- 1Department of Neurosurgery, Liaoning Cancer Hospital and Institute, Cancer Hospital of China Medical University, Cancer Hospital of Dalian University of Technology, Shenyang, China
- 2Institute of Cancer Medicine, Dalian University of Technology, Dalian, China
- 3Central Laboratory, Liaoning Cancer Hospital and Institute, Cancer Hospital of China Medical University, Cancer Hospital of Dalian University of Technology, Shenyang, China
Cancer is a major killer threatening modern human health and a leading cause of death worldwide. Due to the heterogeneity and complexity of cancer, traditional treatments have limited effectiveness. To address this problem, an increasing number of researchers and medical professionals are working to develop new ways to treat cancer. Bacteria have chemotaxis that can target and colonize tumor tissue, as well as activate anti-tumor immune responses, which makes them ideal for biomedical applications. With the rapid development of nanomedicine and synthetic biology technologies, bacteria are extensively used as carriers for drug delivery to treat tumors, which holds the promise of overcoming the limitations of conventional cancer treatment regimens. This paper summarizes examples of anti-cancer drugs delivered by bacterial carriers, and their strengths and weaknesses. Further, we emphasize the promise of bacterial carrier delivery systems in clinical translation.
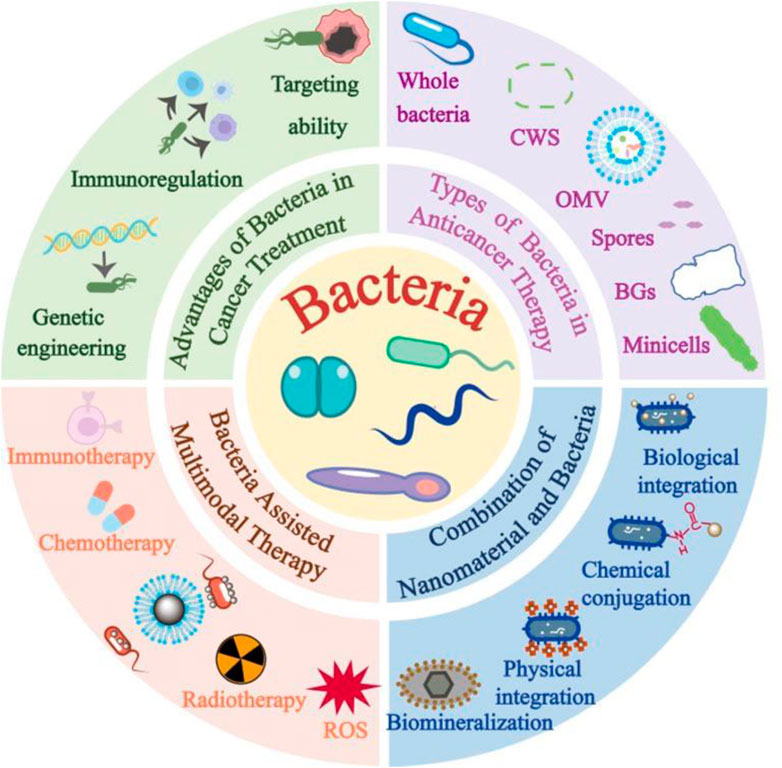
GRAPHICAL ABSTRACT | Schematic illustration of the application of bacterial carriers in anti-tumor therapy, including the advantages of bacterial carriers in cancer treatment, the classification of bacterial carriers, bacteria combined with nanomaterials for cancer therapy, and the strategies for constructive integration of bacteria-nanomaterials.
1 Introduction
Despite the tremendous advances in medical technology, cancer remains an insurmountable challenge. According to the WHO, in 2022, there were nearly 20 million new cases of cancer and 9.7 million deaths from cancer worldwide (Ferlay et al., 2024; Bray et al., 2024). It is estimated that about one-fifth of people will develop cancer in their lifetime, while about one in nine men and one in twelve women will die of cancer. Demographically based projections indicate that by 2050, there will be 35 million new cases of cancer (Bray et al., 2024). Furthermore, cancer is not only a serious threat to people’s health but also carries significant social and macro costs (Chen et al., 2023). At present, conventional treatments for cancer include surgery, chemotherapy, and radiotherapy. In addition, there are new therapies such as gene therapy, immunotherapy, and stem cell therapy (Mathis et al., 2019). Despite the good progress made in all these cancer treatments, each has its limitations and side effects (Maman and Witz, 2018), and therefore it has become necessary to develop a more promising solution.
Drug Delivery Systems (DDS) are innovative and viable therapeutic modalities that fully regulate the dissemination of drugs in the body concerning dosage, space, and time (Park et al., 2022). The use of nanomaterials as drug carriers to release drugs into target tissues or cells through specific pathways is a new type of drug delivery technology. This technology can increase drug utilization, improve therapeutic effects, reduce costs, and minimize side effects, and has now become the forefront of research in medicine. Currently, common drug nanocarriers can be broadly categorized into lipid nanoparticles, polymer nanoparticles, and inorganic nanoparticles, with lipid nanoparticles and polymer nanoparticles being the most commonly used drug delivery carriers. However, these delivery systems suffer from poor stability and barrier penetration, low cellular uptake, and difficulty assembling functional components, making it difficult for therapeutic agents to reach the tumor site (Cao and Liu, 2020). Therefore, developing new drug delivery carriers to improve the effectiveness of antitumor therapy has become crucial.
Bacteria are the oldest, most abundant, and most widely distributed organisms in the world, colonizing various physiological organs in the human body and regulating organ function (Cubillos-Ruiz et al., 2021; Tamburini et al., 2016; Stewart et al., 2018). As early as the 18th century (Starnes, 1993; Cann et al., 2002), it was recorded that certain bacterial infections in cancer patients with malignant disease could be relieved. In the early 19th century, Vautier recorded that tumors receded in patients who suffered from gangrene (Mowday et al., 2016). Subsequently, during the late 1800s, William Coley used Streptococcus pyogenes infections to regress patients’ sarcomas, which inaugurated bacterial-mediated microbial therapy (Coley, 1893). The results showed that various species of bacteria such as Streptococcus, Salmonella, Escherichia coli, Clostridium, Bifidobacterium, Listeria, and Lactococcus were effective in anticancer therapy (Yin et al., 2022). Some bacterial-based treatment regimens are also widely used for their excellent efficacy: for example, Mycobacterium bovis bacillus Calmette-Guérin (BCG) vaccine, which has been successfully used in the therapy of non-muscle invasive bladder cancer (Pettenati and Ingersoll, 2018). In the mid-1990s, recombinant DNA technology produced more efficient, safer, and functionally abundant engineered bacteria (Anderson et al., 2006). However, their application has been limited due to lag, uncontrollability, and inadequate targeting efficiency. Nowadays, with the rise and advancement of nanomaterials science and synthetic biology technology, the safety and efficacy of bacterial therapies have continued to improve, rekindling the enthusiasm for bacterial therapies (Lin et al., 2021; Redenti et al., 2024; Scott et al., 2017; Solomon et al., 2015). DDS based on bacterial carriers is an innovative and promising approach. Bacterial carriers include bacteria and bacterial derivatives. Compared to synthetic materials such as liposomes and polymers, bacteria, and their derivatives have many attractive properties. Firstly, bacteria have good tumor colonization ability and can accumulate at the lesion site. Moreover, bacteria are biocompatible and can pass through the body’s physiological barriers. In addition, bacteria have the advantage of being easy to manipulate for gene editing and their derivatives can cause immune activation. The flagellum of bacteria makes them chemotaxis, allowing for more efficient drug delivery and a quantum leap in anticancer therapeutic efficacy (Figure 1) (Wu et al., 2022).
In this article, we summarize the advantages of bacterial treatment of tumors and show the classification of bacterial carriers and examples of the delivery of anticancer drugs by various types of carriers. In addition, we concentrate on the application of combining nanotechnology with bacterial carriers in antitumor therapy. This work also summarizes current strategies for combining nanoparticles with bacteria and bacterial derivatives. Finally, we emphasize the advantages and disadvantages of bacterial carriers when used to treat cancer and their potential for clinical translation.
2 Advantages of bacteria in cancer treatment
2.1 Targeting the tumor microenvironment (TME)
The TME includes hypoxia, acidic pH, angiogenesis, and immunosuppression. Due to the rapid growth of tumor tissues with active metabolism, it increases its oxygen demand. At the same time, the vascular system in and around the tumor is disorganized and irregular, resulting in inadequate oxygen diffusion (Jain, 2013). Therefore, the central area of the tumor presents a necrotic, hypoxic environment with an oxygen partial pressure as low as 7–28 mmHg, whereas the oxygen partial pressure of normal tissue is 40–60 mmHg (West and Slevin, 2019). Bacteria, as natural prokaryotic cells, can actively migrate into the hypoxic TME by their autonomous propulsion and anaerobic properties (Kasinskas and Forbes, 2006). Live facultative/obligate anaerobes such as E. coli, Salmonella,Bifidobacterium bifidum, and S. pyogenes can sense hypoxic environments by using their chemoreceptors, which are naturally targeted to hypoxic and necrotic areas of tumors (Kucerova and Cervinkova, 2016).
Due to insufficient oxygen supply, tumor cells are limited to metabolizing energy through anaerobic fermentation, which leads to lactic acid accumulation and the formation of an acidic tumor microenvironment (Kumari et al., 2021). Tumor acidic environment can also induce bacterial-specific gene activation. Flentie et al. (2012) co-cultured Salmonella with melanoma cells or colon cancer cells and identified 5 genes that can be activated specifically by cancer cells. Further studies revealed that the activation of these genes may be caused by the acidic microenvironment of the tumor cells, suggesting that the bacteria are capable of responding positively to the acidic TME. Tumor cells release a substantial quantity of vascular endothelial growth factor during growth, inducing the formation of chaotic and irregular tumor-specific blood vessels. These blood vessels can enclose the bacteria, not only promoting bacterial colonization but also providing an adequate supply of nutrients to the bacteria, which is conducive to bacterial proliferation (Khalaf et al., 2021). Hypoxia also leads to suppression of local immune cell function resulting in an immunosuppressive microenvironment (Chouaib et al., 2017). In tumor tissues, the ability of immune cells to recognize and kill bacteria is inhibited, which provides a favorable environment for bacterial survival. It has been shown that bacteria are more likely to survive in a tumor-immunosuppressive environment (Stritzker et al., 2010; Tian et al., 2022).
In conclusion, the characteristics of the TME and the interactions between bacteria and tumors are not only important for the natural targeting of bacteria to tumors but also provide a basis for modifying bacteria and improving their targeting ability.
2.2 Colonization ability
Most bacteria used for tumor therapy have flagella, which mainly perform chemotaxis, motile, and invasive functions. These functions performed by the flagellum can help the bacteria to better penetrate the intratumor tissue, which is crucial for bacterial colonization in tumors (Min et al., 2008; Zhao et al., 2005; St Jean et al., 2008; Kasinskas and Forbes, 2007).
2.3 Immunomodulatory effects
Bacteria and their secreted metabolites such as peptidoglycan, and lipopolysaccharide (LPS) augment the antigenicity of tumors and provide potent immunostimulatory signals. They combine with pattern recognition receptors (PRRs) expressed by immune cells such as dendritic cells (DCs) and macrophages, initiating an immune response that allows the immune system to recognize and kill tumor cells. Bacteria and their metabolites also initiate an adaptive immune response to kill tumor cells via natural killer cells, CD4+, and CD8+ T cells (Zitvogel et al., 2016).
Furthermore, certain components in bacteria can also cause phenotypic transformation of immune cells. Flagellin was found to convert pro-tumor macrophages into anti-tumor macrophages and to transform the immunosuppressive microenvironment into an environment of normal immune function (Zheng et al., 2017).
2.4 Can be genetically engineered to enhance therapeutic functions
Genetic engineering of bacteria to develop safer and more effective cancer treatments is a major focus of bacterial anticancer therapies. Bacterial genetic modification can reduce bacterial cytotoxic effects to improve safety (Hill et al., 2011)and enhance bacterial targeting, invasiveness, and tumor cell killing (Yoon et al., 2017).
In addition, synthetic biology methods to implant sensing and responsive genetic circuits in bacteria enable bacteria to synthesize or release drugs in response to specific sensing stimuli. Moreover, combining multiple response circuits with bacteria through synthetic biology approaches could enable engineered bacteria to respond to the complex physiological environment of the organism. This allows for better targeting and control of the bacterial carrier delivery system, enabling precise drug delivery and reducing drug side effects (Feng et al., 2023).
3 Types of bacteria in anticancer therapy
3.1 Whole bacteria
Whole bacteria can be categorized into inactivated and live bacteria, which can be improved through genetic engineering or other technological methods to enhance their ability to deliver drugs to treat cancer.
Inactivation of bacteria is a special inactivation technique that prevents the bacteria from growing and reproducing while retaining their original structure and characteristics. Records of inactivated bacteria for the treatment of cancer date as far back as the advent of “Coley’s toxin” in the 19th century, when William Coley injected inactivated S. pyogenes and Serratia marcescens into sarcoma patients (Hoption Cann et al., 2003). Following Coley’s work, Teoh’s laboratory demonstrated that the inactivation of Clostridium sporogene, a naturally tumorigenic bacterium, significantly reduced the proliferation of CT26 and HCT116 colorectal cancer cells to 57.3% and 26.2%, respectively (Figure 2) (Bhave et al., 2015). However, inactivated bacteria often suffer from insufficient anti-tumor effects. To address this problem, Yang and Xuan incorporated 125I/131I into inactivated bacterial carriers, which can prolong the presence of radioactive iodine at the site of the lesion, thus enabling internal radioisotope therapy (IRT) of the tumor (Pei et al., 2022).
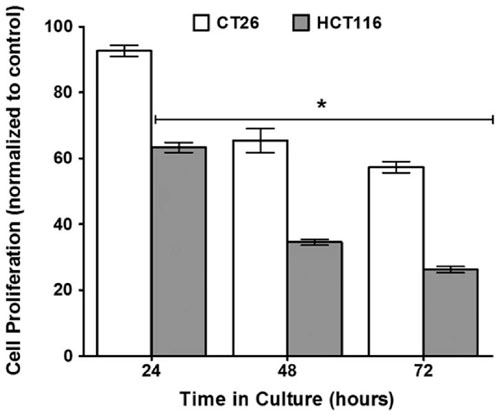
Figure 2. Diagram illustrating that inactivation of Clostridium sporogene, a naturally tumorigenic bacterium, significantly reduced the proliferation of CT26 and HCT116. Adapted from reference (Bhave et al., 2015). Nature, Copyright 2016.
In contrast to inactivated bacteria, live bacteria can actively target most tumors, including metastases (Forbes, 2010), and most live bacteria can better penetrate and colonize intratumoral tissues depending on the flagellum (Felgner et al., 2018). However, the toxicity and uncontrolled immunogenicity of the bacteria greatly limit the use of live bacterial therapies in practice. Besides, bacteria can suppress macrophage migration and disrupt the integration of the barrier established by epithelial cells, thereby allowing tumors to diffuse and invade surrounding healthy tissues (Gagnaire et al., 2017). Therefore, improving the safety of bacterial therapy has become a key issue in bacterial cancer therapy.
The attenuated modification of bacteria is a common method to improve the safety of bacterial therapies. The BCG vaccine is a live bacterial preparation made from attenuated suspensions of Mycobacterium bovis by Charles Calmette and Camille Guérin in 1900 (Kamat et al., 2015). When they cultured M. bovis in bile-containing media, they found that the bacterial virulence gradually decreased and after more than 1,000 passages the attenuated strain that we use today was obtained. Bladder instillation of BCG is a standard treatment option for patients with high-risk non-muscle invasive bladder cancer in the clinic. Compared with traditional means of inactivation and attenuation, obtaining attenuated bacteria by knocking out virulence factors through genetic engineering techniques not only reduces the pathogenicity of the bacteria but also preserves their tumor-targeting properties. For example, an attenuated Salmonella typhimurium strain (named VNP20009) obtained by deletion of the purI and msbB genes not only dramatically improved safety, but also promoted their accumulation in the tumor tissue (Toso et al., 2002).
Subsequently, a large number of researchers have concentrated on attenuating the virulence of various bacteria, including Salmonella, Clostridium, E. coli, and Listeria, through genetic and chemical modifications (Felgner et al., 2018; Mercado-Lubo et al., 2016; Fritz et al., 2016; Dang et al., 2001; Chowdhury et al., 2019; Zhou et al., 2018). In addition, engineered bacteria are a promising carrier for drug delivery, and today substantial studies are using engineered bacteria for targeted delivery of interfering RNA (Jiang et al., 2007), DNA vaccines (Kong et al., 2012), proteins (Binder et al., 2013), and small molecule therapeutics (Xie et al., 2017) for the treatment of malignant tumors. Protein therapeutic molecules, including antibodies (Daassi et al., 2020), inflammatory factors (Schmitt and Greten, 2021), metabolism-blocking proteins (Liu et al., 2017), enzymes (Yu et al., 2024), and apoptosis-inducing agents (Hu and Kavanagh, 2003) have also been successfully combined with engineered bacteria for targeted therapy of tumors (Table 1).
3.2 Bacterial skeleton
Bacterial skeleton includes bacterial ghosts (BGs), and cell wall skeleton (CWs). BGs are cavities formed by lysing Gram-negative bacteria using φX174 phage lysin gene E. BGs have large inner space and intact outer walls, and can be applied as a drug delivery carrier to load cargoes of drugs, antigens, and nucleic acids (Rabea et al., 2020; Xie et al., 2020; Tabrizi et al., 2004). It has been shown that encapsulation of doxorubicin (DOX) in BG derived from Mannheimia haemolytica not only targets human colorectal adenocarcinoma cells (Caco-2) releasing large quantities of DOX within tumor cells but also enhances cytotoxicity against tumor cells (Paukner et al., 2004). In addition, BG retains various antigenic components of the bacteria, allowing them to also be used as a biologic agent and immune adjuvant for immunotherapy (Kudela et al., 2005). For example, when BG carries a plasmid, it is recognized and phagocytosed by antigen-presenting cells and efficiently activates CD4+ and CD8+ T cells to generate an anti-tumor immune response (Warrier et al., 2019).
CWS is prepared from bacteria by hydrothermal treatment and is an agonist of TLR2 and TLR4 and can be used as an immune adjuvant. CWS from M. bovis BCG has Immune activation ability (Ni et al., 2017). Researchers have found that the shape of CWS affects DC internalization (Yoo et al., 2002). The results indicated that the CWS of Lactobacillus rod-shaped exhibited the greatest amount of DC internalization compared to other shapes. Also, rod-shaped Lactobacillus CWS upregulated immunoreactive cytokines, which can be used for TME regulation or loading stimulatory signals in next-generation delivery systems (Seya et al., 2001).
3.3 Bacterial components
Bacterial components include magnetosomes, spore microcells, and bacterial outer membrane vesicles. Magnetotactic bacteria (MTB) were found by American scholars in 1975 (Makela et al., 2022). This group of bacteria can be driven by flagella to move in the direction of magnetic lines of force and are well targeted in cancer therapy. Magnetosomes are intracellular organelles of MTB containing magnetic minerals such as magnetite Fe3O4 or greigite (Fe3S4) encapsulated by proteolipid membranes (Grünberg et al., 2004; Schüler, 2008). Magnetosome membranes are abundant in primary amine groups, which can bind certain chemotherapeutic drugs to the amine groups on the surface of the magnetosome for cancer therapy. Polymers wrapped with small molecule drugs can be attached to the magnetic vesicles to improve their drug-carrying efficiency and stability. Alternatively, chemical drugs with amine groups can be directly coupled to magnetosomes by crosslinking agents (Sun et al., 2011). Some researchers used polyethyleneimine (PEI) as a cross-linking agent to enable the magnetosomes to bind the antitumor drug DOX (loading rate of 57.7%) and small interfering RNAs (siRNAs) via a hydrazone bond (Long et al., 2018). In addition, genipin and polyglutamic acid can be selected as double cross-linking agents to load the anticancer drugs acitretin (Ara-c) and doxycycline (DNR) on the surface of the magnetic vesicles (68.4% encapsulation and 32.4% drug loading rate for Ara-c and 36.1% encapsulation and 17.9% drug loading rate for DNR) (Long et al., 2016). It was found that this magnetic vesicle drug-carrying system exhibited good stability and long-term sustained drug release, and significantly reduced the nonspecific toxicity of the drug. Meanwhile, as magnetic nanoparticles with good magnetic responsiveness, magnetosomes are able to target tumors under the action of the external magnetic field. The targeting ability can be further enhanced by combining specific targeting molecules with chemical groups on the membrane of the magnetosome (Zhang et al., 2018a). Moreover, according to the properties of magnetosomes, it is also possible to combine the delivered drugs with various therapeutic regimens, including chemodynamic therapy (CDT) (Ye et al., 2022), magnetically induced thermotherapy (Liu et al., 2012), magnetically induced photothermal therapy (PTT)and magnetically induced immunotherapy (Zhang F. et al., 2019). For example, the researchers extracted magnetosomes from the Magnetospirillum magneticum AMB-1 and used them as a “magnetosome chassis” (MSC) to generate gold nanoparticles in situ. The resulting hybrid (MSC-Gold) is capable of targeting tumors in a tumor-centric magnetic field and enables combined chemodynamic and photothermal therapies (Figure 3) (Ye et al., 2022).
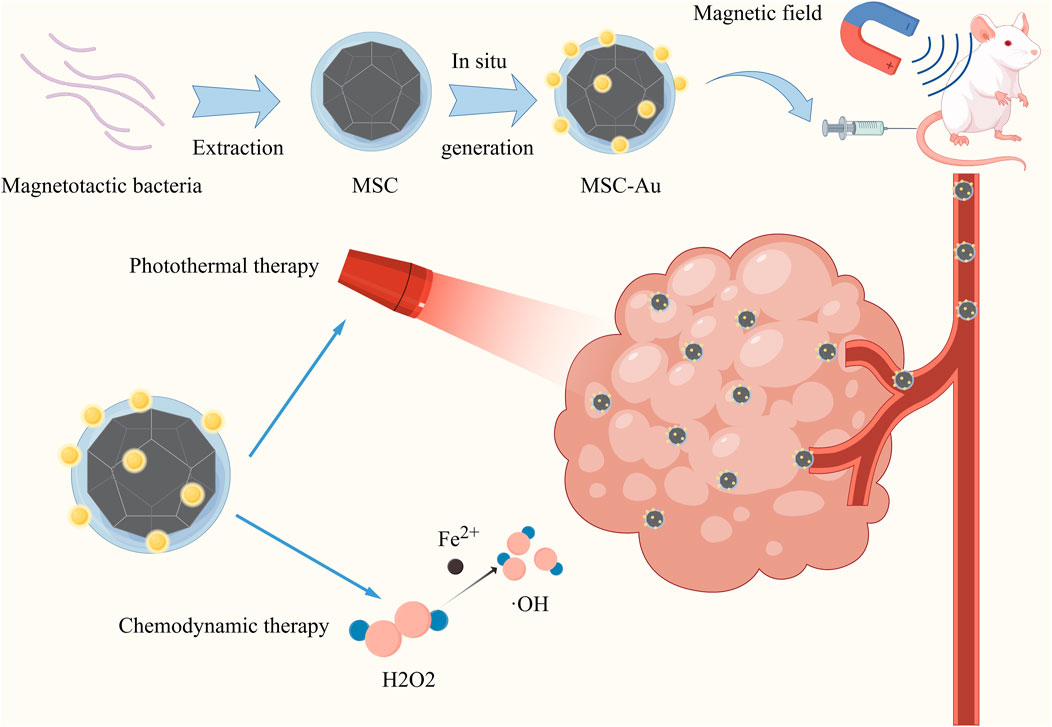
Figure 3. Graph illustration of the MSC was extracted from the Magnetospirillum magneticum AMB-1 bacterium. Subsequently, AuNPs could be grown in situ on this MSC. The resulting hybrid (MSC-Au) was able to target tumors in the presence of a tumor-focused magnetic field, enabling multimodal combination therapy. Reproduced with permission from (Ye et al., 2022) Reference, copyright Wiley, 2022.
Spores are formed in extreme environments and have functional components that can be used in cancer therapy. Clostridium spp. as a spore-producing bacterium can survive in hypoxic environments, and the spores it produces can germinate in hypoxic and necrotic areas of tumors and release carrier substances to shrink tumors (Mengesha et al., 2006; Mengesha et al., 2007). Genetically modified C. novyi-NT spores lack lethal toxins and have anticancer activity along with improved safety. Intratumor injection of Clostridium histolyticum spores and intravenous injection of Clostridium perfringens spores lead to lysis of tumor tissues without adverse effects on normal tissue (Kubiak and Minton, 2015). In addition, bacterial spores serve as carriers for anticancer drugs, therapeutic proteins, and cytotoxic peptides.
Minicells are the products of abnormal bacterial cell division, have a nanometer size, and are controlled by mutated minCDE genes. The capability of minicells to package multiple drugs by unidirectional diffusion, independent of their physicochemical properties, makes them ideally suited for drug delivery (MacDiarmid et al., 2009). In addition, minicells carry all the ingredients of the parental cells except chromosomal DNA and therefore cannot proliferate,which provides great safety for their use as carriers of drug delivery for the treatment of tumors (Di Ventura and Sourjik, 2011). For example, in animal studies, combining DOX with tumor-targeting minicells resulted in significant regression of in situ breast tumors, and no serious toxic side effects were observed.
In addition, minicells are easy to modify, and researchers can enhance their targeting and killing of tumors by modifying minicells with ligands or antibodies. For example, MacDiarmid et al. modified minicells using antibodies that enabled the minicells to target epidermal growth factor receptors (EGFR) or human epidermal growth factor receptor 2 (HER2) receptors that are specifically overexpressed on tumor cell membranes. These modified minicells carry drugs directly into target tumor cells after packaging DOX and paclitaxel (PTX) therapeutic drugs. In addition, the researchers modified the DOX-loaded minicells so that they could target drug delivery to solid tumors (Figure 4) (Zhang et al., 2018b). Minicell-based forms of drug delivery are more effective than free drugs and can effectively reduce the toxic side effects of drugs on normal tissues (MacDiarmid et al., 2007). Currently, EGFR-modified paclitaxel-loaded S. typhimurium minicells have been successfully tested in phase I trials, and no patients have died during the observation period due to adverse effects (Solomon et al., 2015).
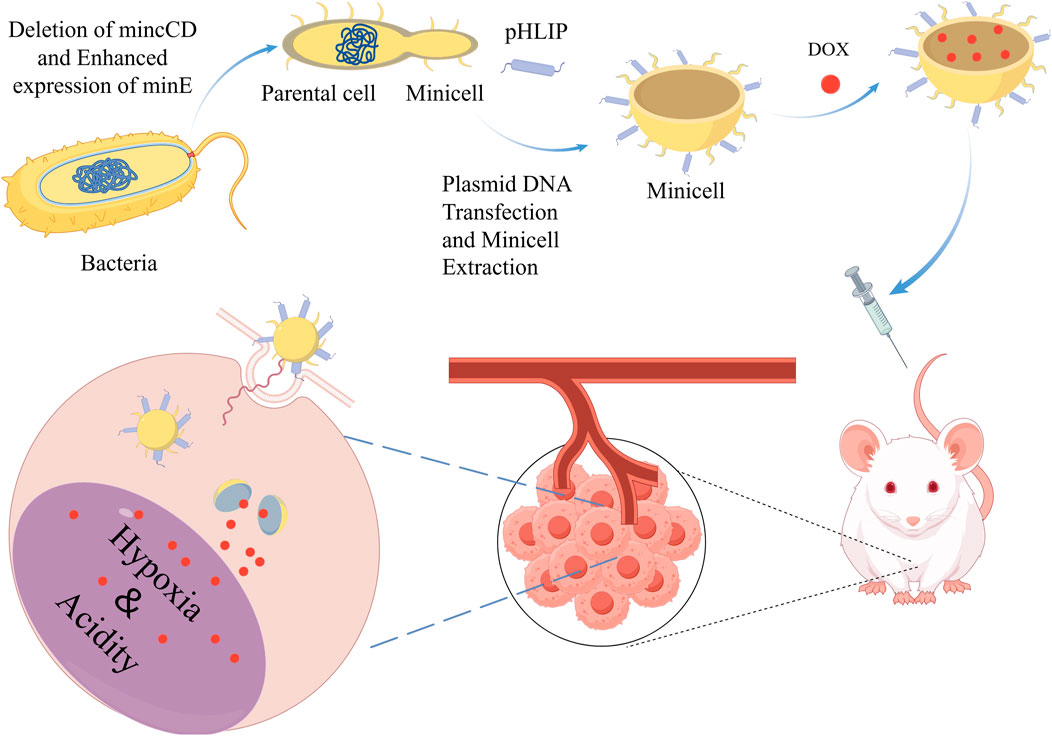
Figure 4. Decorative loading of pH (low) insertion peptide (pHLIP) on the surface of DOX-loaded minicells enables them to target acidic and hypoxic TME and release DOX, thereby killing tumor cells. Adapted from reference (Zhang et al., 2018b) PMC,Copyright 2018.
To deliver drugs, minicells have an advantage in delivering RNAi and can be efficiently loaded with siRNA and shRNA (Jivrajani and Nivsarkar, 2019). Based on this feature, MacDiarmid et al. utilized microcells to deliver siRNA/shRNA for the treatment of cancer, and the loaded siRNA/shRNA induced apoptosis in tumor cells (Jivrajani and Nivsarkar, 2016). And because their cell walls contain varying levels of LPS, they are not only expected to be a drug delivery system (Giacalone et al., 2007) but also to act as immune adjuvants for antitumor effects (Lundin and Checkoway, 2009).
Bacteria secrete membrane vesicles called bacterial extracellular vesicles (bEVs), spherical structures with a lipid bilayer containing various biomolecules from the parent bacterium. bEVs derived from the outer membrane of Gram-negative bacteria are known as outer membrane vesicles (OMVs) (Jagannadham and Chattopadhyay, 2015). The OMVs carry periplasmic and cytoplasmic components, a large number of microbe-associated molecular patterns (MAMPs) (Ellis and Kuehn, 2010), and functional components. bEVs derived from the cytoplasmic membrane of Gram-positive bacteria are known as cytoplasmic membrane vesicles (CMVs), which contain material from the cytoplasm but do not contain components that can cause an acute toxic response (e.g., LPS and other cell wall components, etc.) (Silhavy et al., 2010).
bEVs have many characteristics such as good biocompatibility and easy genetic modification, so they can be used as delivery carriers for anticancer agents. The bEVs-mediated targeted delivery system of chemotherapeutic agents is a perspective method in oncology therapy to improve effects and reduce adverse effects caused by systemic therapy. Currently, bEVs have been successfully loaded with different kinds of chemotherapeutic drugs, such as PTX, DOX, gemcitabine, and tegafur (Figure 5) (Chen Q. et al., 2019; MacDiarmid et al., 2016; Sagnella et al., 2020; Sagnella et al., 2018; Alfaleh et al., 2017).
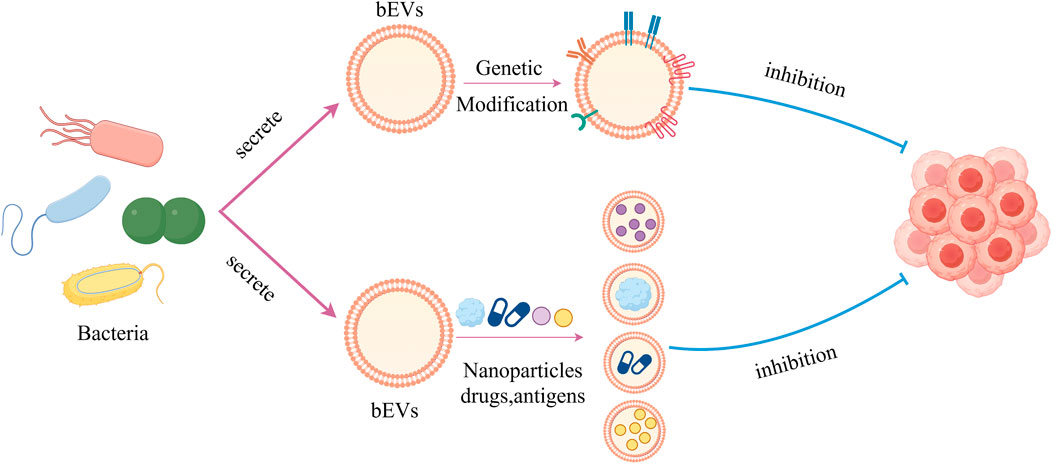
Figure 5. Graph showing bEVs used as anticancer drug delivery carriers as well as genetically modified for cancer therapy.
In addition, bEVs can carry antitumor substances such as antigens, TLR agonists, and photosensitizers (Qing et al., 2020; Chen Q. et al., 2020; Wang et al., 2020). Since bEVs inherit various immunostimulatory molecules from parental bacteria, they can generate immune responses against pathogens, which gives them the potential for immunotherapy of cancer (Nagakubo et al., 2020).
Combining the capacity of bEVs to deliver drugs and immune adjuvants could further enhance their anti-tumor therapeutic potential. This holds promise for complete tumor eradication and prevention of tumor recurrence and metastasis. Examples include loading bEVs with chemotherapeutic agents (Kuerban et al., 2020) or adding photothermal agents to bEVs. Chemotherapeutic agents or PTT can lead to immune cell death in tumor cells, which, in combination with the immune properties of bEVs, can enhance the efficacy of anti-tumor therapy (Wang et al., 2020).
In conclusion, using bacterial components as delivery carriers for anticancer therapy is an innovative and evolving study (Table 2).
4 Bacteria combined with nanomaterials to treat cancer
Currently, drug delivery systems mediated by bacteria and their derivatives are still in the preclinical stage in the field of cancer therapy (Kavan et al., 2023; Tsujikawa et al., 2020; Nelson et al., 2023; Gerstner et al., 2022; Brahmer et al., 2021; Ramalingam et al., 2020). Research has shown that combining bacterial therapy with other treatment options can improve the efficacy and specificity of cancer treatments.
Nowadays, nanomaterials show great potential in cancer treatment and make cancer treatments more diversified. Nanomaterials have many advantages, first of all, the size of nanomaterials can be controlled (Liu et al., 2019), and can be passively or actively targeted to tumors (Zhu et al., 2022). Secondly, the large surface area/volume ratio allows it to be utilized as a drug carrier to deliver drugs (Lv et al., 2021), genes (Mei et al., 2019), and other therapeutic molecules (Wang Y. et al., 2021), and protect them from enzymatic degradation in complex physiological microenvironments. Third, since nanomaterials have a variety of functional groups on their surfaces, it is easy to modify them to enhance their properties (Hu et al., 2021). Fourth, functionalized nanomaterials can control drug release in vivo through various internal and external stimuli and can induce conductive photothermal or photodynamic processes and act as immunomodulators (Shen et al., 2022). Although nanoparticles have many strengths in anti-tumor therapy, they also have some weaknesses. Bacteria, on the other hand, can compensate for the shortcomings of nanotechnology and generate multiple synergistic therapeutic modalities with it. The combination of bacteriotherapy and nanomedicine technologies can be classified as follows 1) Bacteria–nanoparticle biohybrid systems, 2) Intracellular Bacteria Nanoengineering, and 3) Nanoparticle-Based Bionic Bacteria.
4.1 Bacteria-nanoparticle biohybrid systems
Bacteria-nanoparticle biohybrid systems have good biocompatibility degradability, and high drug loading. In this part, we will conclude the use of bacterial-nanoparticle biohybrid systems in combination with different therapeutic regimens (Table 3).
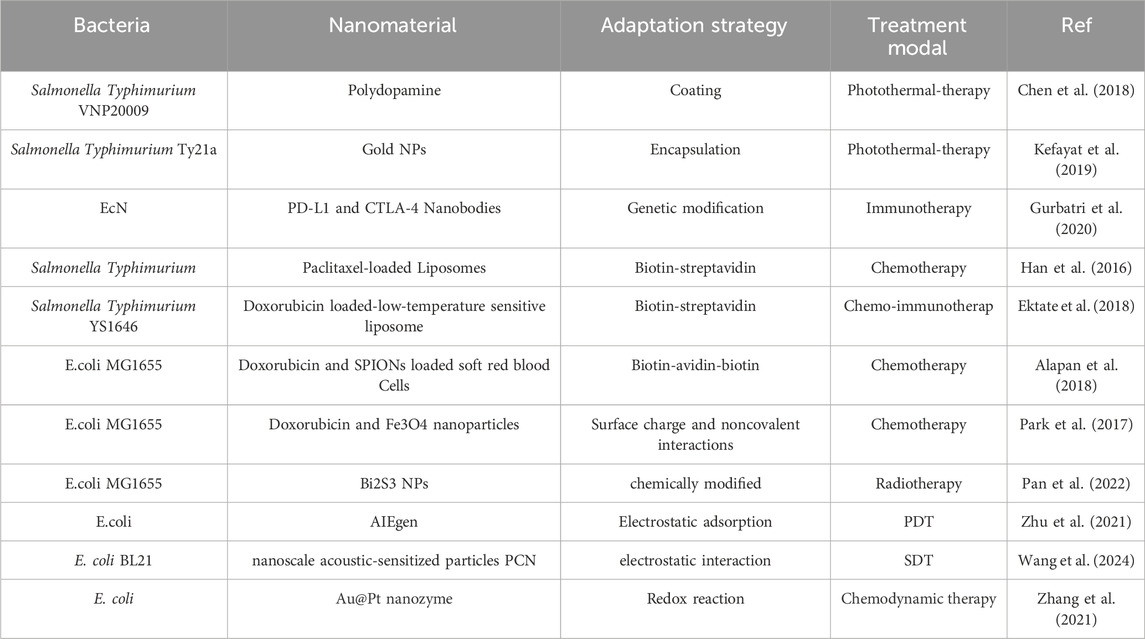
Table 3. Application of Bacteria-nanoparticle biohybrid systems of anticancer drugs in cancer treatment.
4.1.1 Chemotherapy
Chemotherapy has been widely used in the past decades to treat various types and stages of tumors (Ouyang et al., 2020). However, standard chemotherapeutic agents have limited therapeutic efficacy owing to their lack of ability to target tumors. Besides, insufficient targeting can produce serious side effects and may lead to long-term respiratory, urinary, circulatory, neurological, and reproductive adverse effects (Zhang et al., 2022; Bhoyrul et al., 2021; Su et al., 2023). Bacteria-nanoparticle biohybrid systems with good targeting can greatly minimize systemic toxicity and improve the efficacy of chemotherapy. Salmonella typhimurium is one of the most researched anticancer bacterial populations, and some researchers have used biotin-streptavidin chemistry to combine S. typhimurium with liposomes containing paclitaxel. The study demonstrated that binding bacteria can exhibit better anti-tumor capabilities compared to the liposome-encapsulated drug alone (Han et al., 2016). Another of the most researched anticancer strains is E. coli Nissle 1917 (EcN), a probiotic with a high safety profile. For example, Xie et al. bound adriamycin to EcN via an acid-unstable cis-conjugated anhydride linker for tumor targeting and drug-responsive release (Xie et al., 2017).
However, the synergy between biological therapy and chemotherapy goes far beyond this. Thermobots synthesized by attaching adriamycin-containing cryo-sensitive liposomes to the surface of attenuated Salmonella can be used for immunotherapy of colon cancer (Ektate et al., 2018). After reaching the tumor site, the thermal robot uses high-intensity focused ultrasound at 40–42° to release adriamycin from the liposomes and stimulate polarized macrophages to change to the M1 phenotype. Additionally, the delivery of CaO2 NPs and doxorubicin (DOX) to tumor tissue using Bifidobacterium infantis (Bif) for synergistic chemotherapy and CDT (Figure 6) (Li et al., 2023). Bacteria can be modified not only by binding to drug-carrying nanomaterials but also by combining with natural materials to enhance their intrinsic properties. For instance, For example, Alapan et al. attached soft erythrocytes loaded with DOX and superparamagnetic iron oxide nanoparticles (SPION) to the surface of engineered E. coli to form microswimmers that could enable on-demand delivery and release of chemotherapeutic drugs (Alapan et al., 2018).
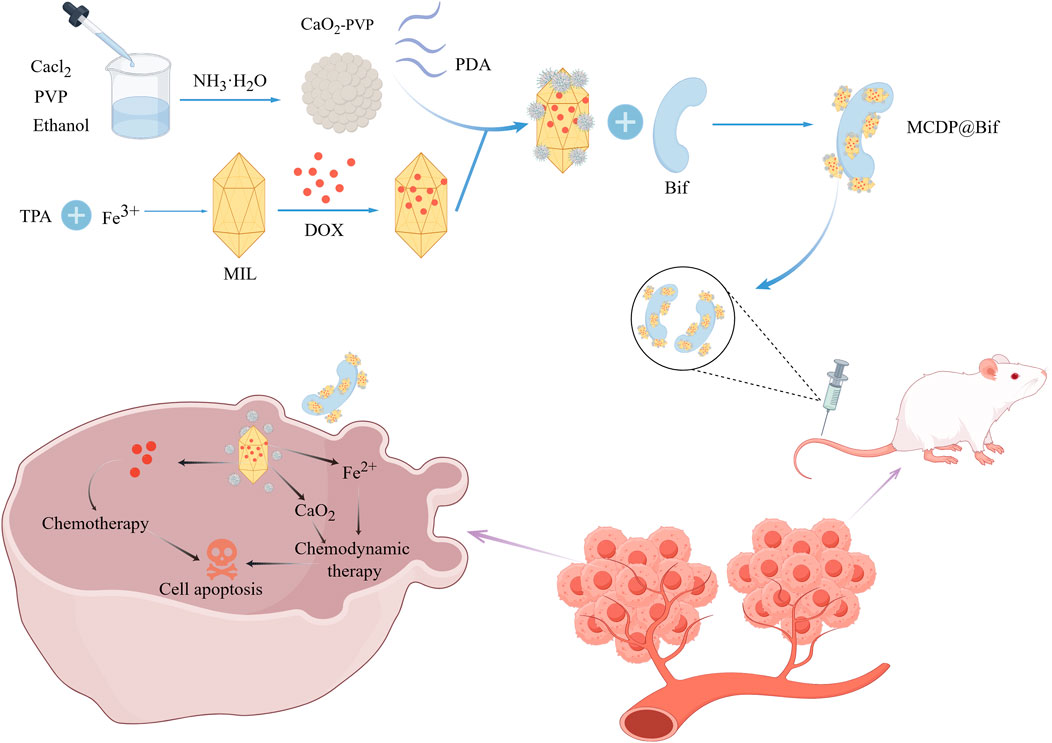
Figure 6. The graph depicts the CaO2 NPs and DOX were loaded onto iron-based MOFs (named as MIL), and the resulting MIL + CaO2 + DOX + polydopamine (PDA) (MCDP) NPs were attached to the surface of Bifidobacterium infantis (Bif) through PDA coating. Achieved CaO2 and iron ion induced chemodynamic therapy combined with DOX chemotherapeutic therapy. Adapted from reference (Li et al., 2023). Wiley, Copyright 2023.
4.1.2 Radiotherapy
Radiotherapy is a therapeutic method that uses high-energy rays to locally irradiate tumor cells, which has a history of more than a hundred years and is an important and effective means of antitumor therapy (Kasat et al., 2010). Radiotherapy using ionizing radiation can induce the production of reactive oxygen species (ROS) causing DNA double-strand breaks in tumor cells, thereby killing the tumor (Ding et al., 2022). The oxygen content of tissues in this process plays a decisive role in the treatment (Alapan et al., 2018). Many experimental studies have shown that hypoxic cells are more resistant to ionizing radiation than normoxic cells, and therefore hypoxic and necrotic areas of tumors often lead to poor therapeutic outcomes or even treatment failure (Barker et al., 2015). Bacteria can deliver radiotherapeutic agents. For example, loading the radioisotope 188-rhenium (Figure 7) and the radiotherapeutic drug 32-phosphorus with attenuated live Listeria monocytogenes induces ROS production and kills tumor cells (Quispe-Tintaya et al., 2013; Chandra et al., 2017).
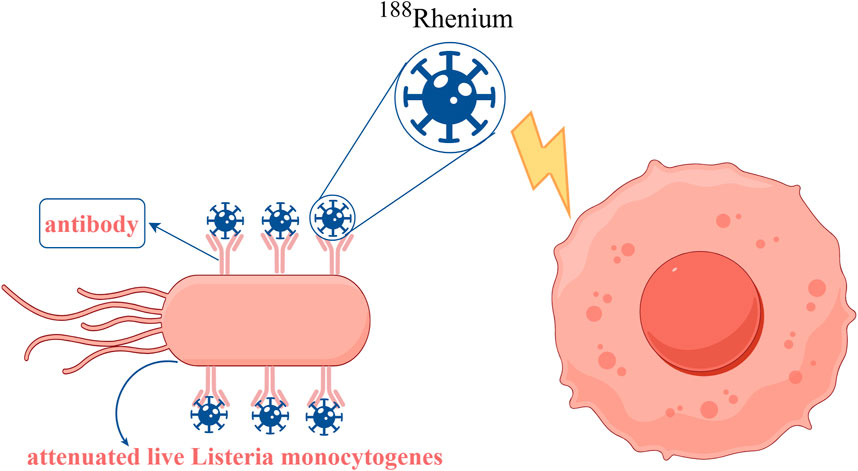
Figure 7. Decoration of the attenuated live Listeria monocytogenes conjugated with antibodies and then combined with the radioisotope 188Rhenium to produce radioactive Listeria monocytogenes (RL), which is effective in killing tumor cells. Adapted from reference (Quispe-Tintaya et al., 2013).
Cytolysin A (ClyA) overexpressed E. coli MG1655 was combined with bismuth trisulfide nanoparticles (BNPs). It has excellent tumor-targeting and penetration properties, which can rapidly accumulate in the lesion and achieve a deep penetration effect. It can suppress tumor proliferation through the secretion of ClyA, while the BNPs act as nano-radiological sensitizers capable of triggering a local ROS burst and DNA damage in tumor cells (Pan et al., 2022).
4.1.3 Photothermal therapy
PTT is a novel tumor treatment strategy that converts light energy into heat to kill cancer cells (Xiong et al., 2023; Li et al., 2022). A variety of nanomaterials with photothermal conversion capabilities have been developed, including metallic nanomaterials, carbon-based nanomaterials, and polymer-based nanomaterials (Liu S. et al., 2020).
However, existing PTT monotherapies are not effective (Wang L. et al., 2021). To improve the effectiveness of conventional PTT Luo et al. utilized the anaerobic bacteria Bifidobacterium shortum and Clostridium difficile in combination with gold nanorods (AuNRs) to enhance the effect of PTT through the synergistic effect between the high photothermal conversion efficiency of the gold nanorods and the anaerobic bacteria’s tumor hypoxia-targeting ability (Luo et al., 2016). Additionally, PTT can cause immunogenic death of tumor cells and enhance anti-tumor immunotherapy (Shang et al., 2020). In this way, Ag2S QDs were coupled to the surface of B. bifidum (Zhao et al., 2022). Engineered bacteria can promote the secretion of immune factors and activate T-cells for immunotherapy. Ag2S QDs have a high efficiency of photothermal conversion, which can induce the production of tumor-specific antigens by ICDs, and further enhance the effect of immunotherapy.
4.1.4 Immunotherapy
Tumor immunotherapy is an innovative treatment method that uses the body’s own immune system to fight cancer. With the continuous advancement of medicine, it has become the fourth most popular cancer treatment after surgery, chemotherapy, and radiotherapy. The discovery by researchers in the 19th century that coli toxins can trigger the activity of the immune system against tumors set the precedent for bacterial immunotherapy (Karbach et al., 2012).
In recent years, significant progress has been made in bacterial immunotherapy, and some of these therapies have successfully entered clinical development. For example, attenuated bacteria are programmed to express and deliver various tumor suppressor cytokines to enhance anti-tumor immunity and induce apoptosis in tumor cells (Duong et al., 2019). Other immunotherapeutic agents, such as cytotoxic proteins (He et al., 2019), antigens, and antibodies (Nishikawa et al., 2006), have also been overexpressed in attenuated engineered bacteria and used for tumor therapy.
Bacteria have immunomodulatory effects and have great potential in cancer immunotherapy. Wang et al. further enhanced the immunotherapeutic effect of Salmonella by combining cationic polymer nanoparticles coated on its surface. The complexes could adsorb tumor antigens and transfer them to the periphery of the tumor. The crosstalk between antigens and dendritic cells increased significantly when the antigens were transferred to the periphery of the tumor. Chemotherapy, radiotherapy, and photothermal effects can enhance cancer immunotherapy by inducing immunogenic death and releasing cancer antigens (Huang et al., 2021; Fu et al., 2022; Liu et al., 2023), and the combination of bacterial immunotherapy and the aforementioned therapeutic regimens has become a hot topic of current research.
4.1.5 Other
Although tumor cells can tolerate higher concentrations of ROS, it can also be devastating to cancer cells when the amount of ROS exceeds the cellular tolerance threshold (Yang B. et al., 2020). Based on these mechanisms, a range of ROS-based therapies have been developed, such as radiation therapy, Photodynamic therapy (PDT), sonodynamic therapy (SDT), and CDT. Combining the above treatment options with bacterial therapies may also produce even better cancer outcomes (Figure 8).
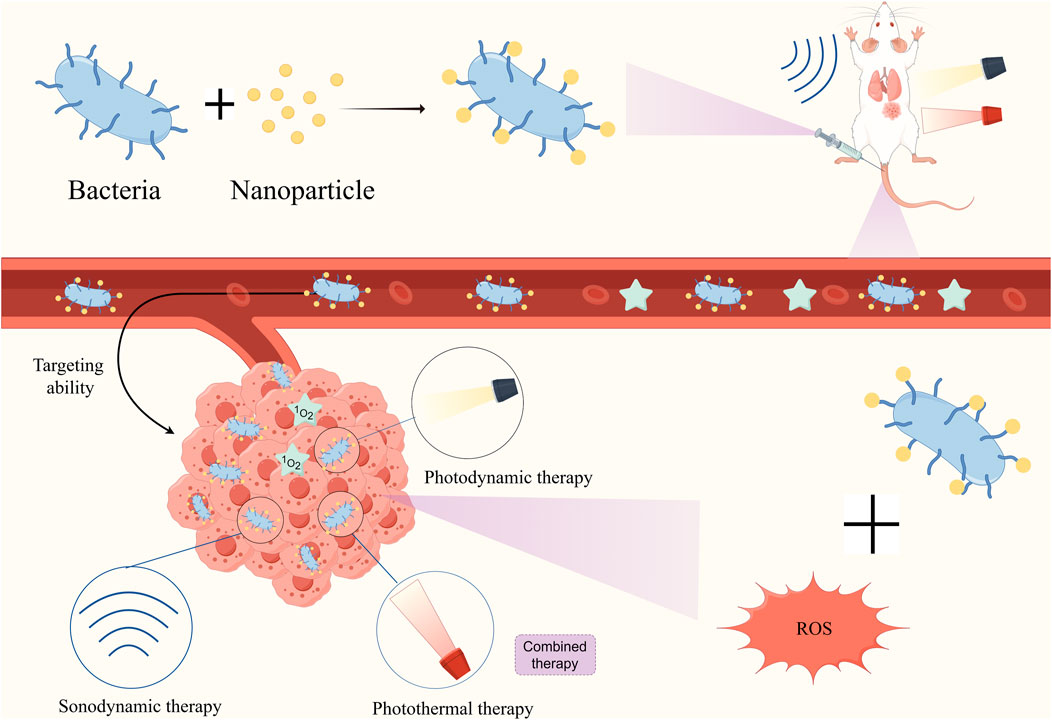
Figure 8. Diagrammatic depiction of combining bacteria with nanomaterials to make ROS-based cancer therapies (PDT CDT SDT) more effective using bacterial targeting properties.
PDT is a treatment that utilizes photosensitizers and specific light sources to selectively kill cancer cells (Karges et al., 2019; Karges, 2022). The photosensitizer or material absorbs light energy and converts it into chemical energy, generating reactive oxygen species capable of killing tumor cells without affecting normal cells (Liu et al., 2015). The use of bacteria to construct an oxygen self-supply system can strengthen the therapeutic efficacy of PDT. For example, by coupling Chlorella with chlorine-6 nanoparticles (Wang H. et al., 2022), Chlorella can produce O2 under 660 nm light irradiation to enhance PDT, and then the enhanced PDT can lyse Chlorella to release adjuvants on the bacterial surface and activate biotherapeutic combined immunotherapy. Type I photosensitizers can produce ROS in the absence of O2, which solves the problem of photosensitization that occurs with conventional type II photosensitizers in oxygen-depleted tumors. This solves the problem of decreased efficiency of photosensitization in oxygen-depleted tumors with conventional type II photosensitizers. Combining type I PDTs with bacteria is of great therapeutic importance for the treatment of tumors (Zhu et al., 2021). Zhu et al. developed a novel bacterial-based AIEgen (TBP-2) nanohybrid system (AE), and the AIEgen nano bio-hybrids significantly inhibited the growth of in situ colon tumors by facilitating the generation of ROS in the hypoxic tumor region under light irradiation.
SDT is an up-and-coming non-invasive tumor treatment that effectively kills tumor cells by activating acoustic sensitizers through ultrasound to produce ROS at the tumor site (Li et al., 2021; Gong and Dai, 2021; Son et al., 2020). It is an effective cancer treatment because of its good tissue penetration, non-invasiveness, and low toxicity (Li et al., 2020). The therapeutic efficacy of SDT is closely dependent on the concentration of oxygen in the tumor, as most acoustic sensitizers require oxygen as a feedstock to generate ROS such as single-linear oxygen under ultrasonic excitation. Plasmid transfection of E. coli BL21 was performed to overexpress catalase, followed by nanoscale acoustic-sensitized particles PCN synthesized from acoustic-sensitizing molecules tetrakis (4-carboxyphenyl) porphyrin (TCPP) and zirconium clusters (Zr6) NPs was adsorbed onto the bacterial surface by electrostatic interaction to obtain the multifunctional biohybrid E.coli-pE@PCN. After intravenous injection, E.coli-pE@PCN showed good tumor targeting and penetration ability, which not only could continuously express catalase to alleviate the tumor hypoxia but also promoted the enrichment and expanded distribution of carried acoustic sensitizers in the tumor site, thus triggering an effective SDT (Wang et al., 2024).
CDT is a promising therapeutic modality that utilizes endogenous overexpression of H2O2 in tumors to generate toxic hydroxyl radicals (·OH) via the Fenton/Fenton-like reaction (Wang Z. et al., 2022; Zhu et al., 2023). Due to the high H2O2 properties of TME, CDT therapy can autonomously generate ROS in TME. Nevertheless, the efficacy of CDT is often restricted by tumor antioxidant capacity (Zhou et al., 2021). By modifying Au@Pt nanozymes (Bac-Au@Pt) on the bacterial surface, the nano-system can effectively release ROS into tumor cells due to the targeting ability of the bacteria and the catalytic properties of Au@Pt nanozymes under the acidic environment. In addition, the complex reduces the antioxidative capacity of the tumor thus further increasing the CDT effect (Zhang et al., 2021).
4.2 Intracellular bacteria nanoengineering
Nanoparticles can be obtained by synthesizing them by various chemical methods, but chemical synthesis methods have disadvantages such as high toxicity, low purity, and poor biocompatibility. Synthesizing various nanoparticles by using bacteria as factories or base materials can solve the above problems, and thus has attracted attention. For example, metal nanoparticles can be synthesized naturally in bacteria or through genetic engineering, and most of the bacteria-mediated synthesis of metal nanoparticles has been shown to have antitumor activity in vitro (Kang et al., 2008; Park et al., 2010).
Almalki and Khalifa, (2020) synthesized silver nanoparticles (AgNPs) with diameters between 15 and 40 nm from Bacillus sphaericus KFU36. They found that the synthesized AgNPs could enter the TME and aggregate to induce significant tumor cell apoptosis. AgNPs synthesized by Yang et al. promoted intracellular ROS production in tumor cells, which increased lipid peroxidation and led to significant tumor shrinkage (Yang et al., 2020b). Vairavel uses Enterococcus faecalis to synthesize gold nanoparticles intracellularly, which inhibits tumor cell proliferation by generating ROS in tumor cells (Vairavel et al., 2020). Rajkumar et al. synthesized selenium nanoparticles (SeNPs) using Pseudomonas Schizosaccharomyces, which significantly inhibited tumor angiogenesis and killed tumor cells at low concentrations (Rajkumar et al., 2020).
4.3 Nanoparticle-based bionic bacteria
Biomimetic nanoparticles (NPs) formed using cell membranes encapsulating NPs are a promising biomedical material. Among various types of cell membranes, OMV shows great potential in the biomedical field (Qing et al., 2019). Coating NPs with bacterial OMVs retains the intrinsic properties of synthetic NPs and also enhances the properties possessed by the OMVs (Figure 9). For example, fusion of drug-carrying liposomes with OMVs can further enhance the drug-carrying capacity (Piffoux et al., 2018). Chen Q. et al. (2019) used bacterial OMVs to encapsulate drug-loaded polymeric micelles. The OMVs activated the host immune response and combined with the chemotherapeutic drugs contained in the polymeric micelles, effectively inhibiting tumor growth. In a report by Wang et al. (2020), OMVs and B16-F10 cancer cell membranes were successfully coated on hollow polydopamine (HPDA) NPs. This material could combine immunotherapy and PTT to treat melanoma. Researchers genetically modified a bacterial strain by expressing a tyrosinase transgene and generated bacterial microvesicles loaded with the biopolymer melanin. These bioengineered bacterial microvesicles were used as bio-nano-heaters for not only in vitro and in vivo tumor imaging but also tumor growth inhibition under near-infrared light irradiation (Gujrati et al., 2019).
Chen et al. formed a hybrid cell membrane by fusing a tumor cell membrane with a bacterial cytoplasmic membrane. By encapsulating polylactic acid-glycolic acid copolymer (PLGA) nanoparticles within the hybridized membrane, antigens and adjuvants from both cell membrane sources could be delivered simultaneously, thereby enhancing the innate immune response. Improved anti-tumor effect while avoiding side effects (Chen et al., 2021). In addition, biofilm components extracted from bacteria can also be used as nanoparticles for drug delivery. Yi et al. assembled rhamnolipids extracted from Pseudomonas aeruginosa with the photosensitizer magnesium chlorophyllate (Pba), and the assembled nanomaterials showed a significant increase in accumulation in tumor tissues and demonstrated significant tumor inhibition (Yi et al., 2019). These encouraging studies suggest that nanoparticle-based bionic bacteria hold promise for cancer therapy.
5 Strategies for combining nanomaterials and bacterial carriers
The binding of nanomaterials to bacteria is a prerequisite for synergistic therapy. Here, we summarize the different ways in which bacteria and their components can be combined with nanomaterials and the resulting biological functions.
5.1 Bioconjugation
Biological strategies allow for the fabrication of nanohybrids via biorecognition processes (e.g., antigen/antibody and biotin/streptavidin) and metabolic pathways (Mostaghaci et al., 2017). Among them, streptavidin and biotin are the most popular binding pairs used in biotechnology applications, which are highly selective and have strong interactions to withstand extreme temperatures (Tm of 112°C), pH, denaturants, and enzymes (Dundas et al., 2013). Suh et al. used this method to attach streptavidin-labeled drug-carrying poly (lactic-ethanolic acid) nanoparticles to Salmonella typhimurium VNP20009, which formed a biotin surface. They demonstrated a 100-fold increase in the ability to target tumors without any external driver (Suh et al., 2019).
To attach nanoparticles to bacteria through streptavidin-biotin interactions, the utilization of genetically engineered bacteria is an alternative method of bioconjugation. For example, by increasing the binding affinity and introducing new binding sites, bacteria can be preferentially adsorbed to specific nanoparticles (Vizsnyiczai et al., 2020). By designing genetic circuits, it is possible to control the binding of bacteria to nanomaterials and maintain bacterial viability and proliferation. Bioconjugate gene modification with high loading capacity can solve the problem of mutual repulsion between negatively charged nanoparticles and negatively charged bacteria, making it a potential platform for a wide range of applications (Yang et al., 2020c).
5.2 Physical
Physical strategies involve the use of bacteria as carriers to load or anchor materials or drugs to the outer surface of the bacteria, including electroporation (Ding et al., 2021), electrostatic interactions (Xie et al., 2021), impregnation (Kuang et al., 2023), or membrane coating (Wu et al., 2019).
Electroporation techniques can facilitate the entry of therapeutic drugs into bacteria by applying an enhanced electric field to increase the permeability of the cell membrane. Electrostatic interactions, dip-coating processes, and cell membrane coating techniques can also be used to construct nano-biological mixtures. by anchoring nanomaterials to the outer surface of bacteria. Of these, electrostatic interactions are relatively flexible in the construction of nanohybrid materials, and using this method bacteria can be used with virtually any charged nanoparticle, polyelectrolyte, protein, or polysaccharide.
Although physical strategies have the advantages of simplicity and rapidity in constructing nanohybrid materials, they also have some drawbacks. For example, electroporation affects bacterial activity, while electrostatic interactions are less stable in vivo applications due to the prevailing competitive reactions (Zoaby et al., 2017).
5.3 Chemistry
Chemical groups on the surface of bacteria make it possible to bind bacteria and nanoparticles, which can provide a more stable bond between bacteria and nanomaterials through chemical bonding. To date, many chemical reactions have been employed to bind nanomaterials to bacterial surfaces, including carbodiimide chemistry (Taherkhani et al., 2014), Michael addition reactions (Xing et al., 2021), intermolecular disulfide bond cross-linking (Liu L. et al., 2020), host-guest chemistry (Chen QW. et al., 2020), Schiff base reactions (Chen QW. et al., 2020), and click chemistry (Xie et al., 2018). Of these, carbodiimide chemistry has received much attention due to the enrichment of bacterial surfaces with amino or carboxyl groups.
5.4 Biomineralization
Biomineralization, the process by which organisms utilize mineral elements, is a promising approach to materials engineering. Unlike the method of combining nanoparticles with bacterial surfaces, this approach can assist bacteria form nanoparticles directly. For example, Chen et al. developed a self-mineralizing photothermal bacterium by biomineralizing palladium nanoparticles (PdNPs) on the surface of the parthenogenetic anaerobic bacterium Shewanella oneidensis MR-1 (188). Biomineralization consists of two main methods: biologically induced and biologically controlled. Mineralization during biologically induced processes usually occurs on the bacterial surface and is not directly regulated by genes. In turn, biologically controlled mineralization processes, such as nucleation, growth, and localization, can be regulated by genes and occur both intracellularly and extracellularly. Magnetic nanoparticles (magnetosomes) produced by MTB are a prime example of biologically controlled mineralization (Liu et al., 2024).
5.5 Bionic nanoparticles encapsulated by bacterial outer membrane vesicles
Currently, there are three main approaches to preparing bacterial OMV-coated nanomaterials. The first method, physical co-extrusion involves the repeated extrusion of a mixture of bacterial outer vesicles and nanoparticles through a thin porous membrane of nanoscale porous polycarbonate using an Avanti micro-extruder (Wu et al., 2020). Although this process is very efficient for the production of OMV-NPs, the deposition of raw materials on filters causes losses and makes it impractical for wide-scale production.
The second method, the ultrasonic fusion method, in which ultrasonic energy is used to promote the encapsulation of nanomaterials by extracellular membrane vesicles, seems to have solved the production scale problem (Zhang Y. et al., 2019). However, encapsulation inhomogeneity and dimensional variations are the shortcomings of this technique.
The third method is microfluidic electroporation (Rao et al., 2017). technique that involves mixing cell membranes with nanoparticles and then facilitating the entry of the nanoparticles into the cell membranes through microfluidic electroporation. It has the advantages of high accuracy and scalability, uniform product size, reproducibility, and stability.
6 Challenges and future directions
Novel therapeutic agents for the treatment of cancer are emerging, and therefore, drug delivery platforms need to evolve to further improve therapeutic efficacy and address the new challenges. Current findings suggest that bacteria and their derivative-mediated drug delivery systems may become a major asset in the fight against cancer. With the natural properties of bacterial carriers, such as tumor targeting, ease of genetic modification, and activation of immune responses, researchers can develop new strategies to further optimize bacterial carriers and enhance their anti-cancer efficacy. One innovative and effective strategy is to combine nanomaterials with bacterial carriers to form a hybrid system. The nanomaterials can enhance the therapeutic effect of the bacterial carriers, while the bacterial carriers can enhance the therapeutic effect of the nanomaterials, thus maximizing the synergistic therapeutic effect. The hybrid system has been shown to perform multimodal synergistic therapies and has shown encouraging results in improving cancer treatment outcomes.
However, research on bacterial carriers is still in the preclinical stage, and much effort is still needed to address some scientific issues before the translation to the clinic can be truly accomplished. Some of the shortcomings that need to be overcome and future research directions mainly include,
(i) The mechanism of interaction between bacteria and their hosts is complex and may lead to unforeseen consequences. Research on relevant mechanisms of action should be improved, and bacterial toxicity reduction programs should be continuously improved without affecting the anti-cancer properties of bacteria.
(ii) It is difficult to quantify the dosage of bacterial therapy. If certain strains of bacteria are out of the normal range, it may disrupt the balance of the bacterial flora, leading to dysbiosis and diseases. Improvement of in vivo monitoring strategies to monitor the distribution of bacteria in the body as a means of assessing their safety in the phase.
(iii) Currently, a single bacterium is mostly used for cancer treatment, and synergistic treatment by forming hybrids of multiple bacteria could be considered.
(iv) Using patient-specific bacterial derivatives, personalized medical protocols can be adapted to provide more targeted and effective treatments for cancer patients.
(v) The route of administration is also a major limitation of bacterial therapies. Systemic injection of bacteria can lead to serious complications, and the acidic environment of the stomach during oral administration limits the accumulation of bacteria in the target area. Enhanced research on routes of administration could improve efficacy and reduce the likelihood of adverse effects
(vi) Establishing reliable methods for the mass production of bacteria-nanomaterials hybrids is of great importance and key to reducing the cost and improving the accessibility of therapies.
Although the main focus of this review is on the use of bacteria for cancer treatment, the use of bacteria as in vivo diagnostics is another promising approach with the rapid advances in nanomedicine and synthetic biology. Overall, bacteria-based therapies could provide a more powerful and targeted treatment for cancer. It is believed that with the concerted efforts of a multidisciplinary team of clinicians and scientific researchers, bacterial carrier drug delivery systems are expected to become another powerful weapon in the clinic in the near future.
Author contributions
SY: Writing–original draft, Writing–review and editing. YG: Writing–original draft, Writing–review and editing. HX: Writing–original draft, Writing–review and editing. HP: Writing–original draft, Writing–review and editing.
Funding
The author(s) declare that financial support was received for the research, authorship, and/or publication of this article. This work was supported by the China Postdoctoral Science Foundation (No. 2023M743907) and the Special Fund for Clinical Research of Wu Jieping Medical Foundation (No. 320.6750.2023-17-13).
Acknowledgments
The authors would like to thank all members of Haozhe Piao Lab for them for their support and help. The Figures 5,7,8,9 was drawn by Figdraw.
Conflict of interest
The authors declare that the research was conducted in the absence of any commercial or financial relationships that could be construed as a potential conflict of interest.
Generative AI statement
The author(s) declare that no Generative AI was used in the creation of this manuscript.
Publisher’s note
All claims expressed in this article are solely those of the authors and do not necessarily represent those of their affiliated organizations, or those of the publisher, the editors and the reviewers. Any product that may be evaluated in this article, or claim that may be made by its manufacturer, is not guaranteed or endorsed by the publisher.
References
Alapan, Y., Yasa, O., Schauer, O., Giltinan, J., Tabak, A. F., Sourjik, V., et al. (2018). Soft erythrocyte-based bacterial microswimmers for cargo delivery. Sci. robotics 3 (17), eaar4423. doi:10.1126/scirobotics.aar4423
Alfaleh, M. A., Howard, C. B., Sedliarou, I., Jones, M. L., Gudhka, R., Vanegas, N., et al. (2017). Targeting mesothelin receptors with drug-loaded bacterial nanocells suppresses human mesothelioma tumour growth in mouse xenograft models. PLoS One 12 (10), e0186137. doi:10.1371/journal.pone.0186137
Almalki, M. A., and Khalifa, A. Y. (2020). Silver nanoparticles synthesis from Bacillus sp KFU36 and its anticancer effect in breast cancer MCF-7 cells via induction of apoptotic mechanism. J. Photochem. Photobiol. B Biol. 204, 111786. doi:10.1016/j.jphotobiol.2020.111786
Anderson, J. C., Clarke, E. J., Arkin, A. P., and Voigt, C. A. (2006). Environmentally controlled invasion of cancer cells by engineered bacteria. J. Mol. Biol. 355 (4), 619–627. doi:10.1016/j.jmb.2005.10.076
Barker, H. E., Paget, J. T., Khan, A. A., and Harrington, K. J. (2015). The tumour microenvironment after radiotherapy: mechanisms of resistance and recurrence. Nat. Rev. Cancer 15 (7), 409–425. doi:10.1038/nrc3958
Bhave, M. S., Hassanbhai, A. M., Anand, P., Luo, K. Q., and Teoh, S. H. (2015). Effect of heat-inactivated Clostridium sporogenes and its conditioned media on 3-dimensional colorectal cancer cell models. Sci. Rep. 5 (1), 15681. doi:10.1038/srep15681
Bhoyrul, B., Asfour, L., Lutz, G., Mitchell, L., Jerjen, R., Sinclair, R. D., et al. (2021). Clinicopathologic characteristics and response to treatment of persistent chemotherapy-induced alopecia in breast cancer survivors. JAMA dermatol. 157 (11), 1335–1342. doi:10.1001/jamadermatol.2021.3676
Binder, D. C., Engels, B., Arina, A., Yu, P., Slauch, J. M., Fu, Y.-X., et al. (2013). Antigen-specific bacterial vaccine combined with anti-PD-L1 rescues dysfunctional endogenous T cells to reject long-established cancer. Cancer Immunol. Res. 1 (2), 123–133. doi:10.1158/2326-6066.cir-13-0058
Brahmer, J. R., Johnson, M. L., Cobo, M., Viteri, S., Sarto, J. C., Sukari, A., et al. (2021). JNJ-64041757 (JNJ-757), a live, attenuated, double-deleted Listeria monocytogenes-based immunotherapy in patients with NSCLC: results from two phase 1 studies. JTO Clin. Res. Rep. 2 (2), 100103. doi:10.1016/j.jtocrr.2020.100103
Bray, F., Laversanne, M., Sung, H., Ferlay, J., Siegel, R. L., Soerjomataram, I., et al. (2024). Global cancer statistics 2022: GLOBOCAN estimates of incidence and mortality worldwide for 36 cancers in 185 countries. CA a cancer J. Clin. 74 (3), 229–263. doi:10.3322/caac.21834
Cann, S. H., Van Netten, J., Van Netten, C., and Glover, D. (2002). Spontaneous regression: a hidden treasure buried in time. Med. hypotheses 58 (2), 115–119. doi:10.1054/mehy.2001.1469
Cao, Z., and Liu, J. (2020). Bacteria and bacterial derivatives as drug carriers for cancer therapy. J. Control. Release 326, 396–407. doi:10.1016/j.jconrel.2020.07.009
Chandra, D., Selvanesan, B. C., Yuan, Z., Libutti, S. K., Koba, W., Beck, A., et al. (2017). 32-Phosphorus selectively delivered by listeria to pancreatic cancer demonstrates a strong therapeutic effect. Oncotarget 8 (13), 20729–20740. doi:10.18632/oncotarget.15117
Chen, F., Zang, Z., Chen, Z., Cui, L., Chang, Z., Ma, A., et al. (2019b). Nanophotosensitizer-engineered Salmonella bacteria with hypoxia targeting and photothermal-assisted mutual bioaccumulation for solid tumor therapy. Biomaterials 214, 119226. doi:10.1016/j.biomaterials.2019.119226
Chen, L., Qin, H., Zhao, R., Zhao, X., Lin, L., Chen, Y., et al. (2021). Bacterial cytoplasmic membranes synergistically enhance the antitumor activity of autologous cancer vaccines. Sci. Transl. Med. 13 (601), eabc2816. doi:10.1126/scitranslmed.abc2816
Chen, Q., Bai, H., Wu, W., Huang, G., Li, Y., Wu, M., et al. (2019a). Bioengineering bacterial vesicle-coated polymeric nanomedicine for enhanced cancer immunotherapy and metastasis prevention. Nano Lett. 20 (1), 11–21. doi:10.1021/acs.nanolett.9b02182
Chen, Q., Huang, G., Wu, W., Wang, J., Hu, J., Mao, J., et al. (2020a). A hybrid eukaryotic–prokaryotic nanoplatform with photothermal modality for enhanced antitumor vaccination. Adv. Mater. 32 (16), 1908185. doi:10.1002/adma.201908185
Chen, Q. W., Liu, X. H., Fan, J. X., Peng, S. Y., Wang, J. W., Wang, X. N., et al. (2020b). Self-mineralized photothermal bacteria hybridizing with mitochondria-targeted metal–organic frameworks for augmenting photothermal tumor therapy. Adv. Funct. Mater. 30 (14), 1909806. doi:10.1002/adfm.201909806
Chen, S., Cao, Z., Prettner, K., Kuhn, M., Yang, J., Jiao, L., et al. (2023). Estimates and projections of the global economic cost of 29 cancers in 204 countries and territories from 2020 to 2050. JAMA Oncol. 9 (4), 465–472. doi:10.1001/jamaoncol.2022.7826
Chen, W., Wang, Y., Qin, M., Zhang, X., Zhang, Z., Sun, X., et al. (2018). Bacteria-driven hypoxia targeting for combined biotherapy and photothermal therapy. ACS Nano 12 (6), 5995–6005. doi:10.1021/acsnano.8b02235
Chouaib, S., Noman, M., Kosmatopoulos, K., and Curran, M. (2017). Hypoxic stress: obstacles and opportunities for innovative immunotherapy of cancer. Oncogene 36 (4), 439–445. doi:10.1038/onc.2016.225
Chowdhury, S., Castro, S., Coker, C., Hinchliffe, T. E., Arpaia, N., and Danino, T. (2019). Programmable bacteria induce durable tumor regression and systemic antitumor immunity. Nat. Med. 25 (7), 1057–1063. doi:10.1038/s41591-019-0498-z
Coley, W. B. (1893). The treatment of malignant tumors by repeated inoculations of erysipelas: with a report of ten original cases. 1. Am. J. Med. Sci. (1827-1924) 105 (6), 487–510. doi:10.1097/00000441-189305000-00001
Cubillos-Ruiz, A., Guo, T., Sokolovska, A., Miller, P. F., Collins, J. J., Lu, T. K., et al. (2021). Engineering living therapeutics with synthetic biology. Nat. Rev. Drug Discov. 20 (12), 941–960. doi:10.1038/s41573-021-00285-3
Daassi, D., Mahoney, K. M., and Freeman, G. J. (2020). The importance of exosomal PDL1 in tumour immune evasion. Nat. Rev. Immunol. 20 (4), 209–215. doi:10.1038/s41577-019-0264-y
Dang, L. H., Bettegowda, C., Huso, D. L., Kinzler, K. W., and Vogelstein, B. (2001). Combination bacteriolytic therapy for the treatment of experimental tumors. Proc. Natl. Acad. Sci. 98 (26), 15155–15160. doi:10.1073/pnas.251543698
Ding, S., Liu, Z., Huang, C., Zeng, N., Jiang, W., and Li, Q. (2021). Novel engineered bacterium/black phosphorus quantum dot hybrid system for hypoxic tumor targeting and efficient photodynamic therapy. ACS Appl. Mater. and Interfaces 13 (8), 10564–10573. doi:10.1021/acsami.0c20254
Ding, Z., Guo, Z., Zheng, Y., Wang, Z., Fu, Q., and Liu, Z. (2022). Radiotherapy reduces N-oxides for prodrug activation in tumors. J. Am. Chem. Soc. 144 (21), 9458–9464. doi:10.1021/jacs.2c02521
Di Ventura, B., and Sourjik, V. (2011). Self-organized partitioning of dynamically localized proteins in bacterial cell division. Mol. Syst. Biol. 7 (1), 457. doi:10.1038/msb.2010.111
Dundas, C. M., Demonte, D., and Park, S. (2013). Streptavidin–biotin technology: improvements and innovations in chemical and biological applications. Appl. Microbiol. Biotechnol. 97, 9343–9353. doi:10.1007/s00253-013-5232-z
Duong, M. T.-Q., Qin, Y., You, S.-H., and Min, J.-J. (2019). Bacteria-cancer interactions: bacteria-based cancer therapy. Exp. and Mol. Med. 51 (12), 1–15. doi:10.1038/s12276-019-0297-0
Ektate, K., Munteanu, M. C., Ashar, H., Malayer, J., and Ranjan, A. (2018). Chemo-immunotherapy of colon cancer with focused ultrasound and Salmonella-laden temperature sensitive liposomes (thermobots). Sci. Rep. 8 (1), 13062. doi:10.1038/s41598-018-30106-4
Ellis, T. N., and Kuehn, M. J. (2010). Virulence and immunomodulatory roles of bacterial outer membrane vesicles. Microbiol. Mol. Biol. Rev. 74 (1), 81–94. doi:10.1128/mmbr.00031-09
Felfoul, O., Mohammadi, M., Taherkhani, S., De Lanauze, D., Zhong Xu, Y., Loghin, D., et al. (2016). Magneto-aerotactic bacteria deliver drug-containing nanoliposomes to tumour hypoxic regions. Nat. Nanotechnol. 11 (11), 941–947. doi:10.1038/nnano.2016.137
Felgner, S., Kocijancic, D., Frahm, M., Heise, U., Rohde, M., Zimmermann, K., et al. (2018). Engineered Salmonella enterica serovar Typhimurium overcomes limitations of anti-bacterial immunity in bacteria-mediated tumor therapy. Oncoimmunology 7 (2), e1382791. doi:10.1080/2162402x.2017.1382791
Feng, Z., Wang, Y., Xu, H., Guo, Y., Xia, W., Zhao, C., et al. (2023). Recent advances in bacterial therapeutics based on sense and response. Acta Pharm. Sin. B 13 (3), 1014–1027. doi:10.1016/j.apsb.2022.09.015
Ferlay, J., Ervik, M., and Lam, F. (2024). Global cancer observatory: cancer today. International Agency for Research on Cancer. Available at: https://www.researchgate.net/publication/378100307_Global_Cancer_Observatory_Cancer_Today
Flentie, K., Kocher, B., Gammon, S. T., Novack, D. V., McKinney, J. S., and Piwnica-Worms, D. (2012). A bioluminescent transposon reporter-trap identifies tumor-specific microenvironment-induced promoters in Salmonella for conditional bacterial-based tumor therapy. Cancer Discov. 2 (7), 624–637. doi:10.1158/2159-8290.cd-11-0201
Forbes, N. S. (2010). Engineering the perfect (bacterial) cancer therapy. Nat. Rev. Cancer 10 (11), 785–794. doi:10.1038/nrc2934
Fritz, S. E., Henson, M. S., Greengard, E., Winter, A. L., Stuebner, K. M., Yoon, U., et al. (2016). A phase I clinical study to evaluate safety of orally administered, genetically engineeredSalmonella entericaserovarTyphimuriumfor canine osteosarcoma. Vet. Med. Sci. 2 (3), 179–190. doi:10.1002/vms3.32
Fu, L., Ma, X., Liu, Y., Xu, Z., and Sun, Z. (2022). Applying nanotechnology to boost cancer immunotherapy by promoting immunogenic cell death. Chin. Chem. Lett. 33 (4), 1718–1728. doi:10.1016/j.cclet.2021.10.074
Gagnaire, A., Nadel, B., Raoult, D., Neefjes, J., and Gorvel, J.-P. (2017). Collateral damage: insights into bacterial mechanisms that predispose host cells to cancer. Nat. Rev. Microbiol. 15 (2), 109–128. doi:10.1038/nrmicro.2016.171
Gerstner, G. J., Ramalingam, S. S., Lisberg, A. E., Farber, C. M., Morganstein, N., Sanborn, R. E., et al. (2022). A phase 2 study of an off-the-shelf, multi-neoantigen vector (ADXS-503) in patients with metastatic non–small cell lung cancer either progressing on prior pembrolizumab or in the first-line setting. J. Clin. Oncol. 40 (16_Suppl. l), 9038. doi:10.1200/jco.2022.40.16_suppl.9038
Giacalone, M. J., Zapata, J. C., Berkley, N. L., Sabbadini, R. A., Chu, Y.-L., Salvato, M. S., et al. (2007). Immunization with non-replicating E. coli minicells delivering both protein antigen and DNA protects mice from lethal challenge with lymphocytic choriomeningitis virus. Vaccine 25 (12), 2279–2287. doi:10.1016/j.vaccine.2006.11.069
Gong, Z., and Dai, Z. (2021). Design and challenges of sonodynamic therapy system for cancer theranostics: from equipment to sensitizers. Adv. Sci. 8 (10), 2002178. doi:10.1002/advs.202002178
Grünberg, K., Müller, E.-C., Otto, A., Reszka, R., Linder, D., Kube, M., et al. (2004). Biochemical and proteomic analysis of the magnetosome membrane in Magnetospirillum gryphiswaldense. Appl. Environ. Microbiol. 70 (2), 1040–1050. doi:10.1128/aem.70.2.1040-1050.2004
Gu, T.-W., Wang, M.-Z., Niu, J., Chu, Y., Guo, K.-R., and Peng, L.-H. (2020). Outer membrane vesicles derived from E. coli as novel vehicles for transdermal and tumor targeting delivery. Nanoscale 12 (36), 18965–18977. doi:10.1039/d0nr03698f
Gujrati, V., Prakash, J., Malekzadeh-Najafabadi, J., Stiel, A., Klemm, U., Mettenleiter, G., et al. (2019). Bioengineered bacterial vesicles as biological nano-heaters for optoacoustic imaging. Nat. Commun. 10 (1), 1114. doi:10.1038/s41467-019-09034-y
Gurbatri, C. R., Lia, I., Vincent, R., Coker, C., Castro, S., Treuting, P. M., et al. (2020). Engineered probiotics for local tumor delivery of checkpoint blockade nanobodies. Sci. Transl. Med. 12 (530), eaax0876. doi:10.1126/scitranslmed.aax0876
Han, J.-W., Choi, Y. J., Cho, S., Zheng, S., Ko, S. Y., Park, J.-O., et al. (2016). Active tumor-therapeutic liposomal bacteriobot combining a drug (paclitaxel)-encapsulated liposome with targeting bacteria (Salmonella Typhimurium). Sensors Actuators B Chem. 224, 217–224. doi:10.1016/j.snb.2015.09.034
He, L., Yang, H., Tang, J., Liu, Z., Chen, Y., Lu, B., et al. (2019). Intestinal probiotics E. coli Nissle 1917 as a targeted vehicle for delivery of p53 and Tum-5 to solid tumors for cancer therapy. J. Biol. Eng. 13, 58–13. doi:10.1186/s13036-019-0189-9
Hill, P. J., Stritzker, J., Scadeng, M., Geissinger, U., Haddad, D., Basse-Lüsebrink, T. C., et al. (2011). Magnetic resonance imaging of tumors colonized with bacterial ferritin-expressing Escherichia coli. PLoS One 6 (10), e25409. doi:10.1371/journal.pone.0025409
Hoption Cann, S., Van Netten, J., and Van Netten, C. (2003). Dr William Coley and tumour regression: a place in history or in the future. Postgrad. Med. J. 79 (938), 672–680. doi:10.1093/postgradmedj/79.938.672
Hu, H., Feng, W., Qian, X., Yu, L., Chen, Y., and Li, Y. (2021). Emerging nanomedicine-enabled/enhanced nanodynamic therapies beyond traditional photodynamics. Adv. Mater. 33 (12), 2005062. doi:10.1002/adma.202005062
Hu, W., and Kavanagh, J. J. (2003). Anticancer therapy targeting the apoptotic pathway. lancet Oncol. 4 (12), 721–729. doi:10.1016/s1470-2045(03)01277-4
Huang, J., Yang, B., Peng, Y., Huang, J., Wong, S. H. D., Bian, L., et al. (2021). Nanomedicine-boosting tumor immunogenicity for enhanced immunotherapy. Adv. Funct. Mater. 31 (21), 2011171. doi:10.1002/adfm.202011171
Jagannadham, M., and Chattopadhyay, M. (2015). Role of outer membrane vesicles of bacteria. Resonance 20, 711–725. doi:10.1007/s12045-015-0228-x
Jain, R. K. (2013). Normalizing tumor microenvironment to treat cancer: bench to bedside to biomarkers. J. Clin. Oncol. 31 (17), 2205–2218. doi:10.1200/jco.2012.46.3653
Jiang, S.-N., Park, S.-H., Lee, H. J., Zheng, J. H., Kim, H.-S., Bom, H.-S., et al. (2013). Engineering of bacteria for the visualization of targeted delivery of a cytolytic anticancer agent. Mol. Ther. 21 (11), 1985–1995. doi:10.1038/mt.2013.183
Jiang, Z., Zhao, P., Zhou, Z., Liu, J., Qin, L., and Wang, H. (2007). Using attenuated salmonella typhi as tumor targeting vector for MDR1 siRNA delivery: an experimental study. Cancer Biol. and Ther. 6 (4), 555–560. doi:10.4161/cbt.6.4.3850
Jivrajani, M., and Nivsarkar, M. (2016). Ligand-targeted bacterial minicells: futuristic nano-sized drug delivery system for the efficient and cost effective delivery of shRNA to cancer cells. Nanomedicine Nanotechnol. Biol. Med. 12 (8), 2485–2498. doi:10.1016/j.nano.2016.06.004
Jivrajani, M., and Nivsarkar, M. (2019). Minicell-based targeted delivery of shRNA to cancer cells: an experimental protocol. RNA Interf. Cancer Ther. Methods Protoc. 1974, 111–139. doi:10.1007/978-1-4939-9220-1_9
Kamat, A. M., Flaig, T. W., Grossman, H. B., Konety, B., Lamm, D., O'donnell, M. A., et al. (2015). Consensus statement on best practice management regarding the use of intravesical immunotherapy with BCG for bladder cancer. Nat. Rev. Urol. 12 (4), 225–235. doi:10.1038/nrurol.2015.58
Kang, S. H., Bozhilov, K. N., Myung, N. V., Mulchandani, A., and Chen, W. (2008). Microbial synthesis of CdS nanocrystals in genetically engineered E. coli. Angew. Chem. Int. Ed. 47 (28), 5186–5189. doi:10.1002/anie.200705806
Karbach, J., Neumann, A., Brand, K., Wahle, C., Siegel, E., Maeurer, M., et al. (2012). Phase I clinical trial of mixed bacterial vaccine (Coley's toxins) in patients with NY-ESO-1 expressing cancers: immunological effects and clinical activity. Clin. Cancer Res. 18 (19), 5449–5459. doi:10.1158/1078-0432.ccr-12-1116
Karges, J. (2022). Clinical development of metal complexes as photosensitizers for photodynamic therapy of cancer. Angew. Chem. Int. Ed. 61 (5), e202112236. doi:10.1002/anie.202112236
Karges, J., Basu, U., Blacque, O., Chao, H., and Gasser, G. (2019). Polymeric encapsulation of novel homoleptic bis (dipyrrinato) zinc (II) complexes with long lifetimes for applications as photodynamic therapy photosensitisers. Angew. Chem. 131 (40), 14472–14478. doi:10.1002/ange.201907856
Kasat, V., Sahuji, S., and Joshi, M. (2010). Radiotherapy: an update. J. indian Acad. oral Med. radiology 22 (Suppl. 1), S26–S30. doi:10.5005/jp-journals-10011-1064
Kasinskas, R. W., and Forbes, N. S. (2006). Salmonella typhimurium specifically chemotax and proliferate in heterogeneous tumor tissue in vitro. Biotechnol. Bioeng. 94 (4), 710–721. doi:10.1002/bit.20883
Kasinskas, R. W., and Forbes, N. S. (2007). Salmonella typhimurium lacking ribose chemoreceptors localize in tumor quiescence and induce apoptosis. Cancer Res. 67 (7), 3201–3209. doi:10.1158/0008-5472.can-06-2618
Kavan, P., Saltzman, D. A., Muegge, J., Moradian, J., and Batist, G. (2023). Abstract CT035: addition of Salmonella-IL2 to FOLFIRINOX for metastatic stage 4 pancreatic cancer nearly doubles median survival. Cancer Res. 83 (8_Suppl. ment), CT035–CT. doi:10.1158/1538-7445.am2023-ct035
Kefayat, A., Ghahremani, F., Motaghi, H., Rostami, S., and Mehrgardi, M. A. (2019). Alive attenuated Salmonella as a cargo shuttle for smart carrying of gold nanoparticles to tumour hypoxic regions. J. drug Target. 27 (3), 315–324. doi:10.1080/1061186x.2018.1523417
Khalaf, K., Hana, D., Chou, J. T.-T., Singh, C., Mackiewicz, A., and Kaczmarek, M. (2021). Aspects of the tumor microenvironment involved in immune resistance and drug resistance. Front. Immunol. 12, 656364. doi:10.3389/fimmu.2021.656364
Kong, W., Brovold, M., Koeneman, B. A., Clark-Curtiss, J., and Curtiss, I. I. I. R. (2012). Turning self-destructing Salmonella into a universal DNA vaccine delivery platform. Proc. Natl. Acad. Sci. 109 (47), 19414–19419. doi:10.1073/pnas.1217554109
Kuang, X., Liu, Y., Luo, H., Li, Q., Wu, F., Fan, C., et al. (2023). Triggerable prodrug nanocoating enables on-demand activation of microbial and small-molecular therapeutics for combination treatment. J. Am. Chem. Soc. 145 (49), 26932–26946. doi:10.1021/jacs.3c10015
Kubiak, A. M., and Minton, N. P. (2015). The potential of clostridial spores as therapeutic delivery vehicles in tumour therapy. Res. Microbiol. 166 (4), 244–254. doi:10.1016/j.resmic.2014.12.006
Kucerova, P., and Cervinkova, M. (2016). Spontaneous regression of tumour and the role of microbial infection–possibilities for cancer treatment. Anti-cancer drugs 27 (4), 269–277. doi:10.1097/cad.0000000000000337
Kudela, P., Paukner, S., Mayr, U. B., Cholujova, D., Schwarczova, Z., Sedlak, J., et al. (2005). Bacterial ghosts as novel efficient targeting vehicles for DNA delivery to the human monocyte-derived dendritic cells. J. Immunother. 28 (2), 136–143. doi:10.1097/01.cji.0000154246.89630.6f
Kuerban, K., Gao, X., Zhang, H., Liu, J., Dong, M., Wu, L., et al. (2020). Doxorubicin-loaded bacterial outer-membrane vesicles exert enhanced anti-tumor efficacy in non-small-cell lung cancer. Acta Pharm. Sin. B 10 (8), 1534–1548. doi:10.1016/j.apsb.2020.02.002
Kumari, S., Advani, D., Sharma, S., Ambasta, R. K., and Kumar, P. (2021). Combinatorial therapy in tumor microenvironment: where do we stand? Biochimica Biophysica Acta (BBA)-Reviews Cancer 1876 (2), 188585. doi:10.1016/j.bbcan.2021.188585
Li, D., Yang, Y., Li, D., Pan, J., Chu, C., and Liu, G. (2021). Organic sonosensitizers for sonodynamic therapy: from small molecules and nanoparticles toward clinical development. Small. 17 (42), 2101976. doi:10.1002/smll.202101976
Li, G., Wang, S., Deng, D., Xiao, Z., Dong, Z., Wang, Z., et al. (2020). Fluorinated chitosan to enhance transmucosal delivery of sonosensitizer-conjugated catalase for sonodynamic bladder cancer treatment post-intravesical instillation. Acs Nano 14 (2), 1586–1599. doi:10.1021/acsnano.9b06689
Li, J., Dai, J., Zhao, L., Lin, S., Wen, Q., Wen, Q., et al. (2023). Bioactive bacteria/MOF hybrids can achieve targeted synergistic chemotherapy and chemodynamic therapy against breast tumors. Adv. Funct. Mater. 33 (42), 2303254. doi:10.1002/adfm.202303254
Li, L., Zhang, X., Zhou, J., Zhang, L., Xue, J., and Tao, W. (2022). Non-invasive thermal therapy for tissue engineering and regenerative medicine. Small 18 (36), 2107705. doi:10.1002/smll.202107705
Lin, Q., Rong, L., Jia, X., Li, R., Yu, B., Hu, J., et al. (2021). IFN-γ-dependent NK cell activation is essential to metastasis suppression by engineered Salmonella. Nat. Commun. 12 (1), 2537. doi:10.1038/s41467-021-22755-3
Liu, J., Yuan, S., Bremmer, A., and Hu, Q. (2024). Convergence of nanotechnology and bacteriotherapy for biomedical applications. Adv. Sci. 11 (16), 2309295. doi:10.1002/advs.202309295
Liu, L., He, H., Luo, Z., Zhou, H., Liang, R., Pan, H., et al. (2020b). In situ photocatalyzed oxygen generation with photosynthetic bacteria to enable robust immunogenic photodynamic therapy in triple-negative breast cancer. Adv. Funct. Mater. 30 (10), 1910176. doi:10.1002/adfm.201910176
Liu, Q., Luo, Q., Halim, A., and Song, G. (2017). Targeting lipid metabolism of cancer cells: a promising therapeutic strategy for cancer. Cancer Lett. 401, 39–45. doi:10.1016/j.canlet.2017.05.002
Liu, R.-t., Liu, J., Tong, J.-q., Tang, T., Kong, W.-C., Wang, X.-w., et al. (2012). Heating effect and biocompatibility of bacterial magnetosomes as potential materials used in magnetic fluid hyperthermia. Prog. Nat. Sci. Mater. Int. 22 (1), 31–39. doi:10.1016/j.pnsc.2011.12.006
Liu, S., Pan, X., and Liu, H. (2020a). Two-dimensional nanomaterials for photothermal therapy. Angew. Chem. 132 (15), 5943–5953. doi:10.1002/ange.201911477
Liu, T., Zhu, M., Chang, X., Tang, X., Yuan, P., Tian, R., et al. (2023). Tumor-specific photothermal-therapy-assisted immunomodulation via multiresponsive adjuvant nanoparticles. Adv. Mater. 35 (18), 2300086. doi:10.1002/adma.202300086
Liu, Y., Liu, Y., Bu, W., Cheng, C., Zuo, C., Xiao, Q., et al. (2015). Hypoxia induced by upconversion-based photodynamic therapy: towards highly effective synergistic bioreductive therapy in tumors. Angew. Chem. 127 (28), 8223–8227. doi:10.1002/ange.201500478
Liu, Y., Zhao, Y., and Chen, X. (2019). Bioengineering of metal-organic frameworks for nanomedicine. Theranostics 9 (11), 3122–3133. doi:10.7150/thno.31918
Long, R., Liu, Y., Dai, Q., Wang, S., Deng, Q., and Zhou, X. (2016). A natural bacterium-produced membrane-bound nanocarrier for drug combination therapy. Materials 9 (11), 889. doi:10.3390/ma9110889
Long, R.-M., Dai, Q.-L., Zhou, X., Cai, D.-H., Hong, Y.-Z., Wang, S.-B., et al. (2018). Bacterial magnetosomes-based nanocarriers for co-delivery of cancer therapeutics in vitro. Int. J. Nanomedicine Vol. 13, 8269–8279. doi:10.2147/ijn.s180503
Lundin, J. I., and Checkoway, H. (2009). Endotoxin and cancer. Environ. health Perspect. 117 (9), 1344–1350. doi:10.1289/ehp.0800439
Luo, C.-H., Huang, C.-T., Su, C.-H., and Yeh, C.-S. (2016). Bacteria-mediated hypoxia-specific delivery of nanoparticles for tumors imaging and therapy. Nano Lett. 16 (6), 3493–3499. doi:10.1021/acs.nanolett.6b00262
Lv, H., Ma, S., Wang, Z., Ji, X., Lv, S., and Ding, C. (2021). Glutathione-triggered non-template synthesized porous carbon nanospheres serve as low toxicity targeted delivery system for cancer multi-therapy. Chin. Chem. Lett. 32 (5), 1765–1769. doi:10.1016/j.cclet.2020.11.058
MacDiarmid, J. A., Amaro-Mugridge, N. B., Madrid-Weiss, J., Sedliarou, I., Wetzel, S., Kochar, K., et al. (2009). Sequential treatment of drug-resistant tumors with targeted minicells containing siRNA or a cytotoxic drug. Nat. Biotechnol. 27 (7), 643–651. doi:10.1038/nbt.1547
MacDiarmid, J. A., Langova, V., Bailey, D., Pattison, S. T., Pattison, S. L., Christensen, N., et al. (2016). Targeted doxorubicin delivery to brain tumors via minicells: proof of principle using dogs with spontaneously occurring tumors as a model. PloS one 11 (4), e0151832. doi:10.1371/journal.pone.0151832
MacDiarmid, J. A., Mugridge, N. B., Weiss, J. C., Phillips, L., Burn, A. L., Paulin, R. P., et al. (2007). Bacterially derived 400 nm particles for encapsulation and cancer cell targeting of chemotherapeutics. Cancer Cell. 11 (5), 431–445. doi:10.1016/j.ccr.2007.03.012
Makela, A. V., Schott, M. A., Madsen, C. S., Greeson, E. M., and Contag, C. H. (2022). Magnetic particle imaging of magnetotactic bacteria as living contrast agents is improved by altering magnetosome arrangement. Nano Lett. 22 (12), 4630–4639. doi:10.1021/acs.nanolett.1c05042
Maman, S., and Witz, I. P. (2018). A history of exploring cancer in context. Nat. Rev. Cancer 18 (6), 359–376. doi:10.1038/s41568-018-0006-7
Mathis, T., Jardel, P., Loria, O., Delaunay, B., Nguyen, A., Lanza, F., et al. (2019). New concepts in the diagnosis and management of choroidal metastases. Prog. Retin. eye Res. 68, 144–176. doi:10.1016/j.preteyeres.2018.09.003
Mei, Y., Wang, R., Jiang, W., Bo, Y., Zhang, T., Yu, J., et al. (2019). Recent progress in nanomaterials for nucleic acid delivery in cancer immunotherapy. Biomaterials Sci. 7 (7), 2640–2651. doi:10.1039/c9bm00214f
Mengesha, A., Dubois, L., Chiu, R. K., Paesmans, K., Wouters, B. G., Lambin, P., et al. (2007). Potential and limitations of bacterial-mediated cancer therapy. Front. Biosci. 12, 3880–3891. doi:10.2741/2357
Mengesha, A., Dubois, L., Lambin, P., Landuyt, W., Chiu, R. K., Wouters, B. G., et al. (2006). Development of a flexible and potent hypoxia-inducible promoter for tumor-targeted gene expression in attenuated s almonella. Cancer Biol. and Ther. 5 (9), 1120–1128. doi:10.4161/cbt.5.9.2951
Mercado-Lubo, R., Zhang, Y., Zhao, L., Rossi, K., Wu, X., Zou, Y., et al. (2016). A Salmonella nanoparticle mimic overcomes multidrug resistance in tumours. Nat. Commun. 7 (1), 12225. doi:10.1038/ncomms12225
Min, J.-J., Kim, H.-J., Park, J. H., Moon, S., Jeong, J. H., Hong, Y.-J., et al. (2008). Noninvasive real-time imaging of tumors and metastases using tumor-targeting light-emitting Escherichia coli. Mol. imaging Biol. 10, 54–61. doi:10.1007/s11307-007-0120-5
Mostaghaci, B., Yasa, O., Zhuang, J., and Sitti, M. (2017). Bioadhesive bacterial microswimmers for targeted drug delivery in the urinary and gastrointestinal tracts. Adv. Sci. 4 (6), 1700058. doi:10.1002/advs.201700058
Mowday, A. M., Guise, C. P., Ackerley, D. F., Minton, N. P., Lambin, P., Dubois, L. J., et al. (2016). Advancing Clostridia to clinical trial: past lessons and recent progress. Cancers 8 (7), 63. doi:10.3390/cancers8070063
Nagakubo, T., Nomura, N., and Toyofuku, M. (2020). Cracking open bacterial membrane vesicles. Front. Microbiol. 10, 3026. doi:10.3389/fmicb.2019.03026
Nelson, B. E., Janku, F., Fu, S., Dumbrava, E. I., Hong, D. S., Karp, D., et al. (2023). Abstract CT107: phase ib study of pembrolizumab in combination with intratumoral injection of Clostridium novyi-NT in patients with advanced solid tumors. Cancer Res. 83 (8_Suppl. ment), CT107–CT. doi:10.1158/1538-7445.am2023-ct107
Ni, D., Qing, S., Ding, H., Yue, H., Yu, D., Wang, S., et al. (2017). Biomimetically engineered demi-bacteria potentiate vaccination against cancer. Adv. Sci. 4 (10), 1700083. doi:10.1002/advs.201700083
Nishikawa, H., Sato, E., Briones, G., Chen, L.-M., Matsuo, M., Nagata, Y., et al. (2006). In vivo antigen delivery by a Salmonella typhimurium type III secretion system for therapeutic cancer vaccines. J. Clin. investigation 116 (7), 1946–1954. doi:10.1172/JCI28045
Ouyang, C., Zhang, S., Xue, C., Yu, X., Xu, H., Wang, Z., et al. (2020). Precision-guided missile-like DNA nanostructure containing warhead and guidance control for aptamer-based targeted drug delivery into cancer cells in vitro and in vivo. J. Am. Chem. Soc. 142 (3), 1265–1277. doi:10.1021/jacs.9b09782
Pan, P., Dong, X., Chen, Y., Zeng, X., and Zhang, X.-Z. (2022). Engineered bacteria for enhanced radiotherapy against breast carcinoma. ACS Nano 16 (1), 801–812. doi:10.1021/acsnano.1c08350
Park, B.-W., Zhuang, J., Yasa, O., and Sitti, M. (2017). Multifunctional bacteria-driven microswimmers for targeted active drug delivery. ACS Nano 11 (9), 8910–8923. doi:10.1021/acsnano.7b03207
Park, H., Otte, A., and Park, K. (2022). Evolution of drug delivery systems: from 1950 to 2020 and beyond. J. Control. Release 342, 53–65. doi:10.1016/j.jconrel.2021.12.030
Park, T. J., Lee, S. Y., Heo, N. S., and Seo, T. S. (2010). In vivo synthesis of diverse metal nanoparticles by recombinant Escherichia coli. Angew. Chem. Int. Ed. 49 (39), 7019–7024. doi:10.1002/anie.201001524
Paukner, S., Kohl, G., and Lubitz, W. (2004). Bacterial ghosts as novel advanced drug delivery systems: antiproliferative activity of loaded doxorubicin in human Caco-2 cells. J. Control. release 94 (1), 63–74. doi:10.1016/j.jconrel.2003.09.010
Pei, P., Zhang, Y., Jiang, Y., Shen, W., Chen, H., Yang, S., et al. (2022). Pleiotropic immunomodulatory functions of radioactive inactivated bacterial vectors for enhanced cancer radio-immunotherapy. ACS Nano 16 (7), 11325–11337. doi:10.1021/acsnano.2c04982
Pettenati, C., and Ingersoll, M. A. (2018). Mechanisms of BCG immunotherapy and its outlook for bladder cancer. Nat. Rev. Urol. 15 (10), 615–625. doi:10.1038/s41585-018-0055-4
Piffoux, M., Silva, A. K., Wilhelm, C., Gazeau, F., and Tareste, D. (2018). Modification of extracellular vesicles by fusion with liposomes for the design of personalized biogenic drug delivery systems. ACS Nano 12 (7), 6830–6842. doi:10.1021/acsnano.8b02053
Qing, G., Gong, N., Chen, X., Chen, J., Zhang, H., Wang, Y., et al. (2019). Natural and engineered bacterial outer membrane vesicles. Biophys. Rep. 5, 184–198. doi:10.1007/s41048-019-00095-6
Qing, S., Lyu, C., Zhu, L., Pan, C., Wang, S., Li, F., et al. (2020). Biomineralized bacterial outer membrane vesicles potentiate safe and efficient tumor microenvironment reprogramming for anticancer therapy. Adv. Mater. 32 (47), 2002085. doi:10.1002/adma.202002085
Quispe-Tintaya, W., Chandra, D., Jahangir, A., Harris, M., Casadevall, A., Dadachova, E., et al. (2013). Nontoxic radioactive Listeria at is a highly effective therapy against metastatic pancreatic cancer. Proc. Natl. Acad. Sci. 110 (21), 8668–8673. doi:10.1073/pnas.1211287110
Rabea, S., Alanazi, F. K., Ashour, A. E., Salem-Bekhit, M. M., Yassin, A. S., Moneib, N. A., et al. (2020). Salmonella-innovative targeting carrier: loading with doxorubicin for cancer treatment. Saudi Pharm. J. 28 (10), 1253–1262. doi:10.1016/j.jsps.2020.08.016
Rajkumar, K., Mvs, S., Koganti, S., and Burgula, S. (2020). Pseudomonas stutzeri. Int. J. nanomedicine Vol. 15, 4523–4540. doi:10.2147/ijn.s247426
Ramalingam, S. S., Stinchcombe, T., Gerstner, G. J., Haigentz, M., Vangala, S., Parsi, M., et al. (2020). A phase I study of ADXS-503 alone and in combination with pembrolizumab in subjects with metastatic squamous or non-squamous non-small cell lung cancer (NSCLC). J. Clin. Oncol. 38 (15_Suppl. l), e21682–e. doi:10.1200/jco.2020.38.15_suppl.e21682
Rao, L., Cai, B., Bu, L.-L., Liao, Q.-Q., Guo, S.-S., Zhao, X.-Z., et al. (2017). Microfluidic electroporation-facilitated synthesis of erythrocyte membrane-coated magnetic nanoparticles for enhanced imaging-guided cancer therapy. ACS Nano 11 (4), 3496–3505. doi:10.1021/acsnano.7b00133
Redenti, A., Im, J., Redenti, B., Li, F., Rouanne, M., Sheng, Z., et al. (2024). Probiotic neoantigen delivery vectors for precision cancer immunotherapy. Nature 635, 453–461. doi:10.1038/s41586-024-08033-4
Sagnella, S. M., Trieu, J., Brahmbhatt, H., MacDiarmid, J. A., MacMillan, A., Whan, R. M., et al. (2018). Targeted doxorubicin-loaded bacterially derived nano-cells for the treatment of neuroblastoma. Mol. cancer Ther. 17 (5), 1012–1023. doi:10.1158/1535-7163.mct-17-0738
Sagnella, S. M., Yang, L., Stubbs, G. E., Boslem, E., Martino-Echarri, E., Smolarczyk, K., et al. (2020). Cyto-immuno-therapy for cancer: a pathway elicited by tumor-targeted, cytotoxic drug-packaged bacterially derived nanocells. Cancer Cell. 37 (3), 354–370. e7. doi:10.1016/j.ccell.2020.02.001
Schmitt, M., and Greten, F. R. (2021). The inflammatory pathogenesis of colorectal cancer. Nat. Rev. Immunol. 21 (10), 653–667. doi:10.1038/s41577-021-00534-x
Schüler, D. (2008). Genetics and cell biology of magnetosome formation in magnetotactic bacteria. FEMS Microbiol. Rev. 32 (4), 654–672. doi:10.1111/j.1574-6976.2008.00116.x
Scott, S. R., Din, M. O., Bittihn, P., Xiong, L., Tsimring, L. S., and Hasty, J. (2017). A stabilized microbial ecosystem of self-limiting bacteria using synthetic quorum-regulated lysis. Nat. Microbiol. 2 (8), 17083–17089. doi:10.1038/nmicrobiol.2017.83
Selvanesan, B. C., Chandra, D., Quispe-Tintaya, W., Jahangir, A., Patel, A., Meena, K., et al. (2022). Listeria delivers tetanus toxoid protein to pancreatic tumors and induces cancer cell death in mice. Sci. Transl. Med. 14 (637), eabc1600. doi:10.1126/scitranslmed.abc1600
Seya, T., Matsumoto, M., Tsuji, S., Begum, N., Nomura, M., Azuma, I., et al. (2001). Two receptor theory in innate immune activation: studies on the receptors for bacillus Culmet Guillen-cell wall skeleton. ARCHIVUM Immunol. Ther. EXPERIMENTALIS-ENGLISH EDITION- 49, S13–S21.
Shang, T., Yu, X., Han, S., and Yang, B. (2020). Nanomedicine-based tumor photothermal therapy synergized immunotherapy. Biomaterials Sci. 8 (19), 5241–5259. doi:10.1039/d0bm01158d
Shen, F., Tao, D., Peng, R., He, Y., Liu, Z., Ji, J., et al. (2022). Immunogenic nanomedicine based on GSH-responsive nanoscale covalent organic polymers for chemo-sonodynamic therapy. Biomaterials 283, 121428. doi:10.1016/j.biomaterials.2022.121428
Silhavy, T. J., Kahne, D., and Walker, S. (2010). The bacterial cell envelope. Cold Spring Harb. Perspect. Biol. 2 (5), a000414. doi:10.1101/cshperspect.a000414
Solomon, B. J., Desai, J., Rosenthal, M., McArthur, G. A., Pattison, S. T., Pattison, S. L., et al. (2015). A first-time-in-human phase I clinical trial of bispecific antibody-targeted, paclitaxel-packaged bacterial minicells. PloS one 10 (12), e0144559. doi:10.1371/journal.pone.0144559
Son, S., Kim, J. H., Wang, X., Zhang, C., Yoon, S. A., Shin, J., et al. (2020). Multifunctional sonosensitizers in sonodynamic cancer therapy. Chem. Soc. Rev. 49 (11), 3244–3261. doi:10.1039/c9cs00648f
Starnes, C. (1993). Coley's toxins, tumor necrosis factor, and cancer research—a historical perspective. J. Immunother. 13 (1), 73. doi:10.1097/00002371-199301000-00058
Stewart, C. J., Ajami, N. J., O’Brien, J. L., Hutchinson, D. S., Smith, D. P., Wong, M. C., et al. (2018). Temporal development of the gut microbiome in early childhood from the TEDDY study. Nature 562 (7728), 583–588. doi:10.1038/s41586-018-0617-x
St Jean, A. T., Zhang, M., and Forbes, N. S. (2008). Bacterial therapies: completing the cancer treatment toolbox. Curr. Opin. Biotechnol. 19 (5), 511–517. doi:10.1016/j.copbio.2008.08.004
Stritzker, J., Weibel, S., Seubert, C., Götz, A., Tresch, A., Van Rooijen, N., et al. (2010). Enterobacterial tumor colonization in mice depends on bacterial metabolism and macrophages but is independent of chemotaxis and motility. Int. J. Med. Microbiol. 300 (7), 449–456. doi:10.1016/j.ijmm.2010.02.004
Su, Y.-W., Lee, A. M., Xu, X., Hua, B., Tapp, H., Wen, X.-S., et al. (2023). Methotrexate chemotherapy causes growth impairments, vitamin D deficiency, bone loss, and altered intestinal metabolism—effects of calcitriol supplementation. Cancers 15 (17), 4367. doi:10.3390/cancers15174367
Suh, S., Jo, A., Traore, M. A., Zhan, Y., Coutermarsh-Ott, S. L., Ringel-Scaia, V. M., et al. (2019). Nanoscale bacteria-enabled autonomous drug delivery system (NanoBEADS) enhances intratumoral transport of nanomedicine. Adv. Sci. 6 (3), 1801309. doi:10.1002/advs.201801309
Sun, J., Li, Y., Liang, X.-J., and Wang, P. C. (2011). Bacterial magnetosome: a novel biogenetic magnetic targeted drug carrier with potential multifunctions. J. Nanomater. 2011 (2011), 469031–469043. doi:10.1155/2011/469031
Sun, M., Ye, H., Shi, Q., Xie, J., Yu, X., Ling, H., et al. (2021). Both-in-one hybrid bacteria suppress the tumor metastasis and relapse via tandem-amplifying reactive oxygen species-immunity responses. Adv. Healthc. Mater. 10 (21), 2100950. doi:10.1002/adhm.202100950
Tabrizi, C. A., Walcher, P., Mayr, U. B., Stiedl, T., Binder, M., McGrath, J., et al. (2004). Bacterial ghosts–biological particles as delivery systems for antigens, nucleic acids and drugs. Curr. Opin. Biotechnol. 15 (6), 530–537. doi:10.1016/j.copbio.2004.10.004
Taherkhani, S., Mohammadi, M., Daoud, J., Martel, S., and Tabrizian, M. (2014). Covalent binding of nanoliposomes to the surface of magnetotactic bacteria for the synthesis of self-propelled therapeutic agents. ACS Nano 8 (5), 5049–5060. doi:10.1021/nn5011304
Tamburini, S., Shen, N., Wu, H. C., and Clemente, J. C. (2016). The microbiome in early life: implications for health outcomes. Nat. Med. 22 (7), 713–722. doi:10.1038/nm.4142
Tian, S., Lin, G., Piao, L., and Liu, X. (2022). Del-1 enhances therapeutic efficacy of bacterial cancer immunotherapy by blocking recruitment of tumor-infiltrating neutrophils. Clin. Transl. Oncol. 24, 244–253. doi:10.1007/s12094-021-02679-6
Toso, J. F., Gill, V. J., Hwu, P., Marincola, F. M., Restifo, N. P., Schwartzentruber, D. J., et al. (2002). Phase I study of the intravenous administration of attenuated Salmonella typhimurium to patients with metastatic melanoma. J. Clin. Oncol. 20 (1), 142–152. doi:10.1200/jco.20.1.142
Tsujikawa, T., Crocenzi, T., Durham, J. N., Sugar, E. A., Wu, A. A., Onners, B., et al. (2020). Evaluation of cyclophosphamide/GVAX pancreas followed by listeria-mesothelin (CRS-207) with or without nivolumab in patients with pancreatic cancer. Clin. Cancer Res. 26 (14), 3578–3588. doi:10.1158/1078-0432.ccr-19-3978
Vairavel, M., Devaraj, E., and Shanmugam, R. (2020). An eco-friendly synthesis of Enterococcus sp.–mediated gold nanoparticle induces cytotoxicity in human colorectal cancer cells. Environ. Sci. Pollut. Res. 27 (8), 8166–8175. doi:10.1007/s11356-019-07511-x
Vizsnyiczai, G., Frangipane, G., Bianchi, S., Saglimbeni, F., Dell’Arciprete, D., and Di Leonardo, R. (2020). A transition to stable one-dimensional swimming enhances E. coli motility through narrow channels. Nat. Commun. 11 (1), 2340. doi:10.1038/s41467-020-15711-0
Wang, C., Chen, L., Zhu, J., Wang, C., Li, M., Miao, Y., et al. (2024). Programmable bacteria-based biohybrids as living biotherapeutics for enhanced cancer sonodynamic-immunotherapy. Adv. Funct. Mater. 34, 2316092. doi:10.1002/adfm.202316092
Wang, D., Liu, C., You, S., Zhang, K., Li, M., Cao, Y., et al. (2020). Bacterial vesicle-cancer cell hybrid membrane-coated nanoparticles for tumor specific immune activation and photothermal therapy. ACS Appl. Mater. and interfaces 12 (37), 41138–41147. doi:10.1021/acsami.0c13169
Wang, H., Liu, H., Guo, Y., Zai, W., Li, X., Xiong, W., et al. (2022b). Photosynthetic microorganisms coupled photodynamic therapy for enhanced antitumor immune effect. Bioact. Mater. 12, 97–106. doi:10.1016/j.bioactmat.2021.10.028
Wang, L., Qin, W., Xu, W., Huang, F., Xie, X., Wang, F., et al. (2021b). Bacteria-mediated tumor therapy via photothermally-programmed cytolysin a expression. Small 17 (40), 2102932. doi:10.1002/smll.202102932
Wang, W., Xu, H., Ye, Q., Tao, F., Wheeldon, I., Yuan, A., et al. (2022a). Systemic immune responses to irradiated tumours via the transport of antigens to the tumour periphery by injected flagellate bacteria. Nat. Biomed. Eng. 6 (1), 44–53. doi:10.1038/s41551-021-00834-6
Wang, Y., Li, X., Zheng, D., Chen, Y., Zhang, Z., and Yang, Z. (2021a). Selective degradation of PD-L1 in cancer cells by enzyme-instructed self-assembly. Adv. Funct. Mater. 31 (45), 2102505. doi:10.1002/adfm.202102505
Wang, Z., Yang, J., Qin, G., Zhao, C., Ren, J., and Qu, X. (2022c). An intelligent nanomachine guided by DNAzyme logic system for precise chemodynamic therapy. Angew. Chem. Int. Ed. 61 (38), e202204291. doi:10.1002/anie.202204291
Warrier, V. U., Makandar, A. I., Garg, M., Sethi, G., Kant, R., Pal, J. K., et al. (2019). Engineering anti-cancer nanovaccine based on antigen cross-presentation. Biosci. Rep. 39 (10), BSR20193220. doi:10.1042/bsr20193220
West, C. M., and Slevin, F. (2019). Tumour hypoxia. Clin. Oncol. 31, 595–599. doi:10.1016/j.clon.2019.06.008
Wu, G., Ji, H., Guo, X., Li, Y., Ren, T., Dong, H., et al. (2020). Nanoparticle reinforced bacterial outer-membrane vesicles effectively prevent fatal infection of carbapenem-resistant Klebsiella pneumoniae. Nanomedicine Nanotechnol. Biol. Med. 24, 102148. doi:10.1016/j.nano.2019.102148
Wu, L., Bao, F., Li, L., Yin, X., and Hua, Z. (2022). Bacterially mediated drug delivery and therapeutics: strategies and advancements. Adv. drug Deliv. Rev. 187, 114363. doi:10.1016/j.addr.2022.114363
Wu, M., Wu, W., Duan, Y., Li, X., Qi, G., and Liu, B. (2019). Photosensitizer-bacteria biohybrids promote photodynamic cancer cell ablation and intracellular protein delivery. Chem. Mater. 31 (18), 7212–7220. doi:10.1021/acs.chemmater.9b01518
Xie, S., Chen, M., Song, X., Zhang, Z., Zhang, Z., Chen, Z., et al. (2018). Bacterial microbots for acid-labile release of hybrid micelles to promote the synergistic antitumor efficacy. Acta Biomater. 78, 198–210. doi:10.1016/j.actbio.2018.07.041
Xie, S., Li, S., Zhang, Z., Chen, M., Ran, P., and Li, X. (2020). Bacterial ghosts for targeting delivery and subsequent responsive release of ciprofloxacin to destruct intracellular bacteria. Chem. Eng. J. 399, 125700. doi:10.1016/j.cej.2020.125700
Xie, S., Zhang, P., Zhang, Z., Liu, Y., Chen, M., Li, S., et al. (2021). Bacterial navigation for tumor targeting and photothermally-triggered bacterial ghost transformation for spatiotemporal drug release. Acta Biomater. 131, 172–184. doi:10.1016/j.actbio.2021.06.030
Xie, S., Zhao, L., Song, X., Tang, M., Mo, C., and Li, X. (2017). Doxorubicin-conjugated Escherichia coli Nissle 1917 swimmers to achieve tumor targeting and responsive drug release. J. Control. Release 268, 390–399. doi:10.1016/j.jconrel.2017.10.041
Xing, J., Yin, T., Li, S., Xu, T., Ma, A., Chen, Z., et al. (2021). Sequential magneto-actuated and optics-triggered biomicrorobots for targeted cancer therapy. Adv. Funct. Mater. 31 (11), 2008262. doi:10.1002/adfm.202008262
Xiong, Y., Rao, Y., Hu, J., Luo, Z., and Chen, C. (2023). Nanoparticle-based photothermal therapy for breast cancer noninvasive treatment. Adv. Mater., 2305140. doi:10.1002/adma.202305140
Yang, B., Ding, L., Yao, H., Chen, Y., and Shi, J. (2020a). A metal-organic framework (MOF) Fenton nanoagent-enabled nanocatalytic cancer therapy in synergy with autophagy inhibition. Adv. Mater. 32 (12), 1907152. doi:10.1002/adma.201907152
Yang, J., Chawla, R., Rhee, K. Y., Gupta, R., Manson, M. D., Jayaraman, A., et al. (2020c). Biphasic chemotaxis of Escherichia coli to the microbiota metabolite indole. Proc. Natl. Acad. Sci. 117 (11), 6114–6120. doi:10.1073/pnas.1916974117
Yang, J., Wang, Q., Wang, C., Yang, R., Ahmed, M., Kumaran, S., et al. (2020b). Pseudomonas aeruginosa synthesized silver nanoparticles inhibit cell proliferation and induce ROS mediated apoptosis in thyroid cancer cell line (TPC1). Artif. cells, nanomedicine, Biotechnol. 48 (1), 800–809. doi:10.1080/21691401.2019.1687495
Ye, P., Li, F., Zou, J., Luo, Y., Wang, S., Lu, G., et al. (2022). In situ generation of gold nanoparticles on bacteria-derived magnetosomes for imaging-guided starving/chemodynamic/photothermal synergistic therapy against cancer. Adv. Funct. Mater. 32 (17), 2110063. doi:10.1002/adfm.202110063
Yi, G., Son, J., Yoo, J., Park, C., and Koo, H. (2019). Rhamnolipid nanoparticles for in vivo drug delivery and photodynamic therapy. Nanomedicine Nanotechnol. Biol. Med. 19, 12–21. doi:10.1016/j.nano.2019.03.015
Yin, T., Diao, Z., Blum, N. T., Qiu, L., Ma, A., and Huang, P. (2022). Engineering bacteria and bionic bacterial derivatives with nanoparticles for cancer therapy. Small 18 (12), 2104643. doi:10.1002/smll.202104643
Yoo, Y. C., Hata, K., Lee, K. B., and Azuma, I. (2002). Inhibitory effect of BCG cell-wall skeletons (BCG-CWS) emulsified in squalane on tumor growth and metastasis in mice. Archives pharmacal Res. 25, 522–527. doi:10.1007/bf02976612
Yoon, W., Park, Y. C., Kim, J., Chae, Y. S., Byeon, J. H., Min, S.-H., et al. (2017). Application of genetically engineered Salmonella typhimurium for interferon-gamma–induced therapy against melanoma. Eur. J. Cancer 70, 48–61. doi:10.1016/j.ejca.2016.10.010
Yu, B., Liu, M., Jiang, L., Xu, C., Hu, H., Huang, T., et al. (2024). Aggregation-induced emission photosensitizer-engineered anticancer nanomedicine for synergistic chemo/chemodynamic/photodynamic therapy. Adv. Healthc. Mater. 13 (11), 2303643. doi:10.1002/adhm.202303643
Zhang, F., Li, F., Lu, G.-H., Nie, W., Zhang, L., Lv, Y., et al. (2019a). Engineering magnetosomes for ferroptosis/immunomodulation synergism in cancer. ACS Nano 13 (5), 5662–5673. doi:10.1021/acsnano.9b00892
Zhang, T., Zhou, M., Xiao, D., Liu, Z., Jiang, Y., Feng, M., et al. (2022). Myelosuppression alleviation and hematopoietic regeneration by tetrahedral-framework nucleic-acid nanostructures functionalized with osteogenic growth peptide. Adv. Sci. 9 (27), 2202058. doi:10.1002/advs.202202058
Zhang, W., Liu, J., Li, X., Zheng, Y., Chen, L., Wang, D., et al. (2021). Precise chemodynamic therapy of cancer by trifunctional bacterium-based nanozymes. ACS Nano 15 (12), 19321–19333. doi:10.1021/acsnano.1c05605
Zhang, Y., Chen, Y., Lo, C., Zhuang, J., Angsantikul, P., Zhang, Q., et al. (2019b). Inhibition of pathogen adhesion by bacterial outer membrane-coated nanoparticles. Angew. Chem. Int. Ed. 58 (33), 11404–11408. doi:10.1002/anie.201906280
Zhang, Y., Ji, W., He, L., Chen, Y., Ding, X., Sun, Y., et al. (2018b). E. coli Nissle 1917-derived minicells for targeted delivery of chemotherapeutic drug to hypoxic regions for cancer therapy. Theranostics 8 (6), 1690–1705. doi:10.7150/thno.21575
Zhang, Y., Ni, Q., Xu, C., Wan, B., Geng, Y., Zheng, G., et al. (2018a). Smart bacterial magnetic nanoparticles for tumor-targeting magnetic resonance imaging of HER2-positive breast cancers. ACS Appl. Mater. and interfaces 11 (4), 3654–3665. doi:10.1021/acsami.8b15838
Zhao, J., Huang, H., Zhao, J., Xiong, X., Zheng, S., Wei, X., et al. (2022). A hybrid bacterium with tumor-associated macrophage polarization for enhanced photothermal-immunotherapy. Acta Pharm. Sin. B 12 (6), 2683–2694. doi:10.1016/j.apsb.2021.10.019
Zhao, M., Yang, M., Li, X.-M., Jiang, P., Baranov, E., Li, S., et al. (2005). Tumor-targeting bacterial therapy with amino acid auxotrophs of GFP-expressing Salmonella typhimurium. Proc. Natl. Acad. Sci. 102 (3), 755–760. doi:10.1073/pnas.0408422102
Zheng, J. H., Nguyen, V. H., Jiang, S.-N., Park, S.-H., Tan, W., Hong, S. H., et al. (2017). Two-step enhanced cancer immunotherapy with engineered Salmonella typhimurium secreting heterologous flagellin. Sci. Transl. Med. 9 (376), eaak9537. doi:10.1126/scitranslmed.aak9537
Zhou, S., Gravekamp, C., Bermudes, D., and Liu, K. (2018). Tumour-targeting bacteria engineered to fight cancer. Nat. Rev. Cancer 18 (12), 727–743. doi:10.1038/s41568-018-0070-z
Zhou, Y., Fan, S., Feng, L., Huang, X., and Chen, X. (2021). Manipulating intratumoral fenton chemistry for enhanced chemodynamic and chemodynamic-synergized multimodal therapy. Adv. Mater. 33 (48), 2104223. doi:10.1002/adma.202104223
Zhu, D., Zhang, J., Luo, G., Duo, Y., and Tang, B. Z. (2021). Bright bacterium for hypoxia-tolerant photodynamic therapy against orthotopic colon tumors by an interventional method. Adv. Sci. 8 (15), 2004769. doi:10.1002/advs.202004769
Zhu, R., Zhang, F., Peng, Y., Xie, T., Wang, Y., and Lan, Y. (2022). Current progress in cancer treatment using nanomaterials. Front. Oncol. 12, 930125. doi:10.3389/fonc.2022.930125
Zhu, Y., Gong, P., Wang, J., Cheng, J., Wang, W., Cai, H., et al. (2023). Amplification of lipid peroxidation by regulating cell membrane unsaturation to enhance chemodynamic therapy. Angew. Chem. Int. Ed. 62 (12), e202218407. doi:10.1002/anie.202218407
Zitvogel, L., Ayyoub, M., Routy, B., and Kroemer, G. (2016). Microbiome and anticancer immunosurveillance. Cell. 165 (2), 276–287. doi:10.1016/j.cell.2016.03.001
Keywords: bacteria, bacterial derivatives, drug delivery, bacteriotherapy, cancer
Citation: Yan S, Gan Y, Xu H and Piao H (2025) Bacterial carrier-mediated drug delivery systems: a promising strategy in cancer therapy. Front. Bioeng. Biotechnol. 12:1526612. doi: 10.3389/fbioe.2024.1526612
Received: 12 November 2024; Accepted: 17 December 2024;
Published: 08 January 2025.
Edited by:
Teruyoshi Sasayama, Kyushu University, JapanReviewed by:
Regina Tavano, University of Padua, ItalyRakesh A. Afre, Assam Down Town University, India
Copyright © 2025 Yan, Gan, Xu and Piao. This is an open-access article distributed under the terms of the Creative Commons Attribution License (CC BY). The use, distribution or reproduction in other forums is permitted, provided the original author(s) and the copyright owner(s) are credited and that the original publication in this journal is cited, in accordance with accepted academic practice. No use, distribution or reproduction is permitted which does not comply with these terms.
*Correspondence: Huizhe Xu, eHVodWl6aGVAY2FuY2VyaG9zcC1sbi1jbXUuY29t; Haozhe Piao, cHpweUAxNjMuY29t