1 Introduction
Cryopreservation is a popular and crucial method for long-term storage of cells, tissues, and other biological samples at low temperatures. During this process, the cells are in a state of “suspended animation” to inhibit biological and chemical reactions (Pegg, 2015; Jang et al., 2017; Chang and Zhao, 2021). Recently, there are two main strategies of cryopreservation: slow freezing and vitrification (Kometas et al., 2021). Slow freezing refers to the freezing of biological samples at a rate of 1°C/min. This can be achieved through laboratory freezing tubes and programmed cooling boxes (Garcia-Flores et al., 2023). Vitrification means that when a small biological sample cools at a very fast rate, the internal water will be transformed into a glassy state (Schulz et al., 2020). The devices for vitrification are various, such as cyrotop (for oocytes) (Miao et al., 2022), plastic straw (for spermatids) (Patra et al., 2021), and cryomesh (for islets) (Zhan, Rao, et al., 2022). However, low temperature can cause a range of damage to biological samples, including protein denaturation (Chen et al., 2022), membrane damage (Lee et al., 2023), oxidative stress (Gualtieri et al., 2021). Since DMSO was first used in bull sperm cryopreservation in 1959, it has been found that the addition of a certain concentration of DMSO could resist these cryodamages (Lovelock and Bishop, 1959; Stubbs et al., 2020). Unfortunately, DMSO can lead to various problems such as differentiation of human stem cells (Davidson et al., 2015), hemolysis (Yi et al., 2017), and alterations in DNA methylation (Verheijen et al., 2019) at body temperature (37°C).Therefore, a series of novel CPAs, such as AFP, proline, etc., have been developed for DMSO-free cryopreservation (Li et al., 2020; Weng and Beauchesne, 2020), and these CPAs can be classified as permeable or impermeable according to whether they can enter cells (Weng et al., 2019; Yong et al., 2020). But none of them can replace DMSO totally. Currently, the most common cryopreservation process involves three steps: 1) mixing DMSO with biological samples and storing them at low temperature; 2) thawing by convection rewarming; and 3) removing DMSO by centrifugation and washing (Jang et al., 2017; Whaley et al., 2021). Although this protocol is widely used in clinics and laboratories, there are still some challenges.
Besides the toxicity of DMSO, commonly used convective rewarming can lead to ice recrystallization and devitrification because of its slow rewarming rates (Marquez-Curtis et al., 2015; Wang et al., 2016). Also, uneven temperature distribution and thermal gradients can induce thermal stress and destroy the biological samples, especially for larger volumes (Taylor et al., 2019). Finally, the manual centrifugation and washing to remove CPA is not only demanding for operators, but may also lead to complex procedures and unintended cell loss (Shu et al., 2014; Hornberger et al., 2019). In general, these procedures may cause damage instead of thoroughly cleaning (Fois et al., 2007).
To solve the problems discussed above, advanced cryopreservation technologies must be employed. Initially, impermeable CPA is widely used in cryopreserving biological samples such as oocytes and red blood cells due to its non-toxicity, high efficiency, and stability (Stoll et al., 2012; Zhang et al., 2016; Huang et al., 2017). However, its impermeability hinders its application in preventing intracellular damage. The use of delivery methods like nanoparticles (Rao et al., 2015) and membrane perturbation (Janis et al., 2021) are required to ensure its presence inside or outside the cells. In addition, novel warming methods such as nanowarming offers a faster and more even heating option compared to convective rewarming. It is especially important in cryopreserving large volume biological samples (Manuchehrabadi et al., 2017). Furthermore, high-quality washing methods have become effective way of convenient removal of CPA (Lusianti and Higgins, 2014; Zhao and Fu, 2017). Therefore, the adoption of these advanced cryopreservation technologies provides an opportunity to achieve efficient and high-quality cryopreservation (Figure 1).
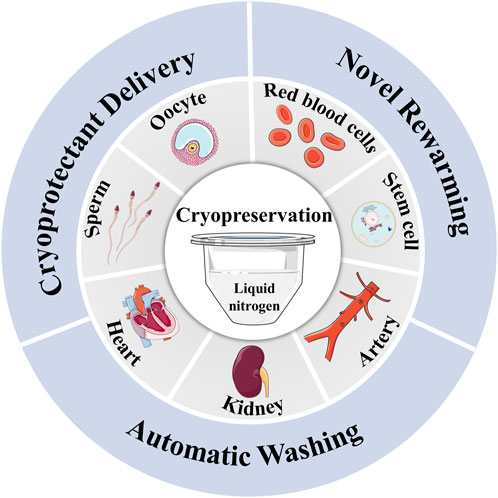
FIGURE 1. The novel delivery, warming and washing methods for cryopreservation of biological samples.
2 CPA delivery methods
On the one hand, trehalose has the ability to form hydrogen bonds with biomacromolecules and promote hydration, enabling cell components to maintain functional conformations. It can also slow metabolic activity by forming glassy substrates with extremely low molecular mobility (He, 2011; Ntai et al., 2018; Hu et al., 2023). On the other hand, AFP can enhance the resistance of cells to cryoinjury by inhibiting ice crystal growth and interaction with membranes (Kim et al., 2017; Baskaran et al., 2021). Due to these properties, trehalose and AFP have gained attention as non-toxic and reliable CPAs (Lee et al., 2013; Huang et al., 2017). However, unlike DMSO, trehalose and AFP cannot penetrate the cell membrane, which limits their use in protecting cells from intracellular ice crystal damage (Chang and Zhao, 2021; Hu et al., 2023). Therefore, effective methods for delivering trehalose and AFP are essential for successful cryopreservation. These methods include nanoparticles carriers and membrane perturbation delivery, depending on cellular structure and function. It must be noted that trehalose cannot be metabolized in cells and the safety of intracellular AFP is unclear, which may hinder their translation to clinic (Campbell and Brockbank, 2012; Dovgan et al., 2017). Detailed information has been summarized in Table 1.
2.1 Nanoparticles carriers
Endocytosis is one of the mechanisms by which nanoparticles (NPs) can deliver trehalose into cells (Stewart and He, 2019). For instance, cold-responsive nanocapsules (CR-NCs) encapsulated trehalose by microfluidics have successfully maintained the glucose-regulating function of pancreatic β cells after cryopreservation (Cheng et al., 2019). Some pH-responsive delivery systems, such as genipin-cross-linked Pluronic F127-chitosan nanoparticles (GNPs) (Rao et al., 2015) and chitosan-tripolyphosphate (CS-TPP) nanoparticles (Yao et al., 2020) have also shown efficient intracellular delivery of trehalose. Remarkably, Poly (l-alanine-co-l-lysine)-graft-trehalose (PAKT) was synthesized as a natural antifreezing glycopolypeptide (AFGP). It can be used as a carrier for trehalose delivery while also mimicking a CPA to inhibit ice recrystallization and protecting cells (Piao et al., 2022).
2.2 Membrane perturbation delivery
Membrane perturbation is another method to deliver impermeable CPAs into cells. The effectiveness of this approach has been demonstrated by the delivery of AFPIII via the location of cell-penetrating peptide pep-1 (Tomás et al., 2019). In non-endocytic human red blood cells, trehalose can be delivered by altering membrane permeability, which depends on the interaction between the polymer attached to the hydrophobic side group and the membrane lipid bilayer (Liu et al., 2022). Phenethylamine-grafted PGA (PGA-g-PEA) synthesized from hydrophobic PEA-modified PGA enhances trehalose loading capacity and reduce hemolysis of red blood cells by self-forming nanoparticles in a phosphate buffer solution (Zhang et al., 2020). Besides nanoparticles, ultrasound and microbubbles can also induce transient perforations to achieve trehalose loading into human red blood cells (Janis et al., 2021).
3 Warming methods
Convective rewarming, which means immersing biological samples in a water bath heated to 37°C, is still considered the gold standard for rewarming (Wolkers and Oldenhof, 2015). However, the slow heating rate resulting from convective rewarming can lead to ice recrystallization and devitrification (Wang et al., 2016). Additionally, convective rewarming may not provide even heating, particularly as the volume of biological samples increases. The resulting thermal gradients can cause biological samples to crack (Mfarrej et al., 2017; Sharma et al., 2021; Sharma et al., 2023). To overcome these limitations, a sequence of methods for rapid and even rewarming were developed, such as nanowarming, rapid joule heating (Zhan, Han, et al., 2022), infrared radiation heating (Bissoyi and Braslavsky, 2021). These methods have been summarized in Table 1.
3.1 Magnetoresponsive induction heating
Néel and Brownian relaxations caused by magnetic moment oscillation can induce the thermal effect of magnetic nanoparticle under an alternating magnetic field (AMF) (Syme et al., 2004; Cazares-Cortes et al., 2017). Therefore, the addition of magnetic nanoparticles to the cryoprotectant solution under an AMF can improve the thermal conductivity of biological samples, resulting in relatively even and rapid heating. This method minimizes damage to biological samples caused by slow and uneven rewarming (Etheridge et al., 2014; Liu et al., 2018). The vitrified organs, including rat hearts (Joshi et al., 2022) and rabbit kidneys (Sharma et al., 2021), have been successfully rewarmed utilizing magnetic iron oxide nanoparticles (IONPs), and the integrity of their structure and function is maintained. But the potential cytotoxicity of nanoparticles must be considered. However, due to the limitation of warming rate, the application of magnetically responsive nanoheating requires high molarity CPA, which brings potential toxicity to biological samples.
3.2 Photoresponsive induction heating
Gold nanorods and carbon black micron-sized particles have also been utilized in rewarming methods to achieve photoresponsive inducing heating (Khosla et al., 2020). Laser vibration in the gold nanoparticles induces heat dissipation. This enables ultra-rapid rewarming of cryopreserved zebrafish embryos and improves embryo survival. However, physical damage from injection site increased the probability of ice formation during rapid freezing (Khosla et al., 2019; Khosla et al., 2020). Carbon black micron-sized particles can suddenly heat up and emit heat after absorbing laser infrared energy. This hate will be transferred to the biological sample through the solution to achieve rapid and even heating (Panhwar et al., 2018).Nonetheless, photoresponsive nanoheating is difficult to apply to large-scale biological samples (Zhan, Han, et al., 2022).
4 Washing methods
Currently, the removal of DMSO from biological samples still relies on manual centrifugation, which requires skilled operators to remove the supernatant and replace it with a washing solution (Shu et al., 2014). However, cell loss is unavoidable during centrifugation, and residual DMSO can be highly toxic (Syme et al., 2004). Fortunately, several techniques for DMSO removal have been developed to address these challenges.
The hollow fiber module with semi-permeable membrane uses the pressure and concentration difference between the cell membrane and the fiber membrane to remove CPA from cells. This technique can also be scaled up for large cryopreserved cell suspensions (Ding et al., 2010). Dual-flow microfluidic devices have been specifically designed to remove intracellular DMSO in a limited time, which is essential for clinical applications (Fleming Glass et al., 2008). Dilution filtration system has been demonstrated to be more efficient and cost-effective than conventional multistep centrifugation or automated centrifugation (Zhou et al., 2011). Sepax-2 and Lovo devices have also been proven effective in removing DMSO from thawed hematopoietic progenitor cells (HPC), while maintaining the viability of CD34 cells before clinical infusion. However, the washing scheme must be flexible, convenient and low-cost for more common applications (Abonnenc et al., 2017; Mfarrej et al., 2017).
5 Conclusion
With the advancement of modern biotechnology, conventional cryopreservation obviously failed to keep pace with current needs. This review generalized the recent advances of delivery, warming and washing methods used in cryopreservation. Delivery methods helped to overcome the major limitation of the ultra-low permeability of impermeable CPAs, enabling their intracellular and extracellular cryopreservation. The use of novel warming methods can achieve rapid and even rewarming while avoiding the adverse effects of devitrification on biological samples. The emergence of various washing methods created a novel platform for convenient and efficient CPA removal. It must be noted that all the novel methods for cryopreservation have not been widely used neither in laboratory nor in clinic due to the high cost and complex operation protocol. Future studies need to focus on making new methods less difficult to perform without reducing their effectiveness, so that they can be applied by more researchers.
Author contributions
WZ: conceptualization and writing-original draft. XL: conceptualization and supervision. YH: investigation and software. ST: writing-review and editing, and supervision. All authors contributed to the article and approved the submitted version.
Acknowledgments
The Figure was partly generated using Servier Medical Art, provided by Servier, licensed under a Creative Commons Attribution 3.0 unported license.
Conflict of interest
The authors declare that the research was conducted in the absence of any commercial or financial relationships that could be construed as a potential conflict of interest.
Publisher’s note
All claims expressed in this article are solely those of the authors and do not necessarily represent those of their affiliated organizations, or those of the publisher, the editors and the reviewers. Any product that may be evaluated in this article, or claim that may be made by its manufacturer, is not guaranteed or endorsed by the publisher.
References
Abonnenc, M., Pesse, B., Tissot, J. D., Barelli, S., and Lion, N. (2017). Automatic washing of thawed haematopoietic progenitor cell grafts: A preclinical evaluation. Vox Sang. 112 (4), 367–378. doi:10.1111/vox.12503
Baskaran, A., Kaari, M., Venugopal, G., Manikkam, R., Joseph, J., and Bhaskar, P. V. (2021). Anti freeze proteins (Afp): Properties, sources and applications - a review. Int. J. Biol. Macromol. 189, 292–305. doi:10.1016/j.ijbiomac.2021.08.105
Bissoyi, A., and Braslavsky, I. (2021). Adherent cell thawing by infrared radiation. Cryobiology 103, 129–140. doi:10.1016/j.cryobiol.2021.08.002
Campbell, L. H., and Brockbank, K. G. (2012). Culturing with trehalose produces viable endothelial cells after cryopreservation. Cryobiology 64 (3), 240–244. doi:10.1016/j.cryobiol.2012.02.006
Cao, Y., Hassan, M., Cheng, Y., Chen, Z., Wang, M., Zhang, X., et al. (2019). Multifunctional photo- and magnetoresponsive graphene oxide-Fe(3)O(4) nanocomposite-alginate hydrogel platform for ice recrystallization inhibition. ACS Appl. Mater Interfaces 11 (13), 12379–12388. doi:10.1021/acsami.9b02887
Cazares-Cortes, E., Espinosa, A., Guigner, J. M., Michel, A., Griffete, N., Wilhelm, C., et al. (2017). Doxorubicin intracellular remote release from biocompatible oligo(ethylene glycol) methyl ether methacrylate-based magnetic nanogels triggered by magnetic hyperthermia. ACS Appl. Mater Interfaces 9 (31), 25775–25788. doi:10.1021/acsami.7b06553
Centner, C. S., Murphy, E. M., Priddy, M. C., Moore, J. T., Janis, B. R., Menze, M. A., et al. (2020). Ultrasound-induced molecular delivery to erythrocytes using a microfluidic system. Biomicrofluidics 14 (2), 024114. doi:10.1063/1.5144617
Chang, T., and Zhao, G. (2021). Ice inhibition for cryopreservation: Materials, strategies, and challenges. Adv. Sci. (Weinh). 8 (6), 2002425. doi:10.1002/advs.202002425
Chen, X., Wu, J., Li, X., Yang, F., Huang, D., Huang, J., et al. (2022). Snow flea antifreeze peptide for cryopreservation of lactic acid bacteria. NPJ Sci. Food 6 (1), 10. doi:10.1038/s41538-022-00128-4
Cheng, Y., Yu, Y., Zhang, Y., Zhao, G., and Zhao, Y. (2019). Cold-responsive nanocapsules enable the sole-cryoprotectant-trehalose cryopreservation of beta cell-laden hydrogels for diabetes treatment. Small 15 (50), e1904290. doi:10.1002/smll.201904290
Davidson, A. F., Glasscock, C., McClanahan, D. R., Benson, J. D., and Higgins, A. Z. (2015). Toxicity minimized cryoprotectant addition and removal procedures for adherent endothelial cells. PLoS One 10 (11), e0142828. doi:10.1371/journal.pone.0142828
Ding, W., Zhou, X., Heimfeld, S., Reems, J. A., and Gao, D. (2010). A steady-state mass transfer model of removing CPAs from cryopreserved blood with hollow fiber modules. J. Biomech. Eng. 132 (1), 011002. doi:10.1115/1.4000110
Dovgan, B., Barlic, A., Knezevic, M., and Miklavcic, D. (2017). Cryopreservation of human adipose-derived stem cells in combination with trehalose and reversible electroporation. J. Membr. Biol. 250 (1), 1–9. doi:10.1007/s00232-016-9916-z
Etheridge, M. L., Xu, Y., Rott, L., Choi, J., Glasmacher, B., and Bischof, J. C. (2014). RF heating of magnetic nanoparticles improves the thawing of cryopreserved biomaterials. Technology 2 (3), 229–242. doi:10.1142/s2339547814500204
Fleming Glass, K. K., Longmire, E. K., and Hubel, A. (2008). Optimization of a microfluidic device for diffusion-based extraction of dmso from a cell suspension. Int. J. Heat. Mass Transf. 51 (23-24), 5749–5757. doi:10.1016/j.ijheatmasstransfer.2008.04.018
Fois, E., Desmartin, M., Benhamida, S., Xavier, F., Vanneaux, V., Rea, D., et al. (2007). Recovery, viability and clinical toxicity of thawed and washed haematopoietic progenitor cells: Analysis of 952 autologous peripheral blood stem cell transplantations. Bone Marrow Transpl. 40 (9), 831–835. doi:10.1038/sj.bmt.1705830
Gao, S., Zhu, K., Zhang, Q., Niu, Q., Chong, J., Ren, L., et al. (2022). Development of icephilic ACTIVE glycopeptides for cryopreservation of human erythrocytes. Biomacromolecules 23 (2), 530–542. doi:10.1021/acs.biomac.1c01372
Garcia-Flores, V., Xu, Y., Pusod, E., Romero, R., Pique-Regi, R., and Gomez-Lopez, N. (2023). Preparation of single-cell suspensions from the human placenta. Nat. Protoc. 18 (3), 732–754. doi:10.1038/s41596-022-00772-w
Gualtieri, R., Kalthur, G., Barbato, V., Di Nardo, M., Adiga, S. K., and Talevi, R. (2021). Mitochondrial dysfunction and oxidative stress caused by cryopreservation in reproductive cells. Antioxidants (Basel) 10 (3), 337. doi:10.3390/antiox10030337
Han, Z., Sharma, A., Gao, Z., Carlson, T. W., O'Sullivan, M. G., Finger, E. B., et al. (2020). Diffusion limited cryopreservation of tissue with radiofrequency heated metal forms. Adv. Healthc. Mater 9 (19), e2000796. doi:10.1002/adhm.202000796
He, X. (2011). Thermostability of biological systems: Fundamentals, challenges, and quantification. Open Biomed. Eng. J. 5, 47–73. doi:10.2174/1874120701105010047
Hornberger, K., Yu, G., McKenna, D., and Hubel, A. (2019). Cryopreservation of hematopoietic stem cells: Emerging assays, cryoprotectant agents, and technology to improve outcomes. Transfus. Med. Hemotherapy 46 (3), 188–196. doi:10.1159/000496068
Hu, Y., Liu, X., Liu, F., Xie, J., Zhu, Q., and Tan, S. (2023). Trehalose in biomedical cryopreservation-properties, mechanisms, delivery methods, applications, benefits, and problems. ACS Biomater. Sci. Eng. 9 (3), 1190–1204. doi:10.1021/acsbiomaterials.2c01225
Huang, H., Zhao, G., Zhang, Y., Xu, J., Toth, T. L., and He, X. (2017). Predehydration and ice seeding in the presence of trehalose enable cell cryopreservation. ACS Biomater. Sci. Eng. 3 (8), 1758–1768. doi:10.1021/acsbiomaterials.7b00201
Jang, T. H., Park, S. C., Yang, J. H., Kim, J. Y., Seok, J. H., Park, U. S., et al. (2017). Cryopreservation and its clinical applications. Integr. Med. Res. 6 (1), 12–18. doi:10.1016/j.imr.2016.12.001
Janis, B. R., Priddy, M. C., Otto, M. R., Kopechek, J. A., and Menze, M. A. (2021). Sonoporation enables high-throughput loading of trehalose into red blood cells. Cryobiology 98, 73–79. doi:10.1016/j.cryobiol.2020.12.005
Joshi, P., Ehrlich, L. E., Gao, Z., Bischof, J. C., and Rabin, Y. (2022). Thermal analyses of nanowarming-assisted recovery of the heart from cryopreservation by vitrification. J. Heat. Transf. 144 (3), 031202. doi:10.1115/1.4053105
Khosla, K., Kangas, J., Liu, Y., Zhan, L., Daly, J., Hagedorn, M., et al. (2020). Cryopreservation and laser nanowarming of zebrafish embryos followed by hatching and spawning. Adv. Biosyst. 4 (11), e2000138. doi:10.1002/adbi.202000138
Khosla, K., Zhan, L., Bhati, A., Carley-Clopton, A., Hagedorn, M., and Bischof, J. (2019). Characterization of laser gold nanowarming: A platform for millimeter-scale cryopreservation. Langmuir 35 (23), 7364–7375. doi:10.1021/acs.langmuir.8b03011
Kim, H. J., Lee, J. H., Hur, Y. B., Lee, C. W., Park, S. H., and Koo, B. W. (2017). Marine antifreeze proteins: Structure, function, and application to cryopreservation as a potential cryoprotectant. Mar. Drugs 15 (2), 27. doi:10.3390/md15020027
Kometas, M., Christman, G. M., Kramer, J., and Rhoton-Vlasak, A. (2021). Methods of ovarian tissue cryopreservation: Is vitrification superior to slow freezing?-ovarian tissue freezing methods. Reprod. Sci. 28 (12), 3291–3302. doi:10.1007/s43032-021-00591-6
Lee, S., Kim, Y. M., Cheong, H. T., Park, C. K., and Lee, S. H. (2023). Effect of magnetized freezing extender on membrane damages, motility, and fertility of boar sperm following cryopreservation. Anim. (Basel) 13 (4), 634. doi:10.3390/ani13040634
Lee, Y. A., Kim, Y. H., Kim, B. J., Kim, B. G., Kim, K. J., Auh, J. H., et al. (2013). Cryopreservation in trehalose preserves functional capacity of murine spermatogonial stem cells. PLoS One 8 (1), e54889. doi:10.1371/journal.pone.0054889
Li, R., Hornberger, K., Dutton, J. R., and Hubel, A. (2020). Cryopreservation of human iPS cell aggregates in a DMSO-free solution-an optimization and comparative study. Front. Bioeng. Biotechnol. 8, 1. doi:10.3389/fbioe.2020.00001
Liu, X., Gao, S., Niu, Q., Zhu, K., Ren, L., and Yuan, X. (2022). Facilitating trehalose entry into hRBCs at 4 °C by alkylated ε-poly(l-lysine) for glycerol-free cryopreservation. J. Mater Chem. B 10 (7), 1042–1054. doi:10.1039/d1tb02674g
Liu, X., Zhao, G., Chen, Z., Panhwar, F., and He, X. (2018). Dual suppression effect of magnetic induction heating and microencapsulation on ice crystallization enables low-cryoprotectant vitrification of stem cell-alginate hydrogel constructs. ACS Appl. Mater Interfaces 10 (19), 16822–16835. doi:10.1021/acsami.8b04496
Lovelock, J. E., and Bishop, M. W. (1959). Prevention of freezing damage to living cells by dimethyl sulphoxide. Nature 183 (4672), 1394–1395. doi:10.1038/1831394a0
Lusianti, R. E., and Higgins, A. Z. (2014). Continuous removal of glycerol from frozen-thawed red blood cells in a microfluidic membrane device. Biomicrofluidics 8 (5), 054124. doi:10.1063/1.4900675
Manuchehrabadi, N., Gao, Z., Zhang, J., Ring, H. L., Shao, Q., Liu, F., et al. (2017). Improved tissue cryopreservation using inductive heating of magnetic nanoparticles. Sci. Transl. Med. 9 (379), eaah4586. doi:10.1126/scitranslmed.aah4586
Marquez-Curtis, L. A., Janowska-Wieczorek, A., McGann, L. E., and Elliott, J. A. (2015). Mesenchymal stromal cells derived from various tissues: Biological, clinical and cryopreservation aspects. Cryobiology 71 (2), 181–197. doi:10.1016/j.cryobiol.2015.07.003
Mfarrej, B., Bouchet, G., Couquiaud, J., Regimbaud, L., Binninger, S., Mercier, M., et al. (2017). Pre-clinical assessment of the Lovo device for dimethyl sulfoxide removal and cell concentration in thawed hematopoietic progenitor cell grafts. Cytotherapy 19 (12), 1501–1508. doi:10.1016/j.jcyt.2017.09.001
Miao, S., Guo, C., Jiang, Z., Wei, H. X., Jiang, X., Gu, J., et al. (2022). Development of an open microfluidic platform for oocyte one-stop vitrification with cryotop method. Biosens. (Basel). 12 (9), 766. doi:10.3390/bios12090766
Ntai, A., La Spada, A., De Blasio, P., and Biunno, I. (2018). Trehalose to cryopreserve human pluripotent stem cells. Stem Cell. Res. 31, 102–112. doi:10.1016/j.scr.2018.07.021
Panhwar, F., Chen, Z., Hossain, S. M. C., Wang, M., Haider, Z., Memon, K., et al. (2018). Near-infrared laser mediated modulation of ice crystallization by two-dimensional nanosheets enables high-survival recovery of biological cells from cryogenic temperatures. Nanoscale 10 (25), 11760–11774. doi:10.1039/c8nr01349g
Patra, T., Pathak, D., and Gupta, M. K. (2021). Strategies for cryopreservation of testicular cells and tissues in cancer and genetic diseases. Cell. Tissue Res. 385 (1), 1–19. doi:10.1007/s00441-021-03437-4
Pegg, D. E. (2015). “Principles of cryopreservation,” in Cryopreservation and freeze-drying protocols. Editors W. F. Wolkers, and H. Oldenhof (Berlin, Germany: Springer), 3–19.
Piao, Z., Patel, M., Park, J. K., and Jeong, B. (2022). Poly(l-alanine-co-l-lysine)-g-Trehalose as a biomimetic cryoprotectant for stem cells. Biomacromolecules 23 (5), 1995–2006. doi:10.1021/acs.biomac.1c01701
Rao, W., Huang, H., Wang, H., Zhao, S., Dumbleton, J., Zhao, G., et al. (2015). Nanoparticle-mediated intracellular delivery enables cryopreservation of human adipose-derived stem cells using trehalose as the sole cryoprotectant. ACS Appl. Mater Interfaces 7 (8), 5017–5028. doi:10.1021/acsami.5b00655
Schulz, M., Risopatron, J., Uribe, P., Isachenko, E., Isachenko, V., and Sanchez, R. (2020). Human sperm vitrification: A scientific report. Andrology 8 (6), 1642–1650. doi:10.1111/andr.12847
Sharma, A., Lee, C. Y., Namsrai, B. E., Han, Z., Tobolt, D., Rao, J. S., et al. (2023). Cryopreservation of whole rat livers by vitrification and nanowarming. Ann. Biomed. Eng. 51 (3), 566–577. doi:10.1007/s10439-022-03064-2
Sharma, A., Rao, J. S., Han, Z., Gangwar, L., Namsrai, B., Gao, Z., et al. (2021). Vitrification and nanowarming of kidneys. Adv. Sci. (Weinh). 8 (19), e2101691. doi:10.1002/advs.202101691
Shu, Z., Heimfeld, S., and Gao, D. (2014). Hematopoietic SCT with cryopreserved grafts: Adverse reactions after transplantation and cryoprotectant removal before infusion. Bone Marrow Transpl. 49 (4), 469–476. doi:10.1038/bmt.2013.152
Sreter, J. A., Foxall, T. L., and Varga, K. (2022). Intracellular and extracellular antifreeze protein significantly improves mammalian cell cryopreservation. Biomolecules 12 (5), 669. doi:10.3390/biom12050669
Stefanic, M., Ward, K., Tawfik, H., Seemann, R., Baulin, V., Guo, Y., et al. (2017). Apatite nanoparticles strongly improve red blood cell cryopreservation by mediating trehalose delivery via enhanced membrane permeation. Biomaterials 140, 138–149. doi:10.1016/j.biomaterials.2017.06.018
Stewart, S., and He, X. (2019). Intracellular delivery of trehalose for cell banking. Langmuir 35 (23), 7414–7422. doi:10.1021/acs.langmuir.8b02015
Stoll, C., Holovati, J. L., Acker, J. P., and Wolkers, W. F. (2012). Synergistic effects of liposomes, trehalose, and hydroxyethyl starch for cryopreservation of human erythrocytes. Biotechnol. Prog. 28 (2), 364–371. doi:10.1002/btpr.1519
Stubbs, C., Bailey, T. L., Murray, K., and Gibson, M. I. (2020). Polyampholytes as emerging macromolecular cryoprotectants. Biomacromolecules 21 (1), 7–17. doi:10.1021/acs.biomac.9b01053
Syme, R., Bewick, M., Stewart, D., Porter, K., Chadderton, T., and Gluck, S. (2004). The role of depletion of dimethyl sulfoxide before autografting: On hematologic recovery, side effects, and toxicity. Biol. Blood Marrow Transpl. 10 (2), 135–141. doi:10.1016/j.bbmt.2003.09.016
Taylor, M. J., Weegman, B. P., Baicu, S. C., and Giwa, S. E. (2019). New approaches to cryopreservation of cells, tissues, and organs. Transfus. Med. Hemother 46 (3), 197–215. doi:10.1159/000499453
Tomás, R. M. F., Bailey, T. L., Hasan, M., and Gibson, M. I. (2019). Extracellular antifreeze protein significantly enhances the cryopreservation of cell monolayers. Biomacromolecules 20 (10), 3864–3872. doi:10.1021/acs.biomac.9b00951
Verheijen, M., Lienhard, M., Schrooders, Y., Clayton, O., Nudischer, R., Boerno, S., et al. (2019). DMSO induces drastic changes in human cellular processes and epigenetic landscape in vitro. Sci. Rep. 9 (1), 4641. doi:10.1038/s41598-019-40660-0
Wang, J., Zhao, G., Zhang, Z., Xu, X., and He, X. (2016). Magnetic induction heating of superparamagnetic nanoparticles during rewarming augments the recovery of hUCM-MSCs cryopreserved by vitrification. Acta Biomater. 33, 264–274. doi:10.1016/j.actbio.2016.01.026
Weng, L., and Beauchesne, P. R. (2020). Dimethyl sulfoxide-free cryopreservation for cell therapy: A review. Cryobiology 94, 9–17. doi:10.1016/j.cryobiol.2020.03.012
Weng, L., Stott, S. L., and Toner, M. (2019). Exploring dynamics and structure of biomolecules, cryoprotectants, and water using molecular dynamics simulations: Implications for biostabilization and biopreservation. Annu. Rev. Biomed. Eng. 21, 1–31. doi:10.1146/annurev-bioeng-060418-052130
Whaley, D., Damyar, K., Witek, R. P., Mendoza, A., Alexander, M., and Lakey, J. R. (2021). Cryopreservation: An overview of principles and cell-specific considerations. Cell. Transpl. 30, 096368972199961. doi:10.1177/0963689721999617
Wolkers, W. F., and Oldenhof, H. (2015). Cryopreservation and freeze-drying protocols. Berlin, Germany: Springer.
Yao, X., Jovevski, J. J., Todd, M. F., Xu, R., Li, Y., Wang, J., et al. (2020). Nanoparticle-Mediated intracellular protection of natural killer cells avoids cryoinjury and retains potent antitumor functions. Adv. Sci. (Weinh). 7 (9), 1902938. doi:10.1002/advs.201902938
Yi, X., Liu, M., Luo, Q., Zhuo, H., Cao, H., Wang, J., et al. (2017). Toxic effects of dimethyl sulfoxide on red blood cells, platelets, and vascular endothelial cells in vitro. FEBS Open Bio 7 (4), 485–494. doi:10.1002/2211-5463.12193
Yong, K. W., Laouar, L., Elliott, J. A. W., and Jomha, N. M. (2020). Review of non-permeating cryoprotectants as supplements for vitrification of mammalian tissues. Cryobiology 96, 1–11. doi:10.1016/j.cryobiol.2020.08.012
Zhan, L., Han, Z., Shao, Q., Etheridge, M. L., Hays, T., and Bischof, J. C. (2022a). Rapid joule heating improves vitrification based cryopreservation. Nat. Commun. 13 (1), 6017. doi:10.1038/s41467-022-33546-9
Zhan, L., Rao, J. S., Sethia, N., Slama, M. Q., Han, Z., Tobolt, D., et al. (2022b). Pancreatic islet cryopreservation by vitrification achieves high viability, function, recovery and clinical scalability for transplantation. Nat. Med. 28 (4), 798–808. doi:10.1038/s41591-022-01718-1
Zhang, M., Oldenhof, H., Sieme, H., and Wolkers, W. F. (2016). Freezing-induced uptake of trehalose into mammalian cells facilitates cryopreservation. Biochim. Biophys. Acta 1858 (6), 1400–1409. doi:10.1016/j.bbamem.2016.03.020
Zhang, Q., Liu, B., Chong, J., Ren, L., Zhao, Y., and Yuan, X. (2020). Combination of hydrophobically modified γ-poly(glutamic acid) and trehalose achieving high cryosurvival of RBCs. Sci. China Technol. Sci. 64 (4), 806–816. doi:10.1007/s11431-020-1549-2
Zhang, Y., Wang, H., Stewart, S., Jiang, B., Ou, W., Zhao, G., et al. (2019). Cold-responsive nanoparticle enables intracellular delivery and rapid release of trehalose for organic-solvent-free cryopreservation. Nano Lett. 19 (12), 9051–9061. doi:10.1021/acs.nanolett.9b04109
Zhao, G., and Fu, J. (2017). Microfluidics for cryopreservation. Biotechnol. Adv. 35 (2), 323–336. doi:10.1016/j.biotechadv.2017.01.006
Keywords: cryopreservation, DMSO, cryoprotectant delivery, novel warming, automatic washing
Citation: Zhang W, Liu X, Hu Y and Tan S (2023) Incorporate delivery, warming and washing methods into efficient cryopreservation. Front. Bioeng. Biotechnol. 11:1215591. doi: 10.3389/fbioe.2023.1215591
Received: 02 May 2023; Accepted: 08 June 2023;
Published: 15 June 2023.
Edited by:
Qingxin Mu, University of Washington, United StatesReviewed by:
Li Zhan, Massachusetts General Hospital and Harvard Medical School, United StatesXiaoyan Yuan, Tianjin University, China
Copyright © 2023 Zhang, Liu, Hu and Tan. This is an open-access article distributed under the terms of the Creative Commons Attribution License (CC BY). The use, distribution or reproduction in other forums is permitted, provided the original author(s) and the copyright owner(s) are credited and that the original publication in this journal is cited, in accordance with accepted academic practice. No use, distribution or reproduction is permitted which does not comply with these terms.
*Correspondence: Songwen Tan, c29uZ3dlbi50YW5AY3N1LmVkdS5jbg==
†These authors have contributed equally to this work and share first authorship