- Department of Cardiothoracic Surgery, Affiliated Hospital and Medical School of Nantong University, Nantong, China
Adipose-derived mesenchymal stem cells (ASCs) represent an innovative candidate to treat ischemic heart disease (IHD) due to their abundance, renewable sources, minor invasiveness to obtain, and no ethical limitations. Compared with other mesenchymal stem cells, ASCs have demonstrated great advantages, especially in the commercialization of stem cell-based therapy. Mechanistically, ASCs exert a cardioprotective effect not only through differentiation into functional cells but also via robust paracrine of various bioactive factors that promote angiogenesis and immunomodulation. Exosomes from ASCs also play an indispensable role in this process. However, due to the distinct biological functions of ASCs from different origins or donors with varing health statuses (such as aging, diabetes, or atherosclerosis), the heterogeneity of ASCs deserves more attention. This prompts scientists to select optimal donors for clinical applications. In addition, to overcome the primary obstacle of poor retention and low survival after transplantation, a variety of studies have been dedicated to the engineering of ASCs with biomaterials. Besides, clinical trials have confirmed the safety and efficacy of ASCs therapy in the context of heart failure or myocardial infarction. This article reviews the theory, efficacy, and advantages of ASCs-based therapy, the factors affecting ASCs function, heterogeneity, engineering strategies and clinical application of ASCs.
Introduction
Ischemic heart disease (IHD), the most prevalent cardiovascular disease, is the culprit in majority of acute heart events, and remains the leading cause of death globally (1). This condition arises from the stenosis and blockage of coronary arteries, which inevitably leads to a decreased blood supply to the heart, resulting in irreversible damage to myocardium filling with necrotic cardiomyocytes. Significant decline in cardiac function develops, progresses to heart failure and death ultimately. Despite remarkable progress in drug development and advancements in interventional and surgical treatments over the centuries, neither of them can reverse the myocardial necrosis caused by extended periods of hypoxia. Given the limited regenerative capacity of cardiovascular tissue after injury in mammals, stem cells have emerged as a promising strategy for treating IHD.
Mesenchymal stem cells (MSCs) are a subset of stem cell family that reside in virtually all tissues with specific stem cell niches in the human body (2). MSCs can be obtained from various depots, including bone marrow (BM-MSCs), umbilical cord (UC-MSCs), adipose tissue. Among them, adipose-derived mesenchymal stem cells (ASCs) are particularly appealing due to their high accessibility, minimally invasive harvesting, high stem cell density, low immunogenicity, and no ethical restrictions (3). In this review, we highlight the advantages of ASCs-based therapy compared to other MSCs and explore the heterogeneity of ASCs. We focus on the therapeutic potential of ASCs in treating IHD through differentiation into functional cells, puissant paracrine that facilitates immunomodulation and angiogenesis, as well as engineering strategies and current clinical applications of ASCs.
What are the advantages of ASCs compared with BM-MSCs and UC-MSCs?
Besides readily access and high yield, ASCs present some unique biological features compared to BM-MSCs and UC-MSCs. Table 1 summarizes the differences among these three types of MSCs. Specifically, to exclude the impact of donor health status, origin of MSCs, and culture strategy on the growth profile and senescence of MSCs, researchers isolated BMSCs and ASCs from the same donor. Their findings suggested that compared with BM-MSCs, ASCs displayed faster proliferation, shorter doubling time, and postponed senescence featured with longer telomere and lower expression of p16ink4a (a characteristic gene of senescence) (4). Intriguingly, ASCs exhibited preferential adipogenesis, while BMSCs retained superior osteogenesis, which might be related to their distinct origin (5–9). Furthermore, ASCs have shown enhanced improvement in wound healing compared to their bone marrow-treated counterparts, suggesting a superior paracrine potential (10). In addition, higher concentration of cytokines including interlukin-6 (IL-6) and transforming growth factor-β (TGF-β) was observed in the supernatant of ASCs compared to BM-MSCs (11). ASCs also exerted potent immunosuppressive effects on T cells and DCs, along with upregulation of indoleamine 2,3-dioxygenase (IDO), a marker of MSCs immunosuppression on mononuclear cells (11–13), insinuating a stronger immunosuppressive capacity.
Meanwhile, it is undeniable that the accessibility, high yield, and cultural expandability of cell candidates in vitro are crucial for successful cell-based therapy. In this respect, ASCs offer several advantages, including abundant sources, efficient scalability, low immunogenicity, and powerful immunosuppressive capacity. These features make ASCs suitable for both autologous and allogeneic transplantation and have exhibited exciting prospects for the commercialization of stem cell-based therapy.
How does it work: the mechanism of ASCs treatment for IHD?
The views on the mechanisms behind the use of ASCs in the treatment of IHD continues to evolve over time. These developing theories will undoubtedly drive the better administration of ASCs. As illustrated in Figure 1, ASCs possess competent differentiation and paracrine potential, enabling them to effectively treat IHD from multiple aspects.
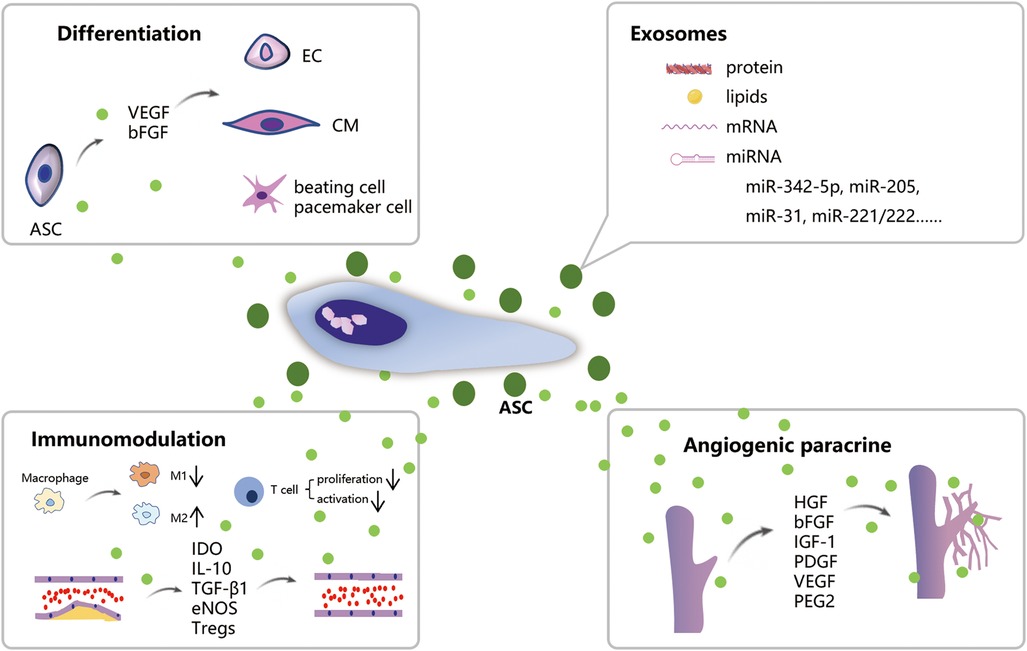
Figure 1. Schematic illustration of the mechanism of ASCs treatment for IHD. ASCs can differentiate into CMs, ECs, and beating cells/pacemaker cells. ASCs own robust paracrine function which promotes angiogenesis and immunomodulation on macrophage polarization and atherosclerosis. Exosomes released from ASCs contain biologically active substances such as proteins, lipids, and mRNA which possess comparable therapeutic effects on IHD.
Is differentiation potential the primary mechanism behind ASCs-based therapy?
The differentiation of ASCs into other cell lines was once perceived as the key mechanism behind ASCs-based therapy. Beyond their fundamental trilineage potential in vitro, ASCs have been widely observed to differentiate into endothelial cells (ECs) and cardiomyocytes (CMs) under certain circumstances (14, 15). For instance, Kendra Clark, et al. observed the endothelial differentiation of ASCs when cultured in endothelial differentiation media. A specific 3D culture system enhanced this process, and a high concentration of vascular endothelial growth factor (VEGF) further augmented endothelial differentiation (16). Meanwhile, hypoxia treatment, which mimics the native physiological niche of ASCs, was found to facilitate the endothelial lineage differentiation of ASCs under stimulation with VEGF and bone morphogenetic protein-4 (BMP4) (17). Except VEGF, basic-fibroblast growth factor (bFGF) is another effective inducer of ECs differentiation from ASCs, with an induction rate exceeding 85% (18). Moreover, shear stress, which simulated the ECs environment in vivo, has been found to facilitate endothelial differentiation of ASCs and upregulate the expression of anti-thrombogenic markers (19). In animal models, plentiful human CD31-positive cells and regenerated blood vessels were observed in mouse hindlimbs injected with human stromal-vascular fraction (SVF), the origin of ASCs, suggesting that SVF cells have the potential to differentiate into endothelial cells and promote vascular regeneration directly (20). In terms of mechanism, miR-145 was identified as a key component in EC differentiation of ASCs, Upregulation of miR-145 suppressed the EC differentiation via regulating ETS1 expression, which can be reversed by overexpression of ETS1 (21). UTP is considered as another regulator of ASCs cardioprotective property in IHD, which not only enhances the revascularization in ischemic myocardium, but also directly promotes the endothelial differentiation of ASCs (22, 23).
On the other hand, although it seems more difficult for ASCs to differentiate into cardiomyocyte-like cells compared to endothelial cells, there are still numerous studies that have discovered this differentiation potential of ASCs. For instance, in 2015, HIROKI, et al. have found ASCs derived from cardiac adipose tissue could directly differentiate into cTnT-positive cells in vivo, while ASCs from subcutaneous, visceral, and subscapular adipose tissue failed to differentiate into cardiomyocytes (15). This finding indirectly highlights the challenges associated with cardiomyocyte differentiation of ASCs. To effectively induce ASC differentiation into cardiomyocytes, Zhang and his colleagues designed a type of gelatin/polycaprolactone fibers which promoted cardiomyocyte differentiation and facilitated ASCs proliferation as well (24). Wang, et al. discovered that chitosan can facilitate cardiac differentiation of ASCs through enhancing the collagen synthesis (25). They developed an injectable chitosan hydrogel as a deliverer for ASCs, which not only enhanced the cardiomyocyte differentiation, but also improve the survival of ASCs in infarcted hearts. Furthermore, Yan, et al, cultured ASCs on a polylactic acid (PLA) nanopillar array, then observed distinct cardiomyocyte-like cell markers and the induced ASCs injected into the myocardium exhibited significant protective effects on ischemic myocardium (26). Therefore, the differentiation of ASCs into cardiomyocytes is not only possible, but also feasible and holds great promise.
Besides, ASCs possess the potential of differentiating into pacemaker cells, giving insight into the treatment of arrhythmia, one common complication of IHD (27). To achieve that, scientists added certain differentiation factors such as BMP4 into the culture medium (28), or transfected ASCs with specific genes closely related to sinoatrial node function, such as TBXs (29, 30).
Nevertheless, the statement that ASCs differentiate into functional cells is not rigorous. It seems more appropriate to call these differentiated cells “endothelial-like cells, cardiomyocyte-like cells, and pacemaker-like cells”. Meanwhile, ASCs implanted into the ischemic myocardium, either locally or systemically, will struggle with a harsh microenvironment characterized by hypoxia, elevated oxidative stress, free radical production, limited nutrient supply, and the presence of proinflammatory cytokines, along with infiltration of immune cells (31). As a result, only a few ASCs are retained within proximity to the graft site and effectively integrate into the affected host tissue (32). Therefore, it may be the other mechanism rather than differentiation capability dominates the ASCs-based therapy.
What is the role of proangiogenic paracrine of ASCs?
Since the implanted cells hardly survive in the tough soil in vivo, the potent paracrine of ASCs may be responsible for the compensatory angiogenesis in the ischemic area. Numerous studies have revealed that ASCs produced a variety of cytokines that promote angiogenesis, including VEGF, bFGF, hepatocyte growth factor (HGF), platelet-derived growth factor (PDGF), insulin-like growth factor-1 (IGF-1), et al. (33–36). Among these various angiogenic cytokines, VEGF is particularly notable due to its close relation to angiogenesis. In vitro studies have shown that ASCs generate high concentration of VEGF into the supernatant, especially when cocultured with human umbilical vein endothelial cells or endothelial progenitor cells (14, 37). Furthermore, under the hypoxic conditions which imitated the in vivo environment of IHD, ASCs secreted higher amounts of VEGF, HGF, and stromal-derived factor-1 (SDF-1) compared to normoxic conditions (38–40). The lesion-associated hypoxia, which curbed the survival of engrafted stem cells, would inevitably lead to the activation of hypoxia-inducible factor 1, resulting in increased VEGF release, its classical target gene (41). Additionally, pretreating ASCs with endothelial differentiation medium dramatically enhanced their proangiogenic action by increasing the amount of microvesicles released by ASCs (42). The underlying mechanism involves the transfer of microRNAs in microvesicles from ASCs to the vascular endothelial cells.
The potent proangiogenic function of ASCs has also been confirmed in vivo. In murine models of skin pressure ulcers and ischemic hindlimbs, injection of ASCs led to the formation of high-density capillary and branched tubelike structures, accompanied by accelerated recovery (43–45). Similarly, in a rat model of myocardial infarction (MI), administration of ASCs increased angiogenesis in the ischemic area, decreased infarct size, and improved heart function (46). Comparable results were observed in the swine model, which was closer to humans (47). Furthermore, a clinical study conducted in Japan utilized autologous ASCs for patients with limb ischemia. The application of ASCs significantly improved the clinical outcomes through angiogenesis without adverse events (48). Collectively, angiogenesis, driven by the robust paracrine action of ASCs, has been identified to play a primary role in the ASC-based IHD therapy.
How does ASCs achieve therapeutic action via immunomodulation?
Following an MI, the innate immune response is triggered, characterized by the recruitment and infiltration of massive inflammatory immune cells, such as monocytes-derived-M1 macrophages, which eventually transformed into anti-inflammatory M2 macrophages, accompanied by the release of various pro- and anti-inflammatory cytokines. Studies have shown that coculture of ASCs and macrophages in vitro significantly induced macrophages toward reparative M2 phenotype and altered their cytokine secretion (49). Additionally, ASCs-based therapy has been found to increase the percentage of M2 macrophages in both spontaneously hypertensive rats and ischemic cardiomyopathy models, leading to improved disease prognoses (50, 51), indicating the therapeutic potential of ASCs in modulating the innate immune system.
Importantly, ASCs transplantation has been extensively proposed as an effective approach to treat atherosclerosis (ATH), which underlies many vascular disorders, such as aneurysm, atherosclerosis obliterans, and IHD. The protective potency of ASCs on ATH is primarily attributed to their robust paracrine action, which involves the release of various bioactive factors, such as IDO, TGF-β1, and IL-10, along with decreased release of pro-inflammatory cytokines, including TNF-α and IL-1β (52, 53). IDO is widely considered to suppress the proliferation of T cell and NK cells, impede TH17 differentiation and DCs maturation (13, 54), while IL-10 blocks macrophage activation, disrupts the production of pro-inflammatory cytokines and matrix metalloproteinase (MMP), and represses T cell proliferation, thereby impacting the local inflammatory response within the lesion (55). Moreover, TGF-β1 is involved in the decrease of NK cells proliferation and the MSC-mediated induction of CD4 + CD25 + Foxp3+ regulatory T cells (Tregs) (53). Tregs then exert a protective effect by suppressing the function of Th1/Th2 cells and DCs and promoting the stability of atherosclerotic lesions by inhibiting the expression of MMP-2 and MMP-9, which are crucial in degrading extracellular matrix proteins (56). Intriguingly, T cell activation was significantly inhibited when cocultured with ASCs under hypoxia, manifested by the upregulation of anti-inflammatory cytokines including PDCD1, Foxp3, and TGFβ1, and downregulation of genes involved in pro-inflammatory response such as IL2 and IFNG (57). In animal models of ATH, ASCs transplantation dramatically reduced the total cholesterol, triglyceride, and low-density lipoprotein cholesterol levels, while increasing high-density lipoprotein cholesterol levels, and ameliorating the pathological status of aortic ATH (58). Therefore, ASCs exert a positive effect on IHD from the immunopathology.
ASC-derived exosomes and IHD
Exosomes are nano-vesicles secreted by cells into the extracellular environment, containing biologically active substances such as proteins, lipids, and mRNA. In recent years, ASC-derived exosomes have demonstrated similar therapeutic effects on IHD as ASCs. For instance, Xing, et, al. revealed ASCs-derived exosome delayed the development of ATH through a miR-342-5p mediated endothelial protection (59). In animal MI models, exosomes extracted from ASCs exerted therapeutic effect by promoting angiogenesis via miR-205 and miR-31 (60, 61), preventing cardiomyocyte apoptosis and hypertrophy via miR-221/222 (62–64), enhancing M2 macrophage polarization (65), ameliorating excessive ROS production, and attenuating cardiac fibrosis (66). To enhance the retention of exosomes in ischemic myocardium, Ankita and his colleagues constructed a polyurethane modified with antioxidant gallic acid (PUGA) and decellularized extracellular matrix dECM combined scaffold patch to deliver ASC-derived exosomes (67). Their results showed decreased fibrosis, promoted angiogenesis, reduced oxidative stress after application of patch, as well as improved cardiac function. Nowadays, due to their low immunogenicity, minimal tumorigenicity, and easy storage and transportation, exosomes have garnered increasing attention as a potential therapeutic tool for IHD.
Does the tissue type or origin of ASCs affect their biological function?
Mammalian adipose tissue comes in three types: white, brown, and beige (68). White adipose tissue (WAT), as the primary energy-storing organ and chief culprit of obesity, occurs in various locations, including intraabdominal and subcutaneous sites; brown adipose tissue (BAT) exists in cervical, axillary, periadrenal, and perirenal area in the fetus and newborn and then transforms into WAT with aging. Nowadays, emerging shreds of evidences have supported the presence of BAT in adults human, located in the supraclavicular, perirenal, and deep neck region, with a thermogenic function (69). Beige adipose tissue, also known as brite adipose tissue, is a phenotype that arises from the “browning” of WAT upon cold exposure (70). Currently, there is limited research on the differences between ASCs obtained from BAT and WAT, partly due to scarcity of BAT depots, especially in humans. However, studies have shown enhanced proliferation, differentiation, and paracrine potential of ASCs isolated from BAT in contrast to WAT. For instance, ASCs obtained from pericardial and thymic depots exhibited longer doubling time compared to those from the subcutaneous or intraperitoneal region (71). Moreover, ASCs obtained from BAT have demonstrated spontaneous differentiation into cardiomyocytes, which can be further accelerated by chitosan hydrogel (25).
Except for tissue type, the organ origin of adipose tissue also affects the characteristics of ASCs. ASCs derived from the epicardial fat of cardiac patients have been found to induce a superior angiogenic effect and produce higher amounts of angiogenic, trophic, and inflammatory cytokines compared with ASCs from subcutaneous fat, which can result in worse heart function after MI due to their proinflammatory properties (36). Furthermore, scientists have demonstrated that subcutaneous ASCs and ASCs from the intraabdominal region, both of which belong to WAT, develop different proliferation and adipogenic differentiation potential. ASCs from subcutaneous adipose tissue display an enhanced adipogenesis potential, while progenitor cells isolated from the infrapatellar fat pad express higher levels of chondrogenic markers (72). Therefore, considering the distinct biological behavior of ASCS from different origins, it is crucial to select suitable cell candidates for preclinical application.
Factors affecting the function of ASCs: aging, diabetes mellitus, and atherosclerosis
Currently, autologous transplantation remains the predominant strategy for stem cell-based therapy. However, the viability and quality of stem cells can be significantly affected by the general health of donors. In clinical settings, patients undergoing cell transplantation usually suffer from systemic pathologies such as hypertension, diabetes, or autoimmune disease. The heterogeneity of ASCs remarkably imposes restrictions on the efficacy of autologous transplantation, highlighting the importance of selecting the optimal donor for allotransplantation. Given that patients with IHD are typically elderly and often have diabetes or atherosclerosis, these three factors have been studied extensively as a matter of course. The factors that impact the function of ASCs are detailed below and summarized in the accompanying figures (Figure 2).
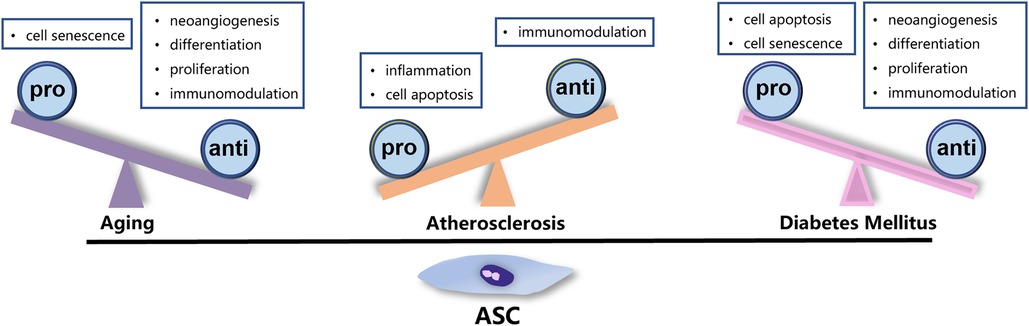
Figure 2. Factors affecting the function of ASCs. Aging, ATH, and DM exert negative impact on the characteristic and function of ASCs through different mechanisms.
Aging
While the decrease in the number of ASCs with increasing age remains controversial (73), there is no denying that aging exerts a negative impact on the biological feature of ASCs. Firstly, studies have shown that the proliferative rate of ASCs obtained from older animals or individuals declined dramatically (74, 75). Additionally, ASCs derived from older donors exhibited typical senescence phenotypes, including an increased percentage of G1/G0 phase-arrested cells, decreased telomere length, elevated β-galactosidase activity, and binucleation as well (76). Moreover, the differentiation capability of ASCs from older donors was reduced, although it might be rescued by different cell culture procedures (77). Furthermore, the decline in function of ASCs from older patients was evident in their reduced production of pro-angiogenic factors such as VEGF, HGF, PIGF, and ANG, as well as their inferior attenuation of CD4+ T cells proliferation, indicating an age-associated reduction in paracrine and immunomodulatory capacity (78). Given that MSCs, including ASCs, play a crucial role in repair after injury, the decreased function due to aging can be a significant pathogenic factor in age-related pathologies such as atherosclerosis, diabetes, and arterial hypertension.
Diabetes mellitus
In the microenvironment of patients with diabetes mellitus (DM), some subtle changes occurs, including hyperglycemia, excessive oxidative stress, mitochondrial dysfunction, proinflammatory cell status, and hypoxia, which inevitably impact ASCs to some extent (79, 80). Scientists have reported decreased proliferation, increased senescence and apoptosis, and downregulated VEGF expression in diabetic ASCs (81, 82), as well as impaired EC differentiation manifested by declined vWF and CD31 compared with healthy counterparts (83, 84). In addition to VEGF, diabetic ASCs secreted lower levels of angiogenic factors than healthy ASCs, including FGF, PDGF, SDF-1, osteopontin, insulin-like growth factor binding protein-3, and monocyte chemoattractant protein-1 (82). They failed to form tubular structures in Matrigel, and their potential of promoting angiogenesis was notably impaired (85). Consistent with in vitro study results, the degree of vascularization and wound healing in the mice wound model with implantation of diabetic ASCs was significantly reduced compared with that with nondiabetic ASCs (82, 86). Besides, the adipogenesis (84) and osteogenic differentiation (87) potential of diabetic ASCs also declined, indicating a loss of cell stemness in the hyperglycemic environment. It has been reported that hyperglycemia generates advanced glycation end products (AGEs), which induces osteoclast formation and apoptosis of osteoblasts (87). However, this effect may differ in humans, as ASCs from DM patients demonstrated robust osteoblast differentiation, indicating the complexity of ASCs biological behavior (88). Moreover, ASCs derived from DM patients exhibit an inflammatory phenotype, characterized by activation of NLRP3 inflammasome and subsequent alterations in immunomodulatory capacity (89). Furthermore, in pressure-ulcer model, mice treated with nondiabetic ASCs displayed less infiltration of inflammatory cells into the dermis and more new blood vessels in situ during the first 2 weeks compared with diabetic ASCs; however, these advantages disappeared afterward (43). The underlying mechanism of adverse effect of hyperglycemic microenvironment of DM on ASCs remains to be further explored.
Atherosclerosis
ASCs play a protective role in the development of ATH. In turn, ASCs from ATH subjects (ATH-ASCs) exhibit distinct features compared with those isolated from non-ATH donors. Scientists have found elevated intracellular reactive oxidative stress (ROS) and mitochondrial ROS in ATH-ASCs (90). ROS activates NF-κB as a secondary messenger, leading to the increased accumulation of HIF-1α and upregulated expression of pro-inflammatory cytokine and chemokine (91). The ROS scavenger N-acetyl-L-cysteine can reduce the secretion of these cytokines in ATH-ASCs, enhance their survival and immune potency. Additionally, patients with ATH displayed a higher level of CD4+ T cells activation compared to those without ATH, and ASCs from non-ATH patients demonstrated superior inhibition on proliferating CD4+ T cells (78). Therefore, it is evident that ATH significantly impairs the immunomodulatory function of ASCs and negatively affect their therapeutic efficiency. In this case, it is advisable to exclude ATH subjects from the selection of suitable donors for regenerative medicine.
Engineered ASCs
The primary obstacles to effective cell therapy include inadequate cell retention and low survival rates, which limit further clinical application. For decades, extensive studies have focused on encapsulation of ASCs within a variety of biomaterials to enhance their delivery and retention in ischemic myocardium. For instance, Follin, et al. (92) embedded human ASCs in an alginate hydrogel and reported no adverse effects on cell viability, phenotype, immunogenic properties, or paracrine activity. Similarly, the fullerenol/alginate hydrogel was found to dramatically scavenge the superoxide anions in ischemic area, thereby enhancing ASCs retention and survival, and ultimately promoting cardiac recovery in a rat MI model (93). Besides, chitosan hydrogel has been considered an ideal carrier due to its components facilitating cardiac differentiation of ASCs (25). In vivo studies have revealed enhanced survival of engrafted ASCs, increased generation of ASCs-derived cardiomyocytes, improved angiogenesis, and preserved cardiac healing. In addition to various hydrogels (94, 95), a growing number of biomaterials have also shown promise in enhancing cell retention and promoting cardiac repair, including Matrigel (96), collagen type-1 scaffold (97), superparamagnetic iron oxide nanoparticles (98), poly(lactic-co-glycolic acid) (PLGA) (99), decellularized pericardium (100), poly(ɛ-caprolactone-co-glycolic acid) and poly(ethylene glycol) (tri-PCG) (101), conductive electrospun nanofibers (102), injectable cryogels (103), et al. Biomaterials for ASCs delivery have demonstrated great potential in preclinical studies and provide guidance for their application in the treatment of MI in human clinical studies.
Clinical trials
Based on various in vitro and in vivo studies, ASCs transplantation has been widely utilized for IHD treatment in clinical trials. Quantities of clinical trials have been conducted to assess the safety, feasibility, and effectiveness of ASC in individuals with heart diseases (Table 2). However, due to the complexity and severity of IHD, the current clinical applications of ASCs in the field remain in the early phases (phage I/II).
The Netherlands clinical trial conducted by Jaco H. Houtgraaf and his colleagues in 2007 (NCT00442806) (104) was the first clinical trial using ASCs in IHD. This trial was a randomized, parallel assigned, double-blinded clinical trial for ST-segment elevation acute myocardial infarction. Totally 14 patients were enrolled and after 6 months of follow-up, the study uncovered that intracoronary injection of freshly isolated ASCs was safe and effective, with no adverse effect related to ASCs implantation, no decline of coronary blood flow, improved cardiac function, and reduction of scar formation. Besides, a recent 3-year follow-up MyStromalCell Trial (NCT01449032) (105) performed by Abbas Ali Qayyum and colleagues published the data of autologous ASCs treatment in 60 patients with chronic refractory angina. This study observed a marked decline in chest discomfort and a decrease in frequency of angina attacks in the ASCs group. However, no significant differences were observed between the two groups in the exercise tolerance testing. In their latest SCIENCE trial (NCT02673164) (106), allogeneic ASCs from healthy donors were injected intramyocardially into 133 IHD patients with reduced ejection fraction. Three-year follow-up data disclosed the safety of allogeneic ASCs therapy. However, there were no significant differences in cardiac function including LVEF, LVESV, and LVEDV between ASCs and placebo group. In clinical trial NCT03797092 (107), scientists utilized the cryopreserved product ASCs from healthy donors to treat patients with IHD and observed improved cardiac function without ASCs-related immune response after 6-months of follow-up. In another research followed-up for 12-month, authors utilized cardiac magnetic resonance to evaluate the improvement of cardiac function. Thirteen patients with IHD were enrolled and accepted ASCs implantation. Their results disclosed increased stroke volume and left ventricle ejection fraction, as well as improved cardiac output after 12 months of follow-up (108).
The completed clinical trials in Table 2 showed the safety and efficacy of ASCs therapy in IHD without exception. However, most studies evaluated the major adverse cardiac and cerebral events (MACCE) as the adverse effect and cardiac function at a very early stage, which might overlook the potential risks and overstate the benefit of ASCs implantation. Inflammation and embolism have been recognized as the major blight of ASCs application. Longtime follow-up clinical trials with a large sample size will provide more supportive data for this procedure.
Conclusion
ASCs are readily obtained, with minimal invasiveness, high yield, low immunogenicity, and no ethical issues, which enable them an innovative option in regenerative medicine. Meanwhile, ASCs have exhibited great advantages both in autologous and allogeneic transplantation, especially in the commercialization of stem cell-based therapy. ASCs play a protective role in IHD through differentiation into cardiomyocytes and endothelial cells, but more importantly acting as “paracrine factories” where a large quantity of cytokines is produced to trigger angiogenesis and modulate the immune system. However, the heterogeneity of ASCs should attract more attention due to that tissue origin or health state of donors affects cell properties and functions. Therefore, a critical and proper criterion will enable us to select the appropriate donors for clinical applications. Moreover, to overcome the limitation of low retention after engraftment, engineered ASCs through biomaterials have exhibited great potential in preclinical studies. Continuously optimized delivery strategies are of great value in clinical transformation of ASCs.
Author contributions
WX: Conceptualization, Formal Analysis, Software, Writing – original draft, Writing – review & editing. JS: Conceptualization, Funding acquisition, Resources, Writing – review & editing.
Funding
The author(s) declare financial support was received for the research, authorship, and/or publication of this article.
This work is supported by the National Natural Science Foundation of China (82370253), Jiangsu Provincial Research Hospital (YJXYY202204), Innovation Team Project of Affiliated Hospital of Nantong University (XNBHCX31773).
Conflict of interest
The authors declare that the research was conducted in the absence of any commercial or financial relationships that could be construed as a potential conflict of interest.
Publisher's note
All claims expressed in this article are solely those of the authors and do not necessarily represent those of their affiliated organizations, or those of the publisher, the editors and the reviewers. Any product that may be evaluated in this article, or claim that may be made by its manufacturer, is not guaranteed or endorsed by the publisher.
References
1. Virani SS, Alonso A, Aparicio HJ, Benjamin EJ, Bittencourt MS, Callaway CW, et al. Heart disease and stroke statistics-2021 update: a report from the American heart association. Circulation. (2021) 143:e254–743. doi: 10.1161/CIR.0000000000000950
2. Singh A, Singh A, Sen D. Mesenchymal stem cells in cardiac regeneration: a detailed progress report of the last 6 years (2010–2015). Stem Cell Res Ther. (2016) 7:82. doi: 10.1186/s13287-016-0341-0
3. Kokai LE, Marra K, Rubin JP. Adipose stem cells: biology and clinical applications for tissue repair and regeneration. Transl Res. (2014) 163(4):399–408. doi: 10.1016/j.trsl.2013.11.009
4. Burrow KL, Hoyland JA, Richardson SM. Human adipose-derived stem cells exhibit enhanced proliferative capacity and retain multipotency longer than donor-matched bone marrow mesenchymal stem cells during expansion in vitro. Stem Cells Int. (2017) 2017(4):2541275. doi: 10.1155/2017/2541275
5. Arévalo-Turrubiarte M, Olmeo C, Accornero P, Baratta M, Martignani E. Analysis of mesenchymal cells (MSCs) from bone marrow, synovial fluid and mesenteric, neck and tail adipose tissue sources from equines. Stem Cell Res. (2019) 37:101442. doi: 10.1016/j.scr.2019.101442
6. Bearden RN, Huggins SS, Cummings KJ, Smith R, Gregory CA, Saunders WB. In-vitro characterization of canine multipotent stromal cells isolated from synovium, bone marrow, and adipose tissue: a donor-matched comparative study. Stem Cell Res Ther. (2017) 8:218. doi: 10.1186/s13287-017-0639-6
7. Xu L, Liu Y, Sun Y, Wang B, Xiong Y, Lin W, et al. Tissue source determines the differentiation potentials of mesenchymal stem cells: a comparative study of human mesenchymal stem cells from bone marrow and adipose tissue. Stem Cell Res Ther. (2017) 8:275. doi: 10.1186/s13287-017-0716-x
8. Waldner M, Zhang W, James IB, Allbright K, Havis E, Bliley JM, et al. Characteristics and immunomodulating functions of adipose-derived and bone marrow-derived mesenchymal stem cells across defined human leukocyte antigen barriers. Front Immunol. (2018) 9:1642. doi: 10.3389/fimmu.2018.01642
9. Mohamed-Ahmed S, Yassin MA, Rashad A, Espedal H, Idris SB, Finne-Wistrand A, et al. Comparison of bone regenerative capacity of donor-matched human adipose-derived and bone marrow mesenchymal stem cells. Cell Tissue Res. (2021) 383:1061–75. doi: 10.1007/s00441-020-03315-5
10. Aboulhoda BE, Abd el Fattah S. Bone marrow-derived versus adipose-derived stem cells in wound healing: value and route of administration. Cell Tissue Res. (2018) 374:285–302. doi: 10.1007/s00441-018-2879-x
11. Melief SM, Zwaginga JJ, Fibbe WE, Roelofs H. Adipose tissue-derived multipotent stromal cells have a higher immunomodulatory capacity than their bone marrow-derived counterparts. Stem Cells Transl Med. (2013) 2(6):455–63. doi: 10.5966/sctm.2012-0184
12. Valencia J, Blanco B, Yáñez R, Vázquez M, Herrero Sánchez C, Fernández-García M, et al. Comparative analysis of the immunomodulatory capacities of human bone marrow- and adipose tissue-derived mesenchymal stromal cells from the same donor. Cytotherapy. (2016) 18(10):1297–311. doi: 10.1016/j.jcyt.2016.07.006
13. Torres Crigna A, Uhlig S, Elvers-Hornung S, Klüter H, Bieback K. Human adipose tissue-derived stromal cells suppress human, but not murine lymphocyte proliferation, via indoleamine 2,3-dioxygenase activity. Cells. (2020) 9(11):2419. doi: 10.3390/cells9112419
14. Lee MS, Wang J, Yuan H, Jiao H, Tsai TL, Squire MW, et al. Endothelin-1 differentially directs lineage specification of adipose- and bone marrow-derived mesenchymal stem cells. FASEB J. (2019) 33:996–1007. doi: 10.1096/fj.201800614R
15. Nagata H, Ii M, Kohbayashi E, Hoshiga M, Hanafusa T, Asahi M. Cardiac adipose-derived stem cells exhibit high differentiation potential to cardiovascular cells in C57BL/6 mice. Stem Cells Transl Med. (2016) 5:141–51. doi: 10.5966/sctm.2015-0083
16. Clark K, Janorkar AV. Milieu for endothelial differentiation of human adipose-derived stem cells. Bioengineering (Basel). (2018) 5(4):82. doi: 10.3390/bioengineering5040082
17. Shang T, Li S, Zhang Y, Lu L, Cui L, Guo FF. Hypoxia promotes differentiation of adipose-derived stem cells into endothelial cells through demethylation of ephrinB2. Stem Cell Res Ther. (2019) 10:133. doi: 10.1186/s13287-019-1233-x
18. Konno M, Hamazaki TS, Fukuda S, Tokuhara M, Uchiyama H, Okazawa H, et al. Efficiently differentiating vascular endothelial cells from adipose tissue-derived mesenchymal stem cells in serum-free culture. Biochem Biophys Res Commun. (2010) 400:461–5. doi: 10.1016/j.bbrc.2010.08.029
19. Zhang P, Moudgill N, Hager E, Tarola N, Dimatteo C, McIlhenny S, et al. Endothelial differentiation of adipose-derived stem cells from elderly patients with cardiovascular disease. Stem Cells Dev. (2011) 20:977–88. doi: 10.1089/scd.2010.0152
20. Planat-Benard V, Silvestre JS, Cousin B, André M, Nibbelink M, Tamarat R, et al. Plasticity of human adipose lineage cells toward endothelial cells: physiological and therapeutic perspectives. Circulation. (2004) 109:656–63. doi: 10.1161/01.CIR.0000114522.38265.61
21. Arderiu G, Peña E, Aledo R, Juan-Babot O, Crespo J, Vilahur G, et al. MicroRNA-145 regulates the differentiation of adipose stem cells toward microvascular endothelial cells and promotes angiogenesis. Circ Res. (2019) 125:74–89. doi: 10.1161/CIRCRESAHA.118.314290
22. Diaz Villamil E, De Roeck L, Vanorlé M, Communi D. UTP regulates the cardioprotective action of transplanted stem cells derived from mouse cardiac adipose tissue. Front Pharmacol. (2022) 13:906173. doi: 10.3389/fphar.2022.906173
23. Vanorlé M, Lemaire A, di Pietrantonio L, Horckmans M, Communi D. UTP is a regulator of in vitro and in vivo angiogenic properties of cardiac adipose-derived stem cells. Purinergic Signal. (2021) 17:681–91. doi: 10.1007/s11302-021-09812-8
24. Zhang D, Yu K, Hu X, Jiang A. Uniformly-aligned gelatin/polycaprolactone fibers promote proliferation in adipose-derived stem cells and have distinct effects on cardiac cell differentiation. Int J Clin Exp Pathol. (2021) 14:680–92.34239669
25. Wang H, Shi J, Wang Y, Yin Y, Wang L, Liu J, et al. Promotion of cardiac differentiation of brown adipose derived stem cells by chitosan hydrogel for repair after myocardial infarction. Biomaterials. (2014) 35:3986–98. doi: 10.1016/j.biomaterials.2014.01.021
26. Yan W, Li Y, Yin J, Liu Q, Shi Y, Tan J, et al. Protective effect of human epicardial adipose-derived stem cells on myocardial injury driven by poly-lactic acid nanopillar array. Biotechnol Appl Biochem. (2023):1–13. doi: 10.1002/bab.2525
27. Karimi T, Pan Z, Potaman VN, Alt EU. Conversion of unmodified stem cells to pacemaker cells by overexpression of key developmental genes. Cells. (2023) 12(10):1381. doi: 10.3390/cells12101381
28. Darche FF, Rivinius R, Rahm AK, Köllensperger E, Leimer U, Germann G, et al. In vivo cardiac pacemaker function of differentiated human mesenchymal stem cells from adipose tissue transplanted into porcine hearts. World J Stem Cells. (2020) 12:1133–51. doi: 10.4252/wjsc.v12.i10.1133
29. Sun AJ, Qiao L, Huang C, Zhang X, Li YQ, Yang XQ. Comparison of mouse brown and white adipose-derived stem cell differentiation into pacemaker-like cells induced by TBX18 transduction. Mol Med Rep. (2018) 17:7055–64. doi: 10.3892/mmr.2018.8792
30. Yang M, Zhang GG, Wang T, Wang X, Tang YH, Huang H, et al. TBX18 Gene induces adipose-derived stem cells to differentiate into pacemaker-like cells in the myocardial microenvironment. Int J Mol Med. (2016) 38:1403–10. doi: 10.3892/ijmm.2016.2736
31. Hu X, Xu Y, Zhong Z, Wu Y, Zhao J, Wang Y, et al. A large-scale investigation of hypoxia-preconditioned allogeneic mesenchymal stem cells for myocardial repair in nonhuman primates: paracrine activity without remuscularization. Circ Res. (2016) 118:970–83. doi: 10.1161/CIRCRESAHA.115.307516
32. Watanabe M, Horie H, Kurata Y, Inoue Y, Notsu T, Wakimizu T, et al. Esm1 and Stc1 as angiogenic factors responsible for protective actions of adipose-derived stem cell sheets on chronic heart failure after rat myocardial infarction. Circ J. (2021) 85:657–66. doi: 10.1253/circj.CJ-20-0877
33. Bhang SH, Lee S, Shin JY, Lee TJ, Jang HK, Kim BS. Efficacious and clinically relevant conditioned medium of human adipose-derived stem cells for therapeutic angiogenesis. Mol Ther. (2014) 22:862–72. doi: 10.1038/mt.2013.301
34. Chen QH, Liu AR, Qiu HB, Yang Y. Interaction between mesenchymal stem cells and endothelial cells restores endothelial permeability via paracrine hepatocyte growth factor in vitro. Stem Cell Res Ther. (2015) 6:44. doi: 10.1186/s13287-015-0025-1
35. Mussano F, Genova T, Corsalini M, Schierano G, Pettini F, Di Venere D, et al. Cytokine, chemokine, and growth factor profile characterization of undifferentiated and osteoinduced human adipose-derived stem cells. Stem Cells Int. (2017) 2017:6202783. doi: 10.1155/2017/6202783
36. Naftali-Shani N, Itzhaki-Alfia A, Landa-Rouben N, Kain D, Holbova R, Adutler-Lieber S, et al. The origin of human mesenchymal stromal cells dictates their reparative properties. J Am Heart Assoc. (2013) 2:e000253. doi: 10.1161/JAHA.113.000253
37. Ell J, Regn S, Buchberger AM, von Bomhard A, Stark T, Schantz JT, et al. Donor-dependent variances of human adipose-derived stem cells in respect to the in-vitro endothelial cell differentiation capability. Adipocyte. (2017) 6:20–32. doi: 10.1080/21623945.2016.1273299
38. He J, Cai Y, Luo LM, Liu HB. Hypoxic adipose mesenchymal stem cells derived conditioned medium protects myocardial infarct in rat. Eur Rev Med Pharmacol Sci. (2015) 19:4397–406.26636529
39. Zhao Y, Zhang M, Lu GL, Huang BX, Wang DW, Shao Y, et al. Hypoxic preconditioning enhances cellular viability and pro-angiogenic paracrine activity: the roles of VEGF-A and SDF-1a in rat adipose stem cells. Front Cell Dev Biol. (2020) 8:580131. doi: 10.3389/fcell.2020.580131
40. Mytsyk M, Cerino G, Reid G, Sole LG, Eckstein FS, Santer D, et al. Long-term severe in vitro hypoxia exposure enhances the vascularization potential of human adipose tissue-derived stromal vascular fraction cell engineered tissues. Int J Mol Sci. (2021) 22(15):7920. doi: 10.3390/ijms22157920
41. Rehman J, Traktuev D, Li J, Merfeld-Clauss S, Temm-Grove CJ, Bovenkerk JE, et al. Secretion of angiogenic and antiapoptotic factors by human adipose stromal cells. Circulation. (2004) 109:1292–8. doi: 10.1161/01.CIR.0000121425.42966.F1
42. Kang T, Jones TM, Naddell C, Bacanamwo M, Calvert JW, Thompson WE, et al. Adipose-derived stem cells induce angiogenesis via microvesicle transport of miRNA-31. Stem Cells Transl Med. (2016) 5:440–50. doi: 10.5966/sctm.2015-0177
43. Xiao S, Liu Z, Yao Y, Wei ZR, Wang D, Deng C. Diabetic human adipose-derived stem cells accelerate pressure ulcer healing by inducing angiogenesis and neurogenesis. Stem Cells Dev. (2019) 28:319–28. doi: 10.1089/scd.2018.0245
44. Qin F, Zhang W, Zhang M, Long X, Si L, Li Z, et al. Adipose-Derived stem cells improve the aging skin of nude mice by promoting angiogenesis and reducing local tissue water. Aesthet Surg J. (2021) 41:NP905–913. doi: 10.1093/asj/sjab001
45. Czapla J, Cichon T, Pilny E, Jarosz-Biej M, Matuszczak S, Drzyzga A, et al. Adipose tissue-derived stromal cells stimulated macrophages-endothelial cells interactions promote effective ischemic muscle neovascularization. Eur J Pharmacol. (2020) 883:173354. doi: 10.1016/j.ejphar.2020.173354
46. Follin B, Ghotbi AA, Clemmensen AE, Bentsen S, Juhl M, Søndergaard RH, et al. Retention and functional effect of adipose-derived stromal cells administered in alginate hydrogel in a rat model of acute myocardial infarction. Stem Cells Int. (2018) 2018:7821461. doi: 10.1155/2018/7821461
47. Jiang Y, Chang P, Pei Y, Li B, Liu Y, Zhang Z, et al. Intramyocardial injection of hypoxia-preconditioned adipose-derived stromal cells treats acute myocardial infarction: an in vivo study in swine. Cell Tissue Res. (2014) 358:417–32. doi: 10.1007/s00441-014-1975-9
48. Katagiri T, Kondo K, Shibata R, Hayashida R, Shintani S, Yamaguchi S, et al. Therapeutic angiogenesis using autologous adipose-derived regenerative cells in patients with critical limb ischaemia in Japan: a clinical pilot study. Sci Rep. (2020) 10:16045. doi: 10.1038/s41598-020-73096-y
49. Liu J, Qiu P, Qin J, Wu X, Wang X, Yang X, et al. Allogeneic adipose-derived stem cells promote ischemic muscle repair by inducing M2 macrophage polarization via the HIF-1α/IL-10 pathway. Stem Cells. (2020) 38:1307–20. doi: 10.1002/stem.3250
50. Lee TM, Harn HJ, Chiou TW, Chuang MH, Chen CH, Chuang CH, et al. Remote transplantation of human adipose-derived stem cells induces regression of cardiac hypertrophy by regulating the macrophage polarization in spontaneously hypertensive rats. Redox Biol. (2019) 27:101170. doi: 10.1016/j.redox.2019.101170
51. Mori D, Miyagawa S, Matsuura R, Sougawa N, Fukushima S, Ueno T, et al. Pioglitazone strengthen therapeutic effect of adipose-derived regenerative cells against ischemic cardiomyopathy through enhanced expression of adiponectin and modulation of macrophage phenotype. Cardiovasc Diabetol. (2019) 18:39. doi: 10.1186/s12933-019-0829-x
52. Li F, Guo X, Chen SY. Function and therapeutic potential of mesenchymal stem cells in atherosclerosis. Front Cardiovasc Med. (2017) 4:32. doi: 10.3389/fcvm.2017.00032
53. Fiori A, Uhlig S, Klüter H, Bieback K. Human adipose tissue-derived mesenchymal stromal cells inhibit CD4+ T cell proliferation and induce regulatory T cells as well as CD127 expression on CD4+ CD25+ T cells. Cells. (2021) 10(1):58. doi: 10.3390/cells10010058
54. Munn DH, Mellor AL. Indoleamine 2,3 dioxygenase and metabolic control of immune responses. Trends Immunol. (2013) 34:137–43. doi: 10.1016/j.it.2012.10.001
55. Han X, Boisvert WA. Interleukin-10 protects against atherosclerosis by modulating multiple atherogenic macrophage function. Thromb Haemost. (2015) 113:505–12. doi: 10.1160/TH14-06-0509
56. Klingenberg R, Gerdes N, Badeau RM, Gisterå A, Strodthoff D, Ketelhuth DF, et al. Depletion of FOXP3+regulatory T cells promotes hypercholesterolemia and atherosclerosis. J Clin Invest. (2013) 123:1323–34. doi: 10.1172/JCI63891
57. Gornostaeva AN, Bobyleva PI, Andreeva ER, Yakubets DA, Buravkova LB. Adipose-derived stromal cell immunosuppression of T cells is enhanced under “physiological” hypoxia. Tissue Cell. (2020) 63:101320. doi: 10.1016/j.tice.2019.101320
58. Fan M, Bai J, Ding T, Yang X, Si Q, Nie D. Adipose-derived stem cell transplantation inhibits vascular inflammatory responses and endothelial dysfunction in rats with atherosclerosis. Yonsei Med J. (2019) 60:1036–44. doi: 10.3349/ymj.2019.60.11.1036
59. Xing X, Li Z, Yang X, Li M, Liu C, Pang Y, et al. Adipose-derived mesenchymal stem cells-derived exosome-mediated microRNA-342-5p protects endothelial cells against atherosclerosis. Aging (Albany NY). (2020) 12:3880–98. doi: 10.18632/aging.102857
60. Wang T, Li T, Niu X, Hu L, Cheng J, Guo D, et al. ADSC-derived exosomes attenuate myocardial infarction injury by promoting miR-205-mediated cardiac angiogenesis. Biol Direct. (2023) 18:6. doi: 10.1186/s13062-023-00361-1
61. Zhu D, Wang Y, Thomas M, McLaughlin K, Oguljahan B, Henderson J, et al. Exosomes from adipose-derived stem cells alleviate myocardial infarction via microRNA-31/FIH1/HIF-1α pathway. J Mol Cell Cardiol. (2022) 162:10–9. doi: 10.1016/j.yjmcc.2021.08.010
62. Zhang J, Zhang J, Jiang X, Jin J, Wang H, Zhang Q. ASCs-EVs inhibit apoptosis and promote myocardial function in the infarcted heart via miR-221. Discov Med. (2023) 35:1077–85. doi: 10.24976/Discov.Med.202335179.104
63. Lai TC, Lee TL, Chang YC, Chen YC, Lin SR, Lin SW, et al. MicroRNA-221/222 mediates ADSC-exosome-induced cardioprotection against ischemia/reperfusion by targeting PUMA and ETS-1. Front Cell Dev Biol. (2020) 8:569150. doi: 10.3389/fcell.2020.569150
64. Lee TL, Lai TC, Lin SR, Lin SW, Chen YC, Pu CM, et al. Conditioned medium from adipose-derived stem cells attenuates ischemia/reperfusion-induced cardiac injury through the microRNA-221/222/PUMA/ETS-1 pathway. Theranostics. (2021) 11:3131–49. doi: 10.7150/thno.52677
65. Deng S, Zhou X, Ge Z, Song Y, Wang H, Liu X, et al. Exosomes from adipose-derived mesenchymal stem cells ameliorate cardiac damage after myocardial infarction by activating S1P/SK1/S1PR1 signaling and promoting macrophage M2 polarization. Int J Biochem Cell Biol. (2019) 114:105564. doi: 10.1016/j.biocel.2019.105564
66. Zhang Y, Yang N, Huang X, Zhu Y, Gao S, Liu Z, et al. Melatonin engineered adipose-derived biomimetic nanovesicles regulate mitochondrial functions and promote myocardial repair in myocardial infarction. Front Cardiovasc Med. (2022) 9:789203. doi: 10.3389/fcvm.2022.789203
67. Das A, Nikhil A, Shiekh PA, Yadav B, Jagavelu K, Kumar A. Ameliorating impaired cardiac function in myocardial infarction using exosome-loaded gallic-acid-containing polyurethane scaffolds. Bioact Mater. (2024) 33:324–40. doi: 10.1016/j.bioactmat.2023.11.009
68. Pfeifer A, Hoffmann LS. Brown, beige, and white: the new color code of fat and its pharmacological implications. Annu Rev Pharmacol Toxicol. (2015) 55:207–27. doi: 10.1146/annurev-pharmtox-010814-124346
69. Cypess AM, White AP, Vernochet C, Schulz TJ, Xue R, Sass CA, et al. Anatomical localization, gene expression profiling and functional characterization of adult human neck brown fat. Nat Med. (2013) 19:635–9. doi: 10.1038/nm.3112
70. Lim S, Honek J, Xue Y, Seki T, Cao Z, Andersson P, et al. Cold-induced activation of brown adipose tissue and adipose angiogenesis in mice. Nat Protoc. (2012) 7:606–15. doi: 10.1038/nprot.2012.013
71. Russo V, Yu C, Belliveau P, Hamilton A, Flynn LE. Comparison of human adipose-derived stem cells isolated from subcutaneous, omental, and intrathoracic adipose tissue depots for regenerative applications. Stem Cells Transl Med. (2014) 3:206–17. doi: 10.5966/sctm.2013-0125
72. Wang T, Hill RC, Dzieciatkowska M, Zhu L, Infante AM, Hu G, et al. Site-dependent lineage preference of adipose stem cells. Front Cell Dev Biol. (2020) 8:237. doi: 10.3389/fcell.2020.00237
73. Efimenko A, Dzhoyashvili N, Kalinina N, Kochegura T, Akchurin R, Tkachuk V, et al. Adipose-derived mesenchymal stromal cells from aged patients with coronary artery disease keep mesenchymal stromal cell properties but exhibit characteristics of aging and have impaired angiogenic potential. Stem Cells Transl Med. (2014) 3:32–41. doi: 10.5966/sctm.2013-0014
74. El-Ftesi S, Chang EI, Longaker MT, Gurtner GC. Aging and diabetes impair the neovascular potential of adipose-derived stromal cells. Plast Reconstr Surg. (2009) 123:475–85. doi: 10.1097/PRS.0b013e3181954d08
75. Efimenko A, Starostina E, Kalinina N, Stolzing A. Angiogenic properties of aged adipose derived mesenchymal stem cells after hypoxic conditioning. J Transl Med. (2011) 9:10. doi: 10.1186/1479-5876-9-10
76. Alicka M, Kornicka-Garbowska K, Kucharczyk K, Kępska M, Röcken M, Marycz K. Age-dependent impairment of adipose-derived stem cells isolated from horses. Stem Cell Res Ther. (2020) 11:4. doi: 10.1186/s13287-019-1512-6
77. Payr S, Schuseil T, Unger M, Seeliger C, Tiefenboeck T, Balmayor ER, et al. Effect of donor age and 3D-cultivation on osteogenic differentiation capacity of adipose-derived mesenchymal stem cells. Sci Rep. (2020) 10:10408. doi: 10.1038/s41598-020-67254-5
78. Kizilay Mancini O, Shum-Tim D, Stochaj U, Correa JA, Colmegna I. Age, atherosclerosis and type 2 diabetes reduce human mesenchymal stromal cell-mediated T-cell suppression. Stem Cell Res Ther. (2015) 6:140. doi: 10.1186/s13287-015-0127-9
79. Karina Pawitan JA, Imam R. Adipose-derived stem cells and their microenvironment (niche) in type 2 diabetes mellitus. Stem Cell Investig. (2020) 7:2. doi: 10.21037/sci.2019.12.02
80. van de Vyver M. Intrinsic mesenchymal stem cell dysfunction in diabetes mellitus: implications for autologous cell therapy. Stem Cells Dev. (2017) 26:1042–53. doi: 10.1089/scd.2017.0025
81. Gu JH, Lee JS, Kim DW, Yoon ES, Dhong ES. Neovascular potential of adipose-derived stromal cells (ASCs) from diabetic patients. Wound Repair Regen. (2012) 20:243–52. doi: 10.1111/j.1524-475X.2012.00765.x
82. Cronk SM, Kelly-Goss MR, Ray HC, Mendel TA, Hoehn KL, Bruce AC, et al. Adipose-derived stem cells from diabetic mice show impaired vascular stabilization in a murine model of diabetic retinopathy. Stem Cells Transl Med. (2015) 4:459–67. doi: 10.5966/sctm.2014-0108
83. Policha A, Zhang P, Chang L, Lamb K, Tulenko T, DiMuzio P. Endothelial differentiation of diabetic adipose-derived stem cells. J Surg Res. (2014) 192:656–63. doi: 10.1016/j.jss.2014.06.041
84. Gustafson B, Nerstedt A, Smith U. Reduced subcutaneous adipogenesis in human hypertrophic obesity is linked to senescent precursor cells. Nat Commun. (2019) 10:2757. doi: 10.1038/s41467-019-10688-x
85. Rennert RC, Sorkin M, Januszyk M, Duscher D, Kosaraju R, Chung MT, et al. Diabetes impairs the angiogenic potential of adipose-derived stem cells by selectively depleting cellular subpopulations. Stem Cell Res Ther. (2014) 5:79. doi: 10.1186/scrt468
86. Sun Y, Song L, Zhang Y, Wang H, Dong X. Adipose stem cells from type 2 diabetic mice exhibit therapeutic potential in wound healing. Stem Cell Res Ther. (2020) 11:298. doi: 10.1186/s13287-020-01817-1
87. Jiao H, Xiao E, Graves DT. Diabetes and its effect on bone and fracture healing. Curr Osteoporos Rep. (2015) 13:327–35. doi: 10.1007/s11914-015-0286-8
88. Skubis-Sikora A, Sikora B, Witkowska A, Mazurek U, Gola J. Osteogenesis of adipose-derived stem cells from patients with glucose metabolism disorders. Mol Med. (2020) 26:67. doi: 10.1186/s10020-020-00192-0
89. Serena C, Keiran N, Ceperuelo-Mallafre V, Ejarque M, Fradera R, Roche K, et al. Obesity and type 2 diabetes alters the immune properties of human adipose derived stem cells. Stem Cells. (2016) 34:2559–73. doi: 10.1002/stem.2429
90. Kizilay Mancini O, Lora M, Cuillerier A, Shum-Tim D, Hamdy R, Burelle Y, et al. Mitochondrial oxidative stress reduces the immunopotency of mesenchymal stromal cells in adults with coronary artery disease. Circ Res. (2018) 122:255–66. doi: 10.1161/CIRCRESAHA.117.311400
91. Bonello S, Zähringer C, BelAiba RS, Djordjevic T, Hess J, Michiels C, et al. Reactive oxygen species activate the HIF-1alpha promoter via a functional NFkappaB site. Arterioscler Thromb Vasc Biol. (2007) 27:755–61. doi: 10.1161/01.ATV.0000258979.92828.bc
92. Follin B, Juhl M, Cohen S, Pedersen AE, Gad M, Kastrup J, et al. Human adipose-derived stromal cells in a clinically applicable injectable alginate hydrogel: phenotypic and immunomodulatory evaluation. Cytotherapy. (2015) 17:1104–18. doi: 10.1016/j.jcyt.2015.04.008
93. Hao T, Li J, Yao F, Dong D, Wang Y, Yang B, et al. Injectable fullerenol/alginate hydrogel for suppression of oxidative stress damage in brown adipose-derived stem cells and cardiac repair. ACS Nano. (2017) 11:5474–88. doi: 10.1021/acsnano.7b00221
94. Dolan EB, Hofmann B, de Vaal MH, Bellavia G, Straino S, Kovarova L, et al. A bioresorbable biomaterial carrier and passive stabilization device to improve heart function post-myocardial infarction. Mater Sci Eng C Mater Biol Appl. (2019) 103:109751. doi: 10.1016/j.msec.2019.109751
95. Long G, Wang Q, Li S, Tao J, Li B, Zhang X, et al. Engineering of injectable hydrogels associate with adipose-derived stem cells delivery for anti-cardiac hypertrophy agents. Drug Deliv. (2021) 28:1334–41. doi: 10.1080/10717544.2021.1943060
96. Wang BZ, Wang MM, Li Y, Shao MH, Zhang D, Yan SQ, et al. Functional enhancement of acute infracted heart by coinjection of autologous adipose-derived stem cells with matrigel. Turk J Biol. (2023) 47:170–85. doi: 10.55730/1300-0152.2653
97. Araña M, Gavira JJ, Peña E, González A, Abizanda G, Cilla M, et al. Epicardial delivery of collagen patches with adipose-derived stem cells in rat and minipig models of chronic myocardial infarction. Biomaterials. (2014) 35:143–51. doi: 10.1016/j.biomaterials.2013.09.083
98. Wang J, Xiang B, Deng J, Lin HY, Zheng D, Freed DH, et al. Externally applied static magnetic field enhances cardiac retention and functional benefit of magnetically iron-labeled adipose-derived stem cells in infarcted hearts. Stem Cells Transl Med. (2016) 5:1380–93. doi: 10.5966/sctm.2015-0220
99. Zhang J, Li J, Qu X, Liu Y, Harada A, Hua Y, et al. Development of a thick and functional human adipose-derived stem cell tissue sheet for myocardial infarction repair in rat hearts. Stem Cell Res Ther. (2023) 14:380. doi: 10.1186/s13287-023-03560-9
100. Kajbafzadeh AM, Tafti S, Khorramirouz R, Sabetkish S, Kameli SM, Orangian S, et al. Evaluating the role of autologous mesenchymal stem cell seeded on decellularized pericardium in the treatment of myocardial infarction: an animal study. Cell Tissue Bank. (2017) 18:527–38. doi: 10.1007/s10561-017-9629-2
101. Yoshizaki Y, Takai H, Mayumi N, Fujiwara S, Kuzuya A, Ohya Y. Cellular therapy for myocardial ischemia using a temperature-responsive biodegradable injectable polymer system with adipose-derived stem cells. Sci Technol Adv Mater. (2021) 22:627–42. doi: 10.1080/14686996.2021.1938212
102. Wei X, Wang L, Duan C, Chen K, Li X, Guo X, et al. Cardiac patches made of brown adipose-derived stem cell sheets and conductive electrospun nanofibers restore infarcted heart for ischemic myocardial infarction. Bioact Mater. (2023) 27:271–87. doi: 10.1016/j.bioactmat.2023.03.023
103. Cheng N, Ren C, Yang M, Wu Y, Zhang H, Wei S, et al. Injectable cryogels associate with adipose-derived stem cells for cardiac healing after acute myocardial infarctions. J Biomed Nanotechnol. (2021) 17:981–8. doi: 10.1166/jbn.2021.3082
104. Houtgraaf JH, den Dekker WK, van Dalen BM, Springeling T, de Jong R, van Geuns RJ, et al. First experience in humans using adipose tissue-derived regenerative cells in the treatment of patients with ST-segment elevation myocardial infarction. J Am Coll Cardiol. (2012) 59:539–40. doi: 10.1016/j.jacc.2011.09.065
105. Qayyum AA, Mathiasen AB, Helqvist S, Jørgensen E, Haack-Sørensen M, Ekblond A, et al. Autologous adipose-derived stromal cell treatment for patients with refractory angina (MyStromalCell trial): 3-years follow-up results. J Transl Med. (2019) 17:360. doi: 10.1186/s12967-019-2110-1
106. Qayyum AA, van Klarenbosch B, Frljak S, Cerar A, Poglajen G, Traxler-Weidenauer D, et al. Effect of allogeneic adipose tissue-derived mesenchymal stromal cell treatment in chronic ischaemic heart failure with reduced ejection fraction—the SCIENCE trial. Eur J Heart Fail. (2023) 25:576–87. doi: 10.1002/ejhf.2772
107. Kastrup J, Haack-Sørensen M, Juhl M, Harary Søndergaard R, Follin B, Drozd Lund L, et al. Cryopreserved off-the-shelf allogeneic adipose-derived stromal cells for therapy in patients with ischemic heart disease and heart failure-a safety study. Stem Cells Transl Med. (2017) 6:1963–71. doi: 10.1002/sctm.17-0040
Keywords: adipose-derived mesenchymal stem cells, stem cell transplantation, ischemic heart disease, differentiation, exosomes, paracrine
Citation: Xiao W and Shi J (2024) Application of adipose-derived stem cells in ischemic heart disease: theory, potency, and advantage. Front. Cardiovasc. Med. 11:1324447. doi: 10.3389/fcvm.2024.1324447
Received: 24 October 2023; Accepted: 9 January 2024;
Published: 19 January 2024.
Edited by:
Diana S. Nascimento, Universidade do Porto, PortugalReviewed by:
Sini Sunny, University of Alabama at Birmingham, United StatesChunying Li, Georgia State University, United States
© 2024 Xiao and Shi. This is an open-access article distributed under the terms of the Creative Commons Attribution License (CC BY). The use, distribution or reproduction in other forums is permitted, provided the original author(s) and the copyright owner(s) are credited and that the original publication in this journal is cited, in accordance with accepted academic practice. No use, distribution or reproduction is permitted which does not comply with these terms.
*Correspondence: Jiahai Shi aGFwcHlzamgxNjdAMTYzLmNvbQ==