- 1Laboratório de Neurogenética, Universidade Federal do ABC, São Bernardo do Campo, Brazil
- 2Laboratório de Sinalização Celular e Nanobiotecnologia, Departamento de Bioquímica e Imunologia, Universidade Federal de Minas Gerais, Belo Horizonte, Brazil
- 3Department of Pharmaceutical Sciences, College of Pharmacy, University of Tennessee Health Science Center, Memphis, TN, United States
It is well established that temporal lobe epilepsy (TLE) is often related to oxidative stress and neuroinflammation. Both processes subserve alterations observed in epileptogenesis and ultimately involve distinct classes of cells, including astrocytes, microglia, and specific neural subtypes. For this reason, molecules associated with oxidative stress response and neuroinflammation have been proposed as potential targets for therapeutic strategies. However, these molecules can participate in distinct intracellular pathways depending on the cell type. To illustrate this, we reviewed the potential role of nicotinamide adenine dinucleotide phosphate (NADPH) oxidase 2 (NOX2) and myeloid differentiation primary response 88 (MyD88) in astrocytes, microglia, and neurons in epileptogenesis. Furthermore, we presented approaches to study genes in different cells, employing single-cell RNA-sequencing (scRNAseq) transcriptomic analyses, transgenic technologies and viral serotypes carrying vectors with specific promoters. We discussed the importance of identifying particular roles of molecules depending on the cell type, endowing more effective therapeutic strategies to treat TLE.
Introduction
Temporal lobe epilepsy (TLE) is the most prevalent form of the disease, corresponding to ∼40% of the cases, of which one-third are refractory to the current pharmacological treatments (Guedes et al., 2006; Organization, W. H, 2019; Falco-Walter, 2020). It is already established that epileptogenesis is an ongoing process even after the first unprovoked seizure, since rearrangements of the neural networks constantly occur (Kinjo et al., 2016; Kinjo et al., 2018; Royero et al., 2020). This is due to stress factors such as free radicals’ production and neuroinflammation, and it contributes to the progression of epilepsy (Pitkänen et al., 2015; Jiang et al., 2019; Borowicz-Reutt and Czuczwar, 2020).
Free radicals, e.g., reactive oxygen species (ROS), play a physiological role in processes such as synaptic plasticity (Beckhauser et al., 2016). They also participate in the pathological aspects of TLE and several brain disorders, such as Alzheimer’s and Parkinson’s diseases, impairing neurotransmission and favoring lipid peroxidation of the neural membrane (Puttachary et al., 2015; Borowicz-Reutt and Czuczwar, 2020). Besides the contribution of ROS in epileptogenesis, damage-associated molecular patterns (DAMPs) interact with Toll-like receptors (TLRs) dependent on myeloid differentiation primary response 88 (MyD88) for glial cell activation, promoting the release of interleukin 1β (IL-1β) and other proinflammatory molecules (Paschon et al., 2016). The following glial activation and the ongoing communication between astrocytes and microglia can be of most importance in deciding the neuroinflammation fate of the tissue in a pathology (Paschon et al., 2016; Kinoshita and Koyama, 2021).
Indeed, genes related to oxidative stress and neuroinflammation play different roles depending on the cell type in epileptogenesis. In this context, we approached the role of nicotinamide adenine dinucleotide phosphate (NADPH) oxidase 2 (NOX2), one of the main sources of ROS triggered by prolonged seizures (Shekh-Ahmad et al., 2019), and MyD88 in epileptogenesis. Furthermore, we discussed the employment of single-cell RNA-sequencing (scRNAseq) transcriptomic analyses, transgenic technologies, and viral serotypes carrying vectors with specific promoters to unravel the role of genes related to oxidative stress and neuroinflammation in epilepsy.
Distinct Roles of NOX2 in Microglia and Neurons
Microglial cells are known as the primary immune cells of the brain. As resident myeloid cells in the brain, they scour the environment removing cellular debris and releasing cytokines and free radicals such as ROS (Rettenbeck et al., 2015; Kinoshita and Koyama, 2021). Therefore, microglia plays a dual role in epileptogenesis, from distinct microglial phenotypes in sclerotic regions of the epileptic brain (Luo et al., 2016; Morin-Brureau et al., 2018) to promoting pro- and/or anti-epileptic activity (Kinoshita and Koyama, 2021).
Several studies demonstrated that status epilepticus (SE) elevates the level of ROS produced by microglia hours after its induction in animals (Dal-Pizzol et al., 2000; Sleven et al., 2006; Ryan et al., 2014). It has also been identified in the hippocampus of patients with refractory epilepsy (Shekh-Ahmad et al., 2019). The time course of ROS level alteration and the early microglial response may be associated with neural injury in the hippocampus following an insult that promotes epileptogenesis (Rettenbeck et al., 2015). Although other isoforms of the NOX family exist, NOX2 is of particular interest given its prominent expression by microglia. NOX2 is one of the main sources of ROS produced and released in epileptogenesis (Mcelroy et al., 2017; Shekh-Ahmad et al., 2019). In microglia, the GTPase Rab27 induces the movement of NOX2 complex to the plasma membrane (Ejlerskov et al., 2012). Nevertheless, in other cell types, Ras GTPase-activating-like protein (IQGAP1) is crucial for NOX2’s movement towards the cell surface (Ikeda et al., 2005). The inhibition of NOX2 or its genetic ablation in mice reduces microglial activity and neuroinflammation (Guemez-Gamboa et al., 2011; Wang et al., 2013; Zhang et al., 2017; Chandran et al., 2018). This data suggests that ROS modulates the inflammatory response and microglial activation in epileptogenesis (Chhor et al., 2013; Shekh-Ahmad et al., 2019).
NOX2 catalyzes molecular oxygen into its oxidative form in microglia and neurons. Its activation is modulated by N-methyl-D-aspartate receptors (NMDAr) and metabotropic glutamatergic receptors of group II (mGluR3) and group III (mGluR4, 6, 7, 8), specifically (Haslund-Vinding et al., 2017). Besides that, DAMPs receptors coupled to G protein, i.e., N- formyl peptide receptors (FPRs) and P2Y receptors (P2YRs), which, upon stimulation, promote phosphorylation via RAC of cytosolic p47phox, leading to its conformational change. Alongside p67phox and p40phox, this protein migrates to the membrane. In their interaction with NOX2 and p22phox, electrons are transferred from the substrate NADPH to molecular oxygen, resulting in superoxide production (Vermot et al., 2021). Additionally, hydrogen peroxide (H2O2) is suitable for triggering the phospholipase A2 (PLA2) pathway through signal-regulated kinase (ERK) and IL-1β production, which targets the IL-1β receptor and MyD88 pathway (Figures 1Ai,ii,iii) (Beckhauser et al., 2016).
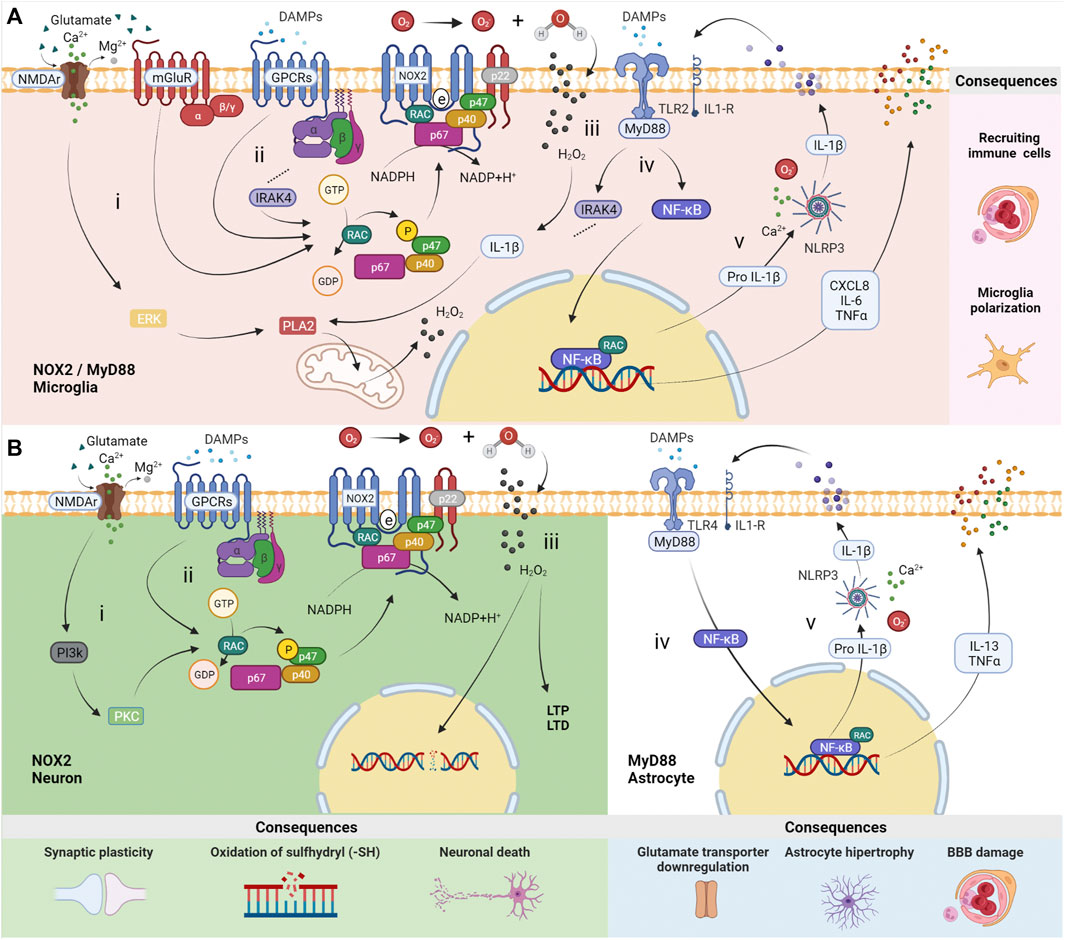
FIGURE 1. Distinct roles of MyD88 and NOX2 in neurons, astrocytes and microglia. (A) In microglia, (i) N-methyl-D-aspartate receptors (NMDAr) promote a calcium inflow, which leads to the production of mitochondrial reactive oxygen species (ROS) via ERK-PLA2. The activation of metabotropic glutamatergic receptors from group II (mGluR3) and group III (mGluR4, 6, 7, 8) induces NOX2 activation throughout Gi/Go alpha subunit. (ii) NOX2 can be activated by receptors of damage-associated molecular patterns (DAMPs) G-protein-dependent (GPCRs), i.e., N-formyl peptide receptors (FPRs) and P2Y receptors (P2YRs) via RAC, followed by phosphorylation of p47phox (p47) and migration to the membrane along with p67phox (p67) and p40phox (p40), resulting in ROS production. (iii) H2O2 produced from hydrogen and O2 can cross membranes to participate in biochemistry reactions. (iv) Stimulation by DAMPs or cytokines throughout Toll-like Receptor 2 (TLR2) and interleukin-1 receptor (IL-1R), activates an intracellular signaling pathway involving the protein adaptor myeloid differentiation primary response 88 (MyD88). Specific consequences occur in microglia, such as NOX2 activation via IRAK4-RAC pathways. (v) NF-κB/RAC transcription factors promote the expression of chemokines CXCL8, IL-6, TNFα, and Pro IL-1β. The latter is transformed into mature IL-1β by the NLRP3 inflammasome dependent on calcium and ROS. The consequences are immune cell recruiting and microglia polarization. (B) Activation of NOX2 in neurons occurs (i) by NMDAr (containing subunit GluN2B) via PI3K-PKC and/or (ii) by DAMPs receptors (FPRs and P2YRs). (iii) H2O2 produced from hydrogen and O2 induces long-term potentiation (LTP) and long-term depression (LTD.) in physiological situations. However, a high concentration of ROS, e.g., in status epilepticus (SE), results in neural DNA damage by oxidation of sulfhydryl compounds (–SH) and cell death due to influx of H2O2 to the nucleus. In astrocytes, (iv) MyD88 activation by TLR4 is followed by NF-κB/RAC transcription factors assembly, (v) promoting the expression of IL-6, TNFα, and Pro IL-1β. The latter is transformed into mature IL-1β by the NLRP3 inflammasome dependent on calcium and ROS. The consequences include ions and neurotransmitters unbalance, astrocytic hypertrophy, and blood-brain barrier (BBB) changes. The illustrations were obtained using BioRender software.
The localization of NOX2 in the postsynaptic region is convenient for its role in synaptic plasticity (Bedard and Krause, 2007; Brennan et al., 2009). ROS physiologically acts as a second messenger in the nervous system, mostly supporting synaptic long-term potentiation (LTP) (Hidalgo and Arias-Cavieres, 2016) or long-term depression (LTD.) (Francis-Oliveira et al., 2018). In the hippocampus, for instance, ROS activates protein kinases that are essential for neural plasticity. Upon stimulation, NMDAr (containing the subunit GluN2B) promotes a calcium inflow into synapses, which leads to the production of ROS via Phosphoinositide 3-Kinase (PI3K)—Protein Kinase C (PKC), H2O2, and neural nitric oxide synthase (nNOS) (Beckhauser et al., 2016). Once produced, H2O2 can pass through the plasma membrane to interact with pre- and postsynaptic proteins, modulating the synaptic transmission (Quinn and Gauss, 2004). However, it can also induce damage on neuronal DNA, including chromosomal alterations, breakage of the DNA column and, in the absence of catalyst, oxidation of sulfhydryl compounds (–SH), leading to cell death in pathological conditions (Figures 1Bi,ii,iii) (Beckhauser et al., 2016).
Distinct Roles of MyD88 in Microglia and Astrocytes
TLRs 2, 4, and 9 are expressed in astrocytes, while TLRs 1-9 are expressed in microglia. However, in neuroinflammation, the innate immune response mediated by microglia and astrocytes depends largely on TLR2 and TLR4, respectively, and IL-1R in both cell types. Most TLRs are coupled to the MyD88 adapter, the exception being TLR3, which is activated by double-strand DNA triggering the TRIF pathway. It is also important to mention that TLR4 can be activated by lipopolysaccharide to signal both pathways MyD88 and TRIF (Paschon et al., 2016; Fiebich et al., 2018).
These receptors can be activated by DAMPs, such as High Mobility Group Box 1 (HMGB-1), as observed in epilepsy, and beta-amyloid plaques and alpha-synuclein, in Alzheimer’s and Parkinson’s disease, respectively (Bernaus et al., 2020). These DAMPs can interact with TLR receptors located in astrocytes and microglia membranes. TLR activation leads both cells to secrete cytokines and inflammatory molecules that activate NADPH, which aids NOX2 and cyclooxygenase 2 (COX2) activation in microglia (Meng and Yao, 2020). The secretion of cytokines promotes autocrine and paracrine signaling, leading astrocytes and microglia activation into a vicious circle (Vezzani et al., 2013; Bernaus et al., 2020).
In the literature, it is regularly reported the expression of correspondent TLRs by astrocytes and microglia, however, the release of specific cytokines by these cells depends on the stimulus or pathology. For instance, after seizure activity, the microglia of patients with TLE release IL-1β, CXCL8, IL-6 and TNFα (Morin-Brureau et al., 2018). On the other hand, in an LPS-induced Parkinson’s model, the up-regulation of IFN-γ, IL-1β, IL-1R, IL-16, IL-17 levels in microglia have been reported. Therefore, it can be inferred that cytokines’ release by microglia depends on many factors, although using the same intracellular signaling pathway, the NF-κB and NLRP3 inflammasome (Chien et al., 2016). When immunologically stimulated by TNFα or IL-1β, post-mortem or biopsy human astrocytes and cell-line NT2 astrocytes release cytokines IL-1β, TNFα, and IL-13. However, post-mortem or biopsy human astrocytes also produced IL-2, IL-7, TNFβ, and IF N-γ (Figures 1iv,v) (Burkert et al., 2012).
Microglia activation by TLRs and MyD88 modulate distinct epileptogenesis features. In this cell type, MyD88 signaling promotes cytokine secretion and induces apoptosis of neighboring cells. Microglia potentializes the immune response by recruiting peripheral immune system cells, increasing the inflammatory environment (Meng and Yao, 2020). TLR2 signalling activates IL-1 Receptor-Associated Kinase 4 (IRAK4) in the MyD88-dependent axis. Once activated, IRAK4 phosphorylates p47phox on several residues to activate NOX2 (Pacquelet et al., 2007). Besides that, microglia disrupts gamma-aminobutyric acid (GABA) signaling and regulates the neural expression of NR1 and NR2b subunits of NMDA receptor. These processes result in high excitability of neurons which, in turn, contributes to epileptogenesis (Vezzani et al., 2013; Liu et al., 2018; Xueying Li et al., 2021).
On the other hand, MyD88 signaling modulates the astroglial tissue-maintenance functions, such as ions and neurotransmitters homeostasis (Li et al., 2020; Vezzani et al., 2013). In neuroinflammation, astroglial activation through the MyD88 pathway creates a harmful environment to the neural tissue, characterized by an astrocytic hypertrophic state, which impairs the blood-brain barrier and neural communication maintenance. (Vezzani et al., 2013; Liu et al., 2018; Xueying Li et al., 2021). Astrocytic activation can occur by MyD88-dependent TLR4 via the extracellular signal-related kinase (ERK) pathway, and promotes excitatory synapse development, resulting in increased seizure susceptibility (Henneberger and Steinhauser, 2016; Shen et al., 2016). In this context, the increased expression of TLR4 in astrocytes, but not in microglia, suggests TLR4’s role in seizure frequency in human epilepsy (Pernhorst et al., 2013).
The crosstalk involving the MyD88 pathway in microglia and astrocytes seems crucial in triggering inflammation in the central nervous system (CNS), given that the absence of microglia in vitro and in vivo leads to a failure of TLR4 activation in astrocytes, consequently reducing the inflammatory response (Barbierato et al., 2013; Liddelow et al., 2017). In epileptogenesis, neuroinflammation contributes to the pathological synaptic plasticity and dendrite growth by activating IL-1R/TLR receptors, transcription factor NF-κB, and cytokines effects (Vezzani et al., 2013; Meng and Yao, 2020).
Therefore, anti-inflammatory therapies and pharmacological drugs that influence the signaling pathway involving TLR4/MyD88/NF-κB/NLRP3/IL-1β, such as baicalin (TLR4 inhibitor) and agmatine (TLR4 down regulator), also reduce the frequency, duration and intensity of seizures (Lun Li et al., 2021; Yang et al., 2021). Furthermore, MyD88 inhibition reduces phosphorylation and expression of NMDAr, modulates proinflammatory microglia, and increases the expression of glutamate receptors in astrocytes (Wang et al., 2017; Liu et al., 2018). These mechanisms support the important role of MyD88 in neuroinflammation associated with epilepsy; in this sense, approaches that directly target MyD88 inhibition demonstrate positive effects in epilepsy. In summary, MyD88 and NOX2 are essential players in epileptogenesis, regulating the neuroinflammatory response in a cell-specific manner, promoting distinct outcomes.
Discussion
Considering that specific cell types have distinct impacts on epilepsy, technologies that allow screening, such as scRNAseq, genetically modified animals, and cell-targeted gene control provide valuable approaches to study epilepsy. New therapeutic strategies are necessary since around one-third of patients with TLE are refractory to clinically available drug treatments.
scRNAseq allows the assessment of the transcriptomic profile of cell-type-specific (Figure 2A). This technique offers insights into the mechanisms involved in epileptogenesis and characterizes the specific contribution of microglia, astrocytes, and neurons in epilepsy (Olsen and Baryawno, 2018; Cid et al., 2021). This approach investigates NOX2 and MyD88 transcriptomic pathways in distinct time points in epileptogenesis in animal models and surgically-extracted nervous tissues. Specific cell types are separately analyzed, and a complete RNA profile is obtained. For instance, RNAseq data of epileptic hippocampus demonstrated the possibility of investigating oxidative stress regulation and inflammatory responses, such as cytokines, cell migration, proliferation, and cell death, which involve TLR2, TLR3, TLR4, TLR7, and C1qa, inflammasome genes, and pyroptosis (Cid et al., 2021).
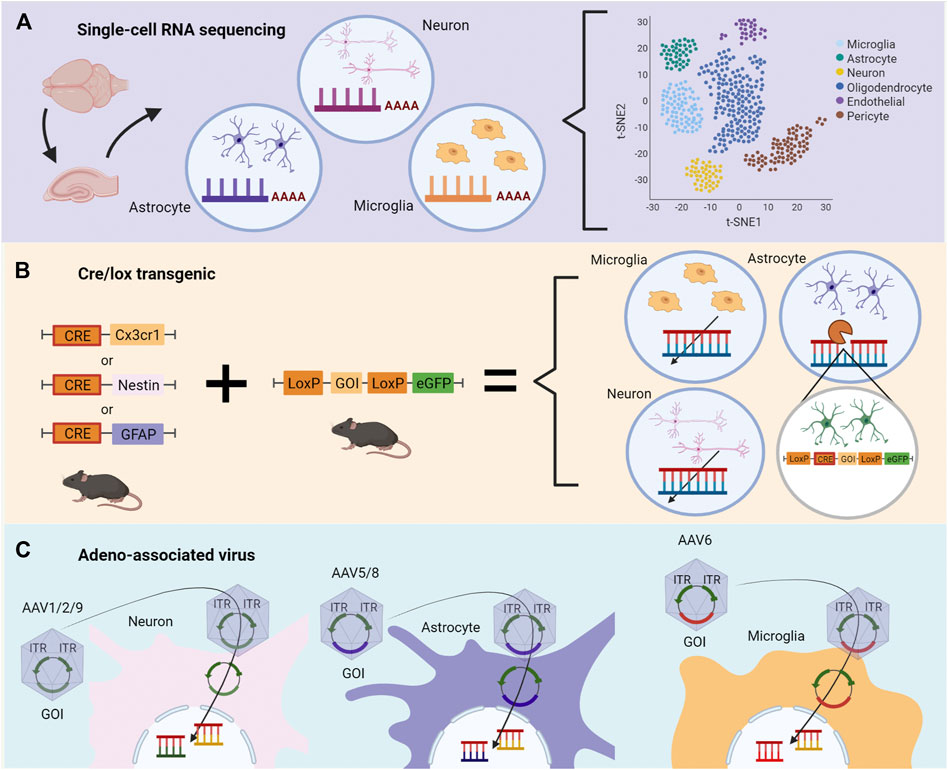
FIGURE 2. Methodological strategies to assess specific roles of genes during epileptogenesis depending on the cell type. (A) Single-cell RNA sequencing (scRNA-seq) of the temporal lobe or hippocampus permits the evaluation of changes in transcriptomics triggered by epileptogenesis. Distinct cell types are separately analyzed, and a complete RNA profile is obtained. Changes in transcriptomics are described according to correlation, heatmaps, and quantitative analysis. (B) Cre/lox transgenic technologies can be used to evaluate the role of genes in cell-type-specific during epileptogenesis in vivo. In the first generation, two distinct animals are bred, one containing the Cre enzyme, the other containing two LoxP sequences between the gene of interest (GOI) and a gene reporter, as eGFP. Littermates receive genetic material necessary for the Cre/flox system to modify, delete or change the GOI. (C) Adeno-associated virus (AAV) contains the GOI with inverted terminal repeats (ITR) and viral capsid necessary for cell-specific targeting. The genetic modification occurs by the transported plasmid carrying the GOI. The illustrations were obtained using BioRender software.
Besides mapping changes in the transcriptome in cell-type-specific, transgenic animals allow the control of gene expression in a cell-specific manner, such as in the Cre/flox system (Figure 2B). Cre-mice breed with LoxP (floxed) mice results in Cre-LoxP animals. In this condition, Cre enzyme promotes the inversion or deletion of the genes flanked by LoxP sequences, which can be replaced by a reporter gene such as eGFP (Kim et al., 2018). Bringing this approach into the context of specific targets in epilepsy, it is possible to track the downstream effects of MyD88 and NOX2 loss-of-function in cell-type-specific. Indeed, the cell-type-specific gene ablation combined with pharmacological approaches has been demonstrated particularly useful in the determination of the roles of COX2 (Serrano et al., 2011) and prostaglandin receptor EP2 (Nagib et al., 2020; Sluter et al., 2021; Varvel et al., 2021) in animal models of SE.
For example, to test the downstream effects of MyD88 loss in microglia, a transgenic mice model was established to target microglia chemokine receptors (Cx3cr1) to knockout the MyD88 gene. The authors showed a relationship between neuron maturation and loss of MyD88 in microglia cells, which disrupts the reward-related-memory formation in morphine addiction (Rivera et al., 2019). On the other hand, the Nestin-Cre model directly induces gene modification into neurons and microglia, which showed that the presence of MyD88 in both cell types is not necessary to respond to a nocive stimulus (Braun et al., 2012). Specific neuron modifications are usually achieved with the Synapsin I Cre model (Mcnair et al., 2020), which is capable of modifying differentiated neurons. Regarding astrocytes, although the most used model is the GFAP-Cre, it also promotes changes in non-specific targets; S100b and GLAST are alternatives pointed out to decrease the off-target effects (Guttenplan and Liddelow, 2019; Mcnair et al., 2020). Also, the generation of brain-specific Mn-SOD-deficient mice (brain-Sod22/) to study ROS in the hypoxia model (Sasaki et al., 2011) and neurodegenerative diseases (Marecki et al., 2014; Park et al., 2022) demonstrates the application of this technology.
In addition to transgenic animal models, genetic modifications through viral vectors have also opened opportunities for studying TLE (Figure 2C). Amongst these, adeno-associated virus (AAV) stands out given that its variations have distinct tropism for cell-type-specific. Also, AAV vectors have been used for gene therapy applications due to their safety and the ability to infect dividing and non-dividing cells, performing a long-term transgene expression profile (Daya and Berns, 2008). For instance, AAV can be applied to increase CCL2, aiming to rescue the expression of cytokine levels (CCL2, IL-1β), since these cytokines were described as reduced by other therapies (Wu et al., 2019).
AAV allows control of the gene expression by inserting the gene of interest (GOI), or a sequence that generates a short hairpin RNA (shRNA) to target the GOI, in the host DNA. This modification promotes overexpression or knockdown of the GOI in the host cell (Daya and Berns, 2008). Several serotypes have been identified in AAV, increasing the probability of transfection of cell-type-specific. For CNS, serotype 2 (AAV2) is the most adopted virus despite the variability of transfection efficiency regarding the cell type, which is overcome by using pseudotyping AAVs by recombining new capsids from different serotypes (Fakhiri and Grimm, 2021). Neurons and astrocytes are efficiently transfected by AAV, both in vivo and in vitro. Also, vectors have been tested for transfection efficiency and transgene expression, demonstrating that AAV5 (Griffin et al., 2019) and AAV8 (Aschauer et al., 2013) are potent viral vectors to transfect astrocytes, while AAV2/1 (Hammond et al., 2017) and AAV9 (Aschauer et al., 2013) are best suitable for neurons. Microglia are the most refractile cells in the nervous system to AAV transfection, but several improvements have been made. For instance, a modified rAAV6 combined with specific microglial promoters succeeded in transfecting these cells in vitro and in vivo (Rosario et al., 2016; Su et al., 2016). Therefore, by employing specific serotypes, it is possible to overexpress or knockdown the expression of genes implied in the epileptogenesis process, such as NOX2 and MyD88, in cell-type-specific.
Cell-type-specific approaches grant benefits in research and scientific study development. However, the lack of reproducibility and technical difficulties might be the reasons for possibly delivering biased results, such as in the RNA-seq case (Whitley et al., 2016). In the Cre/lox system, specific tissue promoters can be expressed in undesired tissues and at uncommon times (Sauer, 1998). At last, their use for in-human treatment may not be indicated, such as in the case of AAV therapies, which hold genotoxicity potential (Colella et al., 2018). Epilepsy has already demonstrated to be multifactorial, therefore a single treatment based on monotherapy will probably not be efficient, especially in refractory TLE (Vezzani et al., 2013; Organization, W. H, 2019; Falco-Walter, 2020).
In summary, cell-type-specific approaches should be applied to investigate mechanisms related to brain disorders. This strategy promotes a better understanding of genes involved in epileptogenesis, bringing new insights into treatments and therapies for TLE (Couvillion et al., 2009).
Author Contributions
Wrote the first draft of the manuscript: CA, RP, MM, GH. Revised the manuscript: RR, JJ, EK, AK. Conceived the study: AK.
Funding
AK is grateful for grants from FAPESP (#2019/17892-8, #2020/11667-0), CNPq (#431000/2016-6 and #312047/2017-7), and CAPES (financial code 001). JJ is grateful for NIH/NINDS grants: R01NS100947, and R61NS124923. The funders had no role in study design, data collection and analysis, decision to publish, or manuscript preparation.
Conflict of Interest
The authors declare that the research was conducted in the absence of any commercial or financial relationships that could be construed as a potential conflict of interest.
Publisher’s Note
All claims expressed in this article are solely those of the authors and do not necessarily represent those of their affiliated organizations, or those of the publisher, the editors and the reviewers. Any product that may be evaluated in this article, or claim that may be made by its manufacturer, is not guaranteed or endorsed by the publisher.
References
Aschauer, D. F., Kreuz, S., and Rumpel, S. (2013). Analysis of Transduction Efficiency, Tropism and Axonal Transport of AAV Serotypes 1, 2, 5, 6, 8 and 9 in the Mouse Brain. PLoS One 8, e76310. doi:10.1371/journal.pone.0076310
Barbierato, M., Facci, L., Argentini, C., Marinelli, C., Skaper, S., and Giusti, P. (2013). Astrocyte-microglia Cooperation in the Expression of a Pro-inflammatory Phenotype. Cnsnddt 12, 608–618. doi:10.2174/18715273113129990064
Beckhauser, T. F., Francis-Oliveira, J., and De Pasquale, R. (2016). Reactive Oxygen Species: Physiological and Physiopathological Effects on Synaptic Plasticity. J. Exp. Neurosci. 10, 23–48. doi:10.4137/JEN.S39887
Bedard, K., and Krause, K.-H. (2007). The NOX Family of ROS-Generating NADPH Oxidases: Physiology and Pathophysiology. Physiol. Rev. 87, 245–313. doi:10.1152/physrev.00044.2005
Bernaus, A., Blanco, S., and Sevilla, A. (2020). Glia Crosstalk in Neuroinflammatory Diseases. Front. Cell. Neurosci. 14, 209. doi:10.3389/fncel.2020.00209
Borowicz-Reutt, K. K., and Czuczwar, S. J. (2020). Role of Oxidative Stress in Epileptogenesis and Potential Implications for Therapy. Pharmacol. Rep. 72, 1218–1226. doi:10.1007/s43440-020-00143-w
Braun, T. P., Grossberg, A. J., Veleva-Rotse, B. O., Maxson, J. E., Szumowski, M., Barnes, A. P., et al. (2012). Expression of Myeloid Differentiation Factor 88 in Neurons Is Not Requisite for the Induction of Sickness Behavior by Interleukin-1β. J. Neuroinflammation 9, 229. doi:10.1186/1742-2094-9-229
Brennan, A. M., Won Suh, S., Joon Won, S., Narasimhan, P., Kauppinen, T. M., Lee, H., et al. (2009). NADPH Oxidase Is the Primary Source of Superoxide Induced by NMDA Receptor Activation. Nat. Neurosci. 12, 857–863. doi:10.1038/nn.2334
Burkert, K., Moodley, K., Angel, C. E., Brooks, A., and Graham, E. S. (2012). Detailed Analysis of Inflammatory and Neuromodulatory Cytokine Secretion from Human NT2 Astrocytes Using Multiplex Bead Array. Neurochem. Int. 60, 573–580. doi:10.1016/j.neuint.2011.09.002
Chandran, R., Kim, T., Mehta, S. L., Udho, E., Chanana, V., Cengiz, P., et al. (2018). A Combination Antioxidant Therapy to Inhibit NOX2 and Activate Nrf2 Decreases Secondary Brain Damage and Improves Functional Recovery after Traumatic Brain Injury. J. Cereb. Blood Flow. Metab. 38, 1818–1827. doi:10.1177/0271678x17738701
Chhor, V., Le Charpentier, T., Lebon, S., Oré, M.-V., Celador, I. L., Josserand, J., et al. (2013). Characterization of Phenotype Markers and Neuronotoxic Potential of Polarised Primary Microglia In Vitro. Brain, Behav. Immun. 32, 70–85. doi:10.1016/j.bbi.2013.02.005
Chien, C.-H., Lee, M.-J., Liou, H.-C., Liou, H.-H., and Fu, W.-M. (2016). Microglia-Derived Cytokines/Chemokines Are Involved in the Enhancement of LPS-Induced Loss of Nigrostriatal Dopaminergic Neurons in DJ-1 Knockout Mice. PLoS One 11, e0151569. doi:10.1371/journal.pone.0151569
Cid, E., Marquez-Galera, A., Valero, M., Gal, B., Medeiros, D. C., Navarron, C. M., et al. (2021). Sublayer- and Cell-type-specific Neurodegenerative Transcriptional Trajectories in Hippocampal Sclerosis. Cell Rep. 35, 109229. doi:10.1016/j.celrep.2021.109229
Colella, P., Ronzitti, G., and Mingozzi, F. (2018). Emerging Issues in AAV-Mediated In Vivo Gene Therapy. Mol. Ther. - Methods & Clin. Dev. 8, 87–104. doi:10.1016/j.omtm.2017.11.007
Couvillion, M. T., Lee, S. R., Hogstad, B., Malone, C. D., Tonkin, L. A., Sachidanandam, R., et al. (2009). Sequence, Biogenesis, and Function of Diverse Small RNA Classes Bound to the Piwi Family Proteins of Tetrahymena Thermophila. Genes. Dev. 23, 2016–2032. doi:10.1101/gad.1821209
Dal-Pizzol, F., Klamt, F., Vianna, M. M. R., Schröder, N., Quevedo, J., Benfato, M. S., et al. (2000). Lipid Peroxidation in hippocampus Early and Late after Status Epilepticus Induced by Pilocarpine or Kainic Acid in Wistar Rats. Neurosci. Lett. 291, 179–182. doi:10.1016/s0304-3940(00)01409-9
Daya, S., and Berns, K. I. (2008). Gene Therapy Using Adeno-Associated Virus Vectors. Clin. Microbiol. Rev. 21, 583–593. doi:10.1128/cmr.00008-08
Ejlerskov, P., Christensen, D. P., Beyaie, D., Burritt, J. B., Paclet, M.-H., Gorlach, A., et al. (2012). NADPH Oxidase Is Internalized by Clathrin-Coated Pits and Localizes to a Rab27A/B GTPase-Regulated Secretory Compartment in Activated Macrophages. J. Biol. Chem. 287, 4835–4852. doi:10.1074/jbc.m111.293696
Fakhiri, J., and Grimm, D. (2021). Best of Most Possible Worlds: Hybrid Gene Therapy Vectors Based on Parvoviruses and Heterologous Viruses. Mol. Ther. 29, 3359–3382. doi:10.1016/j.ymthe.2021.04.005
Falco-Walter, J. (2020). Epilepsy-Definition, Classification, Pathophysiology, and Epidemiology. Semin. Neurol. 40, 617–623. doi:10.1055/s-0040-1718719
Fiebich, B. L., Batista, C. R. A., Saliba, S. W., Yousif, N. M., and De Oliveira, A. C. P. (2018). Role of Microglia TLRs in Neurodegeneration. Front. Cell. Neurosci. 12, 329. doi:10.3389/fncel.2018.00329
Francis-Oliveira, J., Vilar Higa, G. S., Mendonça Munhoz Dati, L., Carvalho Shieh, I., and De Pasquale, R. (2018). Metaplasticity in the Visual Cortex: Crosstalk between Visual Experience and Reactive Oxygen Species. J. Neurosci. 38, 5649–5665. doi:10.1523/jneurosci.2617-17.2018
Griffin, J. M., Fackelmeier, B., Fong, D. M., Mouravlev, A., Young, D., and O’carroll, S. J. (2019). Astrocyte-selective AAV Gene Therapy through the Endogenous GFAP Promoter Results in Robust Transduction in the Rat Spinal Cord Following Injury. Gene Ther. 26, 198–210. doi:10.1038/s41434-019-0075-6
Guedes, F. A., Galvis-Alonso, O. Y., and Leite, J. P. (2006). Plasticidade neuronal associada à epilepsia Do lobo temporal mesial: insights a partir de estudos em humanos e em modelos animais. J. epilepsy Clin. Neurophysiol. 12, 10–17. doi:10.1590/s1676-26492006000200003
Guemez-Gamboa, A., Estrada-Sánchez, A. M., Montiel, T., Páramo, B., Massieu, L., and Morán, J. (2011). Activation of NOX2 by the Stimulation of Ionotropic and Metabotropic Glutamate Receptors Contributes to Glutamate Neurotoxicity In Vivo through the Production of Reactive Oxygen Species and Calpain Activation. J. Neuropathol. Exp. Neurol. 70, 1020–1035. doi:10.1097/nen.0b013e3182358e4e
Guttenplan, K. A., and Liddelow, S. A. (2019). Astrocytes and Microglia: Models and Tools. J. Exp. Med. 216, 71–83. doi:10.1084/jem.20180200
Hammond, S. L., Leek, A. N., Richman, E. H., and Tjalkens, R. B. (2017). Cellular Selectivity of AAV Serotypes for Gene Delivery in Neurons and Astrocytes by Neonatal Intracerebroventricular Injection. PLoS One 12, e0188830. doi:10.1371/journal.pone.0188830
Haslund-Vinding, J., Mcbean, G., Jaquet, V., and Vilhardt, F. (2017). NADPH Oxidases in Oxidant Production by Microglia: Activating Receptors, Pharmacology and Association with Disease. Br. J. Pharmacol. 174, 1733–1749. doi:10.1111/bph.13425
Henneberger, C., and Steinhäuser, C. (2016). Astrocytic TLR4 at the Crossroads of Inflammation and Seizure Susceptibility. J. Cell Biol. 215, 607–609. doi:10.1083/jcb.201611078
Hidalgo, C., and Arias-Cavieres, A. (2016). Calcium, Reactive Oxygen Species, and Synaptic Plasticity. Physiology 31, 201–215. doi:10.1152/physiol.00038.2015
Ikeda, S., Yamaoka-Tojo, M., Hilenski, L., Patrushev, N. A., Anwar, G. M., Quinn, M. T., et al. (2005). IQGAP1 Regulates Reactive Oxygen Species-dependent Endothelial Cell Migration through Interacting with Nox2. Arterioscler Thromb Vasc Biol 25, 2295–2300. doi:10.1161/01.atv.0000187472.55437.af
Jiang, J., Yu, Y., Kinjo, E. R., Du, Y., Nguyen, H. P., and Dingledine, R. (2019). Suppressing Pro-inflammatory Prostaglandin Signaling Attenuates Excitotoxicity-Associated Neuronal Inflammation and Injury. Neuropharmacology 149, 149–160. doi:10.1016/j.neuropharm.2019.02.011
Kim, H., Kim, M., Im, S.-K., and Fang, S. (2018). Mouse Cre-LoxP System: General Principles to Determine Tissue-specific Roles of Target Genes. Lab. Anim. Res. 34, 147–159. doi:10.5625/lar.2018.34.4.147
Kinjo, E. R., Higa, G. S. V., Santos, B. A., De Sousa, E., Damico, M. V., Walter, L. T., et al. (2016). Pilocarpine-induced Seizures Trigger Differential Regulation of microRNA-Stability Related Genes in Rat Hippocampal Neurons. Sci. Rep. 6, 20969. doi:10.1038/srep20969
Kinjo, E, Rodríguez, P, Dos Santos, B, Higa, G, Ferraz, M, Schmeltzer, C., et al. (2018). New Insights on Temporal Lobe Epilepsy Based on Plasticity-Related Network Changes and High-Order Statistics. Mol. Neurobiol. 55, 3990–3998. doi:10.1007/s12035-017-0623-2
Kinoshita, S., and Koyama, R. (2021). Pro- and Anti-epileptic Roles of Microglia. Neural Regen. Res. 16, 1369–1371. doi:10.4103/1673-5374.300976
Liddelow, S. A., Guttenplan, K. A., Clarke, L. E., Bennett, F. C., Bohlen, C. J., Schirmer, L., et al. (2017). Neurotoxic Reactive Astrocytes Are Induced by Activated Microglia. Nature 541, 481–487. doi:10.1038/nature21029
Liu, J.-T., Wu, S.-X., Zhang, H., and Kuang, F. (2018). Inhibition of MyD88 Signaling Skews Microglia/Macrophage Polarization and Attenuates Neuronal Apoptosis in the Hippocampus after Status Epilepticus in Mice. Neurotherapeutics 15, 1093–1111. doi:10.1007/s13311-018-0653-0
Lun Li, L., Acioglu, C., Heary, R. F., and Elkabes, S. (2021). Role of Astroglial Toll-like Receptors (TLRs) in Central Nervous System Infections, Injury and Neurodegenerative Diseases. Brain, Behav. Immun. 91, 740–755. doi:10.1016/j.bbi.2020.10.007
Luo, C., Koyama, R., and Ikegaya, Y. (2016). Microglia Engulf Viable Newborn Cells in the Epileptic Dentate Gyrus. Glia 64, 1508–1517. doi:10.1002/glia.23018
Marecki, J. C., Parajuli, N., Crow, J. P., and Macmillan-Crow, L. A. (2014). The Use of the Cre/loxPSystem to Study Oxidative Stress in Tissue-Specific Manganese Superoxide Dismutase Knockout Models. Antioxidants Redox Signal. 20, 1655–1670. doi:10.1089/ars.2013.5293
Mcelroy, P. B., Liang, L.-P., Day, B. J., and Patel, M. (2017). Scavenging Reactive Oxygen Species Inhibits Status Epilepticus-Induced Neuroinflammation. Exp. Neurol. 298, 13–22. doi:10.1016/j.expneurol.2017.08.009
Mcnair, L. F., Andersen, J. V., Nissen, J. D., Sun, Y., Fischer, K. D., Hodgson, N. W., et al. (2020). Conditional Knockout of GLT-1 in Neurons Leads to Alterations in Aspartate Homeostasis and Synaptic Mitochondrial Metabolism in Striatum and Hippocampus. Neurochem. Res. 45, 1420–1437. doi:10.1007/s11064-020-03000-7
Meng, F., and Yao, L. (2020). The Role of Inflammation in Epileptogenesis. Acta Epileptol. 2, 1–19. doi:10.1186/s42494-020-00024-y
Morin-Brureau, M., Milior, G., Royer, J., Chali, F., Le Duigou, C., Savary, E., et al. (2018). Microglial Phenotypes in the Human Epileptic Temporal Lobe. Brain 141, 3343–3360. doi:10.1093/brain/awy276
Nagib, M. M., Yu, Y., and Jiang, J. (2020). Targeting Prostaglandin Receptor EP2 for Adjunctive Treatment of Status Epilepticus. Pharmacol. Ther. 209, 107504. doi:10.1016/j.pharmthera.2020.107504
Olsen, T, and Baryawno, N. (2018). Introduction to Single-Cell RNA Sequencing. Curr. Protoc. Mol. Biol. 122, e57. doi:10.1002/cpmb.57
Pacquelet, S., Johnson, J. L., Ellis, B. A., Brzezinska, A. A., Lane, W. S., Munafo, D. B., et al. (2007). Cross-talk between IRAK-4 and the NADPH Oxidase. Biochem. J. 403, 451–461. doi:10.1042/bj20061184
Park, J. S., Saeed, K., Jo, M. H., Kim, M. W., Lee, H. J., Park, C. B., et al. (2022). LDHB Deficiency Promotes Mitochondrial Dysfunction Mediated Oxidative Stress and Neurodegeneration in Adult Mouse Brain. Antioxidants (Basel) 11 (2), 261. doi:10.3390/antiox11020261
Paschon, V., Takada, S, Ikebara, J, Sousa, E., Raeisossadati, R., Ulrich, H., et al. (2016). Interplay between Exosomes, microRNAs and Toll-like Receptors in Brain Disorders. Mol. Neurobiol. 53, 2016–2028. doi:10.1007/s12035-015-9142-1
Pernhorst, K., Herms, S., Hoffmann, P., Cichon, S., Schulz, H., Sander, T., et al. (2013). TLR4, ATF-3 and IL8 Inflammation Mediator Expression Correlates with Seizure Frequency in Human Epileptic Brain Tissue. Seizure 22, 675–678. doi:10.1016/j.seizure.2013.04.023
Pitkänen, A., Lukasiuk, K., Edward Dudek, F., and Staley, K. (2015). Epileptogenesis. Cold Spring Harb. Perspect. Med. 5 (10), a022822. doi:10.1101/cshperspect.a022822
Puttachary, S., Sharma, S., Stark, S., and Thippeswamy, T. (2015). Seizure-induced Oxidative Stress in Temporal Lobe Epilepsy. Biomed. Res. Int. 2015, 745613. doi:10.1155/2015/745613
Quinn, M. T., and Gauss, K. A. (2004). Structure and Regulation of the Neutrophil Respiratory Burst Oxidase: Comparison with Nonphagocyte Oxidases. J. Leukoc. Biol. 76, 760–781. doi:10.1189/jlb.0404216
Rettenbeck, M. L., Rüden, E.-L. V., Bienas, S., Carlson, R., Stein, V. M., Tipold, A., et al. (2015). Microglial ROS Production in an Electrical Rat Post-status Epilepticus Model of Epileptogenesis. Neurosci. Lett. 599, 146–151. doi:10.1016/j.neulet.2015.05.041
Rivera, P. D., Hanamsagar, R., Kan, M. J., Tran, P. K., Stewart, D., Jo, Y. C., et al. (2019). Removal of Microglial-specific MyD88 Signaling Alters Dentate Gyrus Doublecortin and Enhances Opioid Addiction-like Behaviors. Brain, Behav. Immun. 76, 104–115. doi:10.1016/j.bbi.2018.11.010
Rosario, A. M., Cruz, P. E., Ceballos-Diaz, C., Strickland, M. R., Siemienski, Z., Pardo, M., et al. (2016). Microglia-specific Targeting by Novel Capsid-Modified AAV6 Vectors. Mol. Ther. - Methods & Clin. Dev. 3, 16026. doi:10.1038/mtm.2016.26
Royero, P. X., Higa, G. S. V., Kostecki, D. S., Dos Santos, B. A., Almeida, C., Andrade, K. A., et al. (2020). Ryanodine Receptors Drive Neuronal Loss and Regulate Synaptic Proteins during Epileptogenesis. Exp. Neurol. 327, 113213. doi:10.1016/j.expneurol.2020.113213
Ryan, K., Liang, L.-P., Rivard, C., and Patel, M. (2014). Temporal and Spatial Increase of Reactive Nitrogen Species in the Kainate Model of Temporal Lobe Epilepsy. Neurobiol. Dis. 64, 8–15. doi:10.1016/j.nbd.2013.12.006
Sasaki, T., Shimizu, T., Koyama, T., Sakai, M., Uchiyama, S., Kawakami, S., et al. (2011). Superoxide Dismutase Deficiency Enhances Superoxide Levels in Brain Tissues during Oxygenation and Hypoxia-Reoxygenation. J. Neurosci. Res. 89, 601–610. doi:10.1002/jnr.22581
Sauer, B. (1998). Inducible Gene Targeting in Mice Using the Cre/loxSystem. Methods 14, 381–392. doi:10.1006/meth.1998.0593
Serrano, G. E., Lelutiu, N., Rojas, A., Cochi, S., Shaw, R., Makinson, C. D., et al. (2011). Ablation of Cyclooxygenase-2 in Forebrain Neurons Is Neuroprotective and Dampens Brain Inflammation after Status Epilepticus. J. Neurosci. 31, 14850–14860. doi:10.1523/jneurosci.3922-11.2011
Shekh-Ahmad, T., Kovac, S., Abramov, A. Y., and Walker, M. C. (2019). Reactive Oxygen Species in Status Epilepticus. Epilepsy & Behav. 101, 106410. doi:10.1016/j.yebeh.2019.07.011
Shen, Y., Qin, H., Chen, J., Mou, L., He, Y., Yan, Y., et al. (2016). Postnatal Activation of TLR4 in Astrocytes Promotes Excitatory Synaptogenesis in Hippocampal Neurons. J. Cell Biol. 215, 719–734. doi:10.1083/jcb.201605046
Sleven, H., Gibbs, J. E., Heales, S., Thom, M., and Cock, H. R. (2006). Depletion of Reduced Glutathione Precedes Inactivation of Mitochondrial Enzymes Following Limbic Status Epilepticus in the Rat hippocampus. Neurochem. Int. 48, 75–82. doi:10.1016/j.neuint.2005.10.002
Sluter, M. N., Hou, R., Li, L., Yasmen, N., Yu, Y., Liu, J., et al. (2021). EP2 Antagonists (2011-2021): A Decade's Journey from Discovery to Therapeutics. J. Med. Chem. 64, 11816–11836. doi:10.1021/acs.jmedchem.1c00816
Su, W., Kang, J., Sopher, B., Gillespie, J., Aloi, M. S., Odom, G. L., et al. (2016). Recombinant Adeno-Associated Viral (rAAV) Vectors Mediate Efficient Gene Transduction in Cultured Neonatal and Adult Microglia. J. Neurochem. 136 (Suppl. 1), 49–62. doi:10.1111/jnc.13081
Varvel, N. H., Espinosa-Garcia, C., Hunter-Chang, S., Chen, D., Biegel, A., Hsieh, A., et al. (2021). Peripheral Myeloid Cell EP2 Activation Contributes to the Deleterious Consequences of Status Epilepticus. J. Neurosci. 41, 1105–1117. doi:10.1523/jneurosci.2040-20.2020
Vermot, A., Petit-Hartlein, I., Smith, S. M. E., and Fieschi, F. (2021). NADPH Oxidases (NOX): An Overview from Discovery,Molecular Mechanisms to Physiology and Pathology. Antioxidants (Basel) 10, 890. doi:10.3390/antiox10060890
Vezzani, A., Friedman, A., and Dingledine, R. J. (2013). The Role of Inflammation in Epileptogenesis. Neuropharmacology 69, 16–24. doi:10.1016/j.neuropharm.2012.04.004
Wang, Z., Wei, X., Liu, K., Zhang, X., Yang, F., Zhang, H., et al. (2013). NOX2 Deficiency Ameliorates Cerebral Injury through Reduction of Complexin II-Mediated Glutamate Excitotoxicity in Experimental Stroke. Free Radic. Biol. Med. 65, 942–951. doi:10.1016/j.freeradbiomed.2013.08.166
Wang, N., Han, X., Liu, H., Zhao, T., Li, J., Feng, Y., et al. (2017). Myeloid Differentiation Factor 88 Is Up-Regulated in Epileptic Brain and Contributes to Experimental Seizures in Rats. Exp. Neurol. 295, 23–35. doi:10.1016/j.expneurol.2017.05.008
Whitley, S. K., Horne, W. T., and Kolls, J. K. (2016). Research Techniques Made Simple: Methodology and Clinical Applications of RNA Sequencing. J. Investigative Dermatology 136, e77–e82. doi:10.1016/j.jid.2016.06.003
Wu, Z., Liu, Y., Huang, J., Huang, Y., and Fan, L. (2019). MiR-206 Inhibits Epilepsy and Seizure-Induced Brain Injury by Targeting CCL2. Cytotechnology 71, 809–818. doi:10.1007/s10616-019-00324-3
Xueying Li, X., Lin, J., Hua, Y., Gong, J., Ding, S., Du, Y., et al. (2021). Agmatine Alleviates Epileptic Seizures and Hippocampal Neuronal Damage by Inhibiting Gasdermin D-Mediated Pyroptosis. Front. Pharmacol. 12, 627557. doi:10.3389/fphar.2021.627557
Yang, J., Jia, Z., Xiao, Z., Zhao, J., Lu, Y., Chu, L., et al. (2021). Baicalin Rescues Cognitive Dysfunction, Mitigates Neurodegeneration, and Exerts Anti-epileptic Effects through Activating TLR4/MYD88/Caspase-3 Pathway in Rats. Drug Des Devel Ther 15, 3163–3180. doi:10.2147/dddt.s314076
Keywords: epilepsy, neuroinflammatory diseases, temporal lobe, single-cell analysis, cre recombinase, adeno-associated virus, toll-like receptors, transgenic mice
Citation: Almeida C, Pongilio RP, Móvio MI, Higa GSV, Resende RR, Jiang J, Kinjo ER and Kihara AH (2022) Distinct Cell-specific Roles of NOX2 and MyD88 in Epileptogenesis. Front. Cell Dev. Biol. 10:926776. doi: 10.3389/fcell.2022.926776
Received: 23 April 2022; Accepted: 15 June 2022;
Published: 04 July 2022.
Edited by:
Xu Wang, Affiliated Hospital of Jiangsu University, ChinaReviewed by:
Shaunik Sharma, The University of Iowa, United StatesXuefeng Wang, Jiangsu University, China
Ha-Reum Lee, Chungnam National University, South Korea
Copyright © 2022 Almeida, Pongilio, Móvio, Higa, Resende, Jiang, Kinjo and Kihara. This is an open-access article distributed under the terms of the Creative Commons Attribution License (CC BY). The use, distribution or reproduction in other forums is permitted, provided the original author(s) and the copyright owner(s) are credited and that the original publication in this journal is cited, in accordance with accepted academic practice. No use, distribution or reproduction is permitted which does not comply with these terms.
*Correspondence: Alexandre Hiroaki Kihara, YWxleGFuZHJla2loYXJhQGdtYWlsLmNvbQ==