- 1Cardiovascular Division, Department of Medicine, Heart and Lung Research Institute (HLRI), University of Cambridge, Cambridge, United Kingdom
- 2British Heart Foundation Centre of Research Excellence, University of Cambridge, Cambridge, United Kingdom
Atherosclerosis, the leading cause of death worldwide, is a chronic inflammatory disease leading to the accumulation of lipid-rich plaques in the intima of large and medium-sized arteries. Accumulating evidence indicates the important regulatory role of the adaptive immune system in atherosclerosis during all stages of the disease. The gut microbiome has also become a key regulator of atherosclerosis and immunomodulation. Whilst existing research extensively explores the impact of the microbiome on the innate immune system, only a handful of studies have explored the regulatory capacity of the microbiome on the adaptive immune system to modulate atherogenesis. Building on these concepts and the pitfalls on the gut microbiota and adaptive immune response interaction, this review explores potential strategies to therapeutically target the microbiome, including the use of prebiotics and vaccinations, which could influence the adaptive immune response and consequently plaque composition and development.
1 Introduction
Cardiovascular diseases (CVDs) are the leading cause of death globally (1). In the majority of cases the underlying cause is atherosclerosis, a complex arterial pathology with multiple genetic and environmental risk factors. Atherogenesis is initiated in response to the trapping of low-density lipoproteins (LDL) in the intima and their acquisition of immunogenic properties through both enzymatic and oxidative modifications. The subsequent immune response involves interactions between many vascular and circulating cells and mediators, and frequently leads to a chronic inflammatory state due, at least in part, to defects in counter-regulatory mechanisms. Extensive evidence supports the inflammatory theory of atherosclerosis (2), and innate and adaptive immune cells have been shown to participate in all stages of the disease from its initiation to progression and plaque rupture or erosion (3). Moreover, atherosclerosis is a metabolic inflammatory disease, sensitive to changes in diet and strongly influenced by the intestinal microbiota.
Microbiota, refers to the collective microbial community inside and on the surface of the human body and plays a very important role in immune homeostasis and atherosclerosis. Several studies have determined the innate immune system as a link between gut dysbiosis and atherosclerosis, but less is known about the contribution of adaptive immunity to the process. In this review we are going to focus on how the gut microbiota influences the adaptive immune response and atherosclerosis, and how it could be modulated and targeted to alter the adaptive immune response to treat atherosclerosis.
1.1 Atherosclerosis and adaptive immune system
The adaptive immune response is a specialized response activated through molecules (antigens) recognized by specialized, highly selected and clonally developed receptors, like immunoglobins (Ig) in B cells and T cell receptors (TCR) in T cells. Innate immune cells, mainly macrophages and dendritic cells (DC), act as antigen presenting cells (APC) and initiate the adaptive immune response. They drive the polarization of naïve CD4+ and CD8+ T cells to effector and/or memory cells of specialized T helper (Th) or T regulatory (Tregs) -cell subsets through exposure of antigenic peptides on major histocompatibility complex (MHC) class I or II molecules, along with the engagement of co-stimulatory pathways and the action of different cytokines in secondary lymphoid organs (4). While Tregs have been proposed to have an atheroprotective role, Th cells exhibit different roles depending of the subtype (5). Th1 are atherogenic cells, while Th2, Th17 and Tfh may play contextual dependent atherogenic or atheroprotective roles. All types of CD4+ T cells have been found in atherosclerotic plaque lesions or the adventitia as well as in the blood of patients with atherosclerotic lesions (6).
Similarly, B cells are also important players in the adaptive immune response in atherosclerosis. There are different B cell subsets with different pro-atherogenic or atheroprotective functions depending on the main antibody type or the cytokine they secrete [reviewed in detail in (7)]. In general B1 (8, 9) and B2-Marginal Zone B cells (MZB) (10–12) cells protect from atherosclerosis by secreting IgM antibodies in a T independent or dependent manner respectively. While B2-Follicular B (FOB) cells are considered atherogenic for promoting the formation of the Germinal Centre (GC) (13) response and atherogenic class switched IgG antibodies.
1.2 Gut microbiota
The human body contains a broad number of microorganisms (∼4 × 1013 microbial cells) including bacteria, viruses, protozoa, archaea and fungi, which constitute the commensal microbiota that mainly resides in the gut. This commensal flora is unique to each individual and has a mutualistic relationship with its host. On one hand, it benefits from a constant supply of host substrates and in return, the host also benefits from bacterial activities that are important to keep a homeostatic physiological body balance (14) like fermentation of non-digestible substrates (i.e., dietary fibres); production of certain vitamins (i.e., vitamin E); maintenance of the correct functioning of the immune system; protection against infections; as well as, controlling the gut-brain communication network between the enteric and the central nervous system. Gut microbiota express over 3 × 106 of genes producing millions of metabolites with diverse functions in the host. Collectively, these genes expressed by the microbiota constitute the microbiome (15, 16). There are several factors that can modulate gut microbiota like host genetics, age and diet. Based on the Twins UK study (17) less than 10% of the gut microbiota taxa may exhibit a heritable trait, thus in general environmental factors like diet are more important determinants of the microbiota composition. Several mouse and human studies have reproducibly shown that a more diverse gut microbiota is associated with a “healthy gut”, conversely, a dramatic imbalance in the composition and function of these microorganisms, termed as gut dysbiosis, leads to a decreased microbial diversity that translates into a “leaky gut” and systemic inflammation causing obesity, autoimmune diseases, type 2 diabetes and cardiovascular diseases (18). Many studies have demonstrated that a diverse gut can be achieved with a diverse diet (19), but other environmental factors like medication and blood clinical markers also have an important role. For example, in a recent large-scale human study integrating microbiota profiles with clinical blood markers, diet and medication, blood LDL levels were strongly negatively associated with gut diversity (20).
There are five major bacterial phyla in the intestinal flora: Bacteroidetes (includes genera like Bacteroides and Prevotella); Firmicutes [includes genera like Clostridium (∼95%), Lactobacillus, Bacillus, Enterococcus, and Ruminicoccus]; Actinobacteria (includes genera like Bifidobacterium); Proteobacteria; Fusobacteria and Verrucomicrobia (21) that have distinct functions and are predominantly located in specific regions along the gut. In the lower intestine anaerobic bacteria are the predominant type particularly Bacteroides, Bifidobacteria, Fusobacteria and Peptostreptococci, while anaerobes and facultative aerobes such as Enterobacteria and Lactobacilli are present at moderate density (22). In homeostasis, more than 90% of bacteria in both mice and human consist of Bacteroidetes and Firmicutes (23). An increased Firmicutes/Bacteroidetes (F/B) ratio has been associated with obesity (24) and CVDs (25), so for many years, this ratio has been widely considered to be a marker of gut health. However, this concept has been challenged by more recent studies on obesity [reviewed in (26, 27)] and the same may apply to CVD, which has been lesser studied than obesity but further experiments are necessary to corroborate this.
1.3 Gut microbiota and atherosclerosis
Gut microbiota does regulate the risk factors (dyslipaemia, hypertension, obesity etc) [extensively reviewed in (28–30)] and the regulators (immune and inflammatory cells and mediators) [extensively reviewed in (31)] that lead to atherosclerosis. Thus, there is no surprise that studies in germ-free animals (GF) have shown that the gut microbiota plays a prominent role in atherosclerosis. Unexpectedly, GF ApoE−/− mice, with higher plasma and hepatic cholesterol levels, developed less atherosclerosis than conventionally raised (Conv) ApoE−/− fed a control diet (early atherosclerosis model) (32, 33). Conversely, there were no significant differences when feeding a high fat high cholesterol (HF HC) diet for 12 weeks to the same mice models (33) or for 16 weeks to Conv and GF LDLr−/− (advanced atherosclerotic models) (34). These experiments suggest that gut microbiota may play a more significant role in early atherosclerosis, a time-point in which the adaptive immune response plays a more prominent role (35). Thus, further experiments are necessary to explore how gut microbiota could be modulating the adaptive immune response and its effect on early atherosclerosis.
In humans there are a very few and small-sized studies that have been performed to identify gut microbiota species that are different between individuals with atherosclerosis or other CVD and healthy controls (Table 1) (36–55). Bacterial DNA resembling that in the gut is present in atherosclerotic plaques, but apart from a handful of descriptive studies there are not functional studies to understand the role of these bacteria in the plaque and if they could be associated with increased CV risk (41, 53). Many different gut species have also been identified as significantly increased or decreased in patients vs. controls, but there is no consensus regarding species that are directly linked to increased risk (36–55). Furthermore, while decreased alpha-diversity is associated with disease in general, only one study comparing controls and atherosclerotic patients found increased diversity in healthy vs. atherosclerotic patients (), so clearly larger epidemiological studies are needed to shed light into the relationship between gut microbiota and CVD. Using a considerable sample from the Framingham Heart Study it was shown that microbial diversity decreased with 10-year CVD risk, and this was mostly driven by BMI and lifestyle factors (54).
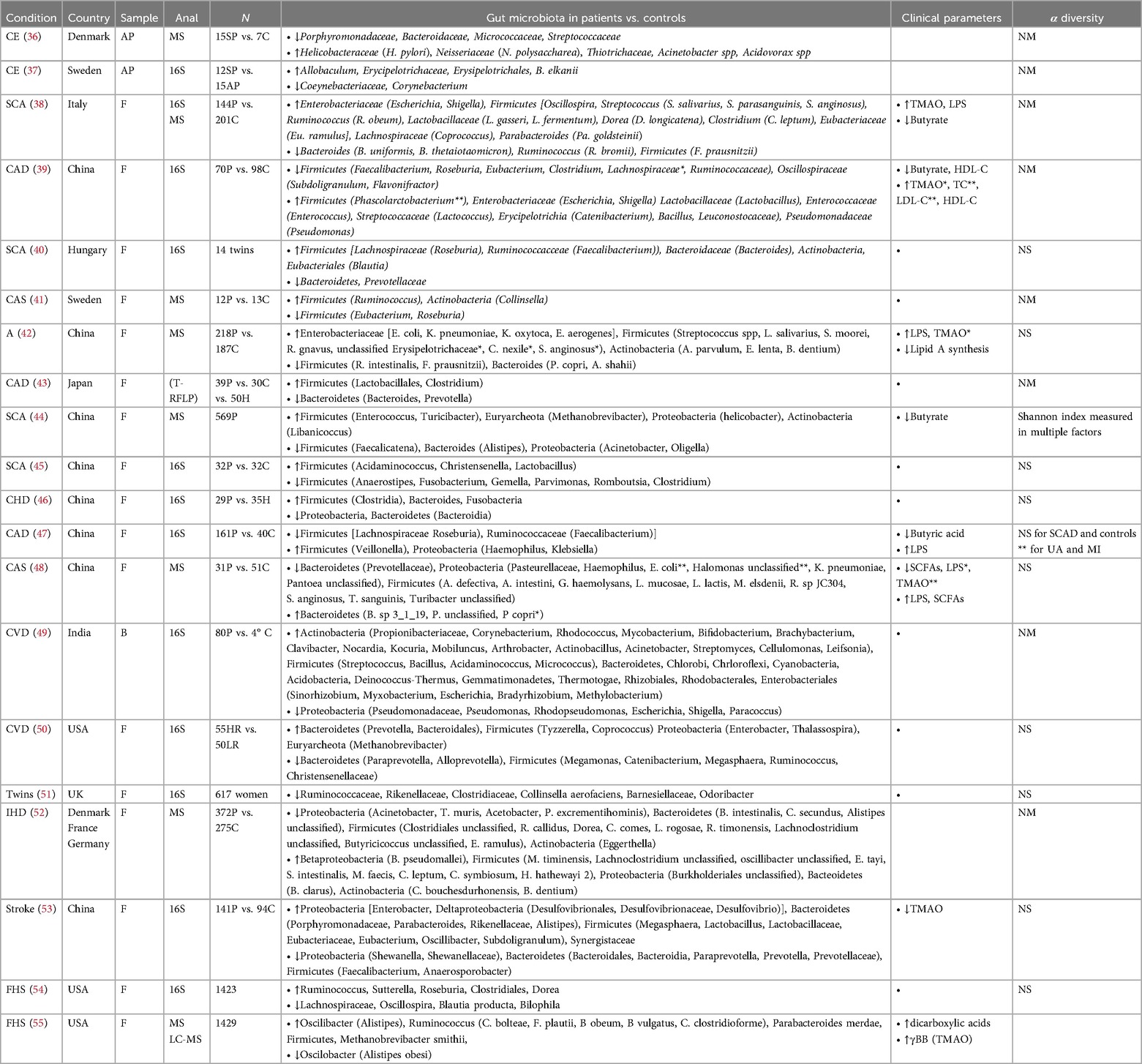
Table 1. List of clinical studies determining atherosclerotic plaque or gut microbiota composition in individuals with atherosclerosis and other associated cardiovascular diseases vs. healthy controls. CE, carotid endarectomy; SCA, subclinical carotid atherosclerosis; CAD, coronary artery disease; CAS, carotid atherosclerosis; A, atherosclerosis; CHD, coronary heart disease; CVD, cardiovascular disease; IHD, ischemic heart disease; AP, atherosclerotic plaques; F, faecal; 16S, 16S rRNA sequencing; MS, metagenomic shotgun sequencing; P, patients; C, control; TMAO, trimethylamine-N-Oxide; LPS, lipopolysaccharide; HDL-C, high density lipoprotein—cholesterol; FHS, framingham heart study. TC, total cholesterol; LDL-C, low density lipoprotein; SCFAs, short-chain fatty acids; NS, not significant differences; NM, not measured. Terminal restriction fragment length polymorphism (T-RFLP).
Despite not specific species have been identified, there is growing evidence on how specific gut microbiota products and metabolites are linked to increased risk of atherosclerosis (p.e. LPS and TMAO), while others exhibit atheroprotective properties [p.e. short chain fatty acids (SCFA)] [reviewed extensively in (57, 58)]. These metabolites also influence the adaptive immune response, an in general those atherogenic metabolites favor proinflammatory subsets while atheroprotective metabolites enhance anti-inflammatory adaptive immune cells (Figure 1) as it will be summarised below.
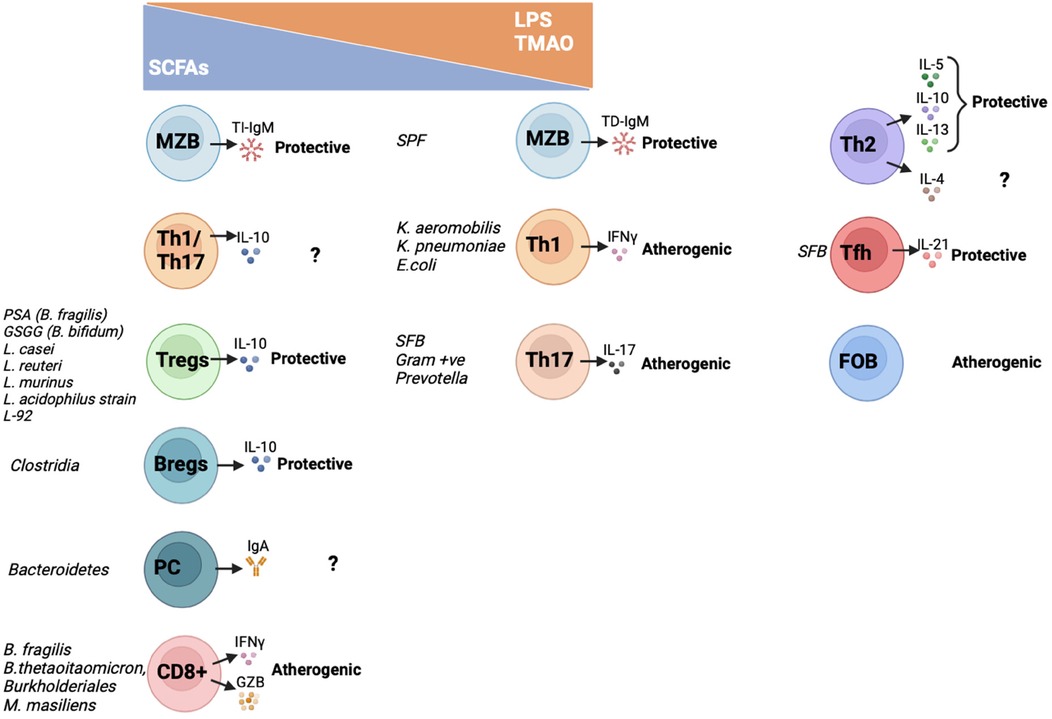
Figure 1. Interactions between metabolites, gut microbiota and adaptive immune cells and their effect on atherosclerosis. In this graph it is summarized how gut microbiota species and derived metabolites regulate activation and cytokine and antibody secretion of the different adaptive immune cell subsets. On one hand, atheroprotective SCFA increase TI-IgM secretion by MZB cells; IL10-Th1/Th17 secreting cells; Tregs; Bregs; IgA-producing PC and CD8T cells. On the other hand, pro-atherogenic TMAO and/or LPS activate TD-IgM secretion by MZB cells; Th1 and Th17 cells. The metabolites that activate Th2, Tfh and FOB have not been characterized yet. SCFAs, short chain fatty acids; LPS, lipopolysaccharide; TMAO, trimethylamine N-oxide; MZB, Marginal Zone B cells; Th, T helper cells; Tfh, T follicular helper cells; Tregs, T regulatory cells; Bregs, B regulatory cells; PC, plasma cells; FOB, Follicular B cells; PSA, polysaccharide A; GSGG, beta-glycan/galactan; SFB, Segmented filamentous bacteria; SPF, specific pathogen free; TI-IgM, T-independent immunoglobulin M; TD-IgM, T-dependent immunoglobulin M; IgA, immunoglobulin A; IL, interleukin; IFNγ, interferon γ; GZB, granzyme B.
1.4 Gut microbiota and the adaptive immune response and its potential role on atherosclerosis
For many years, special attention was paid to the role of the gut microbiota in the gastrointestinal tract and associated tertiary lymphoid structures. More recently, it has been shown that the gut microbiota exerts a remote effect, and may affect the immune response systemically (59, 60). One reason for this is that gut microbiota releases active metabolites and molecules (p.e. LPS, TMAO or SCFA) that can interact with remotely located organs and affect systemic immune responses and atherosclerosis. Another reason for this is because immune cells that change locally due to diet and gut composition in mesenteric lymphoid nodes migrate to the periphery fueling T cell accumulation within atherosclerotic lesions (61). Bellow we will summarize how the different immune cell subsets can be modulated by gut microbiota locally and/or systemically and how this can impact the development of atherosclerosis.
1.4.1 Cd4+ T cells
1.4.1.1 Th1 cells
Th1 cells express the transcription factor T-bet and signal transducer and activator of transcription 4 (STAT-4), and secrete interferon γ (IFN- γ), interleukin 2 (IL-2), IL-3, tumour necrosis factor (TNF) and lymphotoxin (62, 63). Experimental mouse models have shown that Th1 cells are pro-atherogenic [reviewed in (5)] and are the dominant CD4+ T cells in human atherosclerotic lesions (64–66).
Several gut bacteria species (p.e. Klebsiella and E. coli strains from the Enterobacteriaceae family) have been shown to locally modulate Th1 polarization in both mice (67) and humans (68). In accordance with this, Enterobacteriaceae were also significantly increased in the faeces of different atherosclerotic cohorts compared to healthy controls (38, 39, 42, 49, 50) that could potentially contributted to the development of the disease. As expected, atheroprotective SCFAs like butyrate were shown to inhibit T-bet and IFNγ (42), skewing Th1 differentiation into anti-inflammatory IL-10-secreting in a G-coupled protein receptor (GPR)-43 dependent-manner in experimental mouse models of colitis and in human T cells (69). On the contrary, pro-atherogenic LPS and TMAO were shown to enhance the polarization of pro-inflammatory macrophages resulting in the expansion and proliferation of Th1 and Th17 cells in advance mouse models of atherosclerosis (70, 71).
1.4.1.2 Th2 cells
Th2 cells express the transcription factor GATA3 and secrete IL-4, IL-5, IL-10 and IL-13 (72). Because Th2 signature cytokines can counteract the Th1 pro-inflammatory response, they were initially considered protective in atherosclerosis. But now we know, that while IL-5, IL-10 and IL-13 are atheroprotective, controversial results have been found regarding IL-4 [reviewed in (5, 73)]. As expected, those species that enhance a Th1 response have been shown to limit a Th2 response (p.e. Lactobacillus strains and B. fragilis) (67, 74).
1.4.1.3 Th17 cells
Th17 cells expressing the transcription factor RAR-related orphan receptor (ROR) γt, are activated by IL-23 and secrete IL-17. Their role in atherosclerosis remains controversial as both atherogenic (75, 76) and atheroprotective (77) effects have been described.
Th17, especially those located in the intestine, are among the T cells that are more amenable by the gut microbiota and their interactions have been widely studied in autoimmune and metabolic diseases. In fact, mouse studies have shown that the gut microbiota is essential for Th17 differentiation (78, 79). Colonization of GF mice intestine with segmented filamentous bacteria (SFB), gram-positive bacteria and Prevotella induced Th17 differentiation and promoted secretion of IL-17 and IL-22 (67, 80–83). In a similar manner to Th1, SCFA inhibit RORγt and Th17 differentiation (74) and promote Th17 IL-10-secreting cells through inhibition of histone deacetylase (HDAC) and activation of mTOR in a GPR-43 independent manner (84). In atherosclerosis, LDLr−/− mice fed a HF/HC diet supplemented with a cocktail of peptides that can modify the growth from a HF/HC diet derived gut microbiota toward a low-fat diet one, lead to a significant increase of Tregs and a decrease of Th17 reducing atherosclerosis (85).
1.4.1.4 Tfh cells
Tfh cells express the transcription factor Bcl-6 and the chemokine receptors CXCR5, ICOS and PD1. They are a specialized subset of CD4+ T cells that provide help to B cells and are essential for germinal center formation, affinity maturation and the development of high affinity antibodies and memory B cells (86). They are atheroprotective by modulating IgM secretion of MZB cells (10–12).
The relationship between gut microbiota and Tfh is mutual, not only does gut microbiota affect Tfh differentiation and function but Tfh also shapes gut microbiota through receptors that are able to sense the gut microbiota and to produce an appropriate ecosystem for its development. So, on one hand P2X7 (87) and PD1 (88) on Tfh are necessary to secrete gut microbiota specific IgA antibodies and to maintain a more diverse microbiota. And on the other hand, Tfh differentiation are absent in GF mice and restored upon microbial transplant in a TLR2-MyD88 dependent manner (89). Also, SFB in Peyer's patches were shown to enhance pro-inflammatory Tfh differentiation by restricting IL-2 access to CD4+ T cells in a dendritic cell dependent manner and favoring Bcl-6 expression in the gut of a mouse model of arthritis (90).
1.4.1.5 Treg cells
Treg cells express the transcription factor FoxP3 and secrete anti-inflammatory cytokines like IL-10, TGF-β, and IL-35 (91). They preserve immune tolerance, block excessive inflammation and have an immune suppressive activity (92). They exhibit a prominent atheroprotective role (93–96) and clinical trials using low dose IL-2 (which increases Tregs) have been initiated to treat ischaemic heart disease (97, 98). Interestingly, low dose IL-2 has been shown to affect gut microbiota in mice and humans (99) so this interaction may have important therapeutical implications.
Both intestinal and peripheral Treg cells differentiation are regulated by SCFA in a GPR-43-dependent manner (100). Moreover, butyrate and propionate enhance extrathymic Treg production activating intronic enhancer CNS1 (101) or inhibiting HDAC (101, 102) respectively. As expected, in atherosclerosis, ApoE−/− mice fed a HF/HC diet supplemented with propionic acid developed less atherosclerotic plaques than those that were not supplemented due to increased Treg cell numbers and lL-10 levels in the gut microenvironment (103). And these effects were reverted when blocking IL-10R. The promising beneficial effects of propionic acid are all based in animal studies, thus an exhaustive toxicity and safety study should be run before being able to think in translational studies. Other microbiota derived metabolites like polysaccharide A produced by B. fragilis (104) and beta-glycan/galactan produced by B. bifidum also promote expansion of Treg cells and IL-10 production in a TLR2 signalling dependent manner (105). Several specific strains, like Lactobacillus casei (106), L. reuteri (107), L. murinus (108) and L. acidophilus strain L-92 (109) have been associated with increased production of Tregs in small experimental animal models.
1.4.2 Cd8+ T cells
Similarly to CD4+ T cells, CD8+ T cells are generated in the thymus and express the TCR but they recognize antigens presented by MHC class I (located in all nucleated cells). They participate in the host defense against intracellular pathogens and tumor surveillance. They secrete effector cytokines like IFNγ and TNFα but they also have a cytotoxic function by secreting perforins and granzymes (GZ). In human plaques it has been reported that their percentage is lower than CD4+ T cells but, as the disease advances, and specifically in lesions susceptible to rupture, they account for up to half of the T cell population (110–112). Experimental animal models and single cell transcriptomics of human atherosclerotic plaques have shown that GZB and GZK producing CD8+ T cells enhance the development of atherosclerosis (112–114).
CD8+ T cells are reduced in GF mice, and several species have been shown to enhance their activation (102, 115–117). Controversial results have been shown about the impact of butyrate and propionate on CD8+ T cells, some showing an inhibitory effect (118), while others an stimulatory effect by enhancing their IFNγ and GZB secretion in a GPR-41/43 independent manner inhibiting HDAC (115). Thus, experimental studies supplementing with SCFA to increase Tregs will need to check that they do not enhance proatherosclerotic CD8+ T cells.
1.4.3 B lymphocytes
B cells play important roles in both innate and adaptive immune responses. They undergo hypersomatic mutations and become antibody-producing cells (GC, plasmablasts and plasma cells). Antibodies are glycoproteins of the Ig family that are attached to the B cell membranes serving as B cell receptor (BCR) for antigens or can be secreted into the extracellular space and the circulation where they bind to auto- or foreign antigens. Several studies have shown that bacterial antigens are essential for the maturation, differentiation and antibody secretion of all B cells.
1.4.3.1 Follicular B cells (FOB)
FOB cells are B2 cells that are located in the follicles in the spleen and can circulate in both mice and humans between other secondary lymphoid organs like Peyer's patches in the gut (119). They produce class switch antibodies in a T-cell dependent manner through the activation of BCR, TLR and BAFF/APRIL signaling pathways. They are activated in the follicles by Tfh entering the GC response and generating PC and high affinity antibodies (IgG, IgA, IgE etc). Both, FOB and GC B are considered proatherogenic (120–123).
sIgA are the first line of defense in protecting the mucosal tissues from infections and maintaining gut homeostasis within the microbiota (124–126). They are produced by B cells in the Peyer patches and are induced by food antigens and gut bacteria [p.e. gut microbiota derived SCFA and Bacteroidetes are indispensable to generate IgA producing PC by inhibiting HDAC and boosting PC differentiation signalling pathways (like Xbp1) (127)]. Very little is known about the potential role of IgA in atherosclerosis. On one hand, ApoE−/− atherosclerotic mice have higher IgA levels than C57Bl6 WT mice (128). And in humans, high levels of sIgA in blood have recently been associated with increased severity of atherosclerosis in the Rotterdam Study (129). But on another hand, sIgA also have atheroprotective properties like maintaining a diverse microbiota and facilitating Tregs activation (130). Thus, mouse and human studies are needed to clarify the exact role of sIgA in atherosclerosis.
Gut microbiota also has an important role in regulating the rest of the Ig repertoire besides IgA, but it has been less studied. Using ApoE−/− mice treated with high spectrum antibiotics, Chen et al. demonstrated that gut microbiota is an important trigger for the recruitment and activation of pro-atherogenic B2 cells producing IgG in perivascular fat (131). Further studies are needed to establish the importance of gut microbiota in antibody class switching.
1.4.3.2 Marginal zone B cells (MZB)
MZB cells reside only in the outer layer of the follicles in the spleen in mice. But in humans unswitched MZ-like cells account for the majority of the activated B cells in blood (132). We have previously shown that in response to a high fat high cholesterol (HF/HC) diet, MZB cells protect from atherosclerosis by limiting Tfh cells in a Pdl1-dependent manner downstream of TLR and BCR signalling pathways (10). LPS levels were significantly increased in LDLr−/− fed a HF/HC so we hypothesize that gut microbiota is necessary to activate MZB cells atheroprotective programme and further experiments are needed to test if the absence of TLRs specifically in MZB cells would affect their activation and function in the atherosclerotic context.
MZB cells are not affected in GF mice, but several studies have shown that gut microbiota is important in their development and antibody secretion. Mice with a restricted flora (RF) (rich in Firmicutes) show a complete deletion of MZB cells while their development was normal in SPF mice (rich in Bacteroidetes) (133). Regarding their BCR repertoire, human MZB cell precursors migrate to the gut associated lymphoid tissue (GALT) for somatic hypermutation and this process is regulated by gut commensals (134, 135). Furthermore, T independent IgM secretion by MZB cells depend on the presence of peri-MZ neutrophils that colonize that area after post-natal microbial colonization (136) as well as on GPR43, as its deletion in MZB cells, decreases expression of surface proteins (CD21, CD1d, CD24, IgM, etc) and enhances the formation of T-independent IgM antibodies and anti-dsDNA autoantibodies (137).
1.4.3.3 B1 cells
In mice B1 cells reside in the peritoneum and the pleural cavities and are divided into B1-a and B1-b cells. Their human counterparts have started to be identified (138). Experimental mice models have shown that B1 cells protect from atherosclerosis in a T cell independent manner by generating natural IgM antibodies (8, 9, 139). Although B1 cells can also migrate to the intestines, their contribution to the total IgA plasma pool is minimal as demonstrated using gnotobiotic Ig allotype chimeric mice (140). Similarly, to MZB cells, commensal microbe and post-natal microbial colonization drives the pre-immune B cell repertoire of B1 cells and the concomitant development of IgA and IgM secreting PC (141, 142). It has been described that in aging there is an accumulation of pro-inflammatory B1 cells that express 4-1BBL leading to insulin resistance due to a significant decrease of anti-inflammatory A. muciniphila in the gut (143).
1.4.3.4 B regulatory (Breg) cells
Breg cells exert immunosuppressive and regulatory functions. They increase in response to proinflammatory IL-6, IL-1β, IL-21, BAFF and GM-CSF and secrete IL-10 (144). In general, they exhibit an atheroprotective role (145), despite it might be through other functions independently of IL10 secretion (146).
Gut microbiota is necessary for splenic and MLN Breg cells differentiation as well as IL-10 and IL-35 secretion (147). Mice with aberrant or lack of gut microbiota do not develop IL-10-secreting Breg cells in an IL-6/IL-1B (147) and a TLR2/TLR9 ligands dependent manner (148, 149). As expected, Clostridia (that is the main strain that produces SCFA) induces the secretion of IL-10 by Bregs (150).
1.5 How we could target the gut microbiota to treat atherosclerosis regulating the adaptive immune response
1.5.1 Probiotics
Probiotics are live microorganisms that are beneficial for the host's health when administered in sufficient amounts (151). They can be used to combat gut microbiome dysbiosis, inhibiting the growth of harmful bacteria and “triggering” the growth of beneficial bacteria (152). To be considered a probiotic there has to be scientific evidence (153), nevertheless much of this scientific evidence relies on small sample sized studies that also lack appropriate controls. Thus, there is an urgent need for larger studies and meta-analyses to elucidate the effects of probiotics across different diseases and populations. The most frequently used probiotics, found in fermented dairy such as yoghurt are from Lactobacillus and Bifidobacterium genera and have shown to modulate both the adaptive immune responses in a strain dependent manner [reviewed extensively in (154)] and dyslipemia in hypercholesterolemic patients (155) but not in normocholesterolemics (156). In experimental mice models, administration of Akkermansia muciniphila (an intestinal commensal with anti-inflammatory properties) to ApoE−/− mice reduced atherosclerosis due to decreased endotoxemia (157) but did not affect neointima formation in ApoE3-Leiden mice (158). The effect of probiotics on the adaptive immune response during atherosclerosis remains unexplored.
1.5.2 Prebiotics
Prebiotics are indigestible compounds found in certain foods that selectively nourish beneficial bacteria in the gut improving host wellbeing (159). They can be naturally occurring in fruit, vegetables or whole grains or can be made synthetically. Nondigestible carbohydrates, including oligosaccharides and polysaccharides are the most popular prebiotics (160, 161). They primarily influence the adaptive immune system indirectly promoting probiotics’ growth [extensively summarized in (162)], or via altering metabolite production (p.e. increasing SCFA production). Polyphenols, found in tea, vegetable and cereals modulate the gut microbiota and have been reported to have prebiotic effects via enhancing the growth of probiotic families including Lactobacilli, inhibiting pathogenic bacteria such as E.coli (163, 164) and increasing SCFA production (164) in mice studies. In fact, tea, also reduced plaque development in ApoE−/− atherosclerotic mice (165). In humans, several small sized prospective observational studies have associated olive oil, berries, pomegranate juice and cocoa rich in prebiotics with cardioprotective and anti-atherogenic properties (165–171). Nevertheless, in the future larger population studies of the gut microbiota combined with nutritional, genetic and immunephenotyping analysis will be needed to understand thir interaction and how we can utilize it to treat atherosclerosis.
Furthermore, there is an important interaction gut microbiota—anti-atherosclerotic drugs that needs to be studied in more depth to develop more effective and personalized therapies to treat atherosclerosis. For example, statins exhibit prebiotic effects by altering gut microbiota (p.e.: decreasing Clostridium) and/or SCFA (171, 172) while at the same time a more diverse gut microbiota leads to a statin greater response (173).
1.5.3 Fecal Microbiota transplantation (FMT)
FMT refers to the transfer of one's own or a donor's faecal sample to a receiver to restore gut microbiome homeostasis. It can be used to improve the gut microbial ecology, however there is an associated risk of transmitting infectious agents and the challenge of donor selection in humans (174, 175). In humans, FMT has been demonstrated to be successful for treating recurrent infections of Clostridium difficile (176). Animal experiments using FMT to treat atherosclerosis yielded promising results. FMT from atherogenic mice to WT recipient mice resulted in increased plaque size, increasing circulating innate immune cells and elevated proinflammatory cytokines (177). On the contrary, FMT from healthy mice into an atherosclerotic mouse model significantly decreased disease burden (178). In humans FMT from vegan controls into 20 patients with metabolic syndrome did not affect TMAO or other pro-inflammatory markers (179). While in a more recent clinical trial involving 237 patients that received a FMT from healthy controls demonstrated a significant reduction in cardiovascular risk in patients with metabolic syndrome (180). Additional studies to comprehensively examine the potential efficacy, adverse effects, and translatability of FMT are needed to conclude if this could be a promising therapy to treat atherosclerosis.
1.5.4 Vaccinations
Several studies have demonstrated that B cells in atherosclerotic lesions locally produce antibodies that can react against gut microbe antigens. This may be attributed to bacteria originating from the microbiome present in atherosclerotic plaque (54, 181) or by those present in GALT (132). Notably, these antibodies produced by B lymphocytes in plaques have been reported to exhibit cross-reactivity with epitopes such as oxidised low-density lipoprotein (oxLDL) and cytoskeletal proteins (p.e. transgelin type 1) associated with atherogenesis (182). The development of vaccines that promote atheroprotective antibodies or neutralize atherogenic factors could lower cardiovascular risk.
Binder et al. (183) showed that pneumococcal polysaccharide vaccine (PPV) decreased atherosclerotic lesion formation through a molecular mimicry mechanism between heat-killed Streptococcus pneumoniae, found in the microbiome, and oxLDL. This atheroprotective effect was thought to be due to numerous mechanisms including high anti-oxLDL IgM titres blocking uptake of oxLDL by macrophages effectively (184–186). Since then, the link between PPV and cardiovascular events has been controversial. Some trials have provided evidence for an association between PPV and reduced risk of cardiovascular ischaemic events (187, 188) while other studies have not (189–191). However, meta-analyses generally demonstrate significant reductions in CV risk in patients over 65, despite heterogenous samples (192). New clinical studies are ongoing, such as the Australian Study for the Prevention through Immunisation of Cardiovascular Events (AUSPICE), which recruited 4,275 participants, to investigate this further. Although the conclusive findings of this study are pending publication, their preliminary results in smaller subgroups show no changes in CVD and or antibody titers (193, 194).
Additional gut and oral microbial pathogens have been trialed for vaccination. Porphyromonas gingivalis, a microbe found in the oral cavity and atherosclerotic plaques has been shown to expedite atherosclerosis via a cross-reactivity mechanism (195, 196). Immunisation against P. gingivalis was able to mitigate pathogen-induced atherosclerosis in ApoE−/− but not in WT mice (197) or on top of statins (198). Moreover, immunization with the outer membrane protein of Enterobacteriaceae resulted in decreased inflammatory cells and increased M2 macrophages observed in plaques of both ApoE−/− and WT mice (181). Human clinical trials are necessary to investigate their translational effect.
In our opinion, vaccines using microbial antigens is a very promising weapon to treat atherosclerosis. However, the challenge of vaccine development includes identifying the correct antigens, predicting efficacy based on findings made on animal models and determining how to measure reduction in plaque size in humans. Successful vaccine development requires the collaboration of multi-disciplinary teams and integration of various techniques.
2 Conclusion
The microbiome has emerged as a promising target for addressing atherosclerosis, due to its diverse effects spanning from immunomodulation to metabolite secretion. This review provides a comprehensive overview of how microbiome-targeting mechanisms influence the adaptive immune system, contributing to either atheroprotection or atherogenesis. Whilst the impact of the microbiome on the innate immune system has been studied extensively, the potential role in targeting the adaptive immune system is less understood. Harnessing the microbiome as a therapeutic target can yield multifaceted benefits, including specific and specialized immunomodulation, making it a compelling target for the development of more effective therapies to treat atherosclerosis.
Author contributions
DG: Writing – original draft, Writing – review & editing. AI: Writing – original draft, Writing – review & editing. MN: Conceptualization, Funding acquisition, Supervision, Writing – original draft, Writing – review & editing.
Funding
The author(s) declare financial support was received for the research, authorship, and/or publication of this article. This work has been funded by FS/20/23/34784 and PG/22/10898 awarded to MN by the British Heart Foundation.
Conflict of interest
The authors declare that the research was conducted in the absence of any commercial or financial relationships that could be construed as a potential conflict of interest.
Generative AI statement
The author(s) declare that no Generative AI was used in the creation of this manuscript.
Publisher's note
All claims expressed in this article are solely those of the authors and do not necessarily represent those of their affiliated organizations, or those of the publisher, the editors and the reviewers. Any product that may be evaluated in this article, or claim that may be made by its manufacturer, is not guaranteed or endorsed by the publisher.
References
1. Brown JM, Hazen SL. Microbial modulation of cardiovascular disease. Nat Rev Microbiol. (2018) 16(3):171–81. doi: 10.1038/nrmicro.2017.149
2. Ridker PM. From CANTOS to CIRT to COLCOT to clinic: will all atherosclerosis patients soon be treated with combination lipid-lowering and inflammation-inhibiting agents? Circulation. (2020) 141(10):787–9. doi: 10.1161/CIRCULATIONAHA.119.045256
3. Lahoute C, Herbin O, Mallat Z, Tedgui A. Adaptive immunity in atherosclerosis: mechanisms and future therapeutic targets. Nat Rev Cardiol. (2011) 8(6):348–58. doi: 10.1038/nrcardio.2011.62
4. Saigusa R, Winkels H, Ley K. T cell subsets and functions in atherosclerosis. Nat Rev Cardiol. (2020) 17(7):387–401. doi: 10.1038/s41569-020-0352-5
5. Nus M, Mallat Z. Immune-mediated mechanisms of atherosclerosis and implications for the clinic. Expert Rev Clin Immunol. (2016) 12(11):1217–37. doi: 10.1080/1744666X.2016.1195686
6. Fernandez DM, Rahman AH, Fernandez NF, Chudnovskiy A, Amir ED, Amadori L, et al. Single-cell immune landscape of human atherosclerotic plaques. Nat Med. (2019) 25(10):1576–88. doi: 10.1038/s41591-019-0590-4
7. Sage AP, Tsiantoulas D, Binder CJ, Mallat Z. The role of B cells in atherosclerosis. Nat Rev Cardiol. (2019) 16(3):180–96. doi: 10.1038/s41569-018-0106-9
8. Pattarabanjird T, Li C, McNamara C. B cells in atherosclerosis: mechanisms and potential clinical applications. JACC Basic Transl Sci. (2021) 6(6):546–63. doi: 10.1016/j.jacbts.2021.01.006
9. Kyaw T, Tay C, Krishnamurthi S, Kanellakis P, Agrotis A, Tipping P, et al. B1a B lymphocytes are atheroprotective by secreting natural IgM that increases IgM deposits and reduces necrotic cores in atherosclerotic lesions. Circ Res. (2011) 109(8):830–40. doi: 10.1161/CIRCRESAHA.111.248542
10. Nus M, Sage AP, Lu Y, Masters L, Lam BYH, Newland S, et al. Marginal zone B cells control the response of follicular helper T cells to a high-cholesterol diet. Nat Med. (2017) 23(5):601–10. doi: 10.1038/nm.4315
11. Nus M, Basatemur G, Galan M, Cros-Brunso L, Zhao TX, Masters L, et al. NR4A1 deletion in marginal zone B cells exacerbates atherosclerosis in mice-brief report. Arterioscler Thromb Vasc Biol. (2020) 40(11):2598–604. doi: 10.1161/ATVBAHA.120.314607
12. Harrison J, Newland SA, Jiang W, Giakomidi D, Zhao X, Clement M, et al. Marginal zone B cells produce ‘natural’ atheroprotective IgM antibodies in a T cell-dependent manner. Cardiovasc Res. (2024) 120(3):318–28. doi: 10.1093/cvr/cvae027
13. Centa M, Jin H, Hofste L, Hellberg S, Busch A, Baumgartner R, et al. Germinal center-derived antibodies promote atherosclerosis plaque size and stability. Circulation. (2019) 139(21):2466–82. doi: 10.1161/CIRCULATIONAHA.118.038534
14. Krautkramer KA, Kreznar JH, Romano KA, Vivas EI, Barrett-Wilt GA, Rabaglia ME, et al. Diet-microbiota interactions mediate global epigenetic programming in multiple host tissues. Mol Cell. (2016) 64(5):982–92. doi: 10.1016/j.molcel.2016.10.025
15. Lynch SV, Pedersen O. The human intestinal microbiome in health and disease. N Engl J Med. (2016) 375(24):2369–79. doi: 10.1056/NEJMra1600266
16. Zhou W, Cheng Y, Zhu P, Nasser MI, Zhang X, Zhao M. Implication of gut microbiota in cardiovascular diseases. Oxid Med Cell Longev. (2020) 2020:5394096. doi: 10.1155/2020/5394096
17. Goodrich JK, Davenport ER, Beaumont M, Jackson MA, Knight R, Ober C, et al. Genetic determinants of the gut microbiome in UK twins. Cell Host Microbe. (2016) 19(5):731–43. doi: 10.1016/j.chom.2016.04.017
18. Tang WHW, Backhed F, Landmesser U, Hazen SL. Intestinal microbiota in cardiovascular health and disease: JACC state-of-the-art review. J Am Coll Cardiol. (2019) 73(16):2089–105. doi: 10.1016/j.jacc.2019.03.024
19. Heiman ML, Greenway FL. A healthy gastrointestinal microbiome is dependent on dietary diversity. Mol Metab. (2016) 5(5):317–20. doi: 10.1016/j.molmet.2016.02.005
20. Manor O, Dai CL, Kornilov SA, Smith B, Price ND, Lovejoy JC, et al. Health and disease markers correlate with gut microbiome composition across thousands of people. Nat Commun. (2020) 11(1):5206. doi: 10.1038/s41467-020-18871-1
21. Eckburg PB, Bik EM, Bernstein CN, Purdom E, Dethlefsen L, Sargent M, et al. Diversity of the human intestinal microbial flora. Science. (2005) 308(5728):1635–8. doi: 10.1126/science.1110591
22. Macpherson AJ, Harris NL. Interactions between commensal intestinal bacteria and the immune system. Nat Rev Immunol. (2004) 4(6):478–85. doi: 10.1038/nri1373
23. Nguyen TL, Vieira-Silva S, Liston A, Raes J. How informative is the mouse for human gut microbiota research? Dis Model Mech. (2015) 8(1):1–16. doi: 10.1242/dmm.017400
24. Qin J, Li R, Raes J, Arumugam M, Burgdorf KS, Manichanh C, et al. A human gut microbial gene catalogue established by metagenomic sequencing. Nature. (2010) 464(7285):59–65. doi: 10.1038/nature08821
25. Gill SR, Pop M, Deboy RT, Eckburg PB, Turnbaugh PJ, Samuel BS, et al. Metagenomic analysis of the human distal gut microbiome. Science. (2006) 312(5778):1355–9. doi: 10.1126/science.1124234
26. Magne F, Gotteland M, Gauthier L, Zazueta A, Pesoa S, Navarrete P, et al. The Firmicutes/Bacteroidetes ratio: a relevant marker of gut dysbiosis in obese patients? Nutrients. (2020) 12(5):1474. doi: 10.3390/nu12051474
27. Castaner O, Goday A, Park YM, Lee SH, Magkos F, Shiow STE, et al. The gut microbiome profile in obesity: a systematic review. Int J Endocrinol. (2018) 2018:4095789. doi: 10.1155/2018/4095789
28. Li L, Sheng Q, Zeng H, Li W, Wang Q, Ma G, et al. Engineering a functional thyroid as a potential therapeutic substitute for hypothyroidism treatment: a systematic review. Front Endocrinol (Lausanne). (2022) 13:1065410. doi: 10.3389/fendo.2022.1065410
29. O'Donnell JA, Zheng T, Meric G, Marques FZ. The gut microbiome and hypertension. Nat Rev Nephrol. (2023) 19(3):153–67. doi: 10.1038/s41581-022-00654-0
30. Van Hul M, Cani PD. The gut microbiota in obesity and weight management: microbes as friends or foe? Nat Rev Endocrinol. (2023) 19(5):258–71. doi: 10.1038/s41574-022-00794-0
31. Eshghjoo S, Jayaraman A, Sun Y, Alaniz RC. Microbiota-mediated immune regulation in atherosclerosis. Molecules. (2021) 26(1):179. doi: 10.3390/molecules26010179
32. Kasahara K, Tanoue T, Yamashita T, Yodoi K, Matsumoto T, Emoto T, et al. Commensal bacteria at the crossroad between cholesterol homeostasis and chronic inflammation in atherosclerosis. J Lipid Res. (2017) 58(3):519–28. doi: 10.1194/jlr.M072165
33. Lindskog Jonsson A, Caesar R, Akrami R, Reinhardt C, Fak Hallenius F, Boren J, et al. Impact of gut microbiota and diet on the development of atherosclerosis in apoe(-/-) mice. Arterioscler Thromb Vasc Biol. (2018) 38(10):2318–26. doi: 10.1161/ATVBAHA.118.311233
34. Kiouptsi K, Jackel S, Pontarollo G, Grill A, Kuijpers MJE, Wilms E, et al. The microbiota promotes arterial thrombosis in low-density lipoprotein receptor-deficient mice. mBio. (2019) 10(5):02298–19. doi: 10.1128/mBio.02298-19
35. Song L, Leung C, Schindler C. Lymphocytes are important in early atherosclerosis. J Clin Invest. (2001) 108(2):251–9. doi: 10.1172/JCI200111380
36. Mitra S, Drautz-Moses DI, Alhede M, Maw MT, Liu Y, Purbojati RW, et al. In silico analyses of metagenomes from human atherosclerotic plaque samples. Microbiome. (2015) 3:38. doi: 10.1186/s40168-015-0100-y
37. Lindskog Jonsson A, Hallenius FF, Akrami R, Johansson E, Wester P, Arnerlov C, et al. Bacterial profile in human atherosclerotic plaques. Atherosclerosis. (2017) 263:177–83. doi: 10.1016/j.atherosclerosis.2017.06.016
38. Baragetti A, Severgnini M, Olmastroni E, Dioguardi CC, Mattavelli E, Angius A, et al. Gut microbiota functional dysbiosis relates to individual diet in subclinical carotid atherosclerosis. Nutrients. (2021) 13(2):304. doi: 10.3390/nu13020304
39. Zhu Q, Gao R, Zhang Y, Pan D, Zhu Y, Zhang X, et al. Dysbiosis signatures of gut microbiota in coronary artery disease. Physiol Genomics. (2018) 50(10):893–903. doi: 10.1152/physiolgenomics.00070.2018
40. Szabo H, Hernyes A, Piroska M, Ligeti B, Fussy P, Zoldi L, et al. Association between gut microbial diversity and carotid intima-Media thickness. Medicina (Kaunas). (2021) 57(3):195. doi: 10.3390/medicina57030195
41. Karlsson FH, Fak F, Nookaew I, Tremaroli V, Fagerberg B, Petranovic D, et al. Symptomatic atherosclerosis is associated with an altered gut metagenome. Nat Commun. (2012) 3:1245. doi: 10.1038/ncomms2266
42. Jie Z, Xia H, Zhong SL, Feng Q, Li S, Liang S, et al. The gut microbiome in atherosclerotic cardiovascular disease. Nat Commun. (2017) 8(1):845. doi: 10.1038/s41467-017-00900-1
43. Emoto T, Yamashita T, Sasaki N, Hirota Y, Hayashi T, So A, et al. Analysis of gut Microbiota in coronary artery disease patients: a possible link between gut Microbiota and coronary artery disease. J Atheroscler Thromb. (2016) 23(8):908–21. doi: 10.5551/jat.32672
44. Zhu S, Xu K, Jiang Y, Zhu C, Suo C, Cui M, et al. The gut microbiome in subclinical atherosclerosis: a population-based multiphenotype analysis. Rheumatology (Oxford). (2021) 61(1):258–69. doi: 10.1093/rheumatology/keab309
45. Ji L, Chen S, Gu G, Zhou J, Wang W, Ren J, et al. Exploration of crucial mediators for carotid atherosclerosis pathogenesis through integration of microbiome, metabolome, and transcriptome. Front Physiol. (2021) 12:645212. doi: 10.3389/fphys.2021.645212
46. Cui L, Zhao T, Hu H, Zhang W, Hua X. Association study of gut Flora in coronary heart disease through high-throughput sequencing. Biomed Res Int. (2017) 2017:3796359. doi: 10.1155/2017/3796359
47. Liu H, Chen X, Hu X, Niu H, Tian R, Wang H, et al. Alterations in the gut microbiome and metabolism with coronary artery disease severity. Microbiome. (2019) 7(1):68. doi: 10.1186/s40168-019-0683-9
48. Chen J, Qin Q, Yan S, Yang Y, Yan H, Li T, et al. Gut microbiome alterations in patients with carotid atherosclerosis. Front Cardiovasc Med. (2021) 8:739093. doi: 10.3389/fcvm.2021.739093
49. Dinakaran V, Rathinavel A, Pushpanathan M, Sivakumar R, Gunasekaran P, Rajendhran J. Elevated levels of circulating DNA in cardiovascular disease patients: metagenomic profiling of microbiome in the circulation. PLoS One. (2014) 9(8):e105221. doi: 10.1371/journal.pone.0105221
50. Kelly TN, Bazzano LA, Ajami NJ, He H, Zhao J, Petrosino JF, et al. Gut microbiome associates with lifetime cardiovascular disease risk profile among bogalusa heart study participants. Circ Res. (2016) 119(8):956–64. doi: 10.1161/CIRCRESAHA.116.309219
51. Menni C, Lin C, Cecelja M, Mangino M, Matey-Hernandez ML, Keehn L, et al. Gut microbial diversity is associated with lower arterial stiffness in women. Eur Heart J. (2018) 39(25):2390–7. doi: 10.1093/eurheartj/ehy226
52. Fromentin S, Forslund SK, Chechi K, Aron-Wisnewsky J, Chakaroun R, Nielsen T, et al. Microbiome and metabolome features of the cardiometabolic disease spectrum. Nat Med. (2022) 28(2):303–14. doi: 10.1038/s41591-022-01688-4
53. Yin J, Liao SX, He Y, Wang S, Xia GH, Liu FT, et al. Dysbiosis of gut microbiota with reduced trimethylamine-N-oxide level in patients with large-artery atherosclerotic stroke or transient ischemic attack. J Am Heart Assoc. (2015) 4(11):e002699. doi: 10.1161/JAHA.115.002699
54. Koren O, Spor A, Felin J, Fak F, Stombaugh J, Tremaroli V, et al. Human oral, gut, and plaque microbiota in patients with atherosclerosis. Proc Natl Acad Sci U S A. (2011) 108(Suppl 1):4592–8. doi: 10.1073/pnas.1011383107
55. Walker RL, Vlamakis H, Lee JWJ, Besse LA, Xanthakis V, Vasan RS, et al. Population study of the gut microbiome: associations with diet, lifestyle, and cardiometabolic disease. Gen Med. (2021) 13:188. doi: 10.1186/s13073-021-01007-5
56. Li C, Strazar M, Mohamed AMT, Pacheco JA, Walker RL, Lebar T, et al. Gut microbiota and metabolome profiling in Framingham heart study reveals cholesterol-metabolizing bacteria. Cell. (2024) 187:1834–52. doi: 10.1016/j.cell.2024.03.014
57. Kiouptsi K, Pontarollo G, Reinhardt C. Gut Microbiota and the microvasculature. Cold Spring Harb Perspect Med. (2023) 13(8). doi: 10.1101/cshperspect.a041179
58. Formes H, Reinhardt C. The gut microbiota - a modulator of endothelial cell function and a contributing environmental factor to arterial thrombosis. Expert Rev Hematol. (2019) 12(7):541–9. doi: 10.1080/17474086.2019.1627191
59. Chistiakov DA, Bobryshev YV, Kozarov E, Sobenin IA, Orekhov AN. Role of gut microbiota in the modulation of atherosclerosis-associated immune response. Front Microbiol. (2015) 6:671. doi: 10.3389/fmicb.2015.00671
60. Kamada N, Seo SU, Chen GY, Nunez G. Role of the gut microbiota in immunity and inflammatory disease. Nat Rev Immunol. (2013) 13(5):321–35. doi: 10.1038/nri3430
61. Laurans L, Mouttoulingam N, Chajadine M, Lavelle A, Diedisheim M, Bacquer E, et al. An obesogenic diet increases atherosclerosis through promoting microbiota dysbiosis-induced gut lymphocyte trafficking into the periphery. Cell Rep. (2023) 42(11):113350. doi: 10.1016/j.celrep.2023.113350
62. Zundler S, Neurath MF. Interleukin-12: functional activities and implications for disease. Cytokine Growth Factor Rev. (2015) 26(5):559–68. doi: 10.1016/j.cytogfr.2015.07.003
63. Butcher MJ, Filipowicz AR, Waseem TC, McGary CM, Crow KJ, Magilnick N, et al. Atherosclerosis-Driven treg plasticity results in formation of a dysfunctional subset of plastic IFNgamma+ Th1/tregs. Circ Res. (2016) 119(11):1190–203. doi: 10.1161/CIRCRESAHA.116.309764
64. Ait-Oufella H, Taleb S, Mallat Z, Tedgui A. Recent advances on the role of cytokines in atherosclerosis. Arterioscler Thromb Vasc Biol. (2011) 31(5):969–79. doi: 10.1161/ATVBAHA.110.207415
65. Buono C, Come CE, Stavrakis G, Maguire GF, Connelly PW, Lichtman AH. Influence of interferon-gamma on the extent and phenotype of diet-induced atherosclerosis in the LDLR-deficient mouse. Arterioscler Thromb Vasc Biol. (2003) 23(3):454–60. doi: 10.1161/01.ATV.0000059419.11002.6E
66. Niwa T, Wada H, Ohashi H, Iwamoto N, Ohta H, Kirii H, et al. Interferon-gamma produced by bone marrow-derived cells attenuates atherosclerotic lesion formation in LDLR-deficient mice. J Atheroscler Thromb. (2004) 11(2):79–87. doi: 10.5551/jat.11.79
67. Atarashi K, Suda W, Luo C, Kawaguchi T, Motoo I, Narushima S, et al. Ectopic colonization of oral bacteria in the intestine drives T(H)1 cell induction and inflammation. Science. (2017) 358(6361):359–65. doi: 10.1126/science.aan4526
68. Lloyd-Price J, Arze C, Ananthakrishnan AN, Schirmer M, Avila-Pacheco J, Poon TW, et al. Multi-omics of the gut microbial ecosystem in inflammatory bowel diseases. Nature. (2019) 569(7758):655–62. doi: 10.1038/s41586-019-1237-9
69. Sun M, Wu W, Chen L, Yang W, Huang X, Ma C, et al. Microbiota-derived short-chain fatty acids promote Th1 cell IL-10 production to maintain intestinal homeostasis. Nat Commun. (2018) 9(1):3555. doi: 10.1038/s41467-018-05901-2
70. Kuznetsova T, Prange KHM, Glass CK, de Winther MPJ. Transcriptional and epigenetic regulation of macrophages in atherosclerosis. Nat Rev Cardiol. (2020) 17(4):216–28. doi: 10.1038/s41569-019-0265-3
71. Wu K, Yuan Y, Yu H, Dai X, Wang S, Sun Z, et al. The gut microbial metabolite trimethylamine N-oxide aggravates GVHD by inducing M1 macrophage polarization in mice. Blood. (2020) 136(4):501–15. doi: 10.1182/blood.2019003990
72. Winkels H, Ehinger E, Vassallo M, Buscher K, Dinh HQ, Kobiyama K, et al. Atlas of the immune cell repertoire in mouse atherosclerosis defined by single-cell RNA-sequencing and mass cytometry. Circ Res. (2018) 122(12):1675–88. doi: 10.1161/CIRCRESAHA.117.312513
73. Engelbertsen D, Andersson L, Ljungcrantz I, Wigren M, Hedblad B, Nilsson J, et al. T-helper 2 immunity is associated with reduced risk of myocardial infarction and stroke. Arterioscler Thromb Vasc Biol. (2013) 33(3):637–44. doi: 10.1161/ATVBAHA.112.300871
74. Chen L, Ruan G, Cheng Y, Yi A, Chen D, Wei Y. The role of Th17 cells in inflammatory bowel disease and the research progress. Front Immunol. (2023) 13:1055914. doi: 10.3389/fimmu.2022.1055914
75. Eid RE, Rao DA, Zhou J, Lo SF, Ranjbaran H, Gallo A, et al. Interleukin-17 and interferon-gamma are produced concomitantly by human coronary artery-infiltrating T cells and act synergistically on vascular smooth muscle cells. Circulation. (2009) 119(10):1424–32. doi: 10.1161/CIRCULATIONAHA.108.827618
76. Erbel C, Dengler TJ, Wangler S, Lasitschka F, Bea F, Wambsganss N, et al. Expression of IL-17A in human atherosclerotic lesions is associated with increased inflammation and plaque vulnerability. Basic Res Cardiol. (2011) 106(1):125–34. doi: 10.1007/s00395-010-0135-y
77. Mantani PT, Duner P, Bengtsson E, Alm R, Ljungcrantz I, Soderberg I, et al. IL-25 inhibits atherosclerosis development in apolipoprotein E deficient mice. PLoS One. (2015) 10(1):e0117255. doi: 10.1371/journal.pone.0117255
78. Omenetti S, Bussi C, Metidji A, Iseppon A, Lee S, Tolaini M, et al. The intestine harbors functionally distinct homeostatic tissue-resident and inflammatory Th17 cells. Immunity. (2019) 51(1):77–89.e6. doi: 10.1016/j.immuni.2019.05.004
79. Miossec P, Kolls JK. Targeting IL-17 and TH17 cells in chronic inflammation. Nat Rev Drug Discov. (2012) 11(10):763–76. doi: 10.1038/nrd3794
80. Farkas AM, Panea C, Goto Y, Nakato G, Galan-Diez M, Narushima S, et al. Induction of Th17 cells by segmented filamentous bacteria in the murine intestine. J Immunol Methods. (2015) 421:104–11. doi: 10.1016/j.jim.2015.03.020
81. Ivanov II, Atarashi K, Manel N, Brodie EL, Shima T, Karaoz U, et al. Induction of intestinal Th17 cells by segmented filamentous bacteria. Cell. (2009) 139(3):485–98. doi: 10.1016/j.cell.2009.09.033
82. Schnupf P, Gaboriau-Routhiau V, Gros M, Friedman R, Moya-Nilges M, Nigro G, et al. Growth and host interaction of mouse segmented filamentous bacteria in vitro. Nature. (2015) 520(7545):99–103. doi: 10.1038/nature14027
83. Huang Y, Tang J, Cai Z, Zhou K, Chang L, Bai Y, et al. Prevotella induces the production of Th17 cells in the colon of mice. J Immunol Res. (2020) 2020:9607328. doi: 10.1155/2020/9607328
84. Park J, Kim M, Kang SG, Jannasch AH, Cooper B, Patterson J, et al. Short-chain fatty acids induce both effector and regulatory T cells by suppression of histone deacetylases and regulation of the mTOR-S6 K pathway. Mucosal Immunol. (2015) 8(1):80–93. doi: 10.1038/mi.2014.44
85. Chen PB, Black AS, Sobel AL, Zhao Y, Mukherjee P, Molparia B, et al. Directed remodeling of the mouse gut microbiome inhibits the development of atherosclerosis. Nat Biotechnol. (2020) 38(11):1288–97. doi: 10.1038/s41587-020-0549-5
86. Crotty S. T follicular helper cell differentiation, function, and roles in disease. Immunity. (2014) 41(4):529–42. doi: 10.1016/j.immuni.2014.10.004
87. Perruzza L, Gargari G, Proietti M, Fosso B, D'Erchia AM, Faliti CE, et al. T follicular helper cells promote a beneficial gut ecosystem for host metabolic homeostasis by sensing microbiota-derived extracellular ATP. Cell Rep. (2017) 18(11):2566–75. doi: 10.1016/j.celrep.2017.02.061
88. Kawamoto S, Tran TH, Maruya M, Suzuki K, Doi Y, Tsutsui Y, et al. The inhibitory receptor PD-1 regulates IgA selection and bacterial composition in the gut. Science. (2012) 336(6080):485–9. doi: 10.1126/science.1217718
89. Kubinak JL, Petersen C, Stephens WZ, Soto R, Bake E, O'Connell RM, et al. Myd88 signaling in T cells directs IgA-mediated control of the microbiota to promote health. Cell Host Microbe. (2015) 17(2):153–63. doi: 10.1016/j.chom.2014.12.009
90. Teng F, Klinger CN, Felix KM, Bradley CP, Wu E, Tran NL, et al. Gut microbiota drive autoimmune arthritis by promoting differentiation and migration of peyer’s patch T follicular helper cells. Immunity. (2016) 44(4):875–88. doi: 10.1016/j.immuni.2016.03.013
91. Foks AC, Lichtman AH, Kuiper J. Treating atherosclerosis with regulatory T cells. Arterioscler Thromb Vasc Biol. (2015) 35(2):280–7. doi: 10.1161/ATVBAHA.114.303568
92. Campbell DJ. Control of regulatory T cell migration, function, and homeostasis. J Immunol. (2015) 195(6):2507–13. doi: 10.4049/jimmunol.1500801
93. Wolf D, Gerhardt T, Winkels H, Michel NA, Pramod AB, Ghosheh Y, et al. Pathogenic autoimmunity in atherosclerosis evolves from initially protective apolipoprotein B(100)-reactive CD4(+) T-regulatory cells. Circulation. (2020) 142(13):1279–93. doi: 10.1161/CIRCULATIONAHA.119.042863
94. Gaddis DE, Padgett LE, Wu R, McSkimming C, Romines V, Taylor AM, et al. Apolipoprotein AI prevents regulatory to follicular helper T cell switching during atherosclerosis. Nat Commun. (2018) 9(1):1095. doi: 10.1038/s41467-018-03493-5
95. Ait-Oufella H, Salomon BL, Potteaux S, Robertson AK, Gourdy P, Zoll J, et al. Natural regulatory T cells control the development of atherosclerosis in mice. Nat Med. (2006) 12(2):178–80. doi: 10.1038/nm1343
96. Klingenberg R, Gerdes N, Badeau RM, Gistera A, Strodthoff D, Ketelhuth DF, et al. Depletion of FOXP3+regulatory T cells promotes hypercholesterolemia and atherosclerosis. J Clin Invest. (2013) 123(3):1323–34. doi: 10.1172/JCI63891
97. Zhao TX, Sriranjan RS, Tuong ZK, Lu Y, Sage AP, Nus M, et al. Regulatory T-cell response to low-dose interleukin-2 in ischemic heart disease. NEJM Evid. (2022) 1(1):EVIDoa2100009. doi: 10.1056/EVIDoa2100009
98. Sriranjan R, Zhao TX, Tarkin J, Hubsch A, Helmy J, Vamvaka E, et al. Low-dose interleukin 2 for the reduction of vascular inflammation in acute coronary syndromes (IVORY): protocol and study rationale for a randomised, double-blind, placebo-controlled, phase II clinical trial. BMJ Open. (2022) 12(10):e062602. doi: 10.1136/bmjopen-2022-062602
99. Tchitchek N, Nguekap Tchoumba O, Pires G, Dandou S, Campagne J, Churlaud G, et al. Low-dose IL-2 shapes a tolerogenic gut microbiota that improves autoimmunity and gut inflammation. JCI Insight. (2022) 7(17):e159406. doi: 10.1172/jci.insight.159406
100. Smith PM, Howitt MR, Panikov N, Michaud M, Gallini CA, Bohlooly YM, et al. The microbial metabolites, short-chain fatty acids, regulate colonic treg cell homeostasis. Science. (2013) 341(6145):569–73. doi: 10.1126/science.1241165
101. Arpaia N, Campbell C, Fan X, Dikiy S, van der Veeken J, deRoos P, et al. Metabolites produced by commensal bacteria promote peripheral regulatory T-cell generation. Nature. (2013) 504(7480):451–5. doi: 10.1038/nature12726
102. Tanoue T, Morita S, Plichta DR, Skelly AN, Suda W, Sugiura Y, et al. A defined commensal consortium elicits CD8 T cells and anti-cancer immunity. Nature. (2019) 565(7741):600–5. doi: 10.1038/s41586-019-0878-z
103. Haghikia A, Zimmermann F, Schumann P, Jasina A, Roessler J, Schmidt D, et al. Propionate attenuates atherosclerosis by immune-dependent regulation of intestinal cholesterol metabolism. Eur Heart J. (2022) 43(6):518–33. doi: 10.1093/eurheartj/ehab644
104. Round JL, Mazmanian SK. Inducible Foxp3+regulatory T-cell development by a commensal bacterium of the intestinal microbiota. Proc Natl Acad Sci U S A. (2010) 107(27):12204–9. doi: 10.1073/pnas.0909122107
105. Verma R, Lee C, Jeun EJ, Yi J, Kim KS, Ghosh A, et al. Cell surface polysaccharides of Bifidobacterium bifidum induce the generation of Foxp3(+) regulatory T cells. Sci Immunol. (2018) 3(28):eaat6975. doi: 10.1126/sciimmunol.aat6975
106. Wang K, Dong H, Qi Y, Pei Z, Yi S, Yang X, et al. Lactobacillus casei regulates differentiation of Th17/treg cells to reduce intestinal inflammation in mice. Can J Vet Res. (2017) 81(2):122–8.28408780
107. Liu Y, Fatheree NY, Dingle BM, Tran DQ, Rhoads JM. Lactobacillus reuteri DSM 17938 changes the frequency of Foxp3+regulatory T cells in the intestine and mesenteric lymph node in experimental necrotizing enterocolitis. PLoS One. (2013) 8(2):e56547. doi: 10.1371/journal.pone.0056547
108. Bernard-Raichon L, Colom A, Monard SC, Namouchi A, Cescato M, Garnier H, et al. A pulmonary lactobacillus murinus strain induces Th17 and RORgammat(+) regulatory T cells and reduces lung inflammation in tuberculosis. J Immunol. (2021) 207(7):1857–70. doi: 10.4049/jimmunol.2001044
109. Shah MM, Saio M, Yamashita H, Tanaka H, Takami T, Ezaki T, et al. Lactobacillus acidophilus strain L-92 induces CD4(+)CD25(+)Foxp3(+) regulatory T cells and suppresses allergic contact dermatitis. Biol Pharm Bull. (2012) 35(4):612–6. doi: 10.1248/bpb.35.612
110. Lichtman AH, Binder CJ, Tsimikas S, Witztum JL. Adaptive immunity in atherogenesis: new insights and therapeutic approaches. J Clin Invest. (2013) 123(1):27–36. doi: 10.1172/JCI63108
111. Kyaw T, Cui P, Tay C, Kanellakis P, Hosseini H, Liu E, et al. BAFF receptor mAb treatment ameliorates development and progression of atherosclerosis in hyperlipidemic ApoE(-/-) mice. PLoS One. (2013) 8(4):e60430. doi: 10.1371/journal.pone.0060430
112. Kyaw T, Winship A, Tay C, Kanellakis P, Hosseini H, Cao A, et al. Cytotoxic and proinflammatory CD8+ T lymphocytes promote development of vulnerable atherosclerotic plaques in apoE-deficient mice. Circulation. (2013) 127(9):1028–39. doi: 10.1161/CIRCULATIONAHA.112.001347
113. Iqneibi S, Saigusa R, Khan A, Oliaeimotlagh M, Armstrong Suthahar SS, Kumar S, et al. Single cell transcriptomics reveals recent CD8 T cell receptor signaling in patients with coronary artery disease. Front Immunol. (2023) 14:1239148. doi: 10.3389/fimmu.2023.1239148
114. Smit V, de Mol J, Schaftenaar FH, Depuydt MAC, Postel RJ, Smeets D, et al. Single-cell profiling reveals age-associated immunity in atherosclerosis. Cardiovasc Res. (2023) 119(15):2508–21. doi: 10.1093/cvr/cvad099
115. Luu M, Weigand K, Wedi F, Breidenbend C, Leister H, Pautz S, et al. Regulation of the effector function of CD8(+) T cells by gut microbiota-derived metabolite butyrate. Sci Rep. (2018) 8(1):14430. doi: 10.1038/s41598-018-32860-x
116. Sivan A, Corrales L, Hubert N, Williams JB, Aquino-Michaels K, Earley ZM, et al. Commensal Bifidobacterium promotes antitumor immunity and facilitates anti-PD-L1 efficacy. Science. (2015) 350(6264):1084–9. doi: 10.1126/science.aac4255
117. Vetizou M, Pitt JM, Daillere R, Lepage P, Waldschmitt N, Flament C, et al. Anticancer immunotherapy by CTLA-4 blockade relies on the gut microbiota. Science. (2015) 350(6264):1079–84. doi: 10.1126/science.aad1329
118. Nastasi C, Fredholm S, Willerslev-Olsen A, Hansen M, Bonefeld CM, Geisler C, et al. Butyrate and propionate inhibit antigen-specific CD8(+) T cell activation by suppressing IL-12 production by antigen-presenting cells. Sci Rep. (2017) 7(1):14516. doi: 10.1038/s41598-017-15099-w
119. Pillai S, Cariappa A. The follicular versus marginal zone B lymphocyte cell fate decision. Nat Rev Immunol. (2009) 9(11):767–77. doi: 10.1038/nri2656
120. Tsiantoulas D, Bot I, Ozsvar-Kozma M, Goderle L, Perkmann T, Hartvigsen K, et al. Increased plasma IgE accelerate atherosclerosis in secreted IgM deficiency. Circ Res. (2017) 120(1):78–84. doi: 10.1161/CIRCRESAHA.116.309606
121. Kyaw T, Tay C, Khan A, Dumouchel V, Cao A, To K, et al. Conventional B2 B cell depletion ameliorates whereas its adoptive transfer aggravates atherosclerosis. J Immunol. (2010) 185(7):4410–9. doi: 10.4049/jimmunol.1000033
122. Nus M, Tsiantoulas D, Mallat Z. Plan B (-cell) in atherosclerosis. Eur J Pharmacol. (2017) 816:76–81. doi: 10.1016/j.ejphar.2017.09.002
123. Sage AP, Tsiantoulas D, Baker L, Harrison J, Masters L, Murphy D, et al. BAFF receptor deficiency reduces the development of atherosclerosis in mice–brief report. Arterioscler Thromb Vasc Biol. (2012) 32(7):1573–6. doi: 10.1161/ATVBAHA.111.244731
124. Peterson DA, McNulty NP, Guruge JL, Gordon JI. Iga response to symbiotic bacteria as a mediator of gut homeostasis. Cell Host Microbe. (2007) 2(5):328–39. doi: 10.1016/j.chom.2007.09.013
125. Sutherland DB, Suzuki K, Fagarasan S. Fostering of advanced mutualism with gut microbiota by immunoglobulin A. Immunol Rev. (2016) 270(1):20–31. doi: 10.1111/imr.12384
126. Ng KW, Hobbs A, Wichmann C, Victora GD, Donaldson GP. B cell responses to the gut microbiota. Adv Immunol. (2022) 155:95–131. doi: 10.1016/bs.ai.2022.08.003
127. Yu B, Wang L, Chu Y. Gut microbiota shape B cell in health and disease settings. J Leukoc Biol. (2021) 110(2):271–81. doi: 10.1002/JLB.1MR0321-660R
128. Hutchinson MA, Park HS, Zanotti KJ, Alvarez-Gonzalez J, Zhang J, Zhang L, et al. Auto-antibody production during experimental atherosclerosis in ApoE(-/-) mice. Front Immunol. (2021) 12:695220. doi: 10.3389/fimmu.2021.695220
129. Khan SR, Dalm V, Ikram MK, Peeters RP, van Hagen PM, Kavousi M, et al. The association of serum immunoglobulins with risk of cardiovascular disease and mortality: the Rotterdam study. J Clin Immunol. (2023) 43(4):769–79. doi: 10.1007/s10875-023-01433-7
130. Kawamoto S, Maruya M, Kato LM, Suda W, Atarashi K, Doi Y, et al. Foxp3(+) T cells regulate immunoglobulin a selection and facilitate diversification of bacterial species responsible for immune homeostasis. Immunity. (2014) 41(1):152–65. doi: 10.1016/j.immuni.2014.05.016
131. Chen L, Ishigami T, Nakashima-Sasaki R, Kino T, Doi H, Minegishi S, et al. Commensal microbe-specific activation of B2 cell subsets contributes to atherosclerosis development independently of lipid metabolism. EBioMedicine. (2016) 13:237–47. doi: 10.1016/j.ebiom.2016.10.030
132. Weller S, Reynaud CA, Weill JC. Splenic marginal zone B cells in humans: where do they mutate their ig receptor? Eur J Immunol. (2005) 35(10):2789–92. doi: 10.1002/eji.200535446
133. Wei B, Su TT, Dalwadi H, Stephan RP, Fujiwara D, Huang TT, et al. Resident enteric microbiota and CD8+ T cells shape the abundance of marginal zone B cells. Eur J Immunol. (2008) 38(12):3411–25. doi: 10.1002/eji.200838432
134. Weller S, Sterlin D, Fadeev T, Coignard E, Verge de Los Aires A, Goetz C, et al. T-independent responses to polysaccharides in humans mobilize marginal zone B cells prediversified against gut bacterial antigens. Sci Immunol. (2023) 8(79):eade1413. doi: 10.1126/sciimmunol.ade1413
135. Tull TJ, Pitcher MJ, Guesdon W, Siu JHY, Lebrero-Fernandez C, Zhao Y, et al. Human marginal zone B cell development from early T2 progenitors. J Exp Med. (2021) 218(4):e20202001. doi: 10.1084/jem.20202001
136. Puga I, Cols M, Barra CM, He B, Cassis L, Gentile M, et al. B cell-helper neutrophils stimulate the diversification and production of immunoglobulin in the marginal zone of the spleen. Nat Immunol. (2012) 13(2):170–80. doi: 10.1038/ni.2194
137. Rohrbeck L, Adori M, Wang S, He C, Tibbitt CA, Chernyshev M, et al. GPR43 REGULATES marginal zone B-cell responses to foreign and endogenous antigens. Immunol Cell Biol. (2021) 99(2):234–43. doi: 10.1111/imcb.12399
138. Suo C, Dann E, Goh I, Jardine L, Kleshchevnikov V, Park JE, et al. Mapping the developing human immune system across organs. Science. (2022) 376(6597):eabo0510. doi: 10.1126/science.abo0510
139. Rosenfeld SM, Perry HM, Gonen A, Prohaska TA, Srikakulapu P, Grewal S, et al. B-1b cells secrete atheroprotective IgM and attenuate atherosclerosis. Circ Res. (2015) 117(3):e28–39. doi: 10.1161/CIRCRESAHA.117.306044
140. Thurnheer MC, Zuercher AW, Cebra JJ, Bos NA. B1 cells contribute to serum IgM, but not to intestinal IgA, production in gnotobiotic ig allotype chimeric mice. J Immunol. (2003) 170(9):4564–71. doi: 10.4049/jimmunol.170.9.4564
141. Kearney JF, McCarthy MT, Stohrer R, Benjamin WH Jr., Briles DE. Induction of germ-line anti-alpha 1-3 dextran antibody responses in mice by members of the Enterobacteriaceae family. J Immunol. (1985) 135(5):3468–72. doi: 10.4049/jimmunol.135.5.3468
142. New JS, Dizon BLP, King RG, Greenspan NS, Kearney JF. B-1 B cell-derived natural antibodies against N-acetyl-d-glucosamine suppress autoimmune diabetes pathogenesis. J Immunol. (2023) 211(9):1320–31. doi: 10.4049/jimmunol.2300264
143. Bodogai M, O'Connell J, Kim K, Kim Y, Moritoh K, Chen C, et al. Commensal bacteria contribute to insulin resistance in aging by activating innate B1a cells. Sci Transl Med. (2018) 10(467):eaat4271. doi: 10.1126/scitranslmed.aat4271
144. Rosser EC, Mauri C. Regulatory B cells: origin, phenotype, and function. Immunity. (2015) 42(4):607–12. doi: 10.1016/j.immuni.2015.04.005
145. Strom AC, Cross AJ, Cole JE, Blair PA, Leib C, Goddard ME, et al. B regulatory cells are increased in hypercholesterolaemic mice and protect from lesion development via IL-10. Thromb Haemost. (2015) 114(4):835–47. doi: 10.1160/TH14-12-1084
146. Sage AP, Nus M, Baker LL, Finigan AJ, Masters LM, Mallat Z. Regulatory B cell-specific interleukin-10 is dispensable for atherosclerosis development in mice. Arterioscler Thromb Vasc Biol. (2015) 35(8):1770–3. doi: 10.1161/ATVBAHA.115.305568
147. Rosser EC, Oleinika K, Tonon S, Doyle R, Bosma A, Carter NA, et al. Regulatory B cells are induced by gut microbiota-driven interleukin-1beta and interleukin-6 production. Nat Med. (2014) 20(11):1334–9. doi: 10.1038/nm.3680
148. Mu Q, Edwards MR, Swartwout BK, Cabana Puig X, Mao J, Zhu J, et al. Gut microbiota and bacterial DNA suppress autoimmunity by stimulating regulatory B cells in a murine model of lupus. Front Immunol. (2020) 11:593353. doi: 10.3389/fimmu.2020.593353
149. Ramakrishna C, Kujawski M, Chu H, Li L, Mazmanian SK, Cantin EM. Bacteroides fragilis polysaccharide A induces IL-10 secreting B and T cells that prevent viral encephalitis. Nat Commun. (2019) 10(1):2153. doi: 10.1038/s41467-019-09884-6
150. Alhabbab R, Blair P, Elgueta R, Stolarczyk E, Marks E, Becker PD, et al. Diversity of gut microflora is required for the generation of B cell with regulatory properties in a skin graft model. Sci Rep. (2015) 5:11554. doi: 10.1038/srep11554
151. Gerritsen J, Smidt H, Rijkers GT, de Vos WM. Intestinal microbiota in human health and disease: the impact of probiotics. Genes Nutr. (2011) 6(3):209–40. doi: 10.1007/s12263-011-0229-7
152. Zucko J, Starcevic A, Diminic J, Oros D, Mortazavian AM, Putnik P. Probiotic - friend or foe? Curr Opin Food Sci. (2020) 32:45–9. doi: 10.1016/j.cofs.2020.01.007
154. Ding KF, Petricoin EF, Finlay D, Yin H, Hendricks WPD, Sereduk C, et al. Nonlinear mixed effects dose response modeling in high throughput drug screens: application to melanoma cell line analysis. Oncotarget. (2018) 9(4):5044–57. doi: 10.18632/oncotarget.23495
155. Cho YA, Kim J. Effect of probiotics on blood lipid concentrations: a meta-analysis of randomized controlled trials. Medicine (Baltimore). (2015) 94(43):e1714. doi: 10.1097/MD.0000000000001714
156. Shimizu M, Hashiguchi M, Shiga T, Tamura HO, Mochizuki M. Meta-analysis: effects of probiotic supplementation on lipid profiles in normal to mildly hypercholesterolemic individuals. PLoS One. (2015) 10(10):e0139795. doi: 10.1371/journal.pone.0139795
157. Li J, Lin S, Vanhoutte PM, Woo CW, Xu A. Akkermansia muciniphila protects against atherosclerosis by preventing metabolic endotoxemia-induced inflammation in apoe-/- mice. Circulation. (2016) 133(24):2434–46. doi: 10.1161/CIRCULATIONAHA.115.019645
158. Katiraei S, de Vries MR, Costain AH, Thiem K, Hoving LR, van Diepen JA, et al. Akkermansia muciniphila exerts lipid-lowering and immunomodulatory effects without affecting neointima formation in hyperlipidemic APOE*3-Leiden.CETP mice. Mol Nutr Food Res. (2020) 64(15):e1900732. doi: 10.1002/mnfr.201900732
159. Gibson GR, Probert HM, Loo JV, Rastall RA, Roberfroid MB. Dietary modulation of the human colonic microbiota: updating the concept of prebiotics. Nutr Res Rev. (2004) 17(2):259–75. doi: 10.1079/NRR200479
160. Colantonio AG, Werner SL, Brown M. The effects of prebiotics and substances with prebiotic properties on metabolic and inflammatory biomarkers in individuals with type 2 diabetes Mellitus: a systematic review. J Acad Nutr Diet. (2020) 120(4):587–607.e2. doi: 10.1016/j.jand.2018.12.013
161. Farias DD, de Araujo FF, Neri-Numa IA, Pastore GM. Prebiotics: trends in food, health and technological applications. Trends Food Sci Tech. (2019) 93:23–35. doi: 10.1016/j.tifs.2019.09.004
162. Wang C, Deng H, Liu F, Yin Q, Xia L. Role of gut microbiota in the immunopathology of atherosclerosis: focus on immune cells. Scand J Immunol. (2022) 96(1):e13174. doi: 10.1111/sji.13174
163. Mithul Aravind S, Wichienchot S, Tsao R, Ramakrishnan S, Chakkaravarthi S. Role of dietary polyphenols on gut microbiota, their metabolites and health benefits. Food Res Int. (2021) 142:110189. doi: 10.1016/j.foodres.2021.110189
164. Morais CA, de Rosso VV, Estadella D, Pisani LP. Anthocyanins as inflammatory modulators and the role of the gut microbiota. J Nutr Biochem. (2016) 33:1–7. doi: 10.1016/j.jnutbio.2015.11.008
165. Liao ZL, Zeng BH, Wang W, Li GH, Wu F, Wang L, et al. Impact of the consumption of tea polyphenols on early atherosclerotic lesion formation and intestinal bifidobacteria in high-fat-fed ApoE(-/-) mice. Front Nutr. (2016) 3:42. doi: 10.3389/fnut.2016.00042
166. Behl T, Bungau S, Kumar K, Zengin G, Khan F, Kumar A, et al. Pleotropic effects of polyphenols in cardiovascular system. Biomed Pharmacother. (2020) 130:110714. doi: 10.1016/j.biopha.2020.110714
167. Guasch-Ferre M, Hu FB, Martinez-Gonzalez MA, Fito M, Bullo M, Estruch R, et al. Olive oil intake and risk of cardiovascular disease and mortality in the PREDIMED study. BMC Med. (2014) 12:78. doi: 10.1186/1741-7015-12-78
168. Sun Y, Zimmermann D, De Castro CA, Actis-Goretta L. Dose-response relationship between cocoa flavanols and human endothelial function: a systematic review and meta-analysis of randomized trials. Food Funct. (2019) 10(10):6322–30. doi: 10.1039/C9FO01747J
169. Wang L, Zeng B, Liu Z, Liao Z, Zhong Q, Gu L, et al. Green tea polyphenols modulate colonic microbiota diversity and lipid metabolism in high-fat diet treated HFA mice. J Food Sci. (2018) 83(3):864–73. doi: 10.1111/1750-3841.14058
170. Gonzalez-Sarrias A, Romo-Vaquero M, Garcia-Villalba R, Cortes-Martin A, Selma MV, Espin JC. The endotoxemia marker lipopolysaccharide-binding protein is reduced in overweight-obese subjects consuming pomegranate extract by modulating the gut Microbiota: a randomized clinical trial. Mol Nutr Food Res. (2018) 62(11):e1800160. doi: 10.1002/mnfr.201800160
171. Zhu L, Zhang D, Zhu H, Zhu J, Weng S, Dong L, et al. Berberine treatment increases Akkermansia in the gut and improves high-fat diet-induced atherosclerosis in apoe(-/-) mice. Atherosclerosis. (2018) 268:117–26. doi: 10.1016/j.atherosclerosis.2017.11.023
172. She J, Tuerhongjiang G, Guo M, Liu J, Hao X, Guo L, et al. Statins aggravate insulin resistance nthrough reduced blood glucagon-like peptide-1 levels in a microbiota-dependent manner. Cell Metab. (2024) 36(2):408–421.e5. doi: 10.1016/j.cmet.2023.12.027
173. Chen P, Li K. Statin Therapy ang gut microbiota. in Statins - From Lipid-lowering benefits to Pleiotropic Effects. London: IntecOpen (2023).
174. Bartolomaeus H, Balogh A, Yakoub M, Homann S, Marko L, Hoges S, et al. Short-chain fatty acid propionate protects from hypertensive cardiovascular damage. Circulation. (2019) 139(11):1407–21. doi: 10.1161/CIRCULATIONAHA.118.036652
175. Antushevich H. Fecal microbiota transplantation in disease therapy. Clin Chim Acta. (2020) 503:90–8. doi: 10.1016/j.cca.2019.12.010
176. Hanssen NMJ, de Vos WM, Nieuwdorp M. Fecal microbiota transplantation in human metabolic diseases: from a murky past to a bright future? Cell Metab. (2021) 33(6):1098–110. doi: 10.1016/j.cmet.2021.05.005
177. Brandsma E, Kloosterhuis NJ, Koster M, Dekker DC, Gijbels MJJ, van der Velden S, et al. A proinflammatory gut microbiota increases systemic inflammation and accelerates atherosclerosis. Circ Res. (2019) 124(1):94–100. doi: 10.1161/CIRCRESAHA.118.313234
178. Kim ES, Yoon BH, Lee SM, Choi M, Kim EH, Lee BW, et al. Fecal microbiota transplantation ameliorates atherosclerosis in mice with C1q/TNF-related protein 9 genetic deficiency. Exp Mol Med. (2022) 54(2):103–14. doi: 10.1038/s12276-022-00728-w
179. Smits LP, Kootte RS, Levin E, Prodan A, Fuentes S, Zoetendal EG, et al. Effect of vegan fecal microbiota transplantation on carnitine- and choline-derived trimethylamine-N-oxide production and vascular inflammation in patients with metabolic syndrome. J Am Heart Assoc. (2018) 7(7). doi: 10.1161/JAHA.117.008342
180. Wu L, Lu XJ, Lin DJ, Chen WJ, Xue XY, Liu T, et al. Washed microbiota transplantation improves patients with metabolic syndrome in south China. Front Cell Infect Microbiol. (2022) 12:1044957. doi: 10.3389/fcimb.2022.1044957
181. Saita D, Ferrarese R, Foglieni C, Esposito A, Canu T, Perani L, et al. Adaptive immunity against gut microbiota enhances apoE-mediated immune regulation and reduces atherosclerosis and western-diet-related inflammation. Sci Rep. (2016) 6:29353. doi: 10.1038/srep29353
182. Canducci F, Saita D, Foglieni C, Piscopiello MR, Chiesa R, Colombo A, et al. Cross-reacting antibacterial auto-antibodies are produced within coronary atherosclerotic plaques of acute coronary syndrome patients. PLoS One. (2012) 7(8):e42283. doi: 10.1371/journal.pone.0042283
183. Binder CJ, Horkko S, Dewan A, Chang MK, Kieu EP, Goodyear CS, et al. Pneumococcal vaccination decreases atherosclerotic lesion formation: molecular mimicry between Streptococcus pneumoniae and oxidized LDL. Nat Med. (2003) 9(6):736–43. doi: 10.1038/nm876
184. Binder CJ, Papac-Milicevic N, Witztum JL. Innate sensing of oxidation-specific epitopes in health and disease. Nat Rev Immunol. (2016) 16(8):485–97. doi: 10.1038/nri.2016.63
185. Que X, Hung MY, Yeang C, Gonen A, Prohaska TA, Sun X, et al. Oxidized phospholipids are proinflammatory and proatherogenic in hypercholesterolaemic mice. Nature. (2018) 558(7709):301–6. doi: 10.1038/s41586-018-0198-8
186. Sun X, Seidman JS, Zhao P, Troutman TD, Spann NJ, Que X, et al. Neutralization of oxidized phospholipids ameliorates non-alcoholic steatohepatitis. Cell Metab. (2020) 31(1):189–206.e8. doi: 10.1016/j.cmet.2019.10.014
187. Eurich DT, Johnstone JJ, Minhas-Sandhu JK, Marrie TJ, Majumdar SR. Pneumococcal vaccination and risk of acute coronary syndromes in patients with pneumonia: population-based cohort study. Heart. (2012) 98(14):1072–7. doi: 10.1136/heartjnl-2012-301743
188. Lamontagne F, Garant MP, Carvalho JC, Lanthier L, Smieja M, Pilon D. Pneumococcal vaccination and risk of myocardial infarction. CMAJ. (2008) 179(8):773–7. doi: 10.1503/cmaj.070221
189. Grievink HW, Gal P, Ozsvar Kozma M, Klaassen ES, Kuiper J, Burggraaf J, et al. The effect of a 13-valent conjugate pneumococcal vaccine on circulating antibodies against oxidized LDL and phosphorylcholine in man, A randomized placebo-controlled clinical trial. Biology (Basel). (2020) 9(11):345. doi: 10.3390/biology9110345
190. Ochoa-Gondar O, Vila-Corcoles A, Rodriguez-Blanco T, de Diego-Cabanes C, Hospital-Guardiola I, Jariod-Pamies M, et al. Evaluating the clinical effectiveness of pneumococcal vaccination in preventing myocardial infarction: the CAPAMIS study, three-year follow-up. Vaccine. (2014) 32(2):252–7. doi: 10.1016/j.vaccine.2013.11.017
191. Tseng HF, Slezak JM, Quinn VP, Sy LS, Van den Eeden SK, Jacobsen SJ. Pneumococcal vaccination and risk of acute myocardial infarction and stroke in men. JAMA. (2010) 303(17):1699–706. doi: 10.1001/jama.2010.529
192. Ren S, Newby D, Li SC, Walkom E, Miller P, Hure A, et al. Effect of the adult pneumococcal polysaccharide vaccine on cardiovascular disease: a systematic review and meta-analysis. Open Heart. (2015) 2(1):e000247. doi: 10.1136/openhrt-2015-000247
193. Ren S, Hansbro PM, Srikusalanukul W, Horvat JC, Hunter T, Brown AC, et al. Generation of cardio-protective antibodies after pneumococcal polysaccharide vaccine: early results from a randomised controlled trial. Atherosclerosis. (2022) 346:68–74. doi: 10.1016/j.atherosclerosis.2022.02.011
194. Attia J, Horvat JC, Hunter T, Hansbro PM, Hure A, Peel R, et al. Persistence of detectable anti-pneumococcal antibodies 4 years after pneumococcal polysaccharide vaccination in a randomised controlled trial: the Australian study for the prevention through immunisation of cardiovascular events (AUSPICE). Heart Lung Circ. (2023) 32(11):1378–85. doi: 10.1016/j.hlc.2023.09.006
195. Hayashi C, Viereck J, Hua N, Phinikaridou A, Madrigal AG, Gibson FC 3rd, et al. Porphyromonas gingivalis accelerates inflammatory atherosclerosis in the innominate artery of ApoE deficient mice. Atherosclerosis. (2011) 215(1):52–9. doi: 10.1016/j.atherosclerosis.2010.12.009
196. Miyamoto T, Yumoto H, Takahashi Y, Davey M, Gibson FC, Genco CA. Pathogen-accelerated atherosclerosis occurs early after exposure and can be prevented via immunization. Infect Immun. (2006) 74(2):1376–80. doi: 10.1128/IAI.74.2.1376-1380.2006
197. Koizumi Y, Kurita-Ochiai T, Oguchi S, Yamamoto M. Nasal immunization with Porphyromonas gingivalis outer membrane protein decreases P. gingivalis-induced atherosclerosis and inflammation in spontaneously hyperlipidemic mice. Infect Immun. (2008) 76(7):2958–65. doi: 10.1128/IAI.01572-07
Keywords: atherosclerosis, gut microbiota, T cells, B cells, metabolites
Citation: Giakomidi D, Ishola A and Nus M (2025) Targeting gut microbiota to regulate the adaptive immune response in atherosclerosis. Front. Cardiovasc. Med. 12:1502124. doi: 10.3389/fcvm.2025.1502124
Received: 26 September 2024; Accepted: 20 January 2025;
Published: 31 January 2025.
Edited by:
Jürgen Bernhagen, Ludwig Maximilian University of Munich, GermanyReviewed by:
Ashraf Yusuf Rangrez, University of Kiel, GermanyKazuyuki Kasahara, Nanyang Technological University, Singapore
Copyright: © 2025 Giakomidi, Ishola and Nus. This is an open-access article distributed under the terms of the Creative Commons Attribution License (CC BY). The use, distribution or reproduction in other forums is permitted, provided the original author(s) and the copyright owner(s) are credited and that the original publication in this journal is cited, in accordance with accepted academic practice. No use, distribution or reproduction is permitted which does not comply with these terms.
*Correspondence: Meritxell Nus, bW40MjFAY2FtLmFjLnVr
†ORCID:
Despina Giakomidi
orcid.org/0000-0003-1538-7175
Meritxell Nus
orcid.org/0000-0002-6378-8910