- 1Nano-Bio Regenerative Medical Institute, College of Medicine, Hallym University, Chuncheon, Republic of Korea
- 2Departments of Otorhinolaryngology-Head and Neck Surgery, Chuncheon Sacred Heart Hospital, School of Medicine, Hallym University, Chuncheon, Republic of Korea
- 3Department of Breast and Endocrine Surgery, Hallym University Sacred Heart Hospital, Anyang, Republic of Korea
3D cell culture is gaining momentum in medicine due to its ability to mimic real tissues (in vivo) and provide more accurate biological data compared to traditional methods. This review explores the current state of 3D cell culture in medicine and discusses future directions, including the need for standardization and simpler protocols to facilitate wider use in research.
Purpose: 3D cell culture develops life sciences by mimicking the natural cellular environment. Cells in 3D cultures grow in three dimensions and interact with a matrix, fostering realistic cell behavior and interactions. This enhanced model offers significant advantages for diverse research areas.
Methods: By mimicking the cellular organization and functionalities found in human tissues, 3D cultures provide superior platforms for studying complex diseases like cancer and neurodegenerative disorders. This enables researchers to gain deeper insights into disease progression and identify promising therapeutic targets with greater accuracy. 3D cultures also play a crucial role in drug discovery by allowing researchers to effectively assess potential drugs’ safety and efficacy.
Results: 3D cell culture’s impact goes beyond disease research. It holds promise for tissue engineering. By replicating the natural tissue environment and providing a scaffold for cell growth, 3D cultures pave the way for regenerating damaged tissues, offering hope for treating burns, organ failure, and musculoskeletal injuries. Additionally, 3D cultures contribute to personalized medicine. Researchers can use patient-derived cells to create personalized disease models and identify the most effective treatment for each individual.
Conclusion: With ongoing advancements in cell imaging techniques, the development of novel biocompatible scaffolds and bioreactor systems, and a deeper understanding of cellular behavior within 3D environments, 3D cell culture technology stands poised to revolutionize various aspects of healthcare and scientific discovery.
Introduction
Three-dimensional (3D) cell culture models aim to recreate natural environments outside the body, allowing cells to grow and interact in three dimensions (Haycock, 2011; Ravi et al., 2015; Gu et al., 2020; Yoon, 2023). By culturing various cell types within a 3D extracellular matrix, nutrients, oxygen, and drugs can efficiently reach cells, closely mimicking physiological conditions (Lee et al., 2019). Traditional two-dimensional (2D) cell culture systems, while convenient and cost-effective, lack the ability to accurately replicate the complex architecture and microenvironment found in living tissues (Duval et al., 2017). This limitation can lead to misleading results, particularly regarding the response of cancer cells to anticancer agents, as 2D cultures fail to mimic the true 3D tumor microenvironment (Gao et al., 2017; Brancato et al., 2020).
The emergence of 3D cell culture systems presents promising solutions to bridge the gap between laboratory cell cultures and in vivo conditions (Alghuwainem et al., 2019; Diaz-Rodriguez et al., 2019; Mofazzal Jahromi et al., 2019; Lin et al., 2020; Terrell et al., 2020). These systems offer higher physiological relevance, closely resembling cell behavior within the body, and provide new avenues for cell-based research and clinical trials (van Duinen et al., 2015; Bertucci et al., 2019; Calejo et al., 2019; Ham et al., 2019; Thelu et al., 2020). The intricacy inherent in 3D systems presents multifaceted challenges, notably in the selection of scaffold materials and cell types (Ashok et al., 2020). Researchers are confronted with the delicate decision between natural and synthetic scaffold materials, as well as the nuanced choice between utilizing autologous or adult-derived stem cells. Moreover, the meticulous fabrication of nanoscale scaffolds or the creation of microscale structures with precise architectures to support cell growth requires meticulous deliberation.
The dynamic nature of 3D cultures, moreover, introduces complexities in maintaining optimal culture environments over prolonged periods (Fernandes et al., 2020). Unlike static 2D cultures, 3D systems demand sophisticated strategies to facilitate nutrient diffusion, oxygenation, and waste removal throughout the entire structure. Innovations such as perfusion bioreactors and microfluidic systems have been devised to tackle these challenges, offering enhanced control over culture conditions, and ensuring the sustained viability of 3D constructs.
The integration of diverse cell types within 3D culture systems introduces yet another layer of intricacy (Ryu et al., 2019). Replicating the heterogeneous composition of tissues and organs in vivo often necessitates the co-cultivation of disparate cell populations to accurately simulate physiological interactions. This interdisciplinary endeavor mandates seamless collaboration among cell biologists, materials scientists, engineers, and clinicians to meticulously design and optimize 3D culture platforms tailored for specific applications, encompassing surgical research and regenerative medicine.
This review explores the current state of 3D culture in medicine and discusses future directions, such as standardization and simpler protocols, to facilitate broader adoption in surgical research. Addressing these challenges will be crucial for realizing the possibilities of 3D cell culture in medical applications and beyond.
Environments
Essential cultural platforms in biomedical research have been investigated particularly for drug discovery and anticancer investigations (Kim et al., 2020). The rising demand for advanced 3D cell culture models has intensified the need for sophisticated 3D cultivation techniques, from laboratory setups to industrial-scale production (Brancato et al., 2020; Wan et al., 2020). These bioreactors boast precise control systems, ensuring reproducible spheroid formation, a vital aspect for studying cellular behavior and drug responses. Beyond conventional applications, bioreactors play a crucial role in facilitating dynamic cell interactions and responses (Ferreira et al., 2018). They offer precise control over environmental conditions, including temperature, pH, and nutrient supply, ensuring optimal cell growth and function (Figure 1). Additionally, advancements in bioreactor design enable the integration of various monitoring and control systems for enhanced functionality.
Utilizing established 3D culture models, researchers have made significant strides in understanding cancer progression mechanisms (Dumont et al., 2019; Zhang et al., 2020). These models provide insights into morphological and cellular changes associated with disease progression, offering valuable platforms for drug screening and efficacy testing. Real-time monitoring of 3D cultures allows for the assessment of dynamic cellular behaviors, such as proliferation, migration, and interaction (De Leon et al., 2020). This capability is instrumental in studying complex phenomena like angiogenesis and tumor invasion, providing insights into disease mechanisms and potential therapeutic interventions.
Progression models enable the evaluation of treatment responses and the identification of novel therapeutic targets, contributing to advancements in precision medicine and personalized therapies (Xia et al., 2019). Furthermore, these models aid in deciphering intricate cellular signaling pathways involved in disease progression, paving the way for targeted interventions. Accurate measurement of structural changes in 3D cultures requires precise labeling techniques to track cell proliferation and migration over time (Zhang et al., 2019). Various labeling methods, such as fluorescent proteins and dyes, enable researchers to monitor multiple cell types simultaneously, facilitating comprehensive analyses of complex cellular interactions (Linsley et al., 2019).
3D constructs
Compared to traditional 2D cell cultures, 3D scaffolds provide a more realistic environment for cellular growth and interaction (Gupta et al., 2019). Crafted from diverse materials, these structures offer controlled porosity, permeability, surface chemistry, and mechanical properties, closely resembling the native extracellular matrix (ECM) that supports cellular organization in tissues (Bayir et al., 2019; Huang et al., 2020). This porous network not only supports cellular growth but also facilitates nutrient and oxygen diffusion, essential for maintaining cell viability and function (Terrell et al., 2020).
Fabricating these scaffolds entails various techniques, each tailored to achieve specific structural and mechanical properties (Ashok et al., 2020). Freeze-drying, for instance, utilizes sublimation to create a porous scaffold from a frozen solution, while electrospinning produces fibrous scaffolds through the application of electrostatic forces (Fan et al., 2019). The applications of 3D scaffolds are vast, spanning both tissue engineering and cell culture research. In tissue engineering, these scaffolds serve as a scaffold for the regeneration of damaged organs and tissues. By seeding scaffolds with appropriate cells and growth factors, researchers aim to facilitate tissue repair and functional restoration.
Beyond tissue regeneration, 3D scaffolds play a pivotal role in advancing our understanding of disease mechanisms and cell biology. By providing a more physiologically relevant environment, these scaffolds enable researchers to study cell-cell interactions, drug responses, and disease progression with greater accuracy. 3D scaffolds stand as indispensable tools in tissue engineering and cell culture for studying cellular behavior and advancing regenerative medicine.
Cells
Stem cells, particularly pluripotent stem cells (PSCs), have garnered immense interest due to their ability to generate various cell types and their potential in regenerative medicine (Costamagna et al., 2019; Turco and Moffett, 2019). They offer promising avenues for medical innovation, including drug discovery, cell therapy, and tissue regeneration (Artero Castro et al., 2019; Srivastava and Kilian, 2019). Utilizing advanced 3D cell platforms, researchers have made significant strides in understanding cell signaling and tissue development, particularly in fields.
Culturing cells in 3D models has emerged as a vital tool, providing results that closely mimic natural conditions and aiding in the translation of research findings into clinical applications (Alagarsamy et al., 2019; Balak et al., 2019; Chen and Schoen, 2019; Gibbs et al., 2019; Gopalakrishnan, 2019; Meivar-Levy and Ferber, 2019; Roberts et al., 2019; Nguyen et al., 2020; Raimondi et al., 2020). These models offer a more realistic representation of cellular behavior, ensuring better predictability when moving from the laboratory to real-world settings. Autologous, allogeneic, and xenogeneic cells are employed, with autologous cells preferred to mitigate the risk of rejection (Collins et al., 2020).
Adipose-derived stem cells (ASCs) have emerged as a significant player in regenerative medicine, offering several advantages over other sources such as bone marrow-derived mesenchymal stem cells (MSCs) (Ryu et al., 2019). ASCs, found within adipose tissue, boast higher yields and greater resistance to senescence, making them an attractive option for therapeutic applications (Seo et al., 2019). However, challenges persist in standardizing isolation methods and understanding their precise characteristics. The unique characteristics of ASCs vary depending on the tissue type and isolation method employed. Techniques like power-assisted liposuction have shown promising results in yielding high-quality ASCs, underscoring the importance of optimization in isolation protocols. Research continues to elucidate ASC behavior and identify specific markers to distinguish them from other cell types accurately.
Despite the progress made in harnessing the potential of stem cells and ASCs, several hurdles remain to be addressed. Standardization of isolation methods, precise differentiation instructions, and clarification of cell characteristics are crucial areas requiring further investigation. Nevertheless, the ongoing research holds significant promise for revolutionizing medical treatments and offering hope to patients with various degenerative diseases and injuries (Sthijns et al., 2019).
Medical applications
Transitioning from traditional 2D cell culture systems to more advanced 3D approaches represents a significant step towards understanding cellular behavior within a physiologically relevant context (Ndyabawe and Kisaalita, 2019; Jensen and Teng, 2020). 3D culture methods offer a more accurate representation of in vivo conditions, bridging the gap between conventional cell culture systems and the intricate physiology of living organisms (Figure 2). This advancement is particularly crucial in the medical research, where cellular interactions and the microenvironment play pivotal roles in tumorigenesis (Chaicharoenaudomrung et al., 2019).
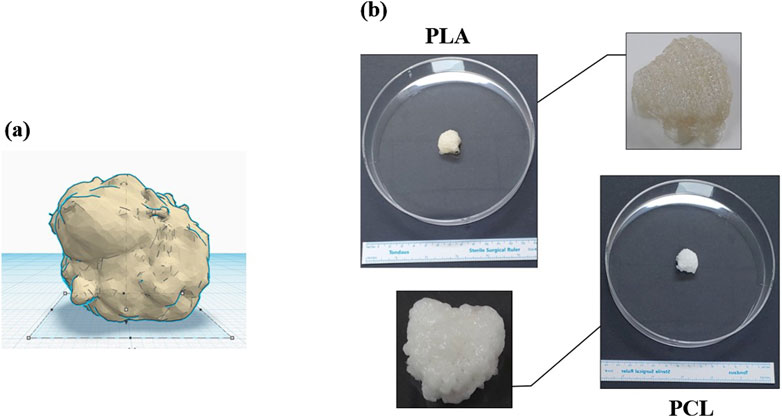
Figure 2. The pictures show: (A) a defect after oncologic surgery, and (B) a 3D bioprinted scaffold (PCL/PLA, MediFab Inc.) for reconstruction. PLA, polylactic acid; PCL, polycaprolactone.
These benefits in tissue engineering and regenerative medicine enable 3D culture to be considered for various clinical applications (Table 1). The previous studies showed the development of 3D bioprinted skin tissue, adipose microtissues, and bone scaffolds, demonstrating substantial improvements in cell viability, differentiation, and functionality (Gholipourmalekabadi et al., 2018; Yan et al., 2019; Yang et al., 2021; Li et al., 2024). Studies on esophageal, gastric, and intestinal organoids provide insights into stem cell potential and disease modeling, revealing the intricate processes of tissue development and regeneration (Spence et al., 2011; DeWard et al., 2014; McCracken et al., 2014). The generation of vascularized liver buds, lung organoids, and pancreatic organoids underscores the progress in creating functional human tissues for therapeutic applications, offering promising avenues for treating related diseases (Takebe et al., 2013; Dye et al., 2015; Boj et al., 2016). The researches on heart defect, prostate cancer, optic cup formation, inner ear sensory tissue, and lingual epithelium organoids emphasize the importance of organoid systems in understanding disease and developing personalized treatments. They suggested innovative approaches in heart patch, musculoskeletal tissue engineering, cartilage repair, and ophthalmopathy treatment, focusing on the potential of stem cell-derived tissues and hybrid scaffolds in regenerative medicine (Eiraku et al., 2011; Hisha et al., 2013; Gao et al., 2014; Koehler and Hashino, 2014; Fischer et al., 2023; Soucy et al., 2025). Some studies explore the possible usage of β -cell generated from human pluripotent stem cells to cure diabetes mellitus, the potential of salivary gland stem cell therapy for treating xerostomia, and the development of bioprinted human skin substitutes (Lee et al., 2014; Nanduri et al., 2014; Millman et al., 2016; Baltazar et al., 2023). The researches on liver organoids derived from primary human hepatocytes and the creation of hybrid scaffolds for musculoskeletal tissue demonstrate the ongoing efforts to enhance the complexity and functionality of engineered tissues (Salas-Silva et al., 2023; Enbergs et al., 2024).
One of the primary advantages of 3D culture over 2D systems is the preservation of critical extracellular matrix components and cell-cell or cell-matrix interactions (Ravi et al., 2015; Gonzalez Diaz et al., 2019; Tomas-Bort et al., 2020). These interactions are essential for various cellular processes, including differentiation, proliferation, and the expression of specific phenotypic traits. By maintaining these critical elements, 3D culture systems provide researchers with a more holistic view of cellular behavior, allowing for more accurate predictions of in vivo responses to various stimuli and treatments.
Studies have demonstrated that 3D tissue cultures can offer novel insights into tumorigenic mechanisms that may not be apparent in conventional 2D models (Liu et al., 2019; Roberts et al., 2019; Bahcecioglu et al., 2020; Zhang et al., 2020). The three-dimensional arrangement of cells in these cultures closely mimics the architecture of tumors in vivo, facilitating the study of complex processes such as invasion, metastasis, and drug resistance. Additionally, 3D culture systems enable the examination of dynamic cellular behaviors, such as migration and morphogenesis, which are challenging to replicate in 2D environments (Yamada and Sixt, 2019).
Moreover, 3D culture methods offer enhanced biomarker expression, providing researchers with valuable tools for studying cellular functions and interactions (Ravi et al., 2015). By accurately recapitulating the native cellular microenvironment, these systems enable the investigation of signaling pathways and regulatory mechanisms that are crucial for understanding disease progression and treatment response (Gonzalez Diaz et al., 2019). This improved biomarker expression also enhances the sensitivity and specificity of assays, leading to more reliable experimental results.
In addition to their utility in basic research, 3D culture systems hold promise for applications in drug development and personalized medicine (Linsley et al., 2019; Brancato et al., 2020; Fernandes et al., 2020; Fisher and Rao, 2020). These systems can serve as cost-effective screening platforms for identifying potential therapeutic agents and predicting their efficacy and safety profiles. By incorporating patient-derived cells into 3D cultures, researchers can tailor treatment strategies to individual patients, improving the likelihood of successful outcomes and minimizing adverse effects.
Overall, the transition from 2D to 3D cell culture represents a paradigm shift in biomedical research, offering researchers a more physiologically relevant model system for studying cellular behavior and disease mechanisms (Gopalakrishnan, 2019). By preserving critical cellular interactions and microenvironmental cues, 3D culture methods offer a more accurate representation of in vivo conditions. These technologies hold the potential to revolutionize medical fields, from cancer biology to regenerative medicine, paving the way for new diagnostic and therapeutic strategies.
Limitations
Limitations in 3D cell culture models present significant challenges both in terms of usability and standardization (Kim et al., 2020). Cultivating cells in three dimensions demands a certain level of expertise due to the necessity of forming cell aggregates, which complicates tasks such as exchanging culture medium and maintaining extracellular matrix integrity, leading to potential issues with cross-contamination during experimentation (Brancato et al., 2020). Addressing these challenges may necessitate the development of culture vessels that facilitate convenient medium exchange, thereby simplifying the experimental process (Gleave et al., 2020).
Standardization of analysis poses another significant challenge in the context of 3D cell culture models (Zhang et al., 2020). The three-dimensional nature of cell aggregates in 3D culture models introduces variability in the diffusion and penetration of these reagents (Czaplinska et al., 2019; Collins et al., 2020; Foglietta et al., 2020). Currently, common methods involve staining and measuring the size of cell aggregates, but these fail to accurately represent the activity of cells within these aggregates (Ashok et al., 2020). Efforts are underway to precisely measure cellular activity within aggregates using techniques such as tissue clearing or confocal microscopy (Ji et al., 2019; De Leon et al., 2020; Foglietta et al., 2020). However, standardization of these techniques remains elusive.
Continued research and development efforts are imperative to address these challenges and standardize the technology. Once these issues are resolved, 3D cell culture models, with their excellent biocompatibility, hold tremendous promise for applications in precision medicine, the pharmaceutical and biotechnology industries, and basic research. With further refinement and standardization, these models could revolutionize various fields by providing more physiologically relevant platforms for drug discovery, toxicity testing, disease modeling, and surgical application, ultimately leading to improved outcomes for patients (Ashraf et al., 2019).
Conclusion
3D cell culture models offer a promising approach to studying cell behavior in vitro, providing a more physiologically relevant environment compared to traditional 2D cultures. Despite the challenges, advancements in 3D cell culture technology hold great potential for revolutionizing medical research and clinical practice.
Author contributions
CP: Conceptualization, Funding acquisition, Writing–review and editing. JP: Funding acquisition, Writing–review and editing. YS: Conceptualization, Data curation, Formal Analysis, Writing–original draft.
Funding
The author(s) declare that financial support was received for the research, authorship, and/or publication of this article. This research was also supported by a grant of the Korea Health Technology R&D Project through the Korea Health Industry Development Institute (KHIDI), funded by the Ministry of Health and Welfare, Republic of Korea (Grant No. HI21C1847).
Acknowledgments
During the preparation of this work, the authors used the GPT-3.5 architecture (OpenAI, California, USA) to ensure natural language flow and nuances. After utilizing this service, the authors reviewed and edited the content as needed and take full responsibility for the publication’s content.
Conflict of interest
The authors declare that the research was conducted in the absence of any commercial or financial relationships that could be construed as a potential conflict of interest.
Publisher’s note
All claims expressed in this article are solely those of the authors and do not necessarily represent those of their affiliated organizations, or those of the publisher, the editors and the reviewers. Any product that may be evaluated in this article, or claim that may be made by its manufacturer, is not guaranteed or endorsed by the publisher.
References
Alagarsamy, K. N., Yan, W., Srivastava, A., Desiderio, V., and Dhingra, S. (2019). Application of injectable hydrogels for cardiac stem cell therapy and tissue engineering. Rev. Cardiovasc Med. 20 (4), 221–230. doi:10.31083/j.rcm.2019.04.534
Alghuwainem, A., Alshareeda, A. T., and Alsowayan, B. (2019). Scaffold-Free 3-D cell sheet technique bridges the gap between 2-D cell culture and animal models. Int. J. Mol. Sci. 20 (19), 4926. doi:10.3390/ijms20194926
Artero Castro, A., Rodriguez Jimenez, F. J., Jendelova, P., and Erceg, S. (2019). Deciphering retinal diseases through the generation of three dimensional stem cell-derived organoids: concise Review. Stem Cells 37 (12), 1496–1504. doi:10.1002/stem.3089
Ashok, A., Choudhury, D., Fang, Y., and Hunziker, W. (2020). Towards manufacturing of human organoids. Biotechnol. Adv. 39, 107460. doi:10.1016/j.biotechadv.2019.107460
Ashraf, M. N., Asghar, M. W., Rong, Y., Doschak, M. R., and Kiang, T. K. L. (2019). Advanced in vitro HepaRG culture systems for xenobiotic metabolism and toxicity characterization. Eur. J. Drug Metab. Pharmacokinet. 44 (4), 437–458. doi:10.1007/s13318-018-0533-3
Bahcecioglu, G., Basara, G., Ellis, B. W., Ren, X., and Zorlutuna, P. (2020). Breast cancer models: engineering the tumor microenvironment. Acta Biomater. 106, 1–21. doi:10.1016/j.actbio.2020.02.006
Balak, J. R. A., Juksar, J., Carlotti, F., Lo Nigro, A., and de Koning, E. J. P. (2019). Organoids from the human fetal and adult pancreas. Curr. Diab Rep. 19 (12), 160. doi:10.1007/s11892-019-1261-z
Baltazar, T., Jiang, B., Moncayo, A., Merola, J., Albanna, M. Z., Saltzman, W. M., et al. (2023). 3D bioprinting of an implantable xeno-free vascularized human skin graft. Bioeng. Transl. Med. 8 (1), e10324. doi:10.1002/btm2.10324
Bayir, E., Sendemir, A., and Missirlis, Y. F. (2019). Mechanobiology of cells and cell systems, such as organoids. Biophys. Rev. 11 (5), 721–728. doi:10.1007/s12551-019-00590-7
Bertucci, C., Koppes, R., Dumont, C., and Koppes, A. (2019). Neural responses to electrical stimulation in 2D and 3D in vitro environments. Brain Res. Bull. 152, 265–284. doi:10.1016/j.brainresbull.2019.07.016
Boj, S. F., Hwang, C. I., Baker, L. A., Engle, D. D., Tuveson, D. A., and Clevers, H. (2016). Model organoids provide new research opportunities for ductal pancreatic cancer. Mol. Cell Oncol. 3 (1), e1014757. doi:10.1080/23723556.2015.1014757
Brancato, V., Oliveira, J. M., Correlo, V. M., Reis, R. L., and Kundu, S. C. (2020). Could 3D models of cancer enhance drug screening? Biomaterials 232, 119744. doi:10.1016/j.biomaterials.2019.119744
Calejo, I., Costa-Almeida, R., Reis, R. L., and Gomes, M. E. (2019). Enthesis tissue engineering: biological requirements meet at the interface. Tissue Eng. Part B Rev. 25 (4), 330–356. doi:10.1089/ten.TEB.2018.0383
Chaicharoenaudomrung, N., Kunhorm, P., and Noisa, P. (2019). Three-dimensional cell culture systems as an in vitro platform for cancer and stem cell modeling. World J. Stem Cells 11 (12), 1065–1083. doi:10.4252/wjsc.v11.i12.1065
Chen, S., and Schoen, J. (2019). Air-liquid interface cell culture: from airway epithelium to the female reproductive tract. Reprod. Domest. Anim. 54 (Suppl. 3), 38–45. doi:10.1111/rda.13481
Collins, A., Miles, G. J., Wood, J., MacFarlane, M., Pritchard, C., and Moss, E. (2020). Patient-derived explants, xenografts and organoids: 3-dimensional patient-relevant pre-clinical models in endometrial cancer. Gynecol. Oncol. 156 (1), 251–259. doi:10.1016/j.ygyno.2019.11.020
Costamagna, G., Andreoli, L., Corti, S., and Faravelli, I. (2019). iPSCs-based neural 3D systems: a multidimensional approach for disease modeling and drug discovery. Cells 8 (11), 1438. doi:10.3390/cells8111438
Czaplinska, D., Elingaard-Larsen, L. O., Rolver, M. G., Severin, M., and Pedersen, S. F. (2019). 3D multicellular models to study the regulation and roles of acid-base transporters in breast cancer. Biochem. Soc. Trans. 47 (6), 1689–1700. doi:10.1042/BST20190131
De Leon, S. E., Pupovac, A., and McArthur, S. L. (2020). Three-Dimensional (3D) cell culture monitoring: opportunities and challenges for impedance spectroscopy. Biotechnol. Bioeng. 117 (4), 1230–1240. doi:10.1002/bit.27270
DeWard, A. D., Cramer, J., and Lagasse, E. (2014). Cellular heterogeneity in the mouse esophagus implicates the presence of a nonquiescent epithelial stem cell population. Cell Rep. 9 (2), 701–711. doi:10.1016/j.celrep.2014.09.027
Diaz-Rodriguez, P., Lopez-Alvarez, M., Serra, J., Gonzalez, P., and Landin, M. (2019). Current stage of marine ceramic grafts for 3D bone tissue regeneration. Mar. Drugs 17 (8), 471. doi:10.3390/md17080471
Dumont, S., Jan, Z., Heremans, R., Van Gorp, T., Vergote, I., and Timmerman, D. (2019). Organoids of epithelial ovarian cancer as an emerging preclinical in vitro tool: a review. J. Ovarian Res. 12 (1), 105. doi:10.1186/s13048-019-0577-2
Duval, K., Grover, H., Han, L. H., Mou, Y., Pegoraro, A. F., Fredberg, J., et al. (2017). Modeling physiological events in 2D vs. 3D cell culture. Physiol. (Bethesda) 32 (4), 266–277. doi:10.1152/physiol.00036.2016
Dye, B. R., Hill, D. R., Ferguson, M. A., Tsai, Y. H., Nagy, M. S., Dyal, R., et al. (2015). In vitro generation of human pluripotent stem cell derived lung organoids. Elife 4, e05098. doi:10.7554/eLife.05098
Eiraku, M., Takata, N., Ishibashi, H., Kawada, M., Sakakura, E., Okuda, S., et al. (2011). Self-organizing optic-cup morphogenesis in three-dimensional culture. Nature 472 (7341), 51–56. doi:10.1038/nature09941
Enbergs, S., Shopperly, L. K., Engels, A., Laue, D., Ertel, W., Sittinger, M., et al. (2024). Additively manufactured 3D clamp-culture system for the investigation of material-cell interactions in multi-material hybrid scaffolds for musculoskeletal tissue defects. J. Biomed. Mater Res. B Appl. Biomater. 112 (11), e35494. doi:10.1002/jbm.b.35494
Fan, D., Staufer, U., and Accardo, A. (2019). Engineered 3D polymer and hydrogel microenvironments for cell culture applications. Bioeng. (Basel) 6 (4), 113. doi:10.3390/bioengineering6040113
Fernandes, D. C., Canadas, R. F., Reis, R. L., and Oliveira, J. M. (2020). Dynamic culture systems and 3D interfaces models for cancer drugs testing. Adv. Exp. Med. Biol. 1230, 137–159. doi:10.1007/978-3-030-36588-2_9
Ferreira, L. P., Gaspar, V. M., and Mano, J. F. (2018). Design of spherically structured 3D in vitro tumor models -Advances and prospects. Acta Biomater. 75, 11–34. doi:10.1016/j.actbio.2018.05.034
Fischer, B., Gwinner, F., Gepp, M. M., Schulz, A., Danz, K., Dehne, A., et al. (2023). A highly versatile biopolymer-based platform for the maturation of human pluripotent stem cell-derived cardiomyocytes enables functional analysis in vitro and 3D printing of heart patches. J. Biomed. Mater Res. A 111 (10), 1600–1615. doi:10.1002/jbm.a.37558
Fisher, M. F., and Rao, S. S. (2020). Three-dimensional culture models to study drug resistance in breast cancer. Biotechnol. Bioeng. 117 (7), 2262–2278. doi:10.1002/bit.27356
Foglietta, F., Canaparo, R., Muccioli, G., Terreno, E., and Serpe, L. (2020). Methodological aspects and pharmacological applications of three-dimensional cancer cell cultures and organoids. Life Sci. 254, 117784. doi:10.1016/j.lfs.2020.117784
Gao, D., Vela, I., Sboner, A., Iaquinta, P. J., Karthaus, W. R., Gopalan, A., et al. (2014). Organoid cultures derived from patients with advanced prostate cancer. Cell 159 (1), 176–187. doi:10.1016/j.cell.2014.08.016
Gao, S., Shen, J., Hornicek, F., and Duan, Z. (2017). Three-dimensional (3D) culture in sarcoma research and the clinical significance. Biofabrication 9 (3), 032003. doi:10.1088/1758-5090/aa7fdb
Gholipourmalekabadi, M., Seifalian, A. M., Urbanska, A. M., Omrani, M. D., Hardy, J. G., Madjd, Z., et al. (2018). 3D protein-based bilayer artificial skin for the guided scarless healing of third-degree burn wounds in vivo. Biomacromolecules 19 (7), 2409–2422. doi:10.1021/acs.biomac.7b01807
Gibbs, S., Roffel, S., Meyer, M., and Gasser, A. (2019). Biology of soft tissue repair: gingival epithelium in wound healing and attachment to the tooth and abutment surface. Eur. Cell Mater 38, 63–78. doi:10.22203/eCM.v038a06
Gleave, A. M., Ci, X., Lin, D., and Wang, Y. (2020). A synopsis of prostate organoid methodologies, applications, and limitations. Prostate 80 (6), 518–526. doi:10.1002/pros.23966
Gonzalez Diaz, E. C., Sinha, S., Avedian, R. S., and Yang, F. (2019). Tissue-engineered 3D models for elucidating primary and metastatic bone cancer progression. Acta Biomater. 99, 18–32. doi:10.1016/j.actbio.2019.08.020
Gopalakrishnan, J. (2019). The emergence of stem cell-based brain organoids: trends and challenges. Bioessays 41 (8), e1900011. doi:10.1002/bies.201900011
Gu, Z., Guo, J., Wang, H., Wen, Y., and Gu, Q. (2020). Bioengineered microenvironment to culture early embryos. Cell Prolif. 53 (2), e12754. doi:10.1111/cpr.12754
Gupta, N., Renugopalakrishnan, V., Liepmann, D., Paulmurugan, R., and Malhotra, B. D. (2019). Cell-based biosensors: recent trends, challenges and future perspectives. Biosens. Bioelectron. 141, 111435. doi:10.1016/j.bios.2019.111435
Ham, J., Lever, L., Fox, M., and Reagan, M. R. (2019). In vitro 3D cultures to reproduce the bone marrow niche. JBMR Plus 3 (10), e10228. doi:10.1002/jbm4.10228
Haycock, J. W. (2011). 3D cell culture: a review of current approaches and techniques. Methods Mol. Biol. 695, 1–15. doi:10.1007/978-1-60761-984-0_1
Hisha, H., Tanaka, T., Kanno, S., Tokuyama, Y., Komai, Y., Ohe, S., et al. (2013). Establishment of a novel lingual organoid culture system: generation of organoids having mature keratinized epithelium from adult epithelial stem cells. Sci. Rep. 3, 3224. doi:10.1038/srep03224
Huang, L., Abdalla, A. M. E., Xiao, L., and Yang, G. (2020). Biopolymer-based microcarriers for three-dimensional cell culture and engineered tissue formation. Int. J. Mol. Sci. 21 (5), 1895. doi:10.3390/ijms21051895
Jensen, C., and Teng, Y. (2020). Is it time to start transitioning from 2D to 3D cell culture? Front. Mol. Biosci. 7, 33. doi:10.3389/fmolb.2020.00033
Ji, K., Sameni, M., Osuala, K., Moin, K., Mattingly, R. R., and Sloane, B. F. (2019). Spatio-temporal modeling and live-cell imaging of proteolysis in the 4D microenvironment of breast cancer. Cancer Metastasis Rev. 38 (3), 445–454. doi:10.1007/s10555-019-09810-8
Kim, J., Koo, B. K., and Knoblich, J. A. (2020). Human organoids: model systems for human biology and medicine. Nat. Rev. Mol. Cell Biol. 21, 571–584. doi:10.1038/s41580-020-0259-3
Koehler, K. R., and Hashino, E. (2014). 3D mouse embryonic stem cell culture for generating inner ear organoids. Nat. Protoc. 9 (6), 1229–1244. doi:10.1038/nprot.2014.100
Lee, J. H., Ho, K. L., and Fan, S. K. (2019). Liver microsystems in vitro for drug response. J. Biomed. Sci. 26 (1), 88. doi:10.1186/s12929-019-0575-0
Lee, V., Singh, G., Trasatti, J. P., Bjornsson, C., Xu, X., Tran, T. N., et al. (2014). Design and fabrication of human skin by three-dimensional bioprinting. Tissue Eng. Part C Methods 20 (6), 473–484. doi:10.1089/ten.TEC.2013.0335
Li, J., Chen, Y., Wei, M., Tang, Y., Zhou, L., Quan, X., et al. (2024). 3D printed sodium alginate/gelatin/tannic acid/calcium chloride scaffolds laden bone marrow mesenchymal stem cells to repair defective thyroid cartilage plate. J. Biomater. Appl., 8853282241300587. doi:10.1177/08853282241300587
Lin, A., Sved Skottvoll, F., Rayner, S., Pedersen-Bjergaard, S., Sullivan, G., Krauss, S., et al. (2020). 3D cell culture models and organ-on-a-chip: meet separation science and mass spectrometry. Electrophoresis 41 (1-2), 56–64. doi:10.1002/elps.201900170
Linsley, J. W., Reisine, T., and Finkbeiner, S. (2019). Cell death assays for neurodegenerative disease drug discovery. Expert Opin. Drug Discov. 14 (9), 901–913. doi:10.1080/17460441.2019.1623784
Liu, T., Yao, R., Pang, Y., and Sun, W. (2019). Review on biofabrication and applications of heterogeneous tumor models. J. Tissue Eng. Regen. Med. 13 (11), 2101–2120. doi:10.1002/term.2949
McCracken, K. W., Cata, E. M., Crawford, C. M., Sinagoga, K. L., Schumacher, M., Rockich, B. E., et al. (2014). Modelling human development and disease in pluripotent stem-cell-derived gastric organoids. Nature 516 (7531), 400–404. doi:10.1038/nature13863
Meivar-Levy, I., and Ferber, S. (2019). Liver to pancreas transdifferentiation. Curr. Diab Rep. 19 (9), 76. doi:10.1007/s11892-019-1198-2
Millman, J. R., Xie, C., Van Dervort, A., Gurtler, M., Pagliuca, F. W., and Melton, D. A. (2016). Generation of stem cell-derived β-cells from patients with type 1 diabetes. Nat. Commun. 7, 11463. doi:10.1038/ncomms11463
Mofazzal Jahromi, M. A., Abdoli, A., Rahmanian, M., Bardania, H., Bayandori, M., Moosavi Basri, S. M., et al. (2019). Microfluidic brain-on-a-chip: perspectives for mimicking neural system disorders. Mol. Neurobiol. 56 (12), 8489–8512. doi:10.1007/s12035-019-01653-2
Nanduri, L. S., Baanstra, M., Faber, H., Rocchi, C., Zwart, E., de Haan, G., et al. (2014). Purification and ex vivo expansion of fully functional salivary gland stem cells. Stem Cell Rep. 3 (6), 957–964. doi:10.1016/j.stemcr.2014.09.015
Ndyabawe, K., and Kisaalita, W. S. (2019). Engineering microsystems to recapitulate brain physiology on a chip. Drug Discov. Today 24 (9), 1725–1730. doi:10.1016/j.drudis.2019.06.008
Nguyen, R., Bae, S. D. W., Zhou, G., Read, S. A., Ahlenstiel, G., George, J., et al. (2020). Application of organoids in translational research of human diseases with a particular focus on gastrointestinal cancers. Biochim. Biophys. Acta Rev. Cancer 1873 (2), 188350. doi:10.1016/j.bbcan.2020.188350
Raimondi, M. T., Donnaloja, F., Barzaghini, B., Bocconi, A., Conci, C., Parodi, V., et al. (2020). Bioengineering tools to speed up the discovery and preclinical testing of vaccines for SARS-CoV-2 and therapeutic agents for COVID-19. Theranostics 10 (16), 7034–7052. doi:10.7150/thno.47406
Ravi, M., Paramesh, V., Kaviya, S. R., Anuradha, E., and Solomon, F. D. (2015). 3D cell culture systems: advantages and applications. J. Cell Physiol. 230 (1), 16–26. doi:10.1002/jcp.24683
Roberts, S., Peyman, S., and Speirs, V. (2019). Current and emerging 3D models to study breast cancer. Adv. Exp. Med. Biol. 1152, 413–427. doi:10.1007/978-3-030-20301-6_22
Ryu, N. E., Lee, S. H., and Park, H. (2019). Spheroid culture system methods and applications for mesenchymal stem cells. Cells 8 (12), 1620. doi:10.3390/cells8121620
Salas-Silva, S., Kim, Y., Kim, T. H., Kim, M., Seo, D., Choi, J., et al. (2023). Human chemically-derived hepatic progenitors (hCdHs) as a source of liver organoid generation: application in regenerative medicine, disease modeling, and toxicology testing. Biomaterials 303, 122360. doi:10.1016/j.biomaterials.2023.122360
Seo, Y., Shin, T. H., and Kim, H. S. (2019). Current strategies to enhance adipose stem cell function: an update. Int. J. Mol. Sci. 20 (15), 3827. doi:10.3390/ijms20153827
Soucy, J. R., Malechka, V. V., and Baranov, P. (2025). Retinal Ganglion cell transplantation: differentiation and quantification strategies. Methods Mol. Biol. 2858, 173–190. doi:10.1007/978-1-0716-4140-8_15
Spence, J. R., Mayhew, C. N., Rankin, S. A., Kuhar, M. F., Vallance, J. E., Tolle, K., et al. (2011). Directed differentiation of human pluripotent stem cells into intestinal tissue in vitro. Nature 470 (7332), 105–109. doi:10.1038/nature09691
Srivastava, P., and Kilian, K. A. (2019). Micro-engineered models of development using induced pluripotent stem cells. Front. Bioeng. Biotechnol. 7, 357. doi:10.3389/fbioe.2019.00357
Sthijns, M., LaPointe, V. L. S., and van Blitterswijk, C. A. (2019). Building complex life through self-organization. Tissue Eng. Part A 25 (19-20), 1341–1346. doi:10.1089/ten.TEA.2019.0208
Takebe, T., Sekine, K., Enomura, M., Koike, H., Kimura, M., Ogaeri, T., et al. (2013). Vascularized and functional human liver from an iPSC-derived organ bud transplant. Nature 499 (7459), 481–484. doi:10.1038/nature12271
Terrell, J. A., Jones, C. G., Kabandana, G. K. M., and Chen, C. (2020). From cells-on-a-chip to organs-on-a-chip: scaffolding materials for 3D cell culture in microfluidics. J. Mater Chem. B 8 (31), 6667–6685. doi:10.1039/d0tb00718h
Thelu, A., Catoire, S., and Kerdine-Romer, S. (2020). Immune-competent in vitro co-culture models as an approach for skin sensitisation assessment. Toxicol Vitro 62, 104691. doi:10.1016/j.tiv.2019.104691
Tomas-Bort, E., Kieler, M., Sharma, S., Candido, J. B., and Loessner, D. (2020). 3D approaches to model the tumor microenvironment of pancreatic cancer. Theranostics 10 (11), 5074–5089. doi:10.7150/thno.42441
Turco, M. Y., and Moffett, A. (2019). Development of the human placenta. Development 146 (22), dev163428. doi:10.1242/dev.163428
van Duinen, V., Trietsch, S. J., Joore, J., Vulto, P., and Hankemeier, T. (2015). Microfluidic 3D cell culture: from tools to tissue models. Curr. Opin. Biotechnol. 35, 118–126. doi:10.1016/j.copbio.2015.05.002
Wan, L., Neumann, C. A., and LeDuc, P. R. (2020). Tumor-on-a-chip for integrating a 3D tumor microenvironment: chemical and mechanical factors. Lab. Chip 20 (5), 873–888. doi:10.1039/c9lc00550a
Xia, X., Li, F., He, J., Aji, R., and Gao, D. (2019). Organoid technology in cancer precision medicine. Cancer Lett. 457, 20–27. doi:10.1016/j.canlet.2019.04.039
Yamada, K. M., and Sixt, M. (2019). Mechanisms of 3D cell migration. Nat. Rev. Mol. Cell Biol. 20 (12), 738–752. doi:10.1038/s41580-019-0172-9
Yan, Y., Chen, H., Zhang, H., Guo, C., Yang, K., Chen, K., et al. (2019). Vascularized 3D printed scaffolds for promoting bone regeneration. Biomaterials 190-191, 97–110. doi:10.1016/j.biomaterials.2018.10.033
Yang, F., Carmona, A., Stojkova, K., Garcia Huitron, E. I., Goddi, A., Bhushan, A., et al. (2021). A 3D human adipose tissue model within a microfluidic device. Lab. Chip 21 (2), 435–446. doi:10.1039/d0lc00981d
Yoon, J. K. (2023). Advanced 3D cell culture platform for tissue engineering. Tissue Eng. Regen. Med. 20 (4), 519–521. doi:10.1007/s13770-023-00562-9
Zhang, C., Yang, Z., Dong, D. L., Jang, T. S., Knowles, J. C., Kim, H. W., et al. (2020). 3D culture technologies of cancer stem cells: promising ex vivo tumor models. J. Tissue Eng. 11, 2041731420933407. doi:10.1177/2041731420933407
Keywords: 3D culture, stem cell, scaffold, biomaterial, bioprinting, medicine
Citation: Park CH, Park JH and Suh YJ (2024) Perspective of 3D culture in medicine: transforming disease research and therapeutic applications. Front. Bioeng. Biotechnol. 12:1491669. doi: 10.3389/fbioe.2024.1491669
Received: 05 September 2024; Accepted: 06 December 2024;
Published: 19 December 2024.
Edited by:
Mona Kamal Marei, Alexandria University, EgyptReviewed by:
Chen Yu Huang, National Cheng Kung University, TaiwanCopyright © 2024 Park, Park and Suh. This is an open-access article distributed under the terms of the Creative Commons Attribution License (CC BY). The use, distribution or reproduction in other forums is permitted, provided the original author(s) and the copyright owner(s) are credited and that the original publication in this journal is cited, in accordance with accepted academic practice. No use, distribution or reproduction is permitted which does not comply with these terms.
*Correspondence: Yong Joon Suh, bmljaXptQGdtYWlsLmNvbQ==