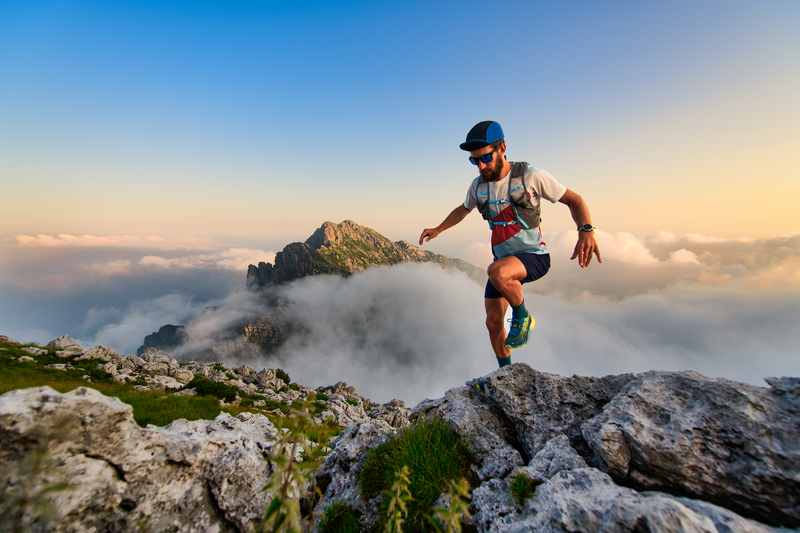
94% of researchers rate our articles as excellent or good
Learn more about the work of our research integrity team to safeguard the quality of each article we publish.
Find out more
REVIEW article
Front. Cardiovasc. Med. , 17 March 2025
Sec. Cardiovascular Metabolism
Volume 12 - 2025 | https://doi.org/10.3389/fcvm.2025.1571998
This article is part of the Research Topic Recent Advances in Mitochondria-Associated Endoplasmic Reticulum Membranes (MAMs) in Heart-Related Diseases: Volume II View all 3 articles
Dilated cardiomyopathy (DCM) is a leading cause of heart failure, yet therapeutic options remain limited. While traditional research has focused on mechanisms such as energy deficits and calcium dysregulation, increasing evidence suggests that mitochondria-associated membranes (MAMs) could provide new insights into understanding and treating DCM. In this narrative review, we summarize the key role of MAMs, crucial endoplasmic reticulum (ER)-mitochondria interfaces, in regulating cellular processes such as calcium homeostasis, lipid metabolism, and mitochondrial dynamics. Disruption of MAMs function may initiate pathological cascades, including ER stress, inflammation, and cell death. These disruptions in MAM function lead to further destabilization of cellular homeostasis. Identifying MAMs as key modulators of cardiac health may provide novel insights for early diagnosis and targeted therapies in DCM.
Dilated cardiomyopathy (DCM) is a progressive disease characterized by left ventricular enlargement and impaired contractile function, often caused by genetic mutations, viral infections, and autoimmune diseases (1). The pathophysiology of DCM includes myocardial fibrosis, cardiomyocyte hypertrophy, apoptosis, and contractile dysfunction, influenced by factors including genetic mutations [e.g., Troponin T2, Cardiac Type, Titin, Lamin A/C(LMNA), Phospholamban (PLN), Tropomyosin 1, Laminin Subunit Alpha 2], myocardial injury, ventricular remodeling, oxidative stress, and mitochondrial dysfunction, which ultimately leads to heart failure (2). Current treatments, including pharmacological therapies (e.g., angiotensin-converting enzyme inhibitors, β-blockers, andSodium-Glucose Cotransporter 2 inhibitors) and device interventions (e.g., implantable cardioverter-defibrillators, left ventricular assist devices), remain limited by their inability to reverse cardiac damage or halt disease progression, underscoring the need for novel therapeutic strategies (3).
Mitochondria and the endoplasmic reticulum (ER) are central to DCM pathogenesis, regulating energy metabolism, calcium homeostasis, and apoptosis. Mitochondria produce ATP essential for cardiac contraction, while the ER ensures calcium storage and protein folding (4, 5). In DCM, dysfunction in these organelles leads to energy deficits, calcium dysregulation, and cellular stress responses, exacerbating cardiac dysfunction (6).
According to recent studies, mitochondria-associated membranes (MAMs), physical connections of the ER and mitochondria, serve as a platform for signal transduction and inter-organelle communication (7). MAMs facilitate calcium transfer, regulate mitochondrial ATP production, and prevent calcium overload-induced apoptosis (8, 9). They are also implicated in ER stress, mitochondrial dynamics, autophagy, inflammation, and oxidative stress, all of which contribute to DCM (10–12). Despite their importance, the precise mechanisms by which MAMs influence DCM remain poorly understood, and their therapeutic potential is largely unexplored. This review aims to elucidate the structure and function of MAMs, summarize their role in DCM development, and highlight their potential as a novel therapeutic target for this complex disease.
MAMs were first reported in the late 1950s and were successfully isolated from rat liver tissue in the 1990s (13). Advances in techniques such as transmission electron microscopy have confirmed that MAMs are subcellular compartments located at the mitochondria–ER contact (MERC) sites, consisting of protein complexes within the gap between the ER and the outer mitochondrial membrane (OMM). These complexes physically link the membranes of the two organelles while maintaining a defined distance, providing a structural platform for inter-organellar communication. The number, width, and length of MAMs are regulated parameters that reflect various physiological and pathological mechanisms (14). For instance, the number of MAMs increases by 2.5-fold during early ER stress in Henrietta Lacks cell (15), and their abundance is also elevated under hypoxic conditions (16). Typically, the distance between the OMM and smooth ER is maintained at 10–25 nm, while the distance from ribosome-rich rough ER is 50–80 nm (17). This distance is dynamic and varies with cellular metabolic states; narrower gaps enhance ER-mitochondria interactions, while wider gaps weaken them (18). For example, lipid transfer often occurs within ultra-tight contacts of <10 nm (19), and apoptotic stimuli reduce the MERC distance in Rat Basophilic Leukemia 2H3 cells from 28.2 to 20 nm (18). MAMs cover varying proportions of the mitochondrial surface depending on cell type, typically ranging from 4% to 20%, and within the same cell type, their coverage varies with stress and metabolic states (20). When the liver is in the postprandial state, the average length of MERC nearly doubles, with the coverage increasing from 4% to 11%, facilitating the metabolic adaptations required for postprandial liver function (20).
To date, in-depth mass spectrometry investigations have revealed over 1,000 proteins associated with MAMs in the brain and liver (21). MAM-associated proteins are categorized into three types based on their cellular localization: (1) proteins that are only found on the MAMs; (2) proteins that are expressed in both the MAMs and other cellular compartments; and (3) proteins that accumulate on the MAMs under specific conditions (22). Resident proteins within MAMs are characterized according to their particular activities. Calcium-regulatory proteins facilitate calcium ion flow between the ER and mitochondria, which is required for mitochondrial ATP generation and cellular stress adaptation (4). Lipid transport proteins aid in the transfer of phospholipids, such as phosphatidylserine and phosphatidylethanolamine, hence promoting mitochondrial membrane integrity and bioenergetic functions (19). Structural anchoring proteins keep mitochondria and the ER in close proximity, facilitating inter-organellar communication and coordinating activities including mitochondrial fusion, fission, and autophagy (11). In addition to their structural functions, MAMs are important regulators of inflammation, oxidative stress, and cell death (23). Dysregulation of calcium homeostasis at MAMs can lead to mitochondrial calcium excess, apoptosis, and energy deficits (4). Similarly, decreased lipid transport can undermine mitochondrial membrane stability, resulting in cardiomyocyte dysfunction (24). These disturbances are directly linked to the pathogenesis of many cardiovascular illnesses, including ventricular hypertrophy, diabetic cardiomyopathy, and myocardial infarction (11). For example, increased calcium signaling at MAMs exacerbates ventricular hypertrophy (25), whereas altered lipid metabolism contributes to energy deficiencies in diabetic cardiomyopathy (26). These results demonstrate the structural and functional functions of MAMs in preserving cellular homeostasis and point to the potential therapeutic benefits of these molecules in the treatment of cardiovascular disorders.
Ca2+ are essential for excitation-contraction (EC) coupling in cardiac myocytes, where they directly contribute to energy production, contractile activation, and cell survival (27). Ca2+ enters the cell during an action potential through the activation of L-type calcium channels, which in turn initiates calcium release from the sarcoplasmic reticulum (SR) via ryanodine receptors 2 (RyR2) (4). Ca2+ is released and binds to contractile proteins, which in turn triggers muscle contraction. The sarcoplasmic/endoplasmic reticulum calcium ATPase (SERCA) either returns Ca2+ to the SR during relaxation or is discharged from the cell through the sodium-calcium exchanger (4). MAMs regulate Ca2+ transfer at the ER-mitochondria interface to ensure the precision of EC coupling, satisfy the high energy demands of cardiac myocytes, and maintain calcium homeostasis. Several essential proteins and regulatory mechanisms are involved in the transfer of Ca2+ at this interface. To begin, the ER membrane's inositol 1,4,5-trisphosphate receptors (IP3Rs) release Ca2+ in response to cellular signaling, thereby establishing a local high-calcium microdomain (28). The Ca2+ subsequently passes through voltage-dependent anion channels (VDACs) on the outer mitochondrial membrane and enters the mitochondrial matrix through the mitochondrial calcium uniporter (MCU) on the inner membrane, thereby supporting ATP production (29).
Key proteins regulating Ca2+ transfer at MAMs include the chaperone glucose-regulated protein 75 (Grp75) (Supplementary Figure S1), which bridges IP3Rs and VDACs to facilitate efficient Ca2+ transfer (28). The Sigma-1 receptor (Sig-1R), which enhances Ca2+ flux under ER stress by interacting with IP3Rs (30). Mitofusin-2 (MFN2) is another key protein that stabilizes ER-mitochondria contact sites, thereby maintaining calcium dynamics and ensuring efficient Ca2+ signaling between these organelles (31). Redox proteins such as Endoplasmic Reticulum Oxidoreductin 1 alpha (Ero1α) and Endoplasmic Reticulum Protein 44 (ERp44) modulate Ca2+ transfer by adjusting the redox state of IP3Rs (32–34), while selenoprotein N1 (SEPN1) (35) and Protein Tyrosine Phosphatase Interacting Protein 51 (PTPIP51) [interacting with ER protein Vesicle-Associated Membrane Protein-Associated Protein B (VAPB)] (36) help regulate ER-mitochondria Ca2+ transfer to prevent excessive flux. Additional proteins, such as calnexin (CNX) and Thioredoxin-related Transmembrane Protein 1, which modulate the redox state of SERCA2b, ensure proper ER Ca2+ levels, thereby supporting mitochondrial oxidative phosphorylation (37, 38). Glycogen Synthase Kinase 3 Beta (Gsk-3β), a kinase involved in mitochondrial apoptosis regulation, can specifically interact with and regulate the protein composition of the IP3R Ca2+ channeling complex (39). FUN14 Domain Containing 1 (FUNDC1), an autophagy receptor at MAMs, interacts with IP3Rs and other calcium-related proteins to maintain calcium homeostasis, protecting cardiac myocytes from ischemic damage (40). Cyclophilin D, a mitochondrial chaperone protein, interacts with the VDAC1/Grp75/IP3R1 complex to regulate Ca2+ transfer and mitochondrial dysfunction under hypoxia-reoxygenation stress (41).
In conclusion, these proteins form a highly integrated network at MAMs that ensures efficient Ca2+ transfer and signaling. By coordinating calcium signaling, MAMs support mitochondrial energy production to meet the workload demands of cardiac myocytes.
Some major proteins found in MAMs regulate the metabolism of phospholipids, cholesterol, and sphingolipids, all of which are essential for mitochondrial membrane integrity and cell function. Among the lipids generated at MAMs, phospholipids such as phosphatidylserine (PS) and phosphatidylethanolamine (PE) are necessary for mitochondrial membrane dynamics, whereas phosphatidylcholine (PC) is an important structural component of the cellular membrane (42, 43). Neutral lipids, such as triacylglycerol and cholesteryl esters, are critical for energy storage, membrane fluidity, and resistance to lipotoxicity and oxidative stress (44). The synthesis of these lipids is facilitated by various enzymes found in MAMs (Supplementary Figure S1).
Phosphatidylserine (PS) is produced by phosphatidylserine synthases (PSS1 and PSS2) on MAMs, transported to mitochondria via the Oxysterol-Binding Protein-Related Protein 5/8 (ORP5/8)-PTPIP51 complex, and converted to phosphatidylethanolamine (PE) by phosphatidylserine decarboxylase (45–47). PE maintains mitochondrial membrane curvature and function (48). Furthermore, phosphatidylethanolamine N-methyltransferase 2 can methylate PE to produce PC, thus completing a critical lipid production cycle (49). Diacylglycerol O-Acyltransferase 2 (DGAT2) catalyzes the formation of neutral lipids such as triglycerides (50). Acetyl-CoA cholesterol acyltransferase 1 (ACAT1) converts cholesterol into cholesteryl esters, hence regulating cholesterol levels (51). Fatty acyl-CoA ligase 4 (FACL4) converts free fatty acids into complex lipids needed for membrane formation and energy storage (52). The interaction of the Steroidogenic Acute Regulatory Protein (StAR) and Voltage-Dependent Anion Channel 2 (VDAC2) at MAMs promotes cholesterol transport into mitochondria, regulating the rate of steroid hormone synthesis and, as a result, cellular lipid balance and function (53).
Lipid transport between the ER and mitochondria is critical for cellular homeostasis and is mediated by proteins such as ATPase family AAA domain-containing protein 3 (ATAD3), which improves structural stability at the ER -mitochondria interface, promotes lipid transport, and ensures the efficient bidirectional exchange required for membrane and energy homeostasis (54). Caveolin-1 has a structural role in MAMs and can regulate membrane curvature and lipid signaling (55). Dysregulation of lipid metabolism at MAMs has a significant impact on cardiovascular pathology. Impaired phospholipid metabolism alters the structure of the mitochondrial membrane, reduces the efficiency of oxidative phosphorylation, and increases susceptibility to oxidative stress, all of which are associated with the onset of cardiovascular diseases (CVDs) (56). Abnormal cholesterol metabolism via ACAT1 causes lipid buildup, which leads to atherosclerosis and endothelial dysfunction (57). Similarly, DGAT2-mediated triglyceride production dysregulation may enhance cardiac steatosis and lipotoxic cardiomyocyte death (58). Dysfunctional lipid transport systems, such as decreased PS transfer via ORP5 or disturbed ER-mitochondrion connections via ATAD3, can affect calcium homeostasis, activate inflammasomes, and cause chronic inflammation. These mechanisms together hasten the progression of CVDs, such as atherosclerosis, diabetic cardiomyopathy, and heart failure (59).
Mitochondrial dynamics is the ongoing cycle of mitochondrial fusion and fission (60). Under physiological conditions, these processes enable mitochondria to constantly change their morphology, shape, and quantity in response to numerous cellular stimuli (61). The dynamic balance between fusion and fission is crucial for maintaining mitochondrial function, cellular health, and energy metabolism, especially in the heart, which has extremely high energy requirements (62).
MAMs play an important role in mitochondrial fission via proteins such as Dynamin-related protein 1 (Drp1), Fission 1 (Fis1), Mitochondrial Fission Factor (Mff), and FUNDC1 (Supplementary Figure S1). Mitochondrial fission begins with the recruitment of Drp1, a major Guanosine Triphosphatase (GTPase), to OMM (63). Several important proteins located at the MAMs, such as mitochondria Fis1, Mff, and mitochondrial dynamics proteins MiD49 and MiD5170, need to work in concert to facilitate this recruitment (64). These proteins cooperate to localize Drp1 at the ER-mitochondria interface, where it oligomerizes and activates its GTPase activity, causing mitochondrial membrane constriction and ultimately resulting in fission. Inverted formin 2 (INF2) is crucial for actin polymerization at MAMs, promoting actin-dependent mitochondrial constriction, Drp1 recruitment, and the increase in mitochondrial calcium levels required for division (65). Ras-related protein Rab-32 promotes Drp1 localization and the assembly of fission machinery at mitochondria (65). Under hypoxic conditions, the OMM protein FUNDC1 accumulates at MAMs and acts as a new Drp1 receptor, binding to the ER-localized protein CNX and facilitating fission (16).
Mitochondrial fusion enables the exchange of contents by integrating the outer and inner membranes, maintaining mitochondrial integrity and function (66). Fusion starts with MFN2 anchoring and merging the outer membrane, followed by Optic Atrophy Protein 1 (OPA1) regulating the inner membrane fusion (67). These stages help to exchange mitochondrial DNA, proteins, and metabolites, which is critical for maintaining mitochondrial integrity and adjusting to cellular energy demands (66). Interestingly, FUNDC1 also appears to play a role in regulating mitochondrial fusion. In response to low oxygen conditions (hypoxia), FUNDC1 facilitates mitochondrial fusion by preventing excessive fission, thus helping cells adapt to metabolic stress (68). MFN2 is found in MAMs, where it tethers mitochondria to the ER and other surrounding organelles by interacting with Mitofusin 1 (MFN1) or another MFN2 and promoting outer membrane fusion (69). OPA1 is located in the mitochondrial inner membrane, where it regulates inner membrane fusion and organizes cristae structures, which are essential for efficient ATP generation and stress response (70). Furthermore, the Endoplasmic Reticulum-Associated Degradation indirectly promotes mitochondrial fusion by removing faulty fusion proteins, hence maintaining mitochondrial dynamics in balance (30). The relationship between fusion and fission emphasizes the importance of MAMs and their associated proteins in mitochondrial dynamics, which is strongly linked to cellular health and disease progression.
Given the crucial role of MAMs in regulating various cellular processes, it is essential to explore their contribution to the development of DCM. In this review, we aim to summarize the key role of MAMs in regulating biological processes such as calcium overload, mitochondrial homeostasis, inflammation, ER stress, apoptosis, autophagy, necrosis, and ferroptosis within the context of DCM pathology. Additionally, we will identify and discuss specific MAM-related proteins involved in these processes (Supplementary Figure S2).
Mutations in the RNA Binding Motif Protein 20 gene lead to calcium overload in myocardial cells, resulting in elevated levels of Ca2+ in the SR (71). The calcium channel blocker verapamil has been shown to effectively alleviate arrhythmias in DCM patients (71), supporting the critical role of calcium dysregulation in the pathogenesis of DCM. Abnormal calcium signaling at MAMs can affect myocardial contractility and relaxation, promoting myocardial fibrosis and cardiac remodeling, which are central factors in the progression of DCM (72). Several key proteins located at MAMs undergo changes during the development of DCM.GSK-3β protein, present at MAMs, interacts with the IP3R complex to regulate calcium exchange (73) (Supplementary Figure S2). Studies have shown that Coxsackievirus B3 infection activates GSK-3β, inducing myocardial injury and apoptosis, leading to DCM (74).
Additionally, calcium/calmodulin-dependent protein kinase II (CaMKII) regulates calcium transfer at MAMs through phosphorylation of the RYR2, and its overactivation is closely associated with DCM (Supplementary Figure S2). Elizabeth et al. (75) reported that CaMKII overexpression in mice leads to severe ATP depletion and SR calcium leakage in myocardial cells, resulting in advanced DCM. LMNA gene mutations cause LMNA-DCM, accounting for 4%–8% of all DCM cases (76). Research by Hang et al. (77) showed that iPSC-derived cardiomyocytes from LMNA-DCM patients exhibit abnormal calcium handling and elevated oxidative stress (ROS). ROS activation of the CaMKII-RyR2 pathway further exacerbates arrhythmias and nuclear membrane deformation (78). Dickkopf 3 ameliorates the development of familial dilated cardiomyopathy by downregulating CaMKII (78).
Another important regulator in calcium cycling is PLN, which controls calcium reuptake by regulating SERCA2, thereby affecting cardiac contraction and relaxation (Supplementary Figure S2). PLN mutations or dysfunction decrease SERCA2 activity, disrupting calcium cycling and accelerating DCM progression (79, 80). After LAVDs support, the significant dysregulation of SERCA2 in DCM patients was restored, further emphasizing the importance of SERCA2 in DCM pathophysiology (81). In Duchenne muscular dystrophy (DMD), mutations in the dystrophin gene not only affect skeletal muscle but also impact cardiac muscle, leading to cardiac dilation and eventual failure, progressing to DCM (82). Studies (83) have shown that in DMD mouse models, early cardiac damage is associated with elevated expression of IP3R1 and its regulatory subunit Sig-1R, enhancing the IP3R1-GRP75-VDAC-MCU complex and increasing mitochondrial calcium content. Metformin may help reverse these changes (84). In patients with DCM and doxorubicin (DOX)-treated mice, a downregulation of FUNDC1 was observed in cardiac tissues. This deficiency exacerbated DOX-induced cardiac damage and heart dysfunction in mice (85). The regulation of Ca2+ homeostasis mediated by MAMs plays a crucial role in maintaining normal myocardial function and cardiomyocyte activity.
Mitochondrial fusion and fission are key mechanisms in maintaining the dynamic balance of mitochondrial morphology and function and are crucial for cardiac homeostasis and normal cardiac remodeling processes. A large number of very small and fragmented mitochondria have been found in the myocardial tissues of end-stage DCM patients, which are associated with the loss of mitochondrial fusion/fission balance (86). Recent studies have suggested that mutations in fission genes may be a cause of DCM. Immediate postnatal gene ablation of Drp1 in mice is lethal (87). In adult Drp1 knockout mice, myocardial mitochondria become enlarged and elongated, with reduced volume. These mice develop replacement fibrosis, increased mitophagy, calcium uptake, superoxide production, and permeability transition pore opening, leading to cardiomyocyte apoptosis. They display typical DCM features, including ventricular wall thinning, dilation, and an increased r/h ratio (left ventricular end-diastolic diameter to wall thickness) (87–89). In models with impaired Drp1 function, upregulation of the fusion factors MFN2 and OPA1 provide some compensatory effects against mitochondrial damage (87). Moreover, in Mff gene-deficient mice, mitochondrial fission capability is significantly reduced, resulting in excessive mitochondrial fusion and network formation, ultimately leading to premature death due to severe DCM (90). However, researchers have found that regulating the expression of fusion-related proteins (such as MFN1 or OPA1) can improve this pathological damage (90). These findings suggest that the balance between mitochondrial fusion and fission is crucial for cardiomyocytes and provides new therapeutic perspectives. In the treatment of DCM caused by defects in fission genes, therapy may not only target fission, with drugs like LCZ696 (a novel angiotensin receptor neprilysin inhibitor) or Drp1-specific inhibitors like Midivi-1 (91), but also employ drugs that modulate fusion proteins (e.g., MFN2 agonists).
Of course, the lack of MFN1 and MFN2 genes is lethal in mouse embryos, while adult mice show mitochondrial fragmentation and respiratory chain dysfunction, leading to fatal DCM (92). A study of endomyocardial biopsies from the interventricular septum of 22 idiopathic dilated cardiomyopathy (IDCM) patients revealed that MFN1 expression was significantly reduced in the myocardium of treatment-resistant IDCM patients. Restoring MFN1 function or increasing its expression may be beneficial for treatment-resistant IDCM patients (93). In addition, abnormal processing of the fusion protein OPA1 leads to excessive mitochondrial fission and fragmentation, ultimately causing ventricular dilation and heart failure. Regulation of key proteases that affect OPA1 processing (such as Opa1 mitochondrial dynamin-like GTPase-1 and YME1 like 1) can significantly improve mitochondrial morphology and function in cardiomyocytes (94). In conclusion, changes in mitochondrial fusion and fission mechanisms in the heart promote mitochondrial metabolic damage, thereby triggeringDCM. The associated proteins are primarily located on MAMs (Supplementary Figure S2), especially MFN2, playing an important regulatory role in the distance between the ER and mitochondria.
Cardiac injury caused by genetic, immune, or infectious factors triggers inflammation and activates immune repair processes. However, sustained or excessive inflammatory responses can lead to fibrosis, oxidative stress, and mitochondrial dysfunction, which are major driving forces in the onset and progression of DCM (2). The NOD-like receptor protein 3 (NLRP3) inflammasome is currently the only inflammasome known to be localized on MAMs (95) (Supplementary Figure S2). On the one hand, its components, including NLRP3, Apoptosis-associated speck-like protein containing a CARD (ASC), and Caspase-1, aggregate and assemble at MAMs (96). On the other hand, MAMs dysfunction-induced calcium overload, oxidative stress, mitochondrial dysfunction (such as the release of ROS, mitochondrial DNA, cardiolipin, mitochondrial-associated antiviral signaling, and thioredoxin-interacting protein) can activate the NLRP3 inflammasome, amplifying inflammatory signaling and triggering downstream effects (97, 98).
Cheng et al. (99) conducted a study on 18 human cardiac tissue samples and found that, compared to patients without a history of heart disease, DCM patients exhibited ASC speck formation in cardiomyocytes, along with elevated protein expression of caspase-1 and the pro-inflammatory cytokines IL-1β and IL-18. Plasma levels of IL-1β and IL-18 were also significantly higher in DCM patients, indicating that the NLRP3 inflammasome is activated in DCM and is likely involved in promoting cardiac dysfunction (100, 101). Following its assembly, the NLRP3 inflammasome catalyzes the production of caspase-1, which cleaves Gasdermin D (GSDMD) to form its active N-terminal functional domain (GSDMD-NT) (102). This process facilitates pore formation in the cell membrane, ultimately inducing pyroptosis. In myocardial tissues from nine DCM patients, GSDMD-NT levels were significantly elevated (99).
Interestingly, the researchers also performed triple immunostaining for active caspase-1, TdT-mediated dUTP Nick-End Labeling, and α-actin on cardiac specimens and observed that pyroptotic cardiomyocytes were markedly more abundant than apoptotic ones in DCM. These findings suggest that NLRP3-mediated pyroptosis may play a critical role in the progression of DCM (99). Furthermore, dapagliflozin has been shown to inhibit the activation of the NLRP3 inflammasome by suppressing p38-dependent Toll-like receptor 4 expression and reducing ROS production, highlighting its potential therapeutic value in treating DCM (103). Additionally, the NLRP3 inflammasome has been found to induce myocardial fibrosis and impair myocardial contractility; however, its specific role in DCM remains to be elucidated (104, 105).
Protein mutations, oxidative stress, ROS production, and aberrant intracellular Ca2+ handling can all increase the demand for protein synthesis, disrupting the ER homeostasis and leading to the accumulation of misfolded proteins within the ER lumen, thereby triggering ER stress (ERS) (106). In response, the cell activates three key sensors of the unfolded protein response (UPR): PKR-like ER kinase (PERK), activating transcription factor 6 (ATF6), and inositol-requiring enzyme 1α (IRE1α) (107). These sensors dissociate from the chaperone protein Binding Immunoglobulin Protein [Bip, also known as Glucose-Regulated Protein 78 (GRP78)] and initiate different signaling pathways to restore ER homeostasis and prevent cell death (107). Proteins such as PERK, IRE1α, and Bip are highly enriched in MAMs (108, 109) (Supplementary Figure S2), which also regulate ERS signaling. For instance, VAPB directly suppresses UPR by interacting with ATF6 (110). Dysfunction of MAMs can exacerbate or induce ERS.
Several studies have shown that in patients with DCM, levels of UPR markers—such as BiP, ATF6, phosphorylated eukaryotic initiation factor 2α (P-eIF2α), and X-box Binding Protein 1—are significantly elevated, indicating that ERS and UPR pathways are activated in the myocardium of DCM patients (81, 111). UPR can serve as a compensatory mechanism, protecting myocardial cells in the short term. For example, PERK activation leads to the phosphorylation of eIF2α, temporarily halting protein synthesis to restore ER balance and alleviate ER stress (112, 113). Furthermore, PERK maintains the expression of SERCA2a, regulating calcium homeostasis in cardiomyocytes, preventing ER stress, and offering protection in heart failure (114–116). However, prolonged activation of the UPR (referred to as maladaptive UPR) can impair cellular homeostasis, triggering autophagy or apoptosis, and further exacerbating cardiac structural and functional damage (117–119). As an important site for calcium signaling and ROS regulation, MAMs play a significant role in amplifying the ER stress response. Under excessive ER stress, IRE1α interacts with the adaptor proteins TNF receptor-associated factor 2 and apoptosis signal-regulating kinase 1 to activate downstream apoptotic signaling (120). Additionally, IRE1α regulates IP3Rs, inducing calcium dysregulation and further promoting apoptosis (121). The accumulation of PERK in MAMs not only activates protective signaling via the eIF2α activating transcription factor 4 (ATF4) pathway but also promotes the expression of pro-apoptotic factors such as C/EBP homologous protein (CHOP), exacerbating cardiomyocyte death under prolonged stress (122). Compounds such as Ferulic Acid, Pterostilbene, and Tyrosol have been shown to alleviate ER stress-induced cardiac injury, likely through their modulation of MAMs. These compounds may reduce the activation of the PERK/eIF2α/ATF4/CHOP pathway, thereby decreasing cardiomyocyte apoptosis and protecting the heart from ER stress-mediated damage. This highlights the therapeutic potential of targeting MAMs in combating ER stress-induced cardiac injury (123).
The release of cytochrome c (Cytc) from mitochondria and the activation of caspase-3 in the myocardial tissue of end-stage DCM patients provide molecular evidence for the involvement of apoptosis in the development of DCM (124, 125). MAMs typically regulate apoptosis by modulating intracellular oxidative stress, mitochondrial function, Ca2+ concentration, and ER stress. Dysregulated expression of Ero1α and SHC Adaptor Protein p66 (p66Shc) on MAMs can directly promote the generation of ROS (Supplementary Figure S2), leading to oxidative stress in cardiomyocytes and triggering cardiomyocyte apoptosis (126, 127). In DCM, factors such as DNA damage and myocardial mechanical stretch increase the expression of Tumor Protein p53 (p53), which accumulates in MAMs and promotes apoptosis through multiple molecular mechanisms (128–130). p53 directly activates the pro-apoptotic gene Bcl-2-associated X protein (Bax), leading to Bax translocation to the outer mitochondrial membrane, where it interacts with the anti-apoptotic protein B-cell lymphoma 2 (Bcl-2), which also relocalizes to MAMs (131, 132). This interaction disrupts the mitochondrial outer membrane integrity, facilitating Cytc release from the mitochondria. Cytc then activates caspase 9, which further triggers downstream caspase activation (caspase 3 and caspase 7), leading to the cleavage of critical cellular proteins and ultimately leading to cardiomyocyte apoptosis (129, 130, 133, 134). Interestingly, although the anti-apoptotic factor Bcl-2 levels increase in DCM, this increase is insufficient to counterbalance the pro-apoptotic signals, suggesting a compensatory mechanism that fails under prolonged stress (133, 135). Moreover, studies have shown that under stress conditions, p53 can also bind to SERCA2, leading to mitochondrial Ca2+ overload and apoptosis (128). However, when the activity of cardiac SERCA2 ATPase is reduced, p53 takes on a protective role (136). The modulation of these key pathways, including the Bcl-2 family, Bax, and the caspase cascade, within MAMs underscores the critical role of MAM dysfunction in exacerbating apoptosis in DCM. The mitochondrial fusion protein OPA1 on MAMs can directly participate in cytochrome c release by affecting the opening of crista junctions, located at the junction of mitochondrial cristae and the boundary membrane, thus regulating apoptosis in the progression of DCM (137, 138). Protein Kinase B (PKB, also known as Akt) on MAMs can phosphorylate IP3R3, reducing the sensitivity of cells to Ca2+-dependent apoptosis (139, 140). The functional loss of its upstream regulator, mechanistic Target of Rapamycin Complex 2 (mTORC2), decreases Akt activity and accelerates cardiomyocyte apoptosis (141, 142). mTORC2 is also localized to MAMs (139). These studies suggest that MAMs play a critical role in cardiomyocyte apoptosis during the progression of DCM.
Autophagy is a key cellular mechanism that helps cells respond to stress by degrading damaged cellular structures, particularly under conditions of ER stress and excessive ROS production, thereby aiding in the restoration of cellular homeostasis; however, excessive autophagy can also lead to cardiomyocyte injury and death (143–145). Although numerous studies have identified abnormal autophagy in DCM, with abundant autophagic vacuoles (autophagosomes and autolysosomes) and lysosomes in myocardial cells, the general conclusion suggests that autophagy plays a protective role in cardiomyocytes, reducing myocardial degeneration and reversing ventricular remodeling (146–148). Some studies indicate that many key proteins directly involved in autophagy, such as Parkin E3 ubiquitin ligase (Parkin), Beclin 1 (BECN1), and AMP-activated protein kinase (AMPK), are localized to MAMs, where autophagosomes are formed (149–151) (Supplementary Figure S2).
Parkin deficiency affects the ubiquitination of MFN2, disrupting the integrity of MAMs, inhibiting autophagy, and leading to mitochondrial dysfunction, which in turn triggers severe DCM (152, 153). Bcl-2 binds to BECN1 to suppress autophagy, but during cellular stress, BECN1 dissociates from Bcl-2, promoting autophagy (154). Researchers have observed that activation of Macrophage Stimulating 1 in DCM strengthens the binding of Bcl-2 to BECN1, thereby inhibiting autophagy and promoting apoptosis (155). AMPK, as an energy sensor, regulates the dynamic stability of MAMs in cardiomyocytes by phosphorylating MFN2 and activating autophagy under energy stress conditions (156). MAMs also influence autophagy through the regulation of mitochondrial function, calcium ion transport, and lipid synthesis (149). Drp1 promotes autophagy by regulating mitochondrial fission, and its dysfunction (such as resistance to oligomer disassembly) leads to mitochondrial autophagy impairment, reduced calcium uptake, and compromised ATP synthesis, thereby triggering DCM (157). PE, a marker of autophagosomal membrane expansion, is a major receptor for Autophagy-Related Gene 8 (ATG8), and in DCM studies, ATG8 is often used as a marker of autophagic activity (158). However, how MAMs regulate the relationship between PE and ATG8 to influence autophagy requires further exploration. Ca2+ promote autophagy by inhibiting the mechanistic target of the rapamycin (mTOR) pathway via AMPK and CaMKII (159–161). However, excessively high calcium concentrations can activate calpains and calcineurin, which negatively regulate autophagy, potentially impairing myocardial function and contributing to the development of DCM (159–161). Tacrolimus and other rapamycin analogs can restore autophagy by blocking mechanistic Target of Rapamycin Complex 1 activity, preventing the progression of LMNA mutation-induced cardiomyopathy (162). In summary, MAMs regulate autophagy through multiple pathways, including mitochondrial fusion and fission, lipid metabolism, and calcium homeostasis.
During the transition from pressure-overload hypertrophy to DCM, the mitochondria-targeted pro-apoptotic Bcl-2 family protein Nix (Nip3-like protein X) induces cardiomyocyte apoptosis by promoting the oligomerization of Bax and Bcl-2 homologous antagonist/killer (163). On the other hand, endoplasmic/sarcoplasmic reticulum (ER/SR)-targeted Nix increases ER/SR calcium storage and triggers mitochondrial permeability transition pore (MPTP) opening in cardiomyocytes via MAMs. This leads to ATP depletion, mitochondrial swelling, OMM rupture, and Cytc release, resulting in a form of programmed cell death distinct from apoptosis, known as necroptosis (164–166) (Supplementary Figure S2). The cardiotoxicity of the anti-cancer drug sorafenib may cause DCM, with its mechanism potentially linked to increased MAMs formation, reduced distance between MAMs and mitochondria, and consequent Ca2+ overload in cardiomyocytes (167). This overload activates CaMKII and the Receptor Interacting Protein Kinase 3 Mixed Lineage Kinase Domain-Like Protein cascade, inducing necroptosis. Overexpression of MFN2 shows promise in mitigating sorafenib-induced cardiac dysfunction (167).
Ferroptosis is a novel iron-dependent form of cell death, whose mechanism involves the generation of free radicals via the Fenton reaction, lipid peroxidation, and the inactivation of intracellular antioxidant systems, such as the depletion of glutathione (GSH) and glutathione peroxidase 4 (GPX4) (168). DOX, gene knockout of ferritin H, and iron deposition induced by various factors can directly trigger ferroptosis in cardiomyocytes, leading to cardiac dysfunction and heart injury (169–171). This suggests that ferroptosis is an important pathological factor in cardiomyopathy. However, evidence regarding the role of ferroptosis in DCM remains limited, with much of the research still in the bioinformatics phase. Studies have shown that disruptions in Ca2+ homeostasis at the MAMs, accumulation of unsaturated fatty acids, and the buildup of ROS can all induce ferroptosis (172–174). Heme Oxygenase 1 enrichment at MAMs plays a critical role in the pathogenesis of DCM by regulating iron homeostasis (175). In a DOX-induced mouse model, overactivation of the Nrf2-HMOX1 axis leads to HMOX1-mediated heme degradation and the release of free iron (Fe2+), which accumulates in mitochondria (169). This iron overload generates ROS through the Fenton reaction, triggering oxidative stress, lipid peroxidation, and ferroptosis, thereby exacerbating myocardial injury and dysfunction. Inhibition of HMOX1 activity by zinc protoporphyrin IX reduces free iron release, significantly alleviating myocardial damage and dysfunction (169). Targeting HMOX1 or MAMs function may provide new therapeutic strategies for DCM (Supplementary Figure S2). In DOX-induced cardiomyopathy, the FUN14 domain containing 2 (FUNDC2) protein at the OMM regulates mitochondrial GSH levels by influencing the stability of the mitochondrial glutathione transporter Solute Carrier Family 25 Member 11 (SLC25A11) and GPX4, thereby promoting ferroptosis (176). Wang et al. (177) through microarray data analysis, identified the signal transducer and activator of transcription 3 (STAT3) as a gene associated with ferroptosis in DCM, and the transcription factor STAT3 encoded by this gene is located in the MAMs (178).
This article delves into the critical role of MAMs in the pathogenesis and progression of DCM. MAMs play an essential role in maintaining cardiac cell function by precisely regulating intracellular calcium homeostasis, lipid metabolism, and mitochondrial dynamics. Dysregulation of MAMs leads to calcium overload, mitochondrial dysfunction, and activation of cell death pathways such as autophagy, apoptosis, necroptosis, ferroptosis, and necrosis, all of which are closely associated with the clinical and pathological features of DCM (Supplementary Table S1). These findings highlight MAMs as a key node in cellular dysfunction and pathological transitions. Modulating the function of MAMs may not only provide early biomarkers for cardiac diseases but also offer new perspectives and potential targets for therapeutic interventions and the development of novel treatment strategies for DCM. However, most experimental studies on MAMs have focused on cardiomyocytes, with relatively fewer studies on fibroblasts and endothelial cells. Further research is needed to explore whether MAMs in different types of cardiac cells have distinct effects on the onset and progression of DCM. Additionally, as indicated by previous analyses, the protein expression profile of MAMs in DCM caused by different genetic mutations often varies, and the roles of some proteins exhibit a dual nature. Therefore, finding a balance between the protective effects and potential risks of MAMs is crucial. It is particularly important to develop more precise intervention strategies tailored to different genetic types of DCM. With the ongoing in-depth investigation of MAMs as a therapeutic target, we anticipate that this will lead to groundbreaking advances in the clinical treatment of DCM, improve patient outcomes, and provide new strategies for comprehensive cardiac disease management.
PH: Visualization, Writing – original draft, Writing – review & editing, Project administration. HC: Writing – review & editing, Project administration, Writing – original draft. YQ: Writing – review & editing. ZW: Funding acquisition, Project administration, Writing – review & editing.
The author(s) declare that financial support was received for the research and/or publication of this article. This study was supported by the National Natural Fund (No. 81573920 and 82205082) and the Henan Provincial Science and Technology Research and Development Joint Fund Project (No. 232301420066).
We want to convey our great appreciation to our colleagues who contributed with their constructive work in this rapidly expanding field.
The authors declare that the research was conducted in the absence of any commercial or financial relationships that could be construed as a potential conflict of interest.
The author(s) declare that no Generative AI was used in the creation of this manuscript.
All claims expressed in this article are solely those of the authors and do not necessarily represent those of their affiliated organizations, or those of the publisher, the editors and the reviewers. Any product that may be evaluated in this article, or claim that may be made by its manufacturer, is not guaranteed or endorsed by the publisher.
The Supplementary Material for this article can be found online at: https://www.frontiersin.org/articles/10.3389/fcvm.2025.1571998/full#supplementary-material
1. Elliott P, Andersson B, Arbustini E, Bilinska Z, Cecchi F, Charron P, et al. Classification of the cardiomyopathies: a position statement from the European society of cardiology working group on myocardial and pericardial diseases. Eur Heart J. (2008) 29:270–6. doi: 10.1093/eurheartj/ehm342
2. Schultheiss H-P, Fairweather D, Caforio ALP, Escher F, Hershberger RE, Lipshultz SE, et al. Dilated cardiomyopathy. Nat Rev Dis Primer. (2019) 5:32. doi: 10.1038/s41572-019-0084-1
3. Heymans S, Lakdawala NK, Tschöpe C, Klingel K. Dilated cardiomyopathy: causes, mechanisms, and current and future treatment approaches. Lancet. (2023) 402:998–1011. doi: 10.1016/S0140-6736(23)01241-2
4. Kohlhaas M, Maack C. Calcium release microdomains and mitochondria. Cardiovasc Res. (2013) 98:259–68. doi: 10.1093/cvr/cvt032
5. Minamino T, Komuro I, Kitakaze M. Endoplasmic reticulum stress as a therapeutic target in cardiovascular disease. Circ Res. (2010) 107:1071–82. doi: 10.1161/CIRCRESAHA.110.227819
6. Ramaccini D, Montoya-Uribe V, Aan FJ, Modesti L, Potes Y, Wieckowski MR, et al. Mitochondrial function and dysfunction in dilated cardiomyopathy. Front Cell Dev Biol. (2021) 8:624216. doi: 10.3389/fcell.2020.624216
7. Vance JE, Stone SJ, Faust JR. Abnormalities in mitochondria-associated membranes and phospholipid biosynthetic enzymes in the mnd/mnd mouse model of neuronal ceroid lipofuscinosis. Biochim Biophys Acta. (1997) 1344:286–99. doi: 10.1016/S0005-2760(96)00153-1
8. Cárdenas C, Miller RA, Smith I, Bui T, Molgó J, Müller M, et al. Essential regulation of cell bioenergetics by constitutive InsP3 receptor Ca2+ transfer to mitochondria. Cell. (2010) 142:270. doi: 10.1016/j.cell.2010.06.007
9. Park SJ, Lee SB, Suh Y, Kim S-J, Lee N, Hong J-H, et al. DISC1 modulates neuronal stress responses by gate-keeping ER-mitochondria Ca2+ transfer through the MAM. Cell Rep. (2017) 21:2748–59. doi: 10.1016/j.celrep.2017.11.043
10. Li YE, Sowers JR, Hetz C, Ren J. Cell death regulation by MAMs: from molecular mechanisms to therapeutic implications in cardiovascular diseases. Cell Death Dis. (2022) 13:504. doi: 10.1038/s41419-022-04942-2
11. Luan Y, Luan Y, Yuan R-X, Feng Q, Chen X, Yang Y. Structure and function of mitochondria-associated endoplasmic reticulum membranes (MAMs) and their role in cardiovascular diseases. Oxid Med Cell Longev. (2021) 2021:4578809. doi: 10.1155/2021/4578809
12. Lu B, Chen X, Ma Y, Gui M, Yao L, Li J, et al. So close, yet so far away: the relationship between MAM and cardiac disease. Front Cardiovasc Med. (2024) 11:1353533. doi: 10.3389/fcvm.2024.1353533
13. Vance JE. Phospholipid synthesis in a membrane fraction associated with mitochondria. J Biol Chem. (1990) 265:7248–56. doi: 10.1016/S0021-9258(19)39106-9
14. Çoku J, Booth DM, Skoda J, Pedrotty MC, Vogel J, Liu K, et al. Reduced ER–mitochondria connectivity promotes neuroblastoma multidrug resistance. EMBO J. (2022) 41:e108272. doi: 10.15252/embj.2021108272
15. Bravo R, Vicencio JM, Parra V, Troncoso R, Munoz JP, Bui M, et al. Increased ER-mitochondrial coupling promotes mitochondrial respiration and bioenergetics during early phases of ER stress. J Cell Sci. (2011) 124:2143–52. doi: 10.1242/jcs.080762
16. Wu W, Lin C, Wu K, Jiang L, Wang X, Li W, et al. FUNDC1 regulates mitochondrial dynamics at the ER-mitochondrial contact site under hypoxic conditions. EMBO J. (2016) 35:1368–84. doi: 10.15252/embj.201593102
17. Giacomello M, Pellegrini L. The coming of age of the mitochondria–ER contact: a matter of thickness. Cell Death Differ. (2016) 23:1417–27. doi: 10.1038/cdd.2016.52
18. Csordás G, Renken C, Várnai P, Walter L, Weaver D, Buttle KF, et al. Structural and functional features and significance of the physical linkage between ER and mitochondria. J Cell Biol. (2006) 174:915–21. doi: 10.1083/jcb.200604016
19. Petrungaro C, Kornmann B. Lipid exchange at ER-mitochondria contact sites: a puzzle falling into place with quite a few pieces missing. Curr Opin Cell Biol. (2019) 57:71–6. doi: 10.1016/j.ceb.2018.11.005
20. Sood A, Jeyaraju DV, Prudent J, Caron A, Lemieux P, McBride HM, et al. A mitofusin-2–dependent inactivating cleavage of Opa1 links changes in mitochondria cristae and ER contacts in the postprandial liver. Proc Natl Acad Sci U S A. (2014) 111:16017–22. doi: 10.1073/pnas.1408061111
21. Poston CN, Krishnan SC, Bazemore-Walker CR. In-depth proteomic analysis of mammalian mitochondria-associated membranes (MAM). J Proteomics. (2013) 79:219–30. doi: 10.1016/j.jprot.2012.12.018
22. Cheng H, Gang X, He G, Liu Y, Wang Y, Zhao X, et al. The molecular mechanisms underlying mitochondria-associated endoplasmic reticulum membrane-induced insulin resistance. Front Endocrinol. (2020) 11:592129. doi: 10.3389/fendo.2020.592129
23. Liu Y, Huo J-L, Ren K, Pan S, Liu H, Zheng Y, et al. Mitochondria-associated endoplasmic reticulum membrane (MAM): a dark horse for diabetic cardiomyopathy treatment. Cell Death Discov. (2024) 10:148. doi: 10.1038/s41420-024-01918-3
24. Da Dalt L, Cabodevilla AG, Goldberg IJ, Norata GD. Cardiac lipid metabolism, mitochondrial function, and heart failure. Cardiovasc Res. (2023) 119:1905–14. doi: 10.1093/cvr/cvad100
25. Luan Y, Jin Y, Zhang P, Li H, Yang Y. Mitochondria-associated endoplasmic reticulum membranes and cardiac hypertrophy: molecular mechanisms and therapeutic targets. Front Cardiovasc Med. (2022) 9:1015722. doi: 10.3389/fcvm.2022.1015722
26. Zeng Y, Li Y, Jiang W, Hou N. Molecular mechanisms of metabolic dysregulation in diabetic cardiomyopathy. Front Cardiovasc Med. (2024) 11:1375400. doi: 10.3389/fcvm.2024.1375400
27. Bers DM. Calcium cycling and signaling in cardiac myocytes. Annu Rev Physiol. (2008) 70:23–49. doi: 10.1146/annurev.physiol.70.113006.100455
28. Szabadkai G, Bianchi K, Várnai P, De Stefani D, Wieckowski MR, Cavagna D, et al. Chaperone-mediated coupling of endoplasmic reticulum and mitochondrial Ca2+ channels. J Cell Biol. (2006) 175:901–11. doi: 10.1083/jcb.200608073
29. Rapizzi E, Pinton P, Szabadkai G, Wieckowski MR, Vandecasteele G, Baird G, et al. Recombinant expression of the voltage-dependent anion channel enhances the transfer of Ca2+ microdomains to mitochondria. J Cell Biol. (2002) 159:613–24. doi: 10.1083/jcb.200205091
30. Zhou Z, Torres M, Sha H, Halbrook CJ, Van den Bergh F, Reinert RB, et al. Endoplasmic reticulum-associated degradation regulates mitochondrial dynamics in brown adipocytes. Science. (2020) 368:54–60. doi: 10.1126/science.aay2494
31. Filadi R, Greotti E, Turacchio G, Luini A, Pozzan T, Pizzo P. Mitofusin 2 ablation increases endoplasmic reticulum-mitochondria coupling. Proc Natl Acad Sci U S A. (2015) 112:E2174–81. doi: 10.1073/pnas.1504880112
32. Gilady SY, Bui M, Lynes EM, Benson MD, Watts R, Vance JE, et al. Ero1alpha requires oxidizing and normoxic conditions to localize to the mitochondria-associated membrane (MAM). Cell Stress Chaperones. (2010) 15:619–29. doi: 10.1007/s12192-010-0174-1
33. Li G, Mongillo M, Chin K-T, Harding H, Ron D, Marks AR, et al. Role of ERO1-alpha-mediated stimulation of inositol 1,4,5-triphosphate receptor activity in endoplasmic reticulum stress-induced apoptosis. J Cell Biol. (2009) 186:783–92. doi: 10.1083/jcb.200904060
34. Higo T, Hattori M, Nakamura T, Natsume T, Michikawa T, Mikoshiba K. Subtype-specific and ER lumenal environment-dependent regulation of inositol 1,4,5-trisphosphate receptor type 1 by ERp44. Cell. (2005) 120:85–98. doi: 10.1016/j.cell.2004.11.048
35. Filipe A, Chernorudskiy A, Arbogast S, Varone E, Villar-Quiles R-N, Pozzer D, et al. Defective endoplasmic reticulum-mitochondria contacts and bioenergetics in SEPN1-related myopathy. Cell Death Differ. (2021) 28:123–38. doi: 10.1038/s41418-020-0587-z
36. Gomez-Suaga P, Paillusson S, Stoica R, Noble W, Hanger DP, Miller CCJ. The ER-mitochondria tethering complex VAPB-PTPIP51 regulates autophagy. Curr Biol. (2017) 27:371–85. doi: 10.1016/j.cub.2016.12.038
37. Gutiérrez T, Simmen T. Endoplasmic reticulum chaperones tweak the mitochondrial calcium rheostat to control metabolism and cell death. Cell Calcium. (2018) 70:64–75. doi: 10.1016/j.ceca.2017.05.015
38. Bers DM. Cardiac excitation-contraction coupling. Nature. (2002) 415:198–205. doi: 10.1038/415198a
39. Gomez L, Thiebaut P-A, Paillard M, Ducreux S, Abrial M, Crola Da Silva C, et al. The SR/ER-mitochondria calcium crosstalk is regulated by GSK3β during reperfusion injury. Cell Death Differ. (2016) 23:313–22. doi: 10.1038/cdd.2015.101
40. Wu S, Lu Q, Wang Q, Ding Y, Ma Z, Mao X, et al. Binding of FUN14 domain containing 1 with inositol 1,4,5-trisphosphate receptor in mitochondria-associated endoplasmic Reticulum membranes maintains mitochondrial dynamics and function in hearts in vivo. Circulation. (2017) 136:2248–66. doi: 10.1161/CIRCULATIONAHA.117.030235
41. Paillard M, Tubbs E, Thiebaut P-A, Gomez L, Fauconnier J, Crola Da Silva C, et al. Depressing mitochondria-reticulum interactions protects cardiomyocytes from lethal hypoxia-reoxygenation injury. Circulation. (2013) 128:1555–65. doi: 10.1161/CIRCULATIONAHA.113.001225
42. Gibellini F, Smith TK. The Kennedy pathway–de novo synthesis of phosphatidylethanolamine and phosphatidylcholine. IUBMB Life. (2010) 62:414–28. doi: 10.1002/iub.337
43. van Meer G, Voelker DR, Feigenson GW. Membrane lipids: where they are and how they behave. Nat Rev Mol Cell Biol. (2008) 9:112. doi: 10.1038/nrm2330
44. Walther TC, Farese RV. Lipid droplets and cellular lipid metabolism. Annu Rev Biochem. (2012) 81:687. doi: 10.1146/annurev-biochem-061009-102430
45. Gaigg B, Simbeni R, Hrastnik C, Paltauf F, Daum G. Characterization of a microsomal subfraction associated with mitochondria of the yeast, Saccharomyces cerevisiae. Involvement in synthesis and import of phospholipids into mitochondria. Biochim Biophys Acta. (1995) 1234:214–20. doi: 10.1016/0005-2736(94)00287-Y
46. Stone SJ, Vance JE. Phosphatidylserine synthase-1 and -2 are localized to mitochondria-associated membranes. J Biol Chem. (2000) 275:34534–40. doi: 10.1074/jbc.M002865200
47. Galmes R, Houcine A, van Vliet AR, Agostinis P, Jackson CL, Giordano F. ORP5/ORP8 localize to endoplasmic reticulum–mitochondria contacts and are involved in mitochondrial function. EMBO Rep. (2016) 17:800–10. doi: 10.15252/embr.201541108
48. van den Brink-van der Laan E, Killian JA, de Kruijff B. Nonbilayer lipids affect peripheral and integral membrane proteins via changes in the lateral pressure profile. Biochim Biophys Acta. (2004) 1666:275–88. doi: 10.1016/j.bbamem.2004.06.010
49. Cui Z, Vance JE, Chen MH, Voelker DR, Vance DE. Cloning and expression of a novel phosphatidylethanolamine N-methyltransferase. A specific biochemical and cytological marker for a unique membrane fraction in rat liver. J Biol Chem. (1993) 268:16655–63. doi: 10.1016/S0021-9258(19)85468-6
50. Guo P-P, Jin X, Zhang J-F, Li Q, Yan C-G, Li X-Z. Overexpression of DGAT2 regulates the differentiation of bovine preadipocytes. Animals. (2023) 13:1195. doi: 10.3390/ani13071195
51. Rusiñol AE, Cui Z, Chen MH, Vance JE. A unique mitochondria-associated membrane fraction from rat liver has a high capacity for lipid synthesis and contains pre-Golgi secretory proteins including nascent lipoproteins. J Biol Chem. (1994) 269:27494–502. doi: 10.1016/S0021-9258(18)47012-3
52. Cao Y, Traer E, Zimmerman GA, McIntyre TM, Prescott SM. Cloning, expression, and chromosomal localization of human long-chain fatty acid-CoA ligase 4 (FACL4). Genomics. (1998) 49:327–30. doi: 10.1006/geno.1998.5268
53. Prasad M, Kaur J, Pawlak KJ, Bose M, Whittal RM, Bose HS. Mitochondria-associated endoplasmic reticulum membrane (MAM) regulates steroidogenic activity via steroidogenic acute regulatory protein (StAR)-voltage-dependent anion channel 2 (VDAC2) interaction. J Biol Chem. (2014) 290:2604. doi: 10.1074/jbc.M114.605808
54. Gilquin B, Taillebourg E, Cherradi N, Hubstenberger A, Gay O, Merle N, et al. The AAA+ ATPase ATAD3A controls mitochondrial dynamics at the interface of the inner and outer membranes. Mol Cell Biol. (2010) 30:1984–96. doi: 10.1128/MCB.00007-10
55. Sala-Vila A, Navarro-Lérida I, Sánchez-Alvarez M, Bosch M, Calvo C, López JA, et al. Interplay between hepatic mitochondria-associated membranes, lipid metabolism and caveolin-1 in mice. Sci Rep. (2016) 6:27351. doi: 10.1038/srep27351
56. Nsiah-Sefaa A, McKenzie M. Combined defects in oxidative phosphorylation and fatty acid β-oxidation in mitochondrial disease. Biosci Rep. (2016) 36:e00313. doi: 10.1042/BSR20150295
57. Dove DE, Su YR, Swift LL, Linton MF, Fazio S. ACAT1 deficiency increases cholesterol synthesis in mouse peritoneal macrophages. Atherosclerosis. (2006) 186:267–74. doi: 10.1016/j.atherosclerosis.2005.08.005
58. Heier C, Haemmerle G. Fat in the heart: the enzymatic machinery regulating cardiac triacylglycerol metabolism. Biochim Biophys Acta. (2016) 1861:1500–12. doi: 10.1016/j.bbalip.2016.02.014
59. Silva-Palacios A, Zazueta C, Pedraza-Chaverri J. ER membranes associated with mitochondria: possible therapeutic targets in heart-associated diseases. Pharmacol Res. (2020) 156:104758. doi: 10.1016/j.phrs.2020.104758
60. Youle RJ, van der Bliek AM. Mitochondrial fission, fusion, and stress. Science. (2012) 337:1062–5. doi: 10.1126/science.1219855
61. Giacomello M, Pyakurel A, Glytsou C, Scorrano L. The cell biology of mitochondrial membrane dynamics. Nat Rev Mol Cell Biol. (2020) 21:204–24. doi: 10.1038/s41580-020-0210-7
62. Chen W, Zhao H, Li Y. Mitochondrial dynamics in health and disease: mechanisms and potential targets. Signal Transduct Target Ther. (2023) 8:333. doi: 10.1038/s41392-023-01547-9
63. Friedman JR, Lackner LL, West M, DiBenedetto JR, Nunnari J, Voeltz GK. ER tubules mark sites of mitochondrial division. Science. (2011) 334:358–62. doi: 10.1126/science.1207385
64. Zhang Z, Liu L, Wu S, Xing D. Drp1, mff, Fis1, and MiD51 are coordinated to mediate mitochondrial fission during UV irradiation-induced apoptosis. FASEB J. (2016) 30:466–76. doi: 10.1096/fj.15-274258
65. Korobova F, Ramabhadran V, Higgs HN. An actin-dependent step in mitochondrial fission mediated by the ER-associated formin INF2. Science. (2013) 339:464–7. doi: 10.1126/science.1228360
66. van der Bliek AM, Shen Q, Kawajiri S. Mechanisms of mitochondrial fission and fusion. Cold Spring Harb Perspect Biol. (2013) 5:a011072. doi: 10.1101/cshperspect.a011072
67. Hoppins S, Lackner L, Nunnari J. The machines that divide and fuse mitochondria. Annu Rev Biochem. (2007) 76:751–80. doi: 10.1146/annurev.biochem.76.071905.090048
68. Chen M, Chen Z, Wang Y, Tan Z, Zhu C, Li Y, et al. Mitophagy receptor FUNDC1 regulates mitochondrial dynamics and mitophagy. Autophagy. (2016) 12:689–702. doi: 10.1080/15548627.2016.1151580
69. Casellas-Díaz S, Larramona-Arcas R, Riqué-Pujol G, Tena-Morraja P, Müller-Sánchez C, Segarra-Mondejar M, et al. Mfn2 localization in the ER is necessary for its bioenergetic function and neuritic development. EMBO Rep. (2021) 22:e51954. doi: 10.15252/embr.202051954
70. Noone J, O’Gorman DJ, Kenny HC. OPA1 regulation of mitochondrial dynamics in skeletal and cardiac muscle. Trends Endocrinol Metab. (2022) 33:710–21. doi: 10.1016/j.tem.2022.07.003
71. van den Hoogenhof MMG, Beqqali A, Amin AS, van der Made I, Aufiero S, Khan MAF, et al. RBM20 mutations induce an arrhythmogenic dilated cardiomyopathy related to disturbed calcium handling. Circulation. (2018) 137:101–15. doi: 10.1161/CIRCULATIONAHA.117.031947
72. Deftereos S, Papoutsidakis N, Giannopoulos G, Angelidis C, Raisakis K, Bouras G, et al. Calcium ions in inherited cardiomyopathies. Med Chem. (2016) 12:139–50. doi: 10.2174/157340641202160209093713
73. Mohamed RMSM, Morimoto S, Ibrahim IAAE-H, Zhan D-Y, Du C-K, Arioka M, et al. GSK-3β heterozygous knockout is cardioprotective in a knockin mouse model of familial dilated cardiomyopathy. Am J Physiol Heart Circ Physiol. (2016) 310:H1808–15. doi: 10.1152/ajpheart.00771.2015
74. Zhou J, Ahmad F, Parikh S, Hoffman NE, Rajan S, Verma VK, et al. Loss of adult cardiac myocyte GSK-3 leads to mitotic catastrophe resulting in fatal dilated cardiomyopathy. Circ Res. (2016) 118:1208–22. doi: 10.1161/CIRCRESAHA.116.308544
75. Luczak ED, Wu Y, Granger JM, Joiner MA, Wilson NR, Gupta A, et al. Mitochondrial CaMKII causes adverse metabolic reprogramming and dilated cardiomyopathy. Nat Commun. (2020) 11:4416. doi: 10.1038/s41467-020-18165-6
76. Hershberger RE, Hedges DJ, Morales A. Dilated cardiomyopathy: the complexity of a diverse genetic architecture. Nat Rev Cardiol. (2013) 10:531–47. doi: 10.1038/nrcardio.2013.105
77. Qiu H, Sun Y, Wang X, Gong T, Su J, Shen J, et al. Lamin A/C deficiency-mediated ROS elevation contributes to pathogenic phenotypes of dilated cardiomyopathy in iPSC model. Nat Commun. (2024) 15:7000. doi: 10.1038/s41467-024-51318-5
78. Lu D, Bao D, Dong W, Liu N, Zhang X, Gao S, et al. Dkk3 prevents familial dilated cardiomyopathy development through Wnt pathway. Lab Invest. (2016) 96:239–48. doi: 10.1038/labinvest.2015.145
79. Minamisawa S, Hoshijima M, Chu G, Ward CA, Frank K, Gu Y, et al. Chronic phospholamban–sarcoplasmic reticulum calcium ATPase interaction is the critical calcium cycling defect in dilated cardiomyopathy. Cell. (1999) 99:313–22. doi: 10.1016/S0092-8674(00)81662-1
80. Hamstra SI, Kurgan N, Baranowski RW, Qiu L, Watson CJF, Messner HN, et al. Low-dose lithium feeding increases the SERCA2a-to-phospholamban ratio, improving SERCA function in murine left ventricles. Exp Physiol. (2020) 105:666–75. doi: 10.1113/EP088061
81. Castillero E, Akashi H, Pendrak K, Yerebakan H, Najjar M, Wang C, et al. Attenuation of the unfolded protein response and endoplasmic reticulum stress after mechanical unloading in dilated cardiomyopathy. Am J Physiol Heart Circ Physiol. (2015) 309:H459–70. doi: 10.1152/ajpheart.00056.2015
82. Wang T, Chowns J, Day SM. Novel insights into DMD-associated dilated cardiomyopathy. Circ Genomic Precis Med. (2023) 16:431–3. doi: 10.1161/CIRCGEN.123.004384
83. Viola HM, Adams AM, Davies SMK, Fletcher S, Filipovska A, Hool LC. Impaired functional communication between the L-type calcium channel and mitochondria contributes to metabolic inhibition in the mdx heart. Proc Natl Acad Sci U S A. (2014) 111:E2905–14. doi: 10.1073/pnas.1402544111
84. Angebault C, Panel M, Lacôte M, Rieusset J, Lacampagne A, Fauconnier J. Metformin reverses the enhanced myocardial SR/ER-mitochondria interaction and impaired complex I-driven respiration in dystrophin-deficient mice. Front Cell Dev Biol. (2020) 8:609493. doi: 10.3389/fcell.2020.609493
85. Bi Y, Xu H, Wang X, Zhu H, Ge J, Ren J, et al. FUNDC1 protects against doxorubicin-induced cardiomyocyte PANoptosis through stabilizing mtDNA via interaction with TUFM. Cell Death Dis. (2022) 13:e5460. doi: 10.1038/s41419-022-05460-x
86. Schaper J, Froede R, Hein S, Buck A, Hashizume H, Speiser B, et al. Impairment of the myocardial ultrastructure and changes of the cytoskeleton in dilated cardiomyopathy. Circulation. (1991) 83:504–12. doi: 10.1161/01.CIR.83.2.504
87. Song M, Mihara K, Chen Y, Scorrano L, Dorn GW. Mitochondrial fission and fusion factors reciprocally orchestrate mitophagic culling in mouse hearts and cultured fibroblasts. Cell Metab. (2015) 21:273–86. doi: 10.1016/j.cmet.2014.12.011
88. Ashrafian H, Docherty L, Leo V, Towlson C, Neilan M, Steeples V, et al. A mutation in the mitochondrial fission gene Dnm1L leads to cardiomyopathy. PLoS Genet. (2010) 6:e1001000. doi: 10.1371/journal.pgen.1001000
89. Qin Y, Li A, Liu B, Jiang W, Gao M, Tian X, et al. Mitochondrial fusion mediated by fusion promotion and fission inhibition directs adult mouse heart function toward a different direction. FASEB J. (2020) 34:663–75. doi: 10.1096/fj.201901671R
90. Chen H, Ren S, Clish C, Jain M, Mootha V, McCaffery JM, et al. Titration of mitochondrial fusion rescues Mff-deficient cardiomyopathy. J Cell Biol. (2015) 211:795–805. doi: 10.1083/jcb.201507035
91. Xia Y, Chen Z, Chen A, Fu M, Dong Z, Hu K, et al. LCZ696 improves cardiac function via alleviating Drp1-mediated mitochondrial dysfunction in mice with doxorubicin-induced dilated cardiomyopathy. J Mol Cell Cardiol. (2017) 108:138–48. doi: 10.1016/j.yjmcc.2017.06.003
92. Chen Y, Liu Y, Dorn GW. Mitochondrial fusion is essential for organelle function and cardiac homeostasis. Circ Res. (2011) 109:1327–31. doi: 10.1161/CIRCRESAHA.111.258723
93. Hsiao YT, Shimizu I, Wakasugi T, Yoshida Y, Ikegami R, Hayashi Y, et al. Cardiac mitofusin-1 is reduced in non-responding patients with idiopathic dilated cardiomyopathy. Sci Rep. (2021) 11:6722. doi: 10.1038/s41598-021-86209-y
94. Wai T, García-Prieto J, Baker MJ, Merkwirth C, Benit P, Rustin P, et al. Imbalanced OPA1 processing and mitochondrial fragmentation cause heart failure in mice. Science. (2015) 350:aad0116. doi: 10.1126/science.aad0116
95. Zhou R, Yazdi AS, Menu P, Tschopp J. A role for mitochondria in NLRP3 inflammasome activation. Nature. (2011) 469:221–5. doi: 10.1038/nature09663
96. Missiroli S, Patergnani S, Caroccia N, Pedriali G, Perrone M, Previati M, et al. Mitochondria-associated membranes (MAMs) and inflammation. Cell Death Dis. (2018) 9:329. doi: 10.1038/s41419-017-0027-2
97. Thoudam T, Jeon J-H, Ha C-M, Lee I-K. Role of mitochondria-associated endoplasmic reticulum membrane in inflammation-mediated metabolic diseases. Mediators Inflamm. (2016) 2016:1851420. doi: 10.1155/2016/1851420
98. Ye L, Zeng Q, Ling M, Ma R, Chen H, Lin F, et al. Inhibition of IP3R/Ca2+ dysregulation protects mice from ventilator-induced lung injury via endoplasmic reticulum and mitochondrial pathways. Front Immunol. (2021) 12:729094. doi: 10.3389/fimmu.2021.729094
99. Zeng C, Duan F, Hu J, Luo B, Huang B, Lou X, et al. NLRP3 inflammasome-mediated pyroptosis contributes to the pathogenesis of non-ischemic dilated cardiomyopathy. Redox Biol. (2020) 34:101523. doi: 10.1016/j.redox.2020.101523
100. Parthenakis FI, Patrianakos A, Prassopoulos V, Papadimitriou E, Nikitovic D, Karkavitsas NS, et al. Relation of cardiac sympathetic innervation to proinflammatory cytokine levels in patients with heart failure secondary to idiopathic dilated cardiomyopathy. Am J Cardiol. (2003) 91:1190–4. doi: 10.1016/s0002-9149(03)00265-0
101. Naito Y, Tsujino T, Fujioka Y, Ohyanagi M, Okamura H, Iwasaki T. Increased circulating interleukin-18 in patients with congestive heart failure. Heart. (2002) 88:296–7. doi: 10.1136/heart.88.3.296
102. Shi J, Zhao Y, Wang K, Shi X, Wang Y, Huang H, et al. Cleavage of GSDMD by inflammatory caspases determines pyroptotic cell death. Nature. (2015) 526:660–5. doi: 10.1038/nature15514
103. Hu J, Xu J, Tan X, Li D, Yao D, Xu B, et al. Dapagliflozin protects against dilated cardiomyopathy progression by targeting NLRP3 inflammasome activation. Naunyn Schmiedebergs Arch Pharmacol. (2023) 396:1461–70. doi: 10.1007/s00210-023-02409-5
104. Zhang W, Xu X, Kao R, Mele T, Kvietys P, Martin CM, et al. Cardiac fibroblasts contribute to myocardial dysfunction in mice with sepsis: the role of NLRP3 inflammasome activation. PLoS One. (2014) 9:e107639. doi: 10.1371/journal.pone.0107639
105. Luo B, Li B, Wang W, Liu X, Xia Y, Zhang C, et al. NLRP3 gene silencing ameliorates diabetic cardiomyopathy in a type 2 diabetes rat model. PLoS One. (2014) 9:e104771. doi: 10.1371/journal.pone.0104771
106. Schönthal AH. Endoplasmic reticulum stress: its role in disease and novel prospects for therapy. Scientifica. (2012) 2012:857516. doi: 10.6064/2012/857516
107. Song S, Tan J, Miao Y, Zhang Q. Crosstalk of ER stress-mediated autophagy and ER-phagy: involvement of UPR and the core autophagy machinery. J Cell Physiol. (2018) 233:3867–74. doi: 10.1002/jcp.26137
108. Verfaillie T, Rubio N, Garg AD, Bultynck G, Rizzuto R, Decuypere J-P, et al. PERK is required at the ER-mitochondrial contact sites to convey apoptosis after ROS-based ER stress. Cell Death Differ. (2012) 19:1880–91. doi: 10.1038/cdd.2012.74
109. Carreras-Sureda A, Jaña F, Urra H, Durand S, Mortenson DE, Sagredo A, et al. Non-canonical function of IRE1α determines mitochondria-associated endoplasmic reticulum composition to control calcium transfer and bioenergetics. Nat Cell Biol. (2019) 21:755–67. doi: 10.1038/s41556-019-0329-y
110. Gkogkas C, Middleton S, Kremer AM, Wardrope C, Hannah M, Gillingwater TH, et al. VAPB interacts with and modulates the activity of ATF6. Hum Mol Genet. (2008) 17:1517–26. doi: 10.1093/hmg/ddn040
111. Ortega A, Roselló-Lletí E, Tarazón E, Molina-Navarro MM, Martínez-Dolz L, González-Juanatey JR, et al. Endoplasmic reticulum stress induces different molecular structural alterations in human dilated and ischemic cardiomyopathy. PLoS One. (2014) 9:e107635. doi: 10.1371/journal.pone.0107635
112. Feyen DAM, Perea-Gil I, Maas RGC, Harakalova M, Gavidia AA, Ataam JA, et al. The unfolded protein response as a compensatory mechanism and potential therapeutic target in PLN R14del cardiomyopathy. Circulation. (2021) 144:382–92. doi: 10.1161/CIRCULATIONAHA.120.049844
113. Bertolotti A, Zhang Y, Hendershot LM, Harding HP, Ron D. Dynamic interaction of BiP and ER stress transducers in the unfolded-protein response. Nat Cell Biol. (2000) 2:326–32. doi: 10.1038/35014014
114. Mekahli D, Bultynck G, Parys JB, De Smedt H, Missiaen L. Endoplasmic-reticulum calcium depletion and disease. Cold Spring Harb Perspect Biol. (2011) 3:a004317. doi: 10.1101/cshperspect.a004317
115. Liu XH, Zhang ZY, Andersson KB, Husberg C, Enger UH, Ræder MG, et al. Cardiomyocyte-specific disruption of Serca2 in adult mice causes sarco(endo)plasmic reticulum stress and apoptosis. Cell Calcium. (2011) 49:201–7. doi: 10.1016/j.ceca.2010.09.009
116. Dally S, Monceau V, Corvazier E, Bredoux R, Raies A, Bobe R, et al. Compartmentalized expression of three novel sarco/endoplasmic reticulum Ca2+ ATPase 3 isoforms including the switch to ER stress, SERCA3f, in non-failing and failing human heart. Cell Calcium. (2009) 45:144–54. doi: 10.1016/j.ceca.2008.08.002
117. Madden E, Logue SE, Healy SJ, Manie S, Samali A. The role of the unfolded protein response in cancer progression: from oncogenesis to chemoresistance. Biol Cell. (2019) 111:1–17. doi: 10.1111/boc.201800050
118. Aghaei M, Dastghaib S, Aftabi S, Aghanoori M-R, Alizadeh J, Mokarram P, et al. The ER stress/UPR axis in chronic obstructive pulmonary disease and idiopathic pulmonary fibrosis. Life Basel Switz. (2020) 11:1. doi: 10.3390/life11010001
119. Ajoolabady A, Lindholm D, Ren J, Pratico D. ER stress and UPR in Alzheimer’s disease: mechanisms, pathogenesis, treatments. Cell Death Dis. (2022) 13:706. doi: 10.1038/s41419-022-05153-5
120. Urano F, Wang X, Bertolotti A, Zhang Y, Chung P, Harding HP, et al. Coupling of stress in the ER to activation of JNK protein kinases by transmembrane protein kinase IRE1. Science. (2000) 287:664–6. doi: 10.1126/science.287.5453.664
121. Son SM, Byun J, Roh S-E, Kim SJ, Mook-Jung I. Reduced IRE1α mediates apoptotic cell death by disrupting calcium homeostasis via the InsP3 receptor. Cell Death Dis. (2014) 5:e1188. doi: 10.1038/cddis.2014.129
122. Parmar VM, Schröder M. Sensing endoplasmic reticulum stress. Adv Exp Med Biol. (2012) 738:153–68. doi: 10.1007/978-1-4614-1680-7_10
123. Monceaux K, Gressette M, Karoui A, Pires Da Silva J, Piquereau J, Ventura-Clapier R, et al. Ferulic acid, pterostilbene, and tyrosol protect the heart from ER-stress-induced injury by activating SIRT1-dependent deacetylation of eIF2α. Int J Mol Sci. (2022) 23:6628. doi: 10.3390/ijms23126628
124. Narula J, Pandey P, Arbustini E, Haider N, Narula N, Kolodgie FD, et al. Apoptosis in heart failure: release of cytochrome c from mitochondria and activation of caspase-3 in human cardiomyopathy. Proc Natl Acad Sci U S A. (1999) 96:8144–9. doi: 10.1073/pnas.96.14.8144
125. Hong BK, Kwon HM, Byun KH, Kim D, Choi EY, Kang TS, et al. Apoptosis in dilated cardiomyopathy. Korean J Intern Med. (2000) 15:56–64. doi: 10.3904/kjim.2000.15.1.56
126. Cesselli D, Jakoniuk I, Barlucchi L, Beltrami AP, Hintze TH, Nadal-Ginard B, et al. Oxidative stress–mediated cardiac cell death is a major determinant of ventricular dysfunction and failure in dog dilated cardiomyopathy. Circ Res. (2001) 89:279–86. doi: 10.1161/hh1501.094115
127. Caillard A, Sadoune M, Cescau A, Meddour M, Gandon M, Polidano E, et al. QSOX1, a novel actor of cardiac protection upon acute stress in mice. J Mol Cell Cardiol. (2018) 119:75–86. doi: 10.1016/j.yjmcc.2018.04.014
128. Giorgi C, Bonora M, Sorrentino G, Missiroli S, Poletti F, Suski JM, et al. P53 at the endoplasmic reticulum regulates apoptosis in a Ca2+-dependent manner. Proc Natl Acad Sci U S A. (2015) 112:1779–84. doi: 10.1073/pnas.1410723112
129. Chen SN, Lombardi R, Karmouch J, Tsai J-Y, Czernuszewicz G, Taylor MRG, et al. DNA damage response/TP53 pathway is activated and contributes to the pathogenesis of dilated cardiomyopathy associated with lamin A/C mutations. Circ Res. (2019) 124:856–73. doi: 10.1161/CIRCRESAHA.118.314238
130. Henpita C, Vyas R, Healy CL, Kieu TL, Gurkar AU, Yousefzadeh MJ, et al. Loss of DNA repair mechanisms in cardiac myocytes induces dilated cardiomyopathy. Aging Cell. (2023) 22:e13782. doi: 10.1111/acel.13782
131. Lalier L, Mignard V, Joalland M-P, Lanoé D, Cartron P-F, Manon S, et al. TOM20-mediated transfer of Bcl2 from ER to MAM and mitochondria upon induction of apoptosis. Cell Death Dis. (2021) 12:182. doi: 10.1038/s41419-021-03471-8
132. Hoppins S, Nunnari J. Mitochondrial dynamics and apoptosis—the ER connection. Science. (2012) 337:1052–4. doi: 10.1126/science.1224709
133. Tsipis A, Athanassiadou AM, Athanassiadou P, Kavantzas N, Agrogiannis G, Patsouris E. Apoptosis-related factors p53, bcl-2 and the defects of force transmission in dilated cardiomyopathy. Pathol Res Pract. (2010) 206:625–30. doi: 10.1016/j.prp.2010.05.007
134. Mazelin L, Panthu B, Nicot A-S, Belotti E, Tintignac L, Teixeira G, et al. mTOR inactivation in myocardium from infant mice rapidly leads to dilated cardiomyopathy due to translation defects and p53/JNK-mediated apoptosis. J Mol Cell Cardiol. (2016) 97:213–25. doi: 10.1016/j.yjmcc.2016.04.011
135. Latif N, Khan MA, Birks E, O’Farrell A, Westbrook J, Dunn MJ, et al. Upregulation of the bcl-2 family of proteins in end stage heart failure. J Am Coll Cardiol. (2000) 35:1769–77. doi: 10.1016/s0735-1097(00)00647-1
136. He X, Cantrell AC, Williams QA, Gu W, Chen Y, Chen JX, et al. p53 acetylation exerts critical roles in pressure overload-induced coronary microvascular dysfunction and heart failure. bioRxiv [Preprint]. (2023) 2023.02.08.527691.
137. Chen J, Shao J, Wang Y, Wu K, Huang M. OPA1, a molecular regulator of dilated cardiomyopathy. J Cell Mol Med. (2023) 27:3017–25. doi: 10.1111/jcmm.17918
138. Yamaguchi R, Lartigue L, Perkins G, Scott RT, Dixit A, Kushnareva Y, et al. Opa1-mediated cristae opening is bax/bak and BH3 dependent, required for apoptosis, and independent of bak oligomerization. Mol Cell. (2008) 31:557–69. doi: 10.1016/j.molcel.2008.07.010
139. Betz C, Stracka D, Prescianotto-Baschong C, Frieden M, Demaurex N, Hall MN. mTOR complex 2-akt signaling at mitochondria-associated endoplasmic reticulum membranes (MAM) regulates mitochondrial physiology. Proc Natl Acad Sci U S A. (2013) 110:12526–34. doi: 10.1073/pnas.1302455110
140. Marchi S, Bittremieux M, Missiroli S, Morganti C, Patergnani S, Sbano L, et al. Endoplasmic reticulum-mitochondria communication through Ca2+ signaling: the importance of mitochondria-associated membranes (MAMs). Adv Exp Med Biol. (2017) 997:49–67. doi: 10.1007/978-981-10-4567-7_4
141. Zhang P, Shan T, Liang X, Deng C, Kuang S. Mammalian target of rapamycin is essential for cardiomyocyte survival and heart development in mice. Biochem Biophys Res Commun. (2014) 452:53–9. doi: 10.1016/j.bbrc.2014.08.046
142. Zhao X, Lu S, Nie J, Hu X, Luo W, Wu X, et al. Phosphoinositide-dependent kinase 1 and mTORC2 synergistically maintain postnatal heart growth and heart function in mice. Mol Cell Biol. (2014) 34:1966–75. doi: 10.1128/MCB.00144-14
143. Mizushima N. Autophagy: process and function. Genes Dev. (2007) 21:2861–73. doi: 10.1101/gad.1599207
144. Russo SB, Baicu CF, Van Laer A, Geng T, Kasiganesan H, Zile MR, et al. Ceramide synthase 5 mediates lipid-induced autophagy and hypertrophy in cardiomyocytes. J Clin Invest. (2012) 122:3919–30. doi: 10.1172/JCI63888
145. Kroemer G, Mariño G, Levine B. Autophagy and the integrated stress response. Mol Cell. (2010) 40:280–93. doi: 10.1016/j.molcel.2010.09.023
146. Kanamori H, Yoshida A, Naruse G, Endo S, Minatoguchi S, Watanabe T, et al. Impact of autophagy on prognosis of patients with dilated cardiomyopathy. J Am Coll Cardiol. (2022) 79:789–801. doi: 10.1016/j.jacc.2021.11.059
147. Sheng S, Li J, Hu X, Wang Y. Regulated cell death pathways in cardiomyopathy. Acta Pharmacol Sin. (2023) 44:1521–35. doi: 10.1038/s41401-023-01068-9
148. Saito T, Asai K, Sato S, Hayashi M, Adachi A, Sasaki Y, et al. Autophagic vacuoles in cardiomyocytes of dilated cardiomyopathy with initially decompensated heart failure predict improved prognosis. Autophagy. (2016) 12:579–87. doi: 10.1080/15548627.2016.1145326
149. Yang M, Li C, Yang S, Xiao Y, Xiong X, Chen W, et al. Mitochondria-associated ER membranes – the origin site of autophagy. Front Cell Dev Biol. (2020) 8:595. doi: 10.3389/fcell.2020.00595
150. Hamasaki M, Furuta N, Matsuda A, Nezu A, Yamamoto A, Fujita N, et al. Autophagosomes form at ER-mitochondria contact sites. Nature. (2013) 495:389–93. doi: 10.1038/nature11910
151. Gelmetti V, De Rosa P, Torosantucci L, Marini ES, Romagnoli A, Di Rienzo M, et al. PINK1 and BECN1 relocalize at mitochondria-associated membranes during mitophagy and promote ER-mitochondria tethering and autophagosome formation. Autophagy. (2017) 13:654–69. doi: 10.1080/15548627.2016.1277309
152. Chen Y, Dorn GW. PINK1-phosphorylated Mitofusin 2 is a Parkin receptor for culling damaged mitochondria. Science. (2013) 340:471–5. doi: 10.1126/science.1231031
153. Bhandari P, Song M, Chen Y, Burelle Y, Dorn GW. Mitochondrial contagion induced by Parkin deficiency in Drosophila hearts and its containment by suppressing mitofusin. Circ Res. (2014) 114:257–65. doi: 10.1161/CIRCRESAHA.114.302734
154. Maejima Y, Isobe M, Sadoshima J. Regulation of autophagy by beclin 1 in the heart. J Mol Cell Cardiol. (2016) 95:19–25. doi: 10.1016/j.yjmcc.2015.10.032
155. Maejima Y, Kyoi S, Zhai P, Liu T, Li H, Ivessa A, et al. Mst1 inhibits autophagy by promoting the interaction between Beclin1 and Bcl-2. Nat Med. (2013) 19:1478–88. doi: 10.1038/nm.3322
156. Hu Y, Chen H, Zhang L, Lin X, Li X, Zhuang H, et al. The AMPK-MFN2 axis regulates MAM dynamics and autophagy induced by energy stresses. Autophagy. (2021) 17:1142–56. doi: 10.1080/15548627.2020.1749490
157. Cahill TJ, Leo V, Kelly M, Stockenhuber A, Kennedy NW, Bao L, et al. Resistance of dynamin-related protein 1 oligomers to disassembly impairs mitophagy, resulting in myocardial inflammation and heart failure. J Biol Chem. (2015) 290:25907–19. doi: 10.1074/jbc.M115.665695
158. Nair U, Yen W-L, Mari M, Cao Y, Xie Z, Baba M, et al. A role for Atg8-PE deconjugation in autophagosome biogenesis. Autophagy. (2012) 8:780–93. doi: 10.4161/auto.19385
159. Ji X, Zheng D, Ni R, Wang J, Shao J, Vue Z, et al. Sustained over-expression of calpain-2 induces age-dependent dilated cardiomyopathy in mice through aberrant autophagy. Acta Pharmacol Sin. (2022) 43:2873–84. doi: 10.1038/s41401-022-00965-9
160. Cho GW, Altamirano F, Hill JA. Chronic heart failure: Ca2+, catabolism, and catastrophic cell death. Biochim Biophys Acta. (2016) 1862:763–77. doi: 10.1016/j.bbadis.2016.01.011
161. He H, Liu X, Lv L, Liang H, Leng B, Zhao D, et al. Calcineurin suppresses AMPK-dependent cytoprotective autophagy in cardiomyocytes under oxidative stress. Cell Death Dis. (2014) 5:e997. doi: 10.1038/cddis.2013.533
162. Choi JC, Muchir A, Wu W, Iwata S, Homma S, Morrow JP, et al. Temsirolimus activates autophagy and ameliorates cardiomyopathy caused by lamin A/C gene mutation. Sci Transl Med. (2012) 4:144ra102. doi: 10.1126/scitranslmed.3003875
163. Diwan A, Wansapura J, Syed FM, Matkovich SJ, Lorenz JN, Dorn GW. Nix-mediated apoptosis links myocardial fibrosis, cardiac remodeling, and hypertrophy decompensation. Circulation. (2008) 117:396–404. doi: 10.1161/CIRCULATIONAHA.107.727073
164. Diwan A, Matkovich SJ, Yuan Q, Zhao W, Yatani A, Brown JH, et al. Endoplasmic reticulum–mitochondria crosstalk in NIX-mediated murine cell death. J Clin Invest. (2009) 119:203–12. doi: 10.1172/JCI36445
165. Chen Y, Lewis W, Diwan A, Cheng EH-Y, Matkovich SJ, Dorn GW. Dual autonomous mitochondrial cell death pathways are activated by nix/BNip3l and induce cardiomyopathy. Proc Natl Acad Sci U S A. (2010) 107:9035–42. doi: 10.1073/pnas.0914013107
166. Kung G, Konstantinidis K, Kitsis RN. Programmed necrosis, not apoptosis, in the heart. Circ Res. (2011) 108:1017–36. doi: 10.1161/CIRCRESAHA.110.225730
167. Song Z, Song H, Liu D, Yan B, Wang D, Zhang Y, et al. Overexpression of MFN2 alleviates sorafenib-induced cardiomyocyte necroptosis via the MAM-CaMKIIδ pathway in vitro and in vivo. Theranostics. (2022) 12:1267–85. doi: 10.7150/thno.65716
168. Jiang X, Stockwell BR, Conrad M. Ferroptosis: mechanisms, biology and role in disease. Nat Rev Mol Cell Biol. (2021) 22:266–82. doi: 10.1038/s41580-020-00324-8
169. Fang X, Wang H, Han D, Xie E, Yang X, Wei J, et al. Ferroptosis as a target for protection against cardiomyopathy. Proc Natl Acad Sci U S A. (2019) 116:2672–80. doi: 10.1073/pnas.1821022116
170. Fang X, Cai Z, Wang H, Han D, Cheng Q, Zhang P, et al. Loss of cardiac ferritin H facilitates cardiomyopathy via Slc7a11-mediated ferroptosis. Circ Res. (2020) 127:486–501. doi: 10.1161/CIRCRESAHA.120.316509
171. Murphy CJ, Oudit GY. Iron-overload cardiomyopathy: pathophysiology, diagnosis, and treatment. J Card Fail. (2010) 16:888–900. doi: 10.1016/j.cardfail.2010.05.009
172. Sun Y, Yan C, He L, Xiang S, Wang P, Li Z, et al. Inhibition of ferroptosis through regulating neuronal calcium homeostasis: an emerging therapeutic target for Alzheimer’s disease. Ageing Res Rev. (2023) 87:101899. doi: 10.1016/j.arr.2023.101899
173. Kagan VE, Mao G, Qu F, Angeli JPF, Doll S, Croix CS, et al. Oxidized arachidonic/adrenic phosphatidylethanolamines navigate cells to ferroptosis. Nat Chem Biol. (2017) 13:81–90. doi: 10.1038/nchembio.2238
174. Nechushtai R, Karmi O, Zuo K, Marjault H-B, Darash-Yahana M, Sohn Y-S, et al. The balancing act of NEET proteins: iron, ROS, calcium and metabolism. Biochim Biophys Acta Mol Cell Res. (2020) 1867:118805. doi: 10.1016/j.bbamcr.2020.118805
175. Lynes EM, Bui M, Yap MC, Benson MD, Schneider B, Ellgaard L, et al. Palmitoylated TMX and calnexin target to the mitochondria-associated membrane. EMBO J. (2012) 31:457–70. doi: 10.1038/emboj.2011.384
176. Ta N, Qu C, Wu H, Zhang D, Sun T, Li Y, et al. Mitochondrial outer membrane protein FUNDC2 promotes ferroptosis and contributes to doxorubicin-induced cardiomyopathy. Proc Natl Acad Sci U S A. (2022) 119:e2117396119. doi: 10.1073/pnas.2117396119
177. Wang Z, Xia Q, Su W, Cao M, Sun Y, Zhang M, et al. Exploring the communal pathogenesis, ferroptosis mechanism, and potential therapeutic targets of dilated cardiomyopathy and hypertrophic cardiomyopathy via a microarray data analysis. Front Cardiovasc Med. (2022) 9:824756. doi: 10.3389/fcvm.2022.824756
Keywords: MAMS, DCM, MAMs-associated proteins, cardiomyocytes, mitochondrial dysfunction
Citation: He P, Chang H, Qiu Y and Wang Z (2025) Mitochondria associated membranes in dilated cardiomyopathy: connecting pathogenesis and cellular dysfunction. Front. Cardiovasc. Med. 12:1571998. doi: 10.3389/fcvm.2025.1571998
Received: 6 February 2025; Accepted: 27 February 2025;
Published: 17 March 2025.
Edited by:
Yang Yang, First Affiliated Hospital of Zhengzhou University, ChinaReviewed by:
Mathieu Panel, INSERM U1046 Physiologie et Médecine Expérimentale du Coeur et des Muscles, FranceCopyright: © 2025 He, Chang, Qiu and Wang. This is an open-access article distributed under the terms of the Creative Commons Attribution License (CC BY). The use, distribution or reproduction in other forums is permitted, provided the original author(s) and the copyright owner(s) are credited and that the original publication in this journal is cited, in accordance with accepted academic practice. No use, distribution or reproduction is permitted which does not comply with these terms.
*Correspondence: Zhentao Wang, MTM4MDM4MTc3OTZAMTM5LmNvbQ==
†These authors share first authorship
Disclaimer: All claims expressed in this article are solely those of the authors and do not necessarily represent those of their affiliated organizations, or those of the publisher, the editors and the reviewers. Any product that may be evaluated in this article or claim that may be made by its manufacturer is not guaranteed or endorsed by the publisher.
Research integrity at Frontiers
Learn more about the work of our research integrity team to safeguard the quality of each article we publish.