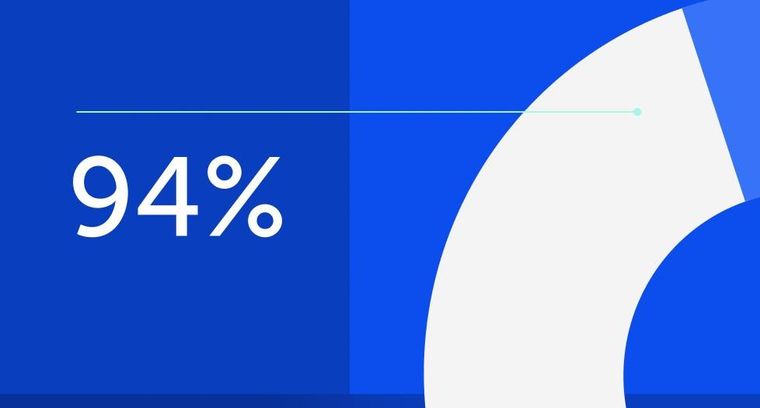
94% of researchers rate our articles as excellent or good
Learn more about the work of our research integrity team to safeguard the quality of each article we publish.
Find out more
ORIGINAL RESEARCH article
Front. Cell Dev. Biol., 22 March 2021
Sec. Stem Cell Research
Volume 9 - 2021 | https://doi.org/10.3389/fcell.2021.606890
This article is part of the Research TopicTissue Engineering and Cell Therapy for Cartilage RestorationView all 15 articles
Collagen is essential for cartilage adhesion and formation. In the present study, histology, immunofluorescence, morphometry, and qRT-PCR suggested that adipose-derived stem cells (ADSCs) stimulated by type V collagen (Col V) induce a significant increase of type II collagen (Col II) in the degenerative area of surgical-induced osteoarthritic rabbit articular cartilage (OA). In vitro, the effects of Col V on the proliferation and differentiation of ADSC were investigated. The expression of the cartilage-related genes Col2a1 and Acan was significantly upregulated and Pou5fl was downregulated post-ADSC/Col V treatment. Post-ADSC/Col V treatment, in vivo analyses revealed that rabbits showed typical signs of osteoarthritic articular cartilage regeneration by hematoxylin and eosin (H&E) and Safranin O/Fast Green staining. Immunohistochemical staining demonstrated that the volume of Col II fibers and the expression of Col II protein were significantly increased, and apoptosis Fas ligand positive significantly decreased post-ADSC/Col V treatment. In conclusion, the expression of Col II was higher in rabbits with surgical-induced osteoarthritic articular cartilage; hence, ADSC/Col V may be a promising therapeutic target for OA treatment.
In humans, osteoarthritis (OA), which involves the degradation of joint cartilage and underlying bone, makes the collagen matrix disorganize and decreases the proteoglycan content within the cartilage (Sanchez-Adams et al., 2014). Without the protective effects of the proteoglycans, the collagen fibers of the cartilage can become susceptible to degradation and, thus, exacerbate the degeneration (MaroudasI, 1976). Inflammation of the synovium and the surrounding joint capsule can also occur. The articular changes cause pain, joint stiffness, and limited joint motion (Gu et al., 2017), strongly impacting the patient’s quality of life and the health system (Pasalini and Fuller, 2018).
Recent studies suggested that the irreparable injury of the cartilage is a major challenge in the treatment of human osteoarthritis and tissue engineering is considered to be an innovative and promising therapy for the patients (Wang et al., 2020; Kader et al., 2021; Tan et al., 2021; Xia et al., 2021). Among various cell therapies, adipose-derived stem cell (ADSC) therapy appears to hold promise (Bistolfi et al., 2021). ADSCs can differentiate into different mesenchymal cell types, including bone, cartilage, and adipose cells. Moreover, ADSCs are immune privileged due to their low immunogenicity. They can enhance the extracellular matrix microenvironment of the articulations by immune modulation of the inflammation and secreting cell growth factors, thus improving tissue repair and cartilage regeneration (Mazor et al., 2014; Wang et al., 2020). Nevertheless, the underlying mechanism is not fully understood.
Chondrocytes are specialized cells that compose the cartilage, synthesizing the collagenous extracellular matrix, an abundant ground substance that is rich in hyaluronic acid. They can be divided according to collagen types into (a) chondrocyte precursor cells (type I collagen), (b) differentiated hyaline chondrocytes (type II, IX, XI, and VI collagen), (c) hypertrophic chondrocytes (type X collagen), and (d) chondrocytes that modulate the synthesis of type I, III, and V collagen. Type II collagen (Col II) constrains the proteoglycans and the extracellular matrix responds to tensile and compressive forces that are experienced by the cartilage, with growth and remodeling of the extracellular matrix.
Type V collagen (Col V) promotes the adhesion and proliferation of chondrocytes and osteogenic cells, thus regulating cartilage adhesion. Col Va1 mutations were detected in patients with osteoarthritis with mild chondrodysplasia (Yang et al., 2018). An interesting study suggested that targeting Col Va2 promoted chondrocyte cell survival and extracellular matrix formation in steroid-induced necrosis of the femoral head (Yang et al., 2018). In addition, Col V is upregulated during adipogenesis and can stimulate adipocyte differentiation in vitro (Nakajima et al., 2002a,b). However, the stimulatory effect of Col V over ADSC proliferation and their ability to synthesize Col II in order to improve articular cartilage regeneration are unknown. Therefore, the present study evaluated the expression profiles of Col II in rabbits with surgical-induced osteoarthritic articular cartilage treated with adipose-derived stem cells (ADSCs) stimulated by Col V.
The animal care and experimental protocols used in this study complied with the ethical principles on animal experimentation, adopted by the Brazilian College of Animal Experimentation (COBEA) of animals used for scientific purpose, and were approved by the ethics committee in the use of animals from the Medical School of the University of São Paulo (Protocol No. 123/14). Twenty-four 15-week-old male New Zealand White rabbits with a mean weight ranging from 2.1 to 2.5 kg were used. The animals were individually housed in specific cages for rabbits, with environmental and food enrichment and under controlled conditions of temperature (room temperature of 22 ± 2°C) and artificial 12-h light–dark cycles. The animals were fed ad libitum with stander feed and water.
Sixteen rabbits were submitted to lipectomy by a longitudinal incision in the interscapular region to isolate the adipose tissue. To isolate ADSCs, adipose tissue from each animal was digested using Liberase (2.5 mg/ml) (Roche Diagnostics GmbH, Penzberg, Germany) for 40 min at 37°C and further centrifuged twice at 2,000 rpm for 5 min at 4°C. The precipitate was transferred to 25 cm2 culture flasks containing Dulbecco’s modified Eagle’s medium (DMEM; Gibco Life Technologies; Invitrogen, Paisley, United Kingdom) supplemented with 10% fetal bovine serum (GibcoTM) and 1% penicillin/streptomycin (Sigma Chemical Co., St. Louis, MO, United States). The culture was maintained at 37°C in an atmosphere of 5% CO2 until confluence. All experimental assays were realized in the third culture passage (Figure 1). The capacity to differentiate toward adipogenic, osteogenic, and chondrogenic lineages was tested using the differentiation kits StemPro®, (Gibco®, Life TechnologiesTM) according to the manufacturer’s recommendations. Cells were stained with Oil Red-O 0.5% (Sigma Chemical Co., St. Louis, MO, United States) in isopropanol 100%, Alcian Blue 1% in HCl 0.1 N, and Alizarin Red (Sigma Chemical Co., St. Louis, MO, United States) to evaluate adipogenic, chondrogenic, and osteogenic differentiation, respectively. Cell lineages were observed under a phase-contrast microscope (Nikon Eclipse TS100, Japan).
Figure 1. Schematic protocol of cell culture, OA induction, and treatment with Col V-stimulated ADSCs and ADSCs (Col V-unstimulated ADSCs). ADSCs, adipose stem cells; Col V, collagen V.
Col V from human placenta (Sigma Chemical Co., St. Louis, MO, United States) was used to stimulate ADSCs. The ideal stimulus concentration of 50 μg/ml of Col V per 72 h was determined by a dose/response curve, using 25, 50, 100, and 200 μg/mL of Col V and control without collagen. The cell cultures were maintained for a period of 1, 2, 3, 7, 14, and 21 days. These cells were cultured over coverslips in DMEM (Gibco Life Technologies, Invitrogen, Paisley, United Kingdom) supplemented with 10% fetal bovine serum (GibcoTM) and 1% penicillin/streptomycin (Sigma Chemical Co., St. Louis, MO, United States) and were maintained at 37°C in an atmosphere of 5% CO2. The expression of Col II, a chondrogenic marker, was the criterion to determinate the ideal concentration and time of ADSC differentiation with Col V stimulus, evaluated by histomorphometry. To evaluate the potential of Col V-stimulated ADSCs, 2.5 × 105 cells in the third culture passage were treated with Col V (50 μg/ml) per 72 h in 25 cm2 culture flasks with DMEM supplemented, in the same above conditions. After this period, cells were harvested with trypsin (Santa Cruz Biotechnology, Inc.), washed with DMEM, centrifuged at 2,000 rpm for 5 min in a conical tube (Falcon), and fixed successively in 4% paraformaldehyde and 70% alcohol. After 1 week, to evaluate Col II expression by immunofluorescence, the pellet was embedded in paraffin and 5 μm sections adhered to the slides, previously treated with 3-dimethylamino silane, dewaxed in xylene, and rehydrated with alcohol in decreasing concentrations, running and distilled water, and PBS. After blocking with 5% BSA, the sections were digested with pepsin (Sigma Chemical Co., St. Louis, MO, United States) 4 mg/ml in 0.5 N acetic acid for 30 min at 37°C. They were then washed in PBS and incubated with mouse polyclonal antibody anti-Col II (1:50, Abcam Inc., Burlingame, CA) overnight at 4°C. Afterward, the specimens were washed in PBS–Tween20 and incubated with goat anti-mouse IgG antibody-Alexa Fluor 488 (1:200) with DAPI (1:200), a nuclear dye. The reaction was visualized utilizing confocal microscopy (ZEISS LSM 510 Meta/UV) and quantified by image analysis software (see below).
The ADSCs were seeded in 75 cm2 culture flasks and divided into three groups: stimulated with Col V (50 μg/ml) for 72 h; TGF-β1 (10 ng/ml) for 72 h, a growth factor widely used to induce cellular differentiation; and without stimulus. Total RNA was extracted by the method of Chomczynski and Sacchi (1987) using the TRIzol reagent and RNA samples were treated with the kit RQ1 RNase-Free DNase (Promega®, Thermo Fisher Scientific, Rockford, IL), according to the manufacturer’s instructions. RT-PCR reactions were performed with the primers drawn to Col2a1 (α1 chain Col II), Acan (aggrecan), and Pou5f1 (POU domain, class 5, transcription factor 1, protein transcription factor involved in the process of self-renewal and maintenance of stem cells) (Table 1).
Gene expression was evaluated using the Real-Time PCR System (Applied Biosystems, Foster City, CA, United States) with the kit SuperScript III Platinum SYBR®, Green One-Step qRT-PCR (Life Technologies). The cycling conditions for the genes were as follows: 50°C for 10 min (for cDNA synthesis) followed by 35 cycles of 95°C for 15 s, 60°C for Gapdh and Col2a1, 59°C for Acan, and 57°C for the Pou5f1 gene for 30 s and 72°C for 30 s. The analysis was performed by the 2–Δ Δ CT method using Gapdh gene as an internal control.
After Col V stimuli evaluation, ADSCs in the third culture passage were cultivated with Col V (50 μg/ml) per 72 h in 75 cm2 culture flasks with DMEM (Gibco Life Technologies, Invitrogen, Paisley, United Kingdom), supplemented with 10% fetal bovine serum (GibcoTM) and 1% penicillin/streptomycin (Sigma Chemical Co., St. Louis, MO, United States), at 37°C in an atmosphere of 5% CO2. The Col V-stimulated ADSCs were cryopreserved in DMEM supplemented with 10% fetal bovine serum and 10% dimethyl sulfoxide (DMSO; Sigma Chemical Co., St. Louis, MO, United States) at −80°C. To therapeutic protocol, the ADSCs viability was evaluated with Trypan blue staining in Neubauer camera and 1 × 106 cells were diluted in 0.3 mL of the Physiological solution.
Animals (n = 24) were anesthetized intramuscularly with xylazine (5 mg/kg) and ketamine (50 mg/kg). The procedures were performed under aseptic conditions. The induction of experimental OA was performed through a lateral parapatellar incision in the right knee followed by the removal of an anterior fourth of the lateral meniscus, preserving the ligaments, according to Moskowitz et al. (1973), with modifications. For the suture, a 4.0 mononylon wire was used at single-spaced points. All animals were given antibiotic (enrofloxacin 5 mg/kg, once daily) for 3 days and an anti-inflammatory (ketoprofen 10 mg/kg, 12/12 h), subcutaneously.
Following the procedure of OA induction and antisepsis of the right knee with iodized alcohol, the OA/ADSC (n = 8) and OA/ADSC/Col V (n = 8) groups were, respectively, treated with autologous intra-articular injections of 0.3 ml of 1 × 106 ADSCs and 0.3 ml of 1 × 106 ADSCs/Col V, which were previously cultured with Col V (50 μg/ml for 72 h). After the cell administration, stretching and flexion movements were made in the knee joint in order to disperse the suspension in the intra-articular space. This treatment was performed monthly for a period of 22 weeks (Figure 1). The OA (n = 8) group received no treatment.
The right (operated) and the left (contralateral unoperated) knee joints of all animals were collected and fixed in 10% buffered formalin about 24 h followed by decalcification with 7% aqueous nitric acid. Then, histological procedures were made for morphological analysis and histological sections (4–5 μm thickness) were stained with H&E and Safranin O/Fast Green.
The following graduation, modified from the OARSI joint injury grade (Pritzker et al., 2006; Pearson et al., 2011; Waldstein et al., 2016), was applied to score the joint injury: grade 0 = when the cartilage surface is smooth; the matrix and chondrocytes are organized into superficial, mid, and deep zones; and the cartilage morphology is intact; grade 1 = when the surface is intact, but with irregularity of superficial fibrillation cluster proliferation and the mid and deep zones are unaffected; grade 2 = when the surface is discontinuous with deep fibrillation, accompanied by cell proliferation and disparity in matrix staining or cell death; grade 3 = when vertical fissures extended vertically until the mid-zone accompanied by cell death and cluster proliferation; grade 4 = when the cartilage is corroded with loss of the cartilaginous matrix, cyst formation within the cartilage matrix, and cluster proliferation; grade 5 = when the matrix is exposed and hyaline cartilage is unmineralized and completely eroded; the bone plate with microfracture and reparative fibrocartilage occupying gaps in the surface; and grade 6 = when the matrix is deformed, with microfracture, fibrocartilaginous, and osseous repair extending above the previous surface. The resulting changes in the contour of the articular surface are illustrated in Supplementary Figure 1.
The characterization of chondrocyte apoptosis and Col II expression was assessed, respectively, by immunoperoxidase (IP) and immunofluorescence (IF). Tissue sections (4 mm thick) were cut from formalin-fixed, paraffin-embedded blocks containing representative cells and tissue and processed for immunohistochemistry (IHC).
The chondrocyte apoptosis in the articular cartilage was evaluated by immunohistochemistry for Fas ligand using the mouse anti-FasL monoclonal antibody (1:20, Neomarkers, Fremont, CA) and the Novolink development kit (Leica Biosystems, New Castle Ltd., United Kingdom), according to the manufacturer’s specifications.
The cartilage sections on slides were deparaffinized in xylene-alcohol and rehydrated in running water, distilled water, and phosphate buffered saline. Firstly, enzymatic digestion with chondroitinase ABC 2UN (Sigma Chemical Co., St. Louis, MO, United States) in buffer containing 50 mM Tris, pH = 8.0, sodium acetate 60 mM, and 0.02% BSA at 37°C for 3 h was performed for exposure and recovery of antigenic sites. Subsequently, antigenic recovery was done with 8 mg/ml porcine pepsin (Sigma Chemical Co., St. Louis, MO, United States, 10,000 UTI/ml) diluted in acetic acid 5 N for 30 min at 37°C. For blocking non-specific sites, the sections were incubated with 5% BSA in PBS for 30 min. Polyclonal mouse anti-Col II antibody (1:20; Abcam, Burlingame, CA, United States) was incubated overnight at 4°C. After a PBS 0.05%–Tween20 washing cycle, goat anti-mouse IgG antibody (Alexa Fluor 488, Invitrogen, Life Technologies) diluted (1:150) in PBS and 0.006% Evans blue was incubated at room temperature for 60 min. After the sections were washed and mounted in glycerin-PBS (v/v), they were analyzed using an Olympus BX51 fluorescence microscope (Olympus Corp., Tokyo, Japan).
The quantification of histologic, immunoperoxidase, and immunofluorescence parameters was done by digital imaging through the Image-Pro Plus 6.0 software. Briefly, the image analysis system consisted of an Olympus camera (Olympus Corporation, St Laurent, Quebec, Canada) coupled to an Olympus microscope (Olympus BX51), from which the images were sent to an LG monitor by means of a digitizing system (Oculus TCX, Coreco Inc., St Laurent, Quebec, Canada) and downloaded to a computer (Pentium 1330 MHz). Ten images from the culture were processed with the software (Image-Pro Plus 6.0).
The histologic sections of femoral condyles and tibial plates stained with H&E were scanned at ×200 magnification to evaluate cartilage thickness. Five vertical lines were drawn from the cartilage surface to the tidemark, starting from a central point of the tissue and 250 and 500 mm to the right and to the left. The cartilage thickness was calculated in μm2 from the arithmetic mean of the drawn lines. The density of the chondrocyte per cartilage area was evaluated by the stereological point-counting method developed by Gundersen et al. (1988) with modifications. Using the measurement tools of the Image-Pro Plus 6.0 software, a reticulum with 100 points orthogonally distributed over the acquired image was constructed. The cells coincident with the points in the reticulum were counted and the result was given as percentage of chondrocyte cells per mm2.
The proteoglycan levels in the cartilage were evaluated based on the quantification of the Safranin O/Fast Green staining intensity using the Image-Pro Plus 6.0 software (Pastoureau et al., 2010). Ten images in ×400 magnification were acquired and the staining intensity was measured by the software. The mean of the area stained in blue was divided by the mean of the total area analyzed, and the result expressed as percentage per mm2.
The Col II immunofluorescence in cultures and tissue was analyzed through images acquired at ×400. The area of each field analyzed was measured in μm2 and the mean of the immunostaining area corresponding to Col II was divided by the mean of the total area analyzed and the final result was expressed as percentage of fibers/mm3.
Statistical analysis was performed using GraphPad Prism 4.0 software (GraphPad Prism, Inc., San Diego, CA, United States). One-way analysis of variance (ANOVA) with Newman–Keuls post-test for comparison between groups or Kruskal–Wallis test followed by multiple Dunn’s test for non-parametric data was used. Results are expressed as mean ± standard errors of the mean (SEM); a P < 0.05 was considered statistically significant.
ADSCs grown in specific differentiation media were able to differentiate into adipogenic, chondrogenic, and osteogenic lineages, characterized, respectively, by the presence of cytoplasmic lipid droplets (Oil Red), synthesis of proteoglycans by the presence of a proteoglycan-rich matrix (Alcian Blue), and aggregates of calcium salts with calcium deposits (Alizarin Red) (Figures 2A–D).
Figure 2. ADSCs grown in specific medio and differentiation into adipogenic, chondrogenic and osteogenic lineages. Adipogenic lineage presents cytoplasmic lipid droplets red stained with Oil Red (B), chondrogenic lineage shows matrix proteoglycans production identified by Alcian Blue staining (blue) (C) and osteogenic lineage exhibit calcium salts identified by Alizarin Red (D). Control ADSCs grown in DMEM (A). The proliferation of ADSCs (E–M) and expression of chondrogenic related genes and were increased post-ADSCs/Col V and TGF-β treatment. The expression of Col2a1 (N), Acan (O) and Poup5f1 (P) at the mRNA level was measured by RT-PCR after 3 and 7 days of incubation in the chondrogenic medium of ColV and TGF-β treated ADSCs. The cell viability of ADSCs was detected by CCK-8. ADSCs, adipose stem cells. The experiments were performed in triplicate. *P < 0.05; **P < 0.001; ***P < 0.0001.
The impact of Col V in ADSC proliferation was assessed (Figures 2E–L). After incubation with Col V 50 μg/ml for 3 days, the proliferation assay revealed that Col V enhanced the proliferation of ADSCs in a dose-dependent manner (Figure 2M). Moreover, the expression of chondrogenic-related genes was measured by real-time qPCR. As expected, the expression of Col2a1 and Acan genes was significantly upregulated post-Col V/ADSC incubation after chondrogenic differentiation at days 3 and 7 (both P < 0.05, Figures 2N,O), whereas Pou5f1 gene expression showed a tendency to be downregulated (P = 0.07, Figure 2P). A similar expression of Col2a1 and Acan genes was found with TGF-β1, a growth factor that stimulates ADSCs (Figures 2N–P).
Figures 3A–G show the immunofluorescence of Col II in vitro and in vivo post-ADSC treatment stimulated with Col V for 3 days (50 μg/ml). A strong and diffuse green birefringence of Col II was observed in the pellets of ADSCs/Col V (Figure 3B), contrasting with the weak and focal birefringence in ADSCs (Figure 3A). These features coincide with the significant increase of Col II expression in ADSCs/Col V when compared with ADSCs (P < 0.01) (Figure 3C). A similar strong and diffuse green birefringence of Col II was observed in osteoarthritic rabbit articular cartilage post-ADSCs stimulated with Col V intra-articular injection (Figure 3F). Of note is the progressive increase of Col II expression from osteoarthritis (Figure 3D) and OA/ADSCs (Figure 3E) to osteoarthritis being maximum and significant in OA/ADSCs/Col V (P < 0.05).
Figure 3. Representative images of the strong green birefringence by immunofluorescence of Col II after 3 days with Col V (50 mg/ml) during the process of Col V/ADSC treatment in vitro (A,B) and in vivo (C–F). The (G) shows the representative graphics of the amount of Col II expression in vitro and in vivo evaluated by Image-Pro Plus 6.1 software. *P < 0.05.
Figure 4A shows the gross characteristics of the femoral condyle from the OA group including thinning of the cartilage and having a pearly white color and several stretch marks of chondral erosions in the cartilage. The lesions were less severe in the OA/ADSC group presenting cartilage with superficial erosions and focal stretch marks of chondral erosions (Figure 4B). These gross characteristics contrast with the bright white color and regular surface of the femoral chondral cartilage in the joints of OA/ADSC/Col V animals (Figure 4C), similar to control (Figure 4D).
Figure 4. The morphogenic changes showed typical signs of osteoarthritic rabbit articular cartilage regeneration post-ADSCs/Col V. (A–D) Coronal section of representative femoral heads. The circle indicates the femoral condyle cartilage degeneration. Hematoxylin and eosin (E–H) and Safranin O/Fast Green (I–L) staining. Chondrocytes apoptosis Fas ligand+(arrows) (M–P). Morphometric analysis showing cartilage parameters of the upper outer subchondral bone of the femoral heads (Q), chondrocytes (R), proteoglycans (S), and apoptosis (T). *P < 0.05.
Histologically, the OA group coincided with severe stretch marks and thinning cartilage, osteophyte formation, subchondral sclerosis, cellular disorganization of chondrocytes and reduction of bone trabeculae, beyond complete tidemark discontinuity (Figure 4E), and weak Safranin O/Fast Green staining intensity of the extracellular matrix indicating reduction of glycosaminoglycans (Figure 4I). The OA/ADSC joints show less severe osteoarthritic histologic changes compared with those of the OA group with superficial fibrillations, moderate cellular disorganization, maintenance of the tidemark, and subchondral bone with less sclerotic aspect (Figure 4F). In this group, Safranin O/Fast Green staining intensity was moderate, indicating minor loss of proteoglycan content (Figure 4J). In contrast, the OA/ADSC/Col V group showed only stretch marks of the cartilaginous surface with recovery of cartilage thickness (Figure 4G), minor cellular disorganization, continuous tidemark, subchondral bone without sclerotic aspect (Figure 4G), and maintenance of proteoglycan content compared with the OA and OA/ADSCs (Figures 4I–K), similar to control cartilage (Figures 4H,L). Supplementary Figure 1 shows the injury grades in OA, OA/ADSCs, and OA/ADSCs/Col V. The OA/ADSCs/Col V presented a significant cartilage regeneration in relation to OA (Figure 5; P = 0.019).
Figure 5. OARSI score grading for histologic alteration in OA, OA/ADSCs, and OA/ADSCs/Col V. *P < 0.05.
These histological changes in the rabbits’ joints from the OA/ADSC/Col V group, which had been injected with ADSCs/Col V, coincide with the significant increase of cartilage thickness (P < 0.05; Figure 4Q), chondrocyte density (P < 0.05; Figure 4R), proteoglycans repair (P < 0.05; Figure 4S), and apoptosis reduction (P < 0.05; Figures 4M–P,T). Regarding the OA/ADSC group, intra-articular injection of ADSCs was also able to significantly increase chondrocyte density and cartilage thickness, and there was reduction of apoptosis than the amount observed in rabbits from the OA group but much less than that from the ADSC/Col V group.
Under the conditions of the present study, we found plenty of associated effects in rabbit OA. They included the following: (1) Col V enhances rabbit ADSC proliferation and differentiation and increases in vitro Col II expression in ADSCs; (2) in the in vitro expression of cartilage-related genes, Col2a1 and Acan were significantly upregulated and Pou5fl was downregulated post-ADSC/Col V treatment; and (3) in vivo, the injury grades post-ADSC/Col V treatment showed typical signs of osteoarthritic articular cartilage regeneration, including decreased cartilage thickness, increased number of chondrocytes, decreased proteoglycan loss, decreased number of apoptotic chondrocytes, and increased expression of Col II protein. The current study showed that ADSCs stimulated by Col V (ADSCs/Col V) induced a significant regeneration of cartilage, indicating that ADSCs/Col V may be a therapeutic target for the treatment of osteoarthritis.
The experiments in the present study were performed in a surgical-induced osteoarthritic rabbit articular cartilage, established by Moskowitz et al. (1973) and modified by Velosa et al. (2007), which reproduces progressively the morphological changes found in human OA. The established period of 22 weeks was described equivalent to an advanced time of disease, with macroscopic lesions of the cartilage and histology features, characterized by cell disorganization, presence of cell clones, fibrillations, and fissures, in addition to loss of collagen and proteoglycans (Velosa et al., 2007).
Regarding the concentration of Col V used to stimulate ADSCs, the treatment time, the number of cells administered, and the route of administration, we employed a therapeutic protocol based on a monthly intra-articular administration of ADSCs 106/Col V 50 μg/ml, which is the ideal stimulus concentration based on cell culture proliferation and expression of Col II, a chondrogenic marker, during a 22-week period (Velosa et al., 2007). Intra-articular administration was chosen considering that the cells will be applied in a joint in a closed environment. Moreover, it simulates clinical practice. In addition, we decided not to perform the treatment with a shorter interval, considering that some studies detected mesenchymal stem cells (MSCs) up to 1 month after intra-articular injection (Satué et al., 2019).
The balance between the synthesis and degradation of matrix proteins in the extracellular matrix (ECM) is crucial for the dynamic stability of tissues (Humphrey et al., 2014). The interaction of chondrocytes with the cartilage ECM plays an important role for chondrocyte anchorage, proliferation, differentiation, and function (He et al., 2014). In cell culture, chondrocytes have the ability to synthesize a large variety of matrix proteins, including collagen types I, II, III, IV, and V and fibronectin (Aigner et al., 1993). Col V is synthesized and deposited in the ECM, where it cross-linked with Col I and III, to form the collagenous matrix skeleton (Mak et al., 2016). By histological and proteomic analyses, Hong et al. (2010) reported that Col V in the ECM improved cell distribution and actin fiber frame promoting adhesion in the initial stage of chondroblast differentiation. The growth and remodeling of the cartilage matrix may revert morphologic injury. Moreover, the present study showed that the gene and protein profile of Col II was significantly higher in OA rabbits, and its upregulation was confirmed both in vitro and in vivo. These findings suggest that the balance between Col V and Col II expression may revert to the pathogenesis of OA.
Chondrocytes are the only cell type composing the articular cartilage and encompass only 2–5% of its compartment, the remaining being occupied by a hydrous ECM of collagens (mostly Col II) and proteoglycans. The main function of chondrocytes is to preserve the integrity of the cartilage by reverting injury to the matrix. Nevertheless, in OA, the biomechanical and biochemical microenvironment of the cartilage is altered, as well as in the performance of chondrocytes. These collateral changes are considered in part due to repression of chondroblast differentiation from MSCs and apoptosis of chondrocytes (Hwang and Kim, 2015). At early stages, OA is mainly characterized by the apoptosis of chondrocytes, then the reparative reaction of the cartilage is initiated. The imbalance between metalloprotease-mediated cartilage resorption and chondroblast-mediated cartilage restoration led to distorted histoarchitecture and progressive breakdown of joints. As the cartilage is the main affected component in OA, molecular studies of OA have focused on the mechanism of damage to the articular cartilage (Bertrand et al., 2010). Cartilage degeneration in OA is a progressive process complemented with the gradual loss of Col II and a gradual decrease in mRNA expression of Acan and Col2a1 (Buckwalter et al., 2004; Brew et al., 2010). As expected, we found that the gene expression of the chondrocyte markers Acan and Col2a1 was increased after ADSC/Col V treatment. In contrast, we found decreased expression of Pou5f1 gene post-ADSC/Col V treatment. Previous studies have reported that Pou5f1 works in maintaining the cancer stem cell fate of osteosarcoma (Siclari and Qin, 2010). Guo et al. (2017) demonstrated that Pou5f1 gene expression was downregulated by miR-335, and suggested that it could repress Pou5f1 expression by post-translational regulation. They also found that cells expressing miR-335 possessed decreased stem cell-like properties. Although Pou5f1 has not been reported in OA pathology, we infer that Pou2f1 might also be a candidate gene connected with OA pathology and ADSC/Col V treatment.
The mechanism of action of Col V in the stimulation of ADSCs to synthesize Col II is unknown. However, based on our results, we infer that the biochemistry characteristics and biologic functions of Col V may induce cell proliferation and differentiation of ADSCs to produce collagen as previously reported (Ruggiero et al., 1994; Mak et al., 2016). Collagen type V works as crucial components for preserving the structural integrity of the cartilage skeleton, promoting adhesion and proliferation of several cell types including fibroblasts and chondrocytes (Fichard et al., 1995; Breuls et al., 2009; Wu et al., 2009). These properties of cell adhesion, migration, and proliferation are primarily due to tripeptide sequence consisting of arginine, glycine, and aspartic acid (RGD) sequences present in greater quantity in the α2(V) chain that bind primarily to the membrane integrins and could stimulate ADSC proliferation and differentiation (Ruggiero et al., 1994, 1996). Col V adhesion may also occur through a 30-kDa α1(V) chain fragment containing a pool of basic amino acid residues with affinity to heparin and heparin sulfate, which could enhance its interaction with anionic heparin molecules. In this way, Col V also interacts with a number of molecules in the extracellular matrix such as proteoglycans (PG), heparin sulfate, and other triggering cellular stimulus signals (Ricard-Blum et al., 2006; Symoens et al., 2011). Col V specifically decreases endothelial cell proliferation promoting cell detachment by the disassembly of F-actin filaments, and cells started to proliferate when recultured on Col I (Mak et al., 2016). Moreover, heterozygous mutation in Col Va2 gene could lead to connective tissue hyperelasticity and joint instability (Johnston et al., 2017). In the current study, we verified that ADSCs stimulated by Col V increased Col II synthesis, the main collagenous protein of the hyaline cartilage matrix, and decreased apoptotic chondrocytes. Based on the study from Nakajima et al. (2002a,b), who demonstrated that Col V was upregulated during adipogenesis and that it can stimulate adipocyte differentiation in vitro, we inferred that ADSCs stimulated by Col V enhance the expression of Col II, increasing the density of cartilage tissue. Another hypothetical translational significance of this finding is that Col II deficiency may participate in the articular deterioration progress of OA. However, how ADSCs stimulated by Col V enhancement of the expression of Col II could promote the healing process of cartilage tissue in OA needs further studies using transgenic Col V ADSCs to unveil the potential role of Col V in OA.
In the present study, we also used TGF-β1, another established growth factor for in vitro ADSC chondrogenic differentiation (Yarak and Okamoto, 2010) and Col II synthesis (Indrawattana et al., 2004; Shirasawa et al., 2006; Kraus, 2011; Das et al., 2015; Kwon et al., 2016), to evaluate and compare the expression of Col2a1 and Acan genes in ADSC stimulated by Col V. Of note, we demonstrated similar Col2a1 and Acan gene expression in ADSCs stimulated with Col V and ADSCs stimulated with TGF-β1 in relation to the control culture. The similar fold change of the expression of these genes between the two stimuli suggests once again that Col V could act as a modulator of the collagen chondrogenic. In fact, recent studies showed that ADSCs stimulated with TGF-β3 and BMP-6 had increased chondrogenic gene expressions and the model of osteoarthritic knees treated with these cells had improvement of cartilage scores (Ude et al., 2018). In addition to the application of MSCs in animal models, clinical trials and meta-analytic studies have shown cartilage improvement, reduction in pain, and improvement in joint function in patients with OA after autologous MSC injections (Centeno et al., 2008; Koh et al., 2013; Robinson et al., 2018; Huang et al., 2020). Additionally, other authors have shown that a single intra-articular injection of integrin α10β1 derived from allogeneic adipose tissue was effective in treating OA in horses, showing decreased articular cartilage fibrillation (Delco et al., 2020). Since the intra-articular cavity is closed, the loss of a few stem cells is expected. Previous studies have shown that MSCs are present up to 1 month after intra-articular injection (Satué et al., 2019), and these were being done in clinical trials (Jo et al., 2014). If stem cells are still present even after 1 month of injection, this reflects a few stem cell loss. In addition, compared with intravenous injection (Eggenhofer et al., 2012), stem cells injected in the intra-articular cavity can stay longer.
In conclusion, by applying immunofluorescence, morphometry, and qRT-PCR analysis, this study demonstrated that post-ADSC stimulation by Col V treatment increased the repair process in an osteoarthritic rabbit articular cartilage model. These findings suggested that surgical-induced OA treated with ADSCs stimulated by Col V may prevent the progression of cartilage injury; nevertheless, more studies are needed in order to unveil the immunological role of ADSCs stimulated by Col V in the pathogenesis of OA.
The raw data supporting the conclusions of this article will be made available by the authors, without undue reservation.
The animal study was reviewed and approved by Ethics Committee in the use of animals from Medical School of the University of São Paulo (Protocol No. 123/14).
IB contributed the methodology, data acquisition, and original draft of the manuscript. AV contributed to the methodology, draft of the manuscript and editing the manuscript, and revising the work critically for intelectual content. SC, EP, and AS contributed to the methodology. CG-S contributed to the resources. CF contributed to the data acquisition, review of the analysis, and interpretation of the data. AG contributed to the data acquisition, review of statistical analysis, and revising the work critically for intelectual content. PS and VC contributed to the review of the analysis and interpretation of data, revising the work critically for intelectual content, and final approval of the version to be published. TF, DB, and RF critically reviewed the manuscript. WT contributed to the conceptualization, draft of the manuscript, funding acquisition, data statistic analysis, and final approval of the version to be published.
The research was supported by the São Paulo Research Foundation (FAPESP – Process number 2014/11419-5; FAPESP 2018/20403-6 to VC) and the National Council for Scientific and Technological Development (CNPq) (483005/2012-6 to VC).
The authors declare that the research was conducted in the absence of any commercial or financial relationships that could be construed as a potential conflict of interest.
The Supplementary Material for this article can be found online at: https://www.frontiersin.org/articles/10.3389/fcell.2021.606890/full#supplementary-material
Aigner, T., Bertling, W., Stöss, H., Weseloh, G., and von der Mark, K. (1993). Independent expression of fibril-forming collagens I, II, and III in chondrocytes of human osteoarthritic cartilage. J. Clin. Invest. 91, 829–837. doi: 10.1172/jci116303
Bertrand, J., Cromme, C., Umlauf, D., Frank, S., and Pap, T. (2010). Molecular mechanisms of cartilage remodelling in osteoarthritis. Int. J. Biochem. Cell Biol. 42, 1594–1601. doi: 10.1016/j.biocel.2010.06.022
Bistolfi, A., Roato, I., Fornelli, G., Sabatini, L., Massč, A., and Ferracini, R. (2021). Treatment of knee osteoarthritis by intra-articular injection of concentrated autologous adipose tissue: a twenty-four-month follow-up study. Int. Orthop. 45, 627–633. doi: 10.1007/s00264-020-04923-0
Breuls, R. G. M., Klumpers, D. D., Everts, V., and Smit, T. H. (2009). Collagen type V modulates fibroblast behavior dependent on substrate stiffness. Biochem. Biophys. Res. Commun. 380, 425–429. doi: 10.1016/j.bbrc.2009.01.110
Brew, C. J., Clegg, P. D., Boot-Handford, R. P., Andrew, J. G., and Hardingham, T. (2010). Gene expression in human chondrocytes in late osteoarthritis is changed in both fibrillated and intact cartilage without evidence of generalised chondrocyte hypertrophy. Ann. Rheum. Dis. 69, 234–240. doi: 10.1136/ard.2008.097139
Buckwalter, J. A., Saltzman, C., and Brown, T. (2004). The impact of osteoarthritis: implications for research. Clin. Orthop. Relat. Res. 427, S6–S15. doi: 10.1097/01.blo.0000143938.30681.9d
Centeno, C. J., Busse, D., Kisiday, J., Keohan, C., and Freeman, M. (2008). Increased knee cartilage volume in degenerative joint disease using percutaneously implanted, autologous mesenchymal stem cells, platelet lysate and dexamethasone. Am. J. Case Rep. 9, 246–251.
Chomczynski, P., and Sacchi, N. (1987). Single-step method of RNA isolation by acid guanidinium thiocyanate-phenol-chloroform extraction. Anal. Biochem. 162, 156–159. doi: 10.1006/abio.1987.9999
Das, R., Timur, U. T., Edip, S., Haak, E., Wruck, C., Weinans, H., et al. (2015). TGF-β2 is involved in the preservation of the chondrocyte phenotype under hypoxic conditions. Ann. Anat. 198, 1–10. doi: 10.1016/j.aanat.2014.11.003
Delco, M. L., Goodale, M., Talts, J. F., Sarah, L., Koff, M. F., Miller, A. D., et al. (2020). Integrin α10β1-selected mesenchymal stem cells mitigate the progression of osteoarthritis in an equine talar impact model. Am. J. Sports Med. 48, 612–623. doi: 10.1177/0363546519899087
Eggenhofer, E., Benseler, V., Kroemer, A., Popp, F. C., Geissler, E. K., Schlitt, H. J., et al. (2012). Mesenchymal stem cells are short-lived and do not migrate beyond the lungs after intravenous infusion. Front. Immunol. 3:297. doi: 10.3389/fimmu.2012.00297
Fichard, A., Kleman, J. P., and Ruggiero, F. (1995). Another look at collagen V and XI molecules. Matrix Biol. 14, 515–531. doi: 10.1016/s0945-053x(05)80001-0
Gu, Y. T., Chen, J., Meng, Z. L., Ge, W. Y., Bian, Y. Y., Cheng, S. W., et al. (2017). Research progress on osteoarthritis treatment mechanisms. Biomed. Pharmacother. 93, 1246–1252.
Gundersen, H. J., Bagger, P., Bendtsen, T. F., Evans, S. M., Korbo, L., Marcussen, N., et al. (1988). The new stereological tools: disector, fractionator, nucleator and point sampled intercepts and their use in pathological research and diagnosis. APMIS 96, 857–881. doi: 10.1111/j.1699-0463.1988.tb00954.x
Guo, X., Yum, L., Zhang, Z., Dai, G., Gao, T., and Guo, W. (2017). miR-335 negatively regulates osteosarcoma stem cell-like properties by targeting POU5F1. Cancer Cell Int. 17, 29. doi: 10.1186/s12935-017-0398-6
He, F., Liu, X., Xiong, K., Chen, S., Zhou, L., and Cui, W. (2014). Extracellular matrix modulates the biological effects of melatonin in mesenchymal stem cells. J. Endocrinol. 223, 167–180. doi: 10.1530/joe-14-0430
Hong, D., Chen, H.-C., Yun, H.-Q., Liang, Y., Wang, C., Lian, Q.-Q., et al. (2010). Morphological and proteomic analysis of early stage of osteoblast differentiation in osteoblastic progenitor cells. Exp. Cell Res. 316, 2291–2300. doi: 10.1016/j.yexcr.2010.05.011
Huang, R., Li, W., Zhao, Y., Yang, F., and Xu, M. (2020). Clinical efficacy and safety of stem cell therapy for knee osteoarthritis: a meta-analysis. Medicine 99, e19434. doi: 10.1097/MD.0000000000019434
Humphrey, J. D., Dufresne, E. R., and Schwartz, M. A. (2014). Mechanotransduction and extracellular matrix homeostasis. Nat. Rev. Mol. Cell Biol. 15, 802–812. doi: 10.1038/nrm3896
Hwang, H. S., and Kim, H. A. (2015). Chondrocyte apoptosis in the pathogenesis of osteoarthritis. Int. J. Mol. Sci. 16, 26035–26054. doi: 10.3390/ijms161125943
Indrawattana, N., Chen, G., Tadokoro, M., Shann, L. H., Ohgushi, H., Tateishi, T., et al. (2004). Growth factor combination for chondrogenic induction from human mesenchymal stem cell. Biochem. Biophys. Res. Commun. 320, 914–919. doi: 10.1016/j.bbrc.2004.06.029
Jo, C. H., Lee, Y. G., Shin, W. H., Kim, H., Chai, J. W., Jeong, E. C., et al. (2014). Intra-articular injection of mesenchymal stem cells for the treatment of osteoarthritis of the knee: a proof-of-concept clinical trial. Stem Cells 32, 1254–1266. doi: 10.1002/stem.1634
Johnston, J. M., Connizzo, B. K., Shetye, S. S., Robinson, K. A., Huegel, J., Rodriguez, A. B., et al. (2017). Collagen V haploinsufficiency in a murine model of classic Ehlers-Danlos syndrome is associated with deficient structural and mechanical healing in tendons. J. Orthop. Res. 35, 2707–2715. doi: 10.1002/jor.23571
Kader, N., Asopa, V., Baryeh, K., Sochart, D., Maffulli, N., and Kader, D. (2021). Cell-based therapy in soft tissue sports injuries of the knee: a systematic review. Expert Opin. Biol. Ther. 19, 1–13. doi: 10.1080/14712598.2021.1872538
Koh, Y. G., Jo, S. B., Kwon, O. R., Suh, D. S., Lee, S. W., Park, S. H., et al. (2013). Mesenchymal stem cell injections improve symptoms of knee osteoarthritis. Arthroscopy 29, 748–755. doi: 10.1016/j.arthro.2012.11.017
Kraus, V. B. (2011). Osteoarthritis year 2010 in review: biochemical markers. Osteoarthritis Cartilage 19, 346–353. doi: 10.1016/j.joca.2011.02.002
Kwon, H., Paschos, N. K., Hu, J. C., and Athanasiou, K. (2016). Articular cartilage tissue engineering: the role of signaling molecules. Cell. Mol. Life Sci. 73, 1173–1194. doi: 10.1007/s00018-015-2115-8
Mak, K. M., Png, C. Y., and Lee, D. J. (2016). Type V collagen in health, disease, and fibrosis. Anat. Rec. 299, 613–629. doi: 10.1002/ar.23330
Maroudas, A. I (1976). Balance between swelling pressure and collagen tension in normal and degenerate cartilage. Nature 260, 808–809. doi: 10.1038/260808a0
Mazor, M., Lespessailles, E., Coursier, R., Daniellou, R., Best, T. M., and Toumi, H. (2014). Mesenchymal stem-cell potential in cartilage repair: an update. J. Cell. Mol. Med. 18, 2340–2350.
Moskowitz, R. W., Davis, W., Sammarco, J., Martens, M., Baker, J., Mayor, M., et al. (1973). Experimentally induced degenerative joint lesions following partial meniscectomy in the rabbit. Arthritis Rheum. 16, 397–405. doi: 10.1002/art.1780160317
Nakajima, I., Muroya, S., Tanabe, R., and Chikuni, K. (2002a). Extracellular matrix development during differentiation into adipocytes with a unique increase in type v and vi collagen. Biol. Cell 94, 197–203. doi: 10.1016/s0248-4900(02)01189-9
Nakajima, I., Muroya, S., Tanabe, R., and Chikuni, K. (2002b). Positive effect of collagen V and VI on triglyceride accumulation during differentiation in cultures of bovine intramuscular adipocytes. Differentiation 70, 84–91. doi: 10.1046/j.1432-0436.2002.700203.x
Pasalini, T. S. P., and Fuller, R. (2018). Public social security burden of musculoskeletal diseases in Brazil – descriptive study. Rev. Assoc. Med. Bras. 64, 339–345. doi: 10.1590/1806-9282.64.04.339
Pastoureau, P. C., Hunziker, E. B., and Pelletier, J. P. (2010). Cartilage, bone and synovial histomorphometry in animal models of osteoarthritis. Osteoarthritis Cartilage 3, S106–S112. doi: 10.1016/j.joca.2010.05.024
Pearson, R. G., Kurien, T., Shu, K. S., and Scammell, B. E. (2011). Histopathology grading systems for characterisation of human knee osteoarthritis – reproducibility, variability, reliability, correlation, and validity. Osteoarthritis Cartilage 19, 324–331. doi: 10.1016/j.joca.2010.12.005
Pritzker, K. P. H., Gay, S., Jimenez, S. A., Ostergaard, K., Pelletier, J. P., Revell, P. A., et al. (2006). Osteoarthritis cartilage histopathology: grading and staging. Osteoarthritis Cartilage 14, 13–29. doi: 10.1016/j.joca.2005.07.014
Ricard-Blum, S., Beraud, M., Raynal, N., Farndale, R. W., and Ruggiero, F. (2006). Structural requirements for heparin/heparan sulfate binding to type V collagen. J. Biol. Chem. 281, 25195–25204. doi: 10.1074/jbc.M603096200
Robinson, P. G., Iain, R. M., West, C. C., Goudie, E. B., Yong, L. Y., White, T. O., et al. (2018). Reporting of mesenchymal stem cell preparation protocols and composition: a systematic review of the clinical orthopaedic literature. Am. J. Sports Med. 47, 991–1000. doi: 10.1177/0363546518758667
Ruggiero, F., Champliaud, M. F., Garrone, R., and Aumailley, M. (1994). Interactions between cells and collagen V molecules or single chains involve distinct mechanisms. Exp. Cell Res. 210, 215–223. doi: 10.1006/excr.1994.1032
Ruggiero, F., Comte, J., Cabañas, C., and Garrone, R. (1996). Structural requirements for alpha 1 beta 1 and alpha 2 beta 1 integrin mediated cell adhesion to collagen V. J. Cell Sci. 109, 1865–1874.
Sanchez-Adams, J., Leddy, H. A., McNulty, A. L., O’Conor, C. J., and Guilak, F. (2014). The mechanobiology of articular cartilage: bearing the burden of osteoarthritis. Curr. Rheumatol. Rep. 16, 451. doi: 10.1007/s11926-014-0451-6
Satué, M., Schüler, C., Ginner, N., and Erben, R. G. (2019). Intra-articularly injected mesenchymal stem cells promote cartilage regeneration, but do not permanently engraft in distant organs. Sci. Rep. 9, 10153. doi: 10.1038/s41598-019-46554-5
Shirasawa, S., Sekiya, I., Sakaguchi, Y., Yagishita, K., Ichinose, S., and Muneta, T. (2006). In vitro chondrogenesis of human synovium-derived mesenchymal stem cells: optimal condition and comparison with bone marrow-derived cells. J. Cell Biochem. 97, 84–97. doi: 10.1002/jcb.20546
Siclari, V. A., and Qin, L. (2010). Targeting the osteosarcoma cancer stem cell. J. Orthop. Surg. Res. 5, 78. doi: 10.1186/1749-799X-5-78
Symoens, S., Renard, M., Bonod-Bidaud, C., Syx, D., Vaganay, E., Malfait, F., et al. (2011). Identification of binding partners interacting with the α1-N-propeptide of type V collagen. Biochem. J. 433, 371–381. doi: 10.1042/BJ20101061
Tan, S. H. S., Kwan, Y. T., Neo, W. J., Chong, J. Y., Kuek, T. Y. J., See, J. Z. F., et al. (2021). Intra-articular injections of mesenchymal stem cells without adjuvant therapies for knee osteoarthritis: a systematic review and meta-analysis. Am. J. Sports Med. 20, 363546520981704. doi: 10.1177/0363546520981704
Ude, C. C., Shamsul, B. S., Ng, M. H., Chen, H. C., Ohnmar, H., Amaramalar, S. N., et al. (2018). Long-term evaluation of osteoarthritis sheep knee, treated with TGF-β3 and BMP-6 induced multipotent stem cells. Exp. Gerontol. 104, 43–51. doi: 10.1016/j.exger.2018.01.020
Velosa, A. P. P., Oliveira, A. M., Carrasco, S., Capelozzi, V. L., Teodoro, W. R., and Yoshinari, N. H. (2007). Partial meniscectomy as an experimental model of osteoarthritis in rabbits and protector effect of chloroquine diphosphate. Rev. Bras. Reumatol. 47, 401–410.
Waldstein, W., Perino, G., Gilbert, S. L., Maher, S. A., Windhager, R., and Boettner, F. (2016). OARSI osteoarthritis cartilage histopathology assessment system: a biomechanical evaluation in the human knee. J. Orthop. Res. 34, 135–140. doi: 10.1002/jor.23010
Wang, J., Zhou, L., Zhang, Y., Huang, L., and Shi, Q. (2020). Mesenchymal stem cells – a promising strategy for treating knee osteoarthritis. Bone Joint Res. 9, 719–728. doi: 10.1302/2046-3758.910.bjr-2020-0031.r3
Wu, J. J., Weis, M. A., Kim, L. S., Carter, B. G., and Eyre, D. R. (2009). Differences in chain usage and cross-linking specificities of cartilage type V/XI collagen isoforms with age and tissue. J. Biol. Chem. 284, 5539–5545. doi: 10.1074/jbc.m806369200
Xia, P., Wang, X., Wang, Q., Wang, X., Lin, Q., Cheng, K., et al. (2021). Low-Intensity pulsed ultrasound promotes autophagy-mediated migration of mesenchymal stem cells and cartilage repair. Cell Transplant. 30, 963689720986142. doi: 10.1177/0963689720986142
Yang, F., Luo, P., Ding, H., Zhang, C., and Zhu, Z. (2018). Collagen type V a2 (COL5A2) is decreased in steroid-induced necrosis of the femoral head. Am. J. Transl. Res. 10, 2469–2479.
Keywords: adipose-derived stem cells, collagen V, osteoarthritis, experimental model, cartilage treatment, therapy, hyaline cartilage
Citation: Brindo da Cruz IC, Velosa APP, Carrasco S, dos Santos Filho A, Tomaz de Miranda J, Pompeu E, Fernandes TL, Bueno DF, Fanelli C, Goldenstein-Schainberg C, Fabro AT, Fuller R, Silva PL, Capelozzi VL and Teodoro WR (2021) Post-Adipose-Derived Stem Cells (ADSC) Stimulated by Collagen Type V (Col V) Mitigate the Progression of Osteoarthritic Rabbit Articular Cartilage. Front. Cell Dev. Biol. 9:606890. doi: 10.3389/fcell.2021.606890
Received: 15 September 2020; Accepted: 22 February 2021;
Published: 22 March 2021.
Edited by:
Darius Widera, University of Reading, United KingdomReviewed by:
Lucienne A. Vonk, University Medical Center Utrecht, NetherlandsCopyright © 2021 Brindo da Cruz, Velosa, Carrasco, dos Santos Filho, Tomaz de Miranda, Pompeu, Fernandes, Bueno, Fanelli, Goldenstein-Schainberg, Fabro, Fuller, Silva, Capelozzi and Teodoro. This is an open-access article distributed under the terms of the Creative Commons Attribution License (CC BY). The use, distribution or reproduction in other forums is permitted, provided the original author(s) and the copyright owner(s) are credited and that the original publication in this journal is cited, in accordance with accepted academic practice. No use, distribution or reproduction is permitted which does not comply with these terms.
*Correspondence: Walcy Rosolia Teodoro, d2FsY3kudGVvZG9yb0BmbS51c3AuYnI=
Disclaimer: All claims expressed in this article are solely those of the authors and do not necessarily represent those of their affiliated organizations, or those of the publisher, the editors and the reviewers. Any product that may be evaluated in this article or claim that may be made by its manufacturer is not guaranteed or endorsed by the publisher.
Research integrity at Frontiers
Learn more about the work of our research integrity team to safeguard the quality of each article we publish.