- 1Department of Medicine, Einstein-Mount Sinai Diabetes Research Center (ES-DRC), Albert Einstein College of Medicine, Fleischer Institute for Diabetes and Metabolism (FIDAM), Einstein Institute for Aging Research, New York, NY, United States
- 2Department of Molecular Pharmacology, Albert Einstein College of Medicine, Wilf Family Cardiovascular Research Institute, Institute for Neuroimmunology and Inflammation (INI), New York, NY, United States
After an ischemic injury, the heart undergoes a complex process of structural and functional remodeling that involves several steps, including inflammatory and fibrotic responses. In this review, we are focusing on the contribution of microRNAs in the regulation of inflammation and fibrosis after myocardial infarction. We summarize the most updated studies exploring the interactions between microRNAs and key regulators of inflammation and fibroblast activation and we discuss the recent discoveries, including clinical applications, in these rapidly advancing fields.
Introduction
microRNAs (also known as miRNAs or miRs) are small (∼22 nucleotides) non-coding RNA molecules that can regulate gene expression via translational repression and/or post-transcriptional degradation; they have been implied in a number of cardiovascular disorders (1–4). Following myocardial infarction (MI), the heart undergoes a series of structural, functional, and pathophysiological modifications that are commonly known as cardiac remodeling (5–7).
In this minireview, we will focus on the role of miRNAs in two specific components of post-ischemic cardiac remodeling, namely fibrosis and inflammation (Table 1).
Effects of microRNAs on Cardiac Fibroblasts Post-Mi
Cardiac fibroblasts are the most abundant interstitial cell type in the heart (39–43). They play essential roles in the regulation of cardiac remodeling following an ischemic injury; indeed, they are generally activated in response to pathological stress or injury, and start to proliferate quickly and to produce extracellular matrix (ECM), eventually leading to cardiac fibrosis (18, 42, 44–46). Activated cardiac fibroblasts, known as myofibroblasts, exhibit an increased proliferation rate and migratory capacities (47, 48).
The miRNA-212/132 family was originally detected by Ucar et al. (49); miR-132 has been later shown to fine-tune Angiotensin II actions in cardiac fibroblasts (50). These observations led to a clinical trial (51), which will be discussed in detail in the last paragraph of this minireview.
Another miRNA, generally considered to be muscle-specific (52, 53), namely miR-1, was shown to be expressed in cardiac fibroblasts as well, and to be significantly down-regulated upon their activation (18); miR-1 negatively regulates cardiac fibroblast proliferation by targeting Cyclin D2 and CDK6 (18). Glass and Singla demonstrated that miR-1 triggers cardiac differentiation and ameliorates heart function via targeting the PTEN/Akt pathway (19). Likewise, miR-19b (20) and miR-144-3p (14) have been proven to regulate proliferation and migration of cardiac fibroblasts by modulating PTEN expression.
By specifically targeting the signaling pathway that includes transforming growth factor β1 (TGF-β1) and mothers against DPP homologs 7 (SMAD7), miR-21 has been validated as an activator of cardiac fibroblasts post-MI, subsequently eliciting cardiac fibrosis, as well (23, 54); corroborating these findings, miR-21 had been previously shown to upregulate the expression of α-smooth muscle actin (α-SMA), Col-1, and F-actin (21) and to promote fibroblast proliferation and interstitial fibrosis via targeting the CADM1/STAT3 signaling pathway (22); on the other hand, miR-21 suppression reduces cardiac fibroblast proliferation (22). Independent investigators have confirmed that miR-21 expression is upregulated by TGF-β1 and mediates the conversion of quiescent cardiac fibroblasts to activated myofibroblasts via targeting the Notch/Jagged1 pathway (23, 55, 56), and that miR-21 is strategic in mediating the profibrotic role of cardiac macrophages (57).
Our group was the first to demonstrate that two different miRs, namely miR-92a (26) and miR-195 (35), act as transcriptional regulators of SMAD7, an inhibitor of α-SMA, which is a well-established marker of myofibroblast activation (58). We found that miR-92a is significantly upregulated in cardiomyocyte-derived exosomes and in fibroblasts isolated after MI compared with SHAM conditions, indicating that miR-92a is transferred to fibroblasts in form of exosomal cargo and is essential for the activation of cardiac myofibroblast (26). We also observed (35) that miR-195, a cardiomyocyte-specific miRNA that is upregulated in cardiac myocytes after an ischemic insult (59), is secreted by injured cardiomyocytes within cardiac exosomes (cardiosomes) and transferred to fibroblasts, where it relieves the SMAD7-mediated inhibition of α-SMA transcription, eventually leading to myofibroblast phenoconversion (35). The mechanistic involvement of exosomal miRs in cardiac fibroblasts has been more recently also reported by Suresh Verma’s research team, who determined that TGF-β1 activates cardiac fibroblasts and myofibroblasts-derived exosomes causes endothelial dysfunction mediated by miR-200a-3p via PIGF/VEGF-A signaling pathway (36).
Yuan and coworkers demonstrated that miR-590-3p can decrease proliferation, differentiation, and migration of cardiac fibroblasts via targeting ZEB1 expression (38); substantiating these observations, inhibiting miR-590-3p drastically augmented proliferation and migration of cardiac fibroblasts (38). Jazbutyte and colleagues revealed that miR-22 upregulation accelerates the senescence of cardiac fibroblasts by targeting osteoglycin (also known as mimecan) (60). Other reports have also indicated that miR-22 upregulates some specific genes of vascular smooth muscle cells (VSMC), thereby suppressing VSMC proliferation and migration, as well (24, 61–63).
Notably, miR-34a modulates cardiac fibrosis after MI via targeting SMAD4 (25): the upregulation of miR-34a promotes the profibrogenic activity of TGF-β1 in cardiac fibroblasts, whereas suppressing miR-34a has opposite effects (25). Similarly, miR-125b is decisive for the induction of cardiac fibrosis and plays a critical role in inducing fibroblast proliferation by suppressing p53 (27), a growth regulator and anti-fibrotic factor (64, 65). TGF-β1 changes the morphology of fibroblasts from spindle-shaped to well-spread myofibroblast-like cells and causes upregulation of molecular markers of myofibroblast activation, such as α-SMA and Col1; miR-125b was found to be overexpressed in endothelial-to-mesenchymal transition (EndMT)-derived myofibroblast-like cells, and such upregulation, triggered by TGF-β1, causes the inhibition of anti-fibrotic genes thus promoting the proliferation and activation of cardiac fibroblasts, leading to fibrosis (27). The inhibition of miR-155 has been reported to decrease the conversion of fibroblasts to myofibroblasts and to improve the cardiac fibrotic remodeling induced by Angiotensin II (34). Another miRNA that was shown to regulate fibroblast survival and proliferation via targeting the mitofusin-2 (Mfn2) gene is miR-214 (37).
A very recent study by Liao et al. has shown that the upregulation of miR-146b-5p activates fibroblast proliferation, migration, conversion of fibroblast to myofibroblast, and endothelial cell dysfunction (33); in contrast, inhibition of miR-146b-5p has opposite effects and promotes angiogenesis by targeting IRAK1 and CEACAM1 (33). Thus, suppression of miR-146b-5p may be a novel therapeutic approach to treat cardiac fibrotic dysfunction after MI.
Cardiac Inflammation and microRNAs
One of the most studied miRNAs in the regulation of the post-ischemic inflammatory response in the heart is miR-21, which is known to attenuate excessive inflammation and cardiac dysfunction after MI by targeting MKK3/6 and suppressing p38 and NF-κB signaling activation post-MI (9) and to stimulate MAP kinase signaling in fibroblasts (66), whereas its deficiency induces inflammatory reactions post-MI and significantly augments the phosphorylation of p38, IKKα/β, and p65 (9). Of note, miR-21 is also upregulated in cardiac macrophages (57), and nanoparticle-based targeted delivery of miR-21 to cardiac macrophages has been shown to ameliorate cardiac remodeling post-MI, modifying the phenotype of macrophages from a pro-inflammatory to a reparative state (67).
Right after MI, the expression levels of many pro-inflammatory cytokines including IL-1β, IL-6, and TNF-α increase, contributing to cardiac remodeling (68); miR-146a-5p induces expression of pro-inflammatory cytokines including CXCL2, IL-6, and TNF-α, and activates innate immune cells such as CD45+ leukocytes, Ly6Cmid+ monocytes, Ly6G+ neutrophils via a TLR7-dependent mechanism (15). Moreover, miR-146a-5p causes cardiac endothelial barrier dysfunction, further triggering an increased transmigration of monocytes and neutrophils into the myocardium (15). The inhibition of miR-146b-5p considerably increases cytokines such as IL-1β, IL-6, TNF-α, and MCP-1. In addition, in vivo assays demonstrated that CD206+ macrophages are increased due to suppression of miR-146b-5p (33).
Reducing the overexpression of miR-155 modulates the expression of cytokines such as IL-1 and CXCL8 (69); miR-155-enriched exosomes slow down cardiac fibroblast proliferation by downregulating Son of Sevenless 1 (SOS1) expression—which is also involved in the regulation of inflammation (70)—and can promote inflammation and atherosclerotic lesions by increasing STAT3 and NF-κB via targeting Suppressor of Cytokine Signaling 1 (SOCS1) expression (16). By conducting in vivo experiments. Wang et al. observed increased fibroblast proliferation, augmented collagen production, and reduced cardiac inflammation in the hearts of miR-155-deficient mice compared to control animals (71). The expression of miR-155 is also upregulated in exosomes of activated cardiac macrophages post-MI (72, 73).
Another miR fundamental in post-MI remodeling is miR-22: its overexpression triggers the synthesis of proinflammatory cytokines such as IL-1β, IL-6, and IL-8 (74), moreover, the same miR-22 is able to regulate inflammation and angiogenesis by specifically targeting VE-cadherin (10).
The synergistic interplay between inflammation and angiogenesis is crucial in post-ischemic cardiac remodeling and healing (75–80), and several researchers have demonstrated that non-coding RNAs are involved in the regulation of both these processes (78, 81). For instance, miR-133a has been shown to have beneficial effects on infarcted hearts by inhibiting inflammation and angiogenesis via FGFR1 and VEGFR2 signaling pathways (82, 83). Similarly, miR-320 and miR-144-3p, have been shown to be involved in post-MI responses by regulating PTEN/PI3K/Akt signaling pathway (13, 84, 85); miR-144-3p promotes cardiac fibrosis via targeting PTEN following MI (14); miR-199a-3p and miR-590-3p also improve cardiac function after MI (8, 86); miR-19a/19b inhibits the inflammatory response and has been shown to enhance cardiac function post-MI by targeting Bim1 and PTEN (8). All these results are relevant when considering that in vivo studies carried out in infarcted mice revealed that angiogenesis can be improved by inhibiting PTEN via activating the PI3K/Akt/VEGF pathway (13, 87, 88). On the same line, Lu and coworkers reported that the overexpression of miR-130a promotes endothelial cell proliferation and migration by increasing Akt phosphorylation and inhibiting PTEN (30); the same group also demonstrated that the activation of PI3K/Akt signaling enhances angiogenesis and decreases the progression of MI and fibrosis, attenuating myocardial dysfunction and reducing the risk of cardiac rupture post-MI (30). Several members of the miRNA cluster 17∼92 regulate angiogenesis following MI (89). Equally important, suppressing miR-375 was shown to mitigate post-MI inflammatory responses while improving angiogenesis via PDK-1/Akt signaling mechanisms (17).
Endothelial cells play decisive roles in post-MI cardiac remodeling (90, 91), and miR-126 is considered one of the most important miRs in endothelial biology (92–94). In mature endothelial cells, miR-126 promotes vascular homeostasis by preventing angiogenesis and preserving the quiescent endothelial phenotype via the HIF-1α pathway (28, 29). Remarkably, miR-199a-5p inhibition causes upregulation of VEGF-A, enhances nitric oxide (NO) bioavailability by activating eNOS (endothelial NO synthase), and stimulates the formation of network-like structures (95). Likewise, miR-133a causes endothelial dysfunction by suppressing eNOS, and its overexpression significantly reduces endothelial cell survival by targeting GTP Cyclohydrolase 1 GCH1 (31, 32). Lastly, the overexpression of miR-92a inhibits endothelial cell migration and regulates angiogenesis (11, 89) whereas its inhibition enhances endothelial cell proliferation via the activation of the JNK and ERK1/2 pathway (11).
Clinical Perspective: microRNA-Based Drug Development
miRNA-based therapeutics have been proven to be effective for treating cardiovascular diseases (1, 96). Since miRNAs can regulate multiple genes using different signaling pathways, they have a great potential as novel therapeutic agents; therapeutic strategies based on miRNA modulation have been widely utilized in angiogenesis, atherosclerosis, ischemic injury, vascular remodeling, hypertrophy, and fibrosis (97, 98).
Treatment options with miRNA-based drugs include suppression of miRNAs to reduce the levels of upregulated miRNAs and substitute missing miRNA to restore the expression of miRNAs in post-ischemic HF (99). A representation of the work-flow leading to miRNA-based drug development is shown in Figure 1. Several approaches to deliver miRNAs to specific target tissues or organs without degradation have been discovered including viral vectors, vesicles, antagomirs or mimics, plasmids and sponges, with a focus on bioavailability and bio-efficacy (100).
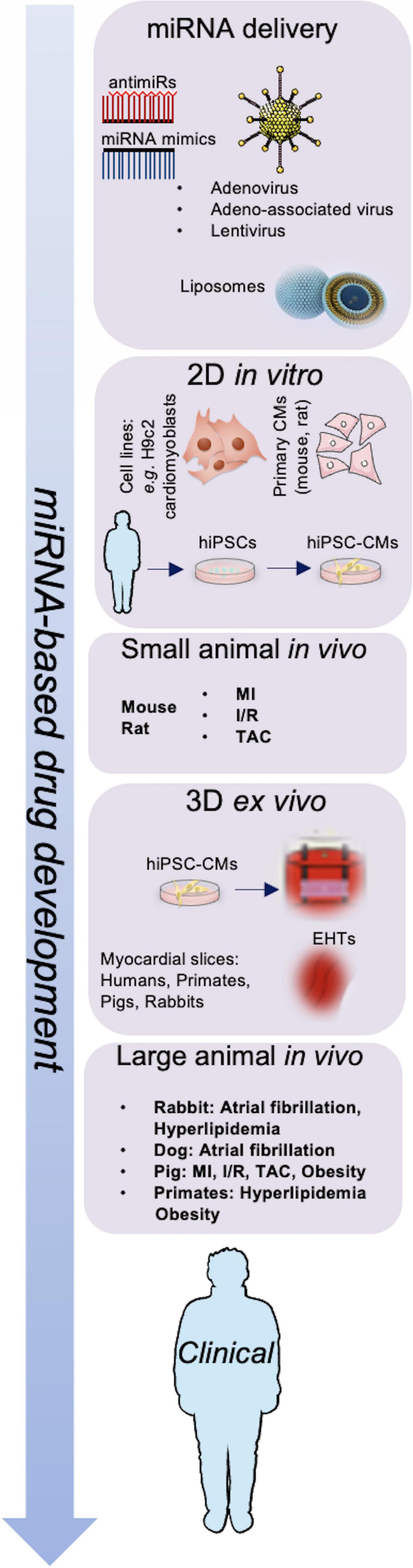
Figure 1. Schematic representation of miRNA-based drug development. Adenovirus, adeno-associated virus (AAV), lentivirus particles, and liposomes are used to deliver miRNA mimics or antimiRs; miRNAs are investigated in vitro, in vivo, and ex vivo models to develop next-generation therapeutics for cardiovascular diseases. CMs, cardiomyocytes; EHTs, engineered heart tissues; hiPSCs, human induced pluripotent stem cells; I/R, ischemia-reperfusion; MI, myocardial infarction; TAC, transverse aortic constriction.
In recent years, miRNA-targeted therapeutics have been tested in clinical trials, mostly in cancer; because of the limited space allowed in this minireview, for these aspects we refer to dedicated reviews (101–104). A successful example of how to develop a miRNA-based therapy in cardiovascular medicine is given by miR-132, which has among its targets FOXO3 and SERCA2a (49, 105).
Several in vitro and in vivo experiments demonstrated that inhibiting miR-132 caused a reduction of cardiac fibrosis, normalization of autophagy, and calcium signaling, and reversal of cardiomyocyte hypertrophy; after a pharmacokinetic assessment, miR-132 inhibition was shown to improve HF in a clinically relevant pig model (96, 106). The following logical step was the clinical investigation: a prospective, randomized, and placebo-controlled phase 1b dose-escalation study was designed to assess safety, pharmacokinetics, target engagement, and exploratory pharmacodynamic effects of miR-132 inhibition, achieved by administering a chemically modified oligonucleotide (CDR132L) containing locked nucleic acid (LNA) nucleotides and phosphonothioate linkages to increase in vivo stability (51). The trial, conducted in patients with stable chronic HF of ischemic origin (20 randomized to CDR132L and 8 to placebo), revealed that CDR132L was overall safe and well-tolerated, confirmed linear plasma pharmacokinetics with no signs of accumulation, and, despite the small size, suggested cardiac functional improvements, reflected in a clinically meaningful median reduction in NT-proBNP and narrowing of the QRS complex (51).
Conclusion
In this review, we have presented the most updated investigations on microRNAs and some primary regulators of inflammation and fibrosis, also discussing the most recent discoveries and actual applications in the clinical scenario.
Author Contributions
GS: conceptualization and supervision. FV and UK: writing—original draft preparation. KD, SW, SSJ, PM, XW, AL, and GS: writing—review and editing. All authors listed have made a substantial, direct, and intellectual contribution to the work, and approved it for publication.
Funding
The Santulli’s Lab was supported in part by the National Institutes of Health (R01-HL146691, R01-DK123259, NIH: R01-HL159062, R01-DK033823, T32-HL144456, and R56-AG066431 to GS), the Diabetes Action Research and Education Foundation (to GS), and the Irma T. Hirschl and Monique Weill-Caulier Trusts (to GS). SW was supported by a Glorney-Raisbeck grant. FV and SSJ hold postdoctoral fellowships from the American Heart Association (AHA-22POST915561 and AHA-21POST836407, respectively).
Conflict of Interest
The authors declare that the research was conducted in the absence of any commercial or financial relationships that could be construed as a potential conflict of interest.
Publisher’s Note
All claims expressed in this article are solely those of the authors and do not necessarily represent those of their affiliated organizations, or those of the publisher, the editors and the reviewers. Any product that may be evaluated in this article, or claim that may be made by its manufacturer, is not guaranteed or endorsed by the publisher.
References
1. Santulli G. MicroRNA: From Molecular Biology to Clinical Practice. G Santulli New York, NY: Springer Nature (2016).
2. Verjans R, Derks WJA, Korn K, Sonnichsen B, Van Leeuwen REW, Schroen B, et al. Functional screening identifies microRNAs as multi-cellular regulators of heart failure. Sci Rep. (2019) 9:6055. doi: 10.1038/s41598-019-41491-9
3. Gioffre S, Chiesa M, Cardinale DM, Ricci V, Vavassori C, Cipolla CM, et al. Circulating microRNAs as potential predictors of anthracycline-induced troponin elevation in breast cancer patients: diverging effects of doxorubicin and epirubicin. J Clin Med. (2020) 9:1418. doi: 10.3390/jcm9051418
4. Kansakar U, Varzideh F, Mone P, Jankauskas SS, Santulli G. Functional role of microRNAs in regulating cardiomyocyte death. Cells (2022) 11:983. doi: 10.3390/cells11060983
5. Kehat I, Molkentin JD. Molecular pathways underlying cardiac remodeling during pathophysiological stimulation. Circulation. (2010) 122:2727–35. doi: 10.1161/CIRCULATIONAHA.110.942268
6. Bretherton R, Bugg D, Olszewski E, Davis J. Regulators of cardiac fibroblast cell state. Matrix Biol. (2020) 9:117–35. doi: 10.1016/j.matbio.2020.04.002
7. Zhao W, Zhao J, Rong J. Pharmacological modulation of cardiac remodeling after myocardial infarction. Oxid Med Cell Longev. (2020) 2020:8815349. doi: 10.1155/2020/8815349
8. Gao F, Kataoka M, Liu N, Liang T, Huang ZP, Gu F, et al. Therapeutic role of miR-19a/19b in cardiac regeneration and protection from myocardial infarction. Nat Commun. (2019) 10:1802. doi: 10.1038/s41467-019-09530-1
9. Yang L, Wang B, Zhou Q, Wang Y, Liu X, Liu Z, et al. MicroRNA-21 prevents excessive inflammation and cardiac dysfunction after myocardial infarction through targeting KBTBD7. Cell Death Dis. (2018) 9:769. doi: 10.1038/s41419-018-0805-5
10. Gu W, Zhan H, Zhou XY, Yao L, Yan M, Chen A, et al. MicroRNA-22 regulates inflammation and angiogenesis via targeting VE-cadherin. FEBS Lett. (2017) 591:513–26. doi: 10.1002/1873-3468.12565
11. Iaconetti C, Polimeni A, Sorrentino S, Sabatino J, Pironti G, Esposito G, et al. Inhibition of miR-92a increases endothelial proliferation and migration in vitro as well as reduces neointimal proliferation in vivo after vascular injury. Basic Res Cardiol. (2012) 107:296. doi: 10.1007/s00395-012-0296-y
12. Soufi-Zomorrod M, Hajifathali A, Kouhkan F, Mehdizadeh M, Rad SM, Soleimani M. MicroRNAs modulating angiogenesis: miR-129-1 and miR-133 act as angio-miR in HUVECs. Tumour Biol. (2016) 37:9527–34. doi: 10.1007/s13277-016-4845-0
13. Feng Q, Li X, Qin X, Yu C, Jin Y, Qian X. PTEN inhibitor improves vascular remodeling and cardiac function after myocardial infarction through PI3k/Akt/VEGF signaling pathway. Mol Med. (2020) 26:111. doi: 10.1186/s10020-020-00241-8
14. Yuan X, Pan J, Wen L, Gong B, Li J, Gao H, et al. MiR-144-3p enhances cardiac fibrosis after myocardial infarction by targeting PTEN. Front Cell Dev Biol (2019) 7:249. doi: 10.3389/fcell.2019.00249
15. Shimada BK, Yang Y, Zhu J, Wang S, Suen A, Kronstadt SM, et al. Extracellular miR-146a-5p induces cardiac innate immune response and cardiomyocyte dysfunction. Immunohorizons. (2020) 4:561–72. doi: 10.4049/immunohorizons.2000075
16. Ye J, Guo R, Shi Y, Qi F, Guo C, Yang L. miR-155 regulated inflammation response by the SOCS1-STAT3-PDCD4 axis in atherogenesis. Mediators Inflamm. (2016) 2016:8060182. doi: 10.1155/2016/8060182
17. Garikipati VNS, Verma SK, Jolardarashi D, Cheng Z, Ibetti J, Cimini M, et al. Therapeutic inhibition of miR-375 attenuates post-myocardial infarction inflammatory response and left ventricular dysfunction via PDK-1-AKT signalling axis. Cardiovasc Res. (2017) 113:938–49. doi: 10.1093/cvr/cvx052
18. Valkov N, King ME, Moeller J, Liu H, Li X, Zhang P. MicroRNA-1-mediated inhibition of cardiac fibroblast proliferation through targeting cyclin D2 and CDK6. Front Cardiovasc Med. (2019) 6:65. doi: 10.3389/fcvm.2019.00065
19. Glass C, Singla DK. MicroRNA-1 transfected embryonic stem cells enhance cardiac myocyte differentiation and inhibit apoptosis by modulating the PTEN/Akt pathway in the infarcted heart. Am J Physiol Heart Circ Physiol. (2011) 301:H2038–49. doi: 10.1152/ajpheart.00271.2011
20. Zhong C, Wang K, Liu Y, Lv D, Zheng B, Zhou Q, et al. miR-19b controls cardiac fibroblast proliferation and migration. J Cell Mol Med. (2016) 20:1191–7. doi: 10.1111/jcmm.12858
21. Yuan J, Chen H, Ge D, Xu Y, Xu H, Yang Y, et al. Mir-21 promotes cardiac fibrosis after myocardial infarction via targeting Smad7. Cell Physiol Biochem. (2017) 42:2207–19. doi: 10.1159/000479995
22. Cao W, Shi P, Ge JJ. miR-21 enhances cardiac fibrotic remodeling and fibroblast proliferation via CADM1/STAT3 pathway. BMC Cardiovasc Disord. (2017) 17:88. doi: 10.1186/s12872-017-0520-7
23. Zhou XL, Xu H, Liu ZB, Wu QC, Zhu RR, Liu JC. miR-21 promotes cardiac fibroblast-to-myofibroblast transformation and myocardial fibrosis by targeting Jagged1. J Cell Mol Med. (2018) 22:3816–24. doi: 10.1111/jcmm.13654
24. Yang F, Chen Q, He S, Yang M, Maguire EM, An W, et al. miR-22 is a novel mediator of vascular smooth muscle cell phenotypic modulation and neointima formation. Circulation. (2018) 137:1824–41. doi: 10.1161/CIRCULATIONAHA.117.027799
25. Huang Y, Qi Y, Du JQ, Zhang DF. MicroRNA-34a regulates cardiac fibrosis after myocardial infarction by targeting Smad4. Expert Opin Ther Targets. (2014) 18:1355–65. doi: 10.1517/14728222.2014.961424
26. Wang X, Morelli MB, Matarese A, Sardu C, Santulli G. Cardiomyocyte-derived exosomal microRNA-92a mediates post-ischemic myofibroblast activation both in vitro and ex vivo. ESC Heart Fail. (2020) 7:284–8. doi: 10.1002/ehf2.12584
27. Nagpal V, Rai R, Place AT, Murphy SB, Verma SK, Ghosh AK, et al. MiR-125b is critical for fibroblast-to-myofibroblast transition and cardiac fibrosis. Circulation. (2016) 133:291–301. doi: 10.1161/circulationaha.115.018174
28. Chistiakov DA, Orekhov AN, Bobryshev YV. The role of miR-126 in embryonic angiogenesis, adult vascular homeostasis, and vascular repair and its alterations in atherosclerotic disease. J Mol Cell Cardiol. (2016) 97:47–55. doi: 10.1016/j.yjmcc.2016.05.007
29. Alique M, Bodega G, Giannarelli C, Carracedo J, Ramirez R. MicroRNA-126 regulates hypoxia-inducible factor-1alpha which inhibited migration, proliferation, and angiogenesis in replicative endothelial senescence. Sci Rep. (2019) 9:7381. doi: 10.1038/s41598-019-43689-3
30. Lu C, Wang X, Ha T, Hu Y, Liu L, Zhang X, et al. Attenuation of cardiac dysfunction and remodeling of myocardial infarction by microRNA-130a are mediated by suppression of PTEN and activation of PI3K dependent signaling. J Mol Cell Cardiol. (2015) 89:87–97. doi: 10.1016/j.yjmcc.2015.10.011
31. Li P, Yin YL, Guo T, Sun XY, Ma H, Zhu ML, et al. Inhibition of aberrant microRNA-133a expression in endothelial cells by statin prevents endothelial dysfunction by targeting GTP cyclohydrolase 1 in vivo. Circulation. (2016) 134:1752–65. doi: 10.1161/CIRCULATIONAHA.116.017949
32. Chen L, Liu C, Sun D, Wang T, Zhao L, Chen W, et al. MicroRNA-133a impairs perfusion recovery after hindlimb ischemia in diabetic mice. Biosci Rep. (2018) 38:BSR20180346. doi: 10.1042/BSR20180346
33. Liao Y, Li H, Cao H, Dong Y, Gao L, Liu Z, et al. Therapeutic silencing miR-146b-5p improves cardiac remodeling in a porcine model of myocardial infarction by modulating the wound reparative phenotype. Protein Cell. (2021) 12:194–212. doi: 10.1007/s13238-020-00750-6
34. Wei Y, Yan X, Yan L, Hu F, Ma W, Wang Y, et al. Inhibition of microRNA155 ameliorates cardiac fibrosis in the process of angiotensin IIinduced cardiac remodeling. Mol Med Rep. (2017) 16:7287–96. doi: 10.3892/mmr.2017.7584
35. Morelli MB, Shu J, Sardu C, Matarese A, Santulli G. Cardiosomal microRNAs are essential in post-infarction myofibroblast phenoconversion. Int J Mol Sci. (2019) 21:201. doi: 10.3390/ijms21010201
36. Ranjan P, Kumari R, Goswami SK, Li J, Pal H, Suleiman Z, et al. Myofibroblast-derived exosome induce cardiac endothelial cell dysfunction. Front Cardiovasc Med. (2021) 8:676267. doi: 10.3389/fcvm.2021.676267
37. Sun M, Yu H, Zhang Y, Li Z, Gao W. MicroRNA-214 mediates isoproterenol-induced proliferation and collagen synthesis in cardiac fibroblasts. Sci Rep. (2015) 5:18351. doi: 10.1038/srep18351
38. Yuan X, Pan J, Wen L, Gong B, Li J, Gao H, et al. MiR-590-3p regulates proliferation, migration and collagen synthesis of cardiac fibroblast by targeting ZEB1. J Cell Mol Med. (2020) 24:227–37. doi: 10.1111/jcmm.14704
39. Camelliti P, Borg TK, Kohl P. Structural and functional characterisation of cardiac fibroblasts. Cardiovasc Res. (2005) 65:40–51. doi: 10.1016/j.cardiores.2004.08.020
40. Deb A, Ubil E. Cardiac fibroblast in development and wound healing. J Mol Cell Cardiol. (2014) 70:47–55. doi: 10.1016/j.yjmcc.2014.02.017
41. Davis J, Salomonis N, Ghearing N, Lin SC, Kwong JQ, Mohan A, et al. MBNL1-mediated regulation of differentiation RNAs promotes myofibroblast transformation and the fibrotic response. Nat Commun. (2015) 6:10084. doi: 10.1038/ncomms10084
42. Travers JG, Kamal FA, Robbins J, Yutzey KE, Blaxall BC. Cardiac fibrosis: the fibroblast awakens. Circ Res. (2016) 118:1021–40. doi: 10.1161/CIRCRESAHA.115.306565
43. Tallquist MD. Cardiac fibroblasts: from origin to injury. Curr Opin Physiol. (2018) 1:75–9. doi: 10.1016/j.cophys.2017.08.002
44. Fan D, Takawale A, Lee J, Kassiri Z. Cardiac fibroblasts, fibrosis and extracellular matrix remodeling in heart disease. Fibrogenesis Tissue Repair. (2012) 5:15. doi: 10.1186/1755-1536-5-15
45. Francisco J, Zhang Y, Jeong JI, Mizushima W, Ikeda S, Ivessa A, et al. Blockade of fibroblast YAP attenuates cardiac fibrosis and dysfunction through MRTF-A inhibition. JACC Basic Transl Sci. (2020) 5:931–45. doi: 10.1016/j.jacbts.2020.07.009
47. Ghelani HS, Rachchh MA, Gokani RH. MicroRNAs as newer therapeutic targets: a big hope from a tiny player. J Pharmacol Pharmacother. (2012) 3:217–27. doi: 10.4103/0976-500X.99416
48. Katsuda T, Ikeda S, Yoshioka Y, Kosaka N, Kawamata M, Ochiya T. Physiological and pathological relevance of secretory microRNAs and a perspective on their clinical application. Biol Chem. (2014) 395:365–73. doi: 10.1515/hsz-2013-0222
49. Ucar A, Gupta SK, Fiedler J, Erikci E, Kardasinski M, Batkai S, et al. The miRNA-212/132 family regulates both cardiac hypertrophy and cardiomyocyte autophagy. Nat Commun. (2012) 3:1078. doi: 10.1038/ncomms2090
50. Eskildsen TV, Schneider M, Sandberg MB, Skov V, Bronnum H, Thomassen M, et al. The microRNA-132/212 family fine-tunes multiple targets in angiotensin II signalling in cardiac fibroblasts. J Renin Angiotensin Aldosterone Syst. (2015) 16:1288–97. doi: 10.1177/1470320314539367
51. Taubel J, Hauke W, Rump S, Viereck J, Batkai S, Poetzsch J, et al. Novel antisense therapy targeting microRNA-132 in patients with heart failure: results of a first-in-human Phase 1b randomized, double-blind, placebo-controlled study. Eur Heart J. (2021) 42:178–88. doi: 10.1093/eurheartj/ehaa898
52. Yang B, Lin H, Xiao J, Lu Y, Luo X, Li B, et al. The muscle-specific microRNA miR-1 regulates cardiac arrhythmogenic potential by targeting GJA1 and KCNJ2. Nat Med. (2007) 13:486–91. doi: 10.1038/nm1569
53. Marceca GP, Nigita G, Calore F, Croce CM. MicroRNAs in skeletal muscle and hints on their potential role in muscle wasting during cancer cachexia. Front Oncol. (2020) 10:607196. doi: 10.3389/fonc.2020.607196
54. Surina S, Fontanella RA, Scisciola L, Marfella R, Paolisso G, Barbieri M. miR-21 in human cardiomyopathies. Front Cardiovasc Med. (2021) 8:767064. doi: 10.3389/fcvm.2021.767064
55. Wang T, Zhang L, Shi C, Sun H, Wang J, Li R, et al. TGF-beta-induced miR-21 negatively regulates the antiproliferative activity but has no effect on EMT of TGF-beta in HaCaT cells. Int J Biochem Cell Biol. (2012) 44:366–76. doi: 10.1016/j.biocel.2011.11.012
56. Wang JY, Gao YB, Zhang N, Zou DW, Wang P, Zhu ZY, et al. miR-21 overexpression enhances TGF-beta1-induced epithelial-to-mesenchymal transition by target smad7 and aggravates renal damage in diabetic nephropathy. Mol Cell Endocrinol. (2014) 392:163–72. doi: 10.1016/j.mce.2014.05.018
57. Ramanujam D, Schon AP, Beck C, Vaccarello P, Felician G, Dueck A, et al. MicroRNA-21-dependent macrophage-to-fibroblast signaling determines the cardiac response to pressure overload. Circulation. (2021) 143:1513–25. doi: 10.1161/CIRCULATIONAHA.120.050682
58. Humeres C, Shinde AV, Hanna A, Alex L, Hernandez SC, Li R, et al. Smad7 effects on TGF-beta and ErbB2 restrain myofibroblast activation and protect from postinfarction heart failure. J Clin Invest. (2022) 132:e146926. doi: 10.1172/JCI146926
59. Gao CK, Liu H, Cui CJ, Liang ZG, Yao H, Tian Y. Roles of MicroRNA-195 in cardiomyocyte apoptosis induced by myocardial ischemia-reperfusion injury. J Genet. (2016) 95:99–108. doi: 10.1007/s12041-016-0616-3
60. Jazbutyte V, Fiedler J, Kneitz S, Galuppo P, Just A, Holzmann A, et al. MicroRNA-22 increases senescence and activates cardiac fibroblasts in the aging heart. Age (Dordr). (2013) 35:747–62. doi: 10.1007/s11357-012-9407-9
61. Song Z, Li G. Role of specific microRNAs in regulation of vascular smooth muscle cell differentiation and the response to injury. J Cardiovasc Transl Res. (2010) 3:246–50. doi: 10.1007/s12265-010-9163-0
62. Huang ZP, Wang DZ. miR-22 in smooth muscle cells: a potential therapy for cardiovascular disease. Circulation. (2018) 137:1842–5. doi: 10.1161/CIRCULATIONAHA.118.033042
63. Kansakar U, Jankauskas SS, Gambardella J, Santulli G. Targeting the phenotypic switch of vascular smooth muscle cells to tackle atherosclerosis. Atherosclerosis. (2021) 324:117–20. doi: 10.1016/j.atherosclerosis.2021.03.034
64. Gudkov AV, Gurova KV, Komarova EA. Inflammation and p53: a tale of two stresses. Genes Cancer. (2011) 2:503–16. doi: 10.1177/1947601911409747
65. Uehara I, Tanaka N. Role of p53 in the regulation of the inflammatory tumor microenvironment and tumor suppression. Cancers (Basel). (2018) 10:219. doi: 10.3390/cancers10070219
66. Thum T, Gross C, Fiedler J, Fischer T, Kissler S, Bussen M, et al. MicroRNA-21 contributes to myocardial disease by stimulating MAP kinase signalling in fibroblasts. Nature. (2008) 456:980–4. doi: 10.1038/nature07511
67. Bejerano T, Etzion S, Elyagon S, Etzion Y, Cohen S. Nanoparticle delivery of miRNA-21 mimic to cardiac macrophages improves myocardial remodeling after myocardial infarction. Nano Lett. (2018) 18:5885–91. doi: 10.1021/acs.nanolett.8b02578
68. DeBerge M, Glinton K, Subramanian M, Wilsbacher LD, Rothlin CV, Tabas I, et al. Macrophage AXL receptor tyrosine kinase inflames the heart after reperfused myocardial infarction. J Clin Invest. (2021) 131:e139576. doi: 10.1172/JCI139576
69. Ceppi M, Pereira PM, Dunand-Sauthier I, Barras E, Reith W, Santos MA MicroRNA-155 modulates the interleukin-1 signaling pathway in activated human monocyte-derived dendritic cells. Proc Natl Acad Sci USA. (2009) 106:2735–40. doi: 10.1073/pnas.0811073106
70. Fuentes-Calvo I, Martinez-Salgado C. Sos1 modulates extracellular matrix synthesis, proliferation, and migration in fibroblasts. Front Physiol. (2021) 12:645044. doi: 10.3389/fphys.2021.645044
71. Wang C, Zhang C, Liu L, A X, Chen B, Li Y, et al. Macrophage-derived mir-155-containing exosomes suppress fibroblast proliferation and promote fibroblast inflammation during cardiac injury. Mol Ther. (2017) 25:192–204. doi: 10.1016/j.ymthe.2016.09.001
72. Heymans S, Corsten MF, Verhesen W, Carai P, Van Leeuwen RE, Custers K, et al. Macrophage microRNA-155 promotes cardiac hypertrophy and failure. Circulation. (2013) 128:1420–32.
73. Jankauskas SS, Gambardella J, Sardu C, Lombardi A, Santulli G. Functional role of miR-155 in the pathogenesis of diabetes mellitus and its complications. Noncoding RNA. (2021) 7:39. doi: 10.3390/ncrna7030039
74. Tahamtan A, Teymoori-Rad M, Nakstad B, Salimi V. Anti-inflammatory microRNAs and their potential for inflammatory diseases treatment. Front Immunol. (2018) 9:1377. doi: 10.3389/fimmu.2018.01377
75. Halade GV, Ma Y, Ramirez TA, Zhang J, Dai Q, Hensler JG, et al. Reduced BDNF attenuates inflammation and angiogenesis to improve survival and cardiac function following myocardial infarction in mice. Am J Physiol Heart Circ Physiol. (2013) 305:H1830–42. doi: 10.1152/ajpheart.00224.2013
76. Guo L, Akahori H, Harari E, Smith SL, Polavarapu R, Karmali V, et al. CD163+ macrophages promote angiogenesis and vascular permeability accompanied by inflammation in atherosclerosis. J Clin Invest. (2018) 128:1106–24. doi: 10.1172/JCI93025
77. Mouton AJ, Ma Y, Rivera Gonzalez OJ, Daseke MJ II, Flynn ER, Freeman TC, et al. Fibroblast polarization over the myocardial infarction time continuum shifts roles from inflammation to angiogenesis. Basic Res Cardiol. (2019) 114:6. doi: 10.1007/s00395-019-0715-4
78. Chen ZL, Chen YX, Zhou J, Li Y, Gong CY, Wang XB. LncRNA HULC alleviates HUVEC inflammation and improves angiogenesis after myocardial infarction through down-regulating miR-29b. Eur Rev Med Pharmacol Sci. (2020) 24:6288–98. doi: 10.26355/eurrev_202006_21527
79. Wu X, Reboll MR, Korf-Klingebiel M, Wollert KC. Angiogenesis after acute myocardial infarction. Cardiovasc Res. (2021) 117:1257–73. doi: 10.1093/cvr/cvaa287
80. Dridi H, Santulli G, Gambardella J, Jankauskas SS, Yuan Q, Yang J, et al. IP3 receptor orchestrates maladaptive vascular responses in heart failure. J Clin Invest. (2022) 132:e152859. doi: 10.1172/JCI152859
81. Yan B, Yao J, Liu JY, Li XM, Wang XQ, Li YJ, et al. lncRNA-MIAT regulates microvascular dysfunction by functioning as a competing endogenous RNA. Circ Res. (2015) 116:1143–56. doi: 10.1161/CIRCRESAHA.116.305510
82. Fan Y, Ding S, Sun Y, Zhao B, Pan Y, Wan J. MiR-377 regulates inflammation and angiogenesis in rats after cerebral ischemic injury. J Cell Biochem. (2018) 119:327–37. doi: 10.1002/jcb.26181
83. Xiao Y, Zhao J, Tuazon JP, Borlongan CV, Yu G. MicroRNA-133a and myocardial infarction. Cell Transplant. (2019) 28:831–8. doi: 10.1177/0963689719843806
84. Scarlatescu AI, Micheu MM, Popa-Fotea NM, Dorobantu M. MicroRNAs in acute ST elevation myocardial infarction-a new tool for diagnosis and prognosis: therapeutic implications. Int J Mol Sci. (2021) 22:4799. doi: 10.3390/ijms22094799
85. Zhang X, Yuan S, Li H, Zhan J, Wang F, Fan J, et al. The double face of miR-320: cardiomyocytes-derived miR-320 deteriorated while fibroblasts-derived miR-320 protected against heart failure induced by transverse aortic constriction. Signal Transduct Target Ther. (2021) 6:69. doi: 10.1038/s41392-020-00445-8
86. Lesizza P, Prosdocimo G, Martinelli V, Sinagra G, Zacchigna S, Giacca M. Single-dose intracardiac injection of pro-regenerative microRNAs improves cardiac function after myocardial infarction. Circ Res. (2017) 120:1298–304. doi: 10.1161/CIRCRESAHA.116.309589
87. Jiang BH, Liu LZ. PI3K/PTEN signaling in angiogenesis and tumorigenesis. Adv Cancer Res. (2009) 102:19–65. doi: 10.1016/s0065-230x(09)02002-8
88. Ma J, Sawai H, Ochi N, Matsuo Y, Xu D, Yasuda A, et al. PTEN regulates angiogenesis through PI3K/Akt/VEGF signaling pathway in human pancreatic cancer cells. Mol Cell Biochem. (2009) 331:161–71.
89. Bonauer A, Carmona G, Iwasaki M, Mione M, Koyanagi M, Fischer A, et al. MicroRNA-92a controls angiogenesis and functional recovery of ischemic tissues in mice. Science. (2009) 324:1710–3. doi: 10.1126/science.1174381
90. Segers VFM, Brutsaert DL, De Keulenaer GW. Cardiac remodeling: endothelial cells have more to say than just NO. Front Physiol. (2018) 9:382. doi: 10.3389/fphys.2018.00382
91. Herrera-Zelada N, Zuniga-Cuevas U, Ramirez-Reyes A, Lavandero S, Riquelme JA. Targeting the endothelium to achieve cardioprotection. Front Pharmacol. (2021) 12:636134. doi: 10.3389/fphar.2021.636134
92. Fish JE, Santoro MM, Morton SU, Yu S, Yeh RF, Wythe JD, et al. miR-126 regulates angiogenic signaling and vascular integrity. Dev Cell. (2008) 15:272–84. doi: 10.1016/j.devcel.2008.07.008
93. Santulli G, Wronska A, Uryu K, Diacovo TG, Gao M, Marx SO, et al. A selective microRNA-based strategy inhibits restenosis while preserving endothelial function. J Clin Invest. (2014) 124:4102–14. doi: 10.1172/JCI76069
94. Yu P, Venkat P, Chopp M, Zacharek A, Shen Y, Ning R, et al. Role of microRNA-126 in vascular cognitive impairment in mice. J Cereb Blood Flow Metab. (2019) 39:2497–511. doi: 10.1177/0271678X18800593
95. Joris V, Gomez EL, Menchi L, Lobysheva I, Di Mauro V, Esfahani H, et al. MicroRNA-199a-3p and microRNA-199a-5p take part to a redundant network of regulation of the NOS (NO synthase)/NO pathway in the endothelium. Arterioscler Thromb Vasc Biol. (2018) 38:2345–57. doi: 10.1161/ATVBAHA.118.311145
96. Foinquinos A, Batkai S, Genschel C, Viereck J, Rump S, Gyongyosi M, et al. Preclinical development of a miR-132 inhibitor for heart failure treatment. Nat Commun. (2020) 11:633. doi: 10.1038/s41467-020-14349-2
97. Lucas T, Bonauer A, Dimmeler S. RNA therapeutics in cardiovascular disease. Circ Res. (2018) 123:205–20. doi: 10.1161/circresaha.117.311311
98. Marracino L, Fortini F, Bouhamida E, Camponogara F, Severi P, Mazzoni E, et al. Adding a “notch” to cardiovascular disease therapeutics: a MicroRNA-based approach. Front Cell Dev Biol. (2021) 9:695114. doi: 10.3389/fcell.2021.695114
99. Das A, Samidurai A, Salloum FN. Deciphering non-coding RNAs in cardiovascular health and disease. Front Cardiovasc Med. (2018) 5:73. doi: 10.3389/fcvm.2018.00073
100. Winkle M, El-Daly SM, Fabbri M, Calin GA. Noncoding RNA therapeutics – challenges and potential solutions. Nat Rev Drug Discov. (2021) 20:629–51. doi: 10.1038/s41573-021-00219-z
101. Rupaimoole R, Slack FJ. MicroRNA therapeutics: towards a new era for the management of cancer and other diseases. Nat Rev Drug Discov. (2017) 16:203–22. doi: 10.1038/nrd.2016.246
102. Xue J, Yang J, Luo M, Cho WC, Liu X. MicroRNA-targeted therapeutics for lung cancer treatment. Expert Opin Drug Discov. (2017) 12:141–57. doi: 10.1080/17460441.2017.1263298
103. Miroshnichenko S, Patutina O. Enhanced inhibition of tumorigenesis using combinations of miRNA-targeted therapeutics. Front Pharmacol. (2019) 10:488. doi: 10.3389/fphar.2019.00488
104. Lamberti MJ, Nigro A, Casolaro V, Rumie Vittar NB, Dal Col J. Damage-Associated molecular patterns modulation by microRNA: relevance on immunogenic cell death and cancer treatment outcome. Cancers (Basel). (2021) 13:2566. doi: 10.3390/cancers13112566
105. Lei Z, Wahlquist C, El Azzouzi H, Deddens JC, Kuster D, Van Mil A, et al. miR-132/212 impairs cardiomyocytes contractility in the failing heart by suppressing SERCA2a. Front Cardiovasc Med. (2021) 8:592362. doi: 10.3389/fcvm.2021.592362
Keywords: cardiac remodeling, clinical trials, drug development, epigenetics, heart failure, ischemic heart disease, non-coding RNA, oxidative stress
Citation: Varzideh F, Kansakar U, Donkor K, Wilson S, Jankauskas SS, Mone P, Wang X, Lombardi A and Santulli G (2022) Cardiac Remodeling After Myocardial Infarction: Functional Contribution of microRNAs to Inflammation and Fibrosis. Front. Cardiovasc. Med. 9:863238. doi: 10.3389/fcvm.2022.863238
Received: 27 January 2022; Accepted: 08 March 2022;
Published: 13 April 2022.
Edited by:
Ariana Foinquinos, AstraZeneca, SwedenReviewed by:
Christian Bär, Hannover Medical School, GermanyCopyright © 2022 Varzideh, Kansakar, Donkor, Wilson, Jankauskas, Mone, Wang, Lombardi and Santulli. This is an open-access article distributed under the terms of the Creative Commons Attribution License (CC BY). The use, distribution or reproduction in other forums is permitted, provided the original author(s) and the copyright owner(s) are credited and that the original publication in this journal is cited, in accordance with accepted academic practice. No use, distribution or reproduction is permitted which does not comply with these terms.
*Correspondence: Gaetano Santulli, Z3NhbnR1bGxpMDAxQGdtYWlsLmNvbQ==
†These authors share first authorship