al., 2010), making it suitable for Dictyostelium because c-di-GMP stimulations above 1 µM induce expression of stalk-specific genes (Chen et al., 2017); however, we only observed significant differences during stalk-cell formation, which is considered to have the highest intracellular c-di-GMP concentration. DgcA is widely expressed in prestalk cells and may function at lower concentrations; alternatively, it may be produced at an earlier time point. PilZ domain protein PA3353 from Pseudomonas aeruginosa has two binding sites, one of which with a Kd of 88 nM, and may be used as a sensitive c-di-GMP sensor (Pultz et al., 2012). As development progresses to multicellular and fruiting bodies, autofluorescence increases, which affects the fluorescence ratio imaging (Figure 4A; Figure 5). The BRET sensor may be useful for measurement with high signal-to-noise ratio, because it can greatly decrease artifacts caused by autofluorescence (Dippel et al., 2018). The c-di-GMP sensor used in this study was not bright enough and required intense excitation, making it difficult to measure at the single-cell level. Since c-di-GMP acts as an extracellular signal (Chen and Schaap, 2016; Chen et al., 2017), measurement of signal dynamics upon stimulation is important. Recently, multiple stable fluorescent proteins have been developed (Hirano et al., 2022), and their use in sensors may enable measurement of responses to external c-di-GMP stimulation.
D. discoideum synthesize and utilize c-di-GMP signal by themselves using lateral gene transfer from bacteria (Chen and Schaap, 2012; Kin and Schaap, 2021). Therefore, D. discoideum is the only known eukaryote species that uses c-di-GMP as a signal (Chen and Schaap, 2012). In mammalian cells, c-di-GMP is recognized as a bacterial signal by the STING pathway and innate immunity functions (Burdette et al., 2011; Yin et al., 2012). The use of Dictyostelium cells as a model for measuring c-di-GMP signaling in eukaryotic cells would provide a model for immunological studies.
Data availability statement
The original contributions presented in the study are included in the article/Supplementary Materials, further inquiries can be directed to the corresponding author.
Author contributions
YM conceived and designed research; HI, YH, and YM performed experiments; HI and YM analyzed data; and YM wrote the paper based on discussions with other authors. All authors contributed to the article and approved the submitted version.
Funding
This research has been supported in part by JSPS KAKENHI Grant Numbers JP21K06099, JP21H05532 and JP23H04082 (to YM) and JST PRESTO Grant Number JPMJPR204B (to YM).
Acknowledgments
We thank Yuko Baba and Chihiro Fukunaga for technical assistance and Takuo Yasunaga and Masahiro Ueda for continuous support and encouragement.
Conflict of interest
The authors declare that the research was conducted in the absence of any commercial or financial relationships that could be construed as a potential conflict of interest.
Publisher’s note
All claims expressed in this article are solely those of the authors and do not necessarily represent those of their affiliated organizations, or those of the publisher, the editors and the reviewers. Any product that may be evaluated in this article, or claim that may be made by its manufacturer, is not guaranteed or endorsed by the publisher.
Supplementary material
The Supplementary Material for this article can be found online at: https://www.frontiersin.org/articles/10.3389/fcell.2023.1237778/full#supplementary-material
References
Arai, Y., Shibata, T., Matsuoka, S., Sato, M. J., Yanagida, T., and Ueda, M. (2010). Self-organization of the phosphatidylinositol lipids signaling system for random cell migration. Proc. Natl. Acad. Sci. U. S. A. 107, 12399–12404. doi:10.1073/pnas.0908278107
Bagorda, A., Das, S., Rericha, E. C., Chen, D., Davidson, J., and Parent, C. A. (2009). Real-time measurements of cAMP production in live Dictyostelium cells. J. Cell. Sci. 122, 3907–3914. doi:10.1242/jcs.051987
Banerjee, T., Biswas, D., Pal, D. S., Miao, Y., Iglesias, P. A., and Devreotes, P. N. (2022). Spatiotemporal dynamics of membrane surface charge regulates cell polarity and migration. Nat. Cell. Biol. 24, 1499–1515. doi:10.1038/s41556-022-00997-7
Basu, S., Fey, P., Jimenez-Morales, D., Dodson, R. J., and Chisholm, R. L. (2015). dictyBase 2015: Expanding data and annotations in a new software environment. Genesis 53, 523–534. doi:10.1002/dvg.22867
Bonner, J. T. (1998). A way of following individual cells in the migrating slugs of Dictyostelium discoideum. Proc. Natl. Acad. Sci. U. S. A. 95, 9355–9359. doi:10.1073/pnas.95.16.9355
Burdette, D. L., Monroe, K. M., Sotelo-Troha, K., Iwig, J. S., Eckert, B., Hyodo, M., et al. (2011). STING is a direct innate immune sensor of cyclic di-GMP. Nature 478, 515–518. doi:10.1038/nature10429
Cheang, Q. W., Xin, L., Chea, R. Y. F., and Liang, Z. X. (2019). Emerging paradigms for PilZ domain-mediated C-di-GMP signaling. Biochem. Soc. Trans. 47, 381–388. doi:10.1042/BST20180543
Chen, G., Zhou, J., Zuo, Y., Huo, W., Peng, J., Li, M., et al. (2021). Structural basis for diguanylate cyclase activation by its binding partner in Pseudomonas aeruginosa. Elife 10, e67289. doi:10.7554/eLife.67289
Chen, Z. H., and Schaap, P. (2016). Secreted cyclic di-GMP induces stalk cell differentiation in the eukaryote Dictyostelium discoideum. J. Bacteriol. 198, 27–31. doi:10.1128/JB.00321-15
Chen, Z. H., and Schaap, P. (2012). The prokaryote messenger c-di-GMP triggers stalk cell differentiation in Dictyostelium. Nature 488, 680–683. doi:10.1038/nature11313
Chen, Z. H., Singh, R., Cole, C., Lawal, H. M., Schilde, C., Febrer, M., et al. (2017). Adenylate cyclase A acting on PKA mediates induction of stalk formation by cyclic diguanylate at the Dictyostelium organizer. Proc. Natl. Acad. Sci. U. S. A. 114, 516–521. doi:10.1073/pnas.1608393114
Chisholm, R. L., and Firtel, R. A. (2004). Insights into morphogenesis from a simple developmental system. Nat. Rev. Mol. Cell. Biol. 5, 531–541. doi:10.1038/nrm1427
Christen, M., Kulasekara, H. D., Christen, B., Kulasekara, B. R., Hoffman, L. R., and Miller, S. I. (2010). Asymmetrical distribution of the second messenger c-di-GMP upon bacterial cell division. Science 328, 1295–1297. doi:10.1126/science.1188658
Dippel, A. B., Anderson, W. A., Evans, R. S., Deutsch, S., and Hammond, M. C. (2018). Chemiluminescent biosensors for detection of second messenger cyclic di-GMP. ACS Chem. Biol. 13, 1872–1879. doi:10.1021/acschembio.7b01019
Fukushima, S., Matsuoka, S., and Ueda, M. (2019). Excitable dynamics of Ras triggers spontaneous symmetry breaking of PIP3 signaling in motile cells. J. Cell. Sci. 132, 224121. doi:10.1242/jcs.224121
Gaudet, P., Williams, J. G., Fey, P., and Chisholm, R. L. (2008). An anatomy ontology to represent biological knowledge in Dictyostelium discoideum. BMC Genomics 9, 130. doi:10.1186/1471-2164-9-130
Gregor, T., Fujimoto, K., Masaki, N., and Sawai, S. (2010). The onset of collective behavior in social amoebae. Science 328, 1021–1025. doi:10.1126/science.1183415
Gross, J. D., Bradbury, J., Kay, R. R., and Peacey, M. J. (1983). Intracellular pH and the control of cell differentiation in Dictyostelium discoideum. Nature 303, 244–245. doi:10.1038/303244a0
Halte, M., Wormann, M. E., Bogisch, M., Erhardt, M., and Tschowri, N. (2022). BldD-based bimolecular fluorescence complementation for in vivo detection of the second messenger cyclic di-GMP. Mol. Microbiol. 117, 705–713. doi:10.1111/mmi.14876
Hashimura, H., Morimoto, Y. V., Hirayama, Y., and Ueda, M. (2022). Calcium responses to external mechanical stimuli in the multicellular stage of Dictyostelium discoideum. Sci. Rep. 12, 12428. doi:10.1038/s41598-022-16774-3
Hashimura, H., Morimoto, Y. V., Yasui, M., and Ueda, M. (2019). Collective cell migration of Dictyostelium without cAMP oscillations at multicellular stages. Commun. Biol. 2, 34. doi:10.1038/s42003-018-0273-6
Hirano, M., Ando, R., Shimozono, S., Sugiyama, M., Takeda, N., Kurokawa, H., et al. (2022). A highly photostable and bright green fluorescent protein. Nat. Biotechnol. 40, 1132–1142. doi:10.1038/s41587-022-01278-2
Ho, C. L., Chong, K. S., Oppong, J. A., Chuah, M. L., Tan, S. M., and Liang, Z. X. (2013). Visualizing the perturbation of cellular cyclic di-GMP levels in bacterial cells. J. Am. Chem. Soc. 135, 566–569. doi:10.1021/ja310497x
Homma, M., and Kojima, S. (2022). Roles of the second messenger c-di-GMP in bacteria: Focusing on the topics of flagellar regulation and Vibrio spp. Genes. cells. 27, 157–172. doi:10.1111/gtc.12921
Horikawa, K., Yamada, Y., Matsuda, T., Kobayashi, K., Hashimoto, M., Matsu-Ura, T., et al. (2010). Spontaneous network activity visualized by ultrasensitive Ca2+ indicators, yellow Cameleon-Nano. Nat. Methods 7, 729–732. doi:10.1038/nmeth.1488
Hou, Y. J., Yang, W. S., Hong, Y., Zhang, Y., Wang, D. C., and Li, D. F. (2020). Structural insights into the mechanism of c-di-GMP-bound YcgR regulating flagellar motility in Escherichia coli. J. Biol. Chem. 295, 808–821. doi:10.1074/jbc.RA119.009739
Jenal, U., Reinders, A., and Lori, C. (2017). Cyclic di-GMP: Second messenger extraordinaire. Nat. Rev. Microbiol. 15, 271–284. doi:10.1038/nrmicro.2016.190
Jumper, J., Evans, R., Pritzel, A., Green, T., Figurnov, M., Ronneberger, O., et al. (2021). Highly accurate protein structure prediction with AlphaFold. Nature 596, 583–589. doi:10.1038/s41586-021-03819-2
Kin, K., and Schaap, P. (2021). Evolution of multicellular complexity in the Dictyostelid social amoebas. Genes. (Basel) 12, 487. doi:10.3390/genes12040487
Krasteva, P. V., Giglio, K. M., and Sondermann, H. (2012). Sensing the messenger: The diverse ways that bacteria signal through c-di-GMP. Protein Sci. 21, 929–948. doi:10.1002/pro.2093
Kunkel, T. A. (1985). Rapid and efficient site-specific mutagenesis without phenotypic selection. Proc. Natl. Acad. Sci. U. S. A. 82, 488–492. doi:10.1073/pnas.82.2.488
Loomis, W. F. (2015). Genetic control of morphogenesis in Dictyostelium. Dev. Biol. 402, 146–161. doi:10.1016/j.ydbio.2015.03.016
Morris, H. R., Taylor, G. W., Masento, M. S., Jermyn, K. A., and Kay, R. R. (1987). Chemical structure of the morphogen differentiation inducing factor from Dictyostelium discoideum. Nature 328, 811–814. doi:10.1038/328811a0
Paul, K., Nieto, V., Carlquist, W. C., Blair, D. F., and Harshey, R. M. (2010). The c-di-GMP binding protein YcgR controls flagellar motor direction and speed to affect chemotaxis by a "backstop brake" mechanism. Mol. Cell. 38, 128–139. doi:10.1016/j.molcel.2010.03.001
Pultz, I. S., Christen, M., Kulasekara, H. D., Kennard, A., Kulasekara, B., and Miller, S. I. (2012). The response threshold of Salmonella PilZ domain proteins is determined by their binding affinities for c-di-GMP. Mol. Microbiol. 86, 1424–1440. doi:10.1111/mmi.12066
Romling, U., Galperin, M. Y., and Gomelsky, M. (2013). Cyclic di-GMP: The first 25 years of a universal bacterial second messenger. Microbiol. Mol. Biol. Rev. 77, 1–52. doi:10.1128/MMBR.00043-12
Ryjenkov, D. A., Simm, R., Romling, U., and Gomelsky, M. (2006). The PilZ domain is a receptor for the second messenger c-di-GMP: The PilZ domain protein YcgR controls motility in enterobacteria. J. Biol. Chem. 281, 30310–30314. doi:10.1074/jbc.C600179200
Saito, T., Kato, A., and Kay, R. R. (2008). DIF-1 induces the basal disc of the Dictyostelium fruiting body. Dev. Biol. 317, 444–453. doi:10.1016/j.ydbio.2008.02.036
Schindelin, J., Arganda-Carreras, I., Frise, E., Kaynig, V., Longair, M., Pietzsch, T., et al. (2012). Fiji: An open-source platform for biological-image analysis. Nat. Methods 9, 676–682. doi:10.1038/nmeth.2019
Schindler, J., and Sussman, M. (1977). Ammonia determines the choice of morphogenetic pathways in Dictyostelium discoideum. J. Mol. Biol. 116, 161–169. doi:10.1016/0022-2836(77)90124-3
Singleton, C. K., Zinda, M. J., Mykytka, B., and Yang, P. (1998). The histidine kinase dhkC regulates the choice between migrating slugs and terminal differentiation in Dictyostelium discoideum. Dev. Biol. 203, 345–357. doi:10.1006/dbio.1998.9049
Song, Y., Luciani, M. F., Giusti, C., and Golstein, P. (2015). c-di-GMP induction of Dictyostelium cell death requires the polyketide DIF-1. Mol. Biol. Cell. 26, 651–658. doi:10.1091/mbc.E14-08-1337
Thompson, C. R., and Kay, R. R. (2000). The role of DIF-1 signaling in Dictyostelium development. Mol. Cell. 6, 1509–1514. doi:10.1016/s1097-2765(00)00147-7
Veltman, D. M., Akar, G., Bosgraaf, L., and Van Haastert, P. J. (2009). A new set of small, extrachromosomal expression vectors for Dictyostelium discoideum. Plasmid 61, 110–118. doi:10.1016/j.plasmid.2008.11.003
Verkerke-Van Wijk, I., Fukuzawa, M., Devreotes, P. N., and Schaap, P. (2001). Adenylyl cyclase A expression is tip-specific in Dictyostelium slugs and directs StatA nuclear translocation and CudA gene expression. Dev. Biol. 234, 151–160. doi:10.1006/dbio.2001.0232
Weijer, C. J. (2004). Dictyostelium morphogenesis. Curr. Opin. Genet. Dev. 14, 392–398. doi:10.1016/j.gde.2004.06.006
Williams, J. G. (2006). Transcriptional regulation of Dictyostelium pattern formation. EMBO Rep. 7, 694–698. doi:10.1038/sj.embor.7400714
Keywords: c-di-GMP, signal transduction, fluorescence microscopy, cell differentiation, Dictyostelium
Citation: Ide H, Hayashida Y and Morimoto YV (2023) Visualization of c-di-GMP in multicellular Dictyostelium stages. Front. Cell Dev. Biol. 11:1237778. doi: 10.3389/fcell.2023.1237778
Received: 10 June 2023; Accepted: 11 July 2023;
Published: 20 July 2023.
Edited by:
Enrico Bracco, University of Turin, ItalyReviewed by:
Arjan Kortholt, University of Groningen, NetherlandsPauline Schaap, University of Dundee, United Kingdom
Copyright © 2023 Ide, Hayashida and Morimoto. This is an open-access article distributed under the terms of the Creative Commons Attribution License (CC BY). The use, distribution or reproduction in other forums is permitted, provided the original author(s) and the copyright owner(s) are credited and that the original publication in this journal is cited, in accordance with accepted academic practice. No use, distribution or reproduction is permitted which does not comply with these terms.
*Correspondence: Yusuke V. Morimoto, eXZtMDAxQHBoeXMua3l1dGVjaC5hYy5qcA==