- 1Katz Department of Oral and Maxillofacial Surgery, The University of Texas Health Science Center at Houston, School of Dentistry, Houston, TX, United States
- 2Department of Chemistry and Department of Bioengineering, Rice University, Houston, TX, United States
Self-assembling peptides are a type of biomaterial rapidly emerging in the fields of biomedicine and material sciences due to their promise in biocompatibility and effectiveness at controlled release. These self-assembling peptides can form diverse nanostructures in response to molecular interactions, making them versatile materials. Once assembled, the peptides can mimic biological functions and provide a combinatorial delivery of therapeutics such as cytokines and drugs. These self-assembling peptides are showing success in biomedical settings yet face unique challenges that must be addressed to be widely applied in the clinic. Herein, we describe self-assembling peptides’ characteristics and current applications in immunomodulatory therapeutics.
1 Introduction
An influx of attention has been drawn to self-assembling peptides as the organization of these nanostructures has made it possible to interact with biological systems and induce bioactivity necessary for applications in tissue engineering, cancer immunotherapy, drug delivery, and vaccine design. Mainly, in tissue engineering, it was common to use scaffolds derived from animal or plant sources such as collagen, chitosan, and alginate (Loo et al., 2015; Peng et al., 2022). These scaffolds can potentially induce immunogenicity (Berthiaume et al., 2011; Loo et al., 2015; Andorko and Jewell, 2017). Self-assembling peptides (SAPs) can mimic tissues’ natural biomechanics and structure and the extracellular matrix (ECM). Besides the self-assembling peptides’ natural framework, SAPs are tunable to include biologically active systems. The adaptable nature of these peptides allows for loading a wide range of modulators that can be modified through their chemical composition to stabilize the scaffold, control release, and influence biological activity (Hoffman, 2002; Habibi et al., 2016; Sankar et al., 2021). SAPs are attractive biomaterials that can deliver immunomodulators known to have rapid clearance and poor metabolic stability (Lee et al., 2019; La Manna et al., 2021). The delivery site of these modulators can range from intracellular to distant targeted tissues. SAPs’ potential in tissue engineering, drug delivery, and immunotherapy can change the way we design and deliver biologics.
Many SAPs form networks of nanofibers that generate hydrogels at low weight percent. Some of the SAP hydrogels have shear-thinning and self-healing properties, which broadens the scope of using them as a biomaterial. Shear-thinning is a material’s ability to decrease viscosity under shear strain and self-heal when the strain is removed (Diesendruck et al., 2015; Chen et al., 2018a). Hydrogels with shear-thinning and self-healing properties are attractive biomaterials because they can be injected for minimally-invasive delivery (Herbst et al., 2013; Chen and Zou, 2019). Upon injection, the hydrogel can recover to the shape of the local environment, an important property for scaffolds in tissue engineering (Overstreet et al., 2012; Uman et al., 2020). These hydrogels experience improved material retention and mechanical properties that allow homogenous sequestration of modulators, making them ideal for the controlled release of small molecules for drug delivery (Lopez-Silva et al., 2019; Chang and Yan, 2020; Han et al., 2020; Uman et al., 2020). Maintaining biomaterial integrity is vital to restore vasculature and bone (Davison et al., 2014; Williams, 2019). Over time, the material can erode, but the early roots of regeneration are necessary to build the foundation of tissue growth (Berthiaume et al., 2011; O'Brien, 2011). Similarly, controlled material degradation is also required to ensure the release of immunomodulators for drug delivery and immunotherapy applications (Langer et al., 1990; Zhang et al., 2018). This review will cover self-assembling peptides’ unique contribution to biological activity and as delivery vehicles (Figure 1). SAPs will be discussed as small molecule mimickers and binding domains that contribute to host response and as delivery vehicles for immunomodulators such as antibodies and cytokines. The mechanism of peptide assembly and their chemical modifications have been recently reviewed in great detail and will not be discussed here (Lee et al., 2019; La Manna et al., 2021; Sinha et al., 2021; Li et al., 2022).
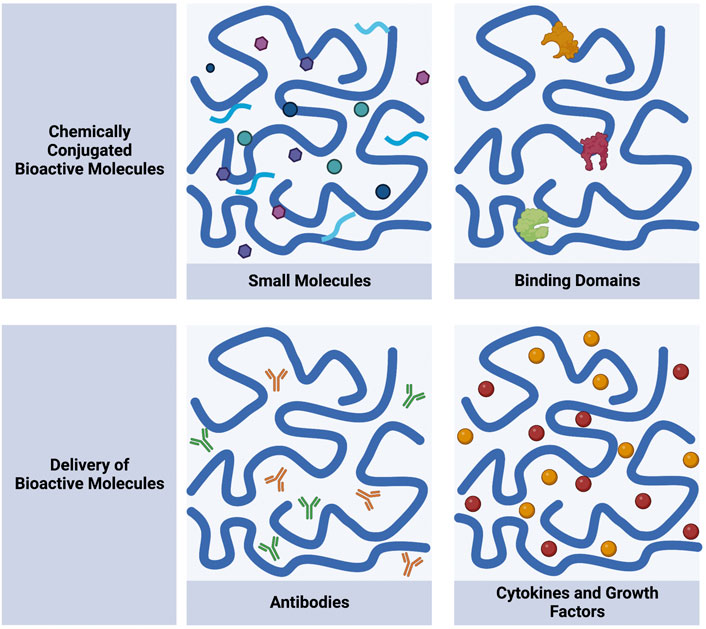
FIGURE 1. Self-assembling peptides can be designed to chemically conjugate and deliver bioactive molecules. Chemically conjugated molecules include small molecules and binding domains. Delivery of molecules includes antibodies and cytokines and growth factors. Schematic was made using Biorender.com.
2 Designing self-assembling peptides for immunomodulation
Self-assembling peptides are a class of peptides in which numerous copies interact with one another through a variety of non-covalent interactions to generate specific nano- and micro-structures in a fashion reminiscent of Lego Blocks. Numerous SAP “building blocks” have been described, each with its own unique characteristics and design criteria for self-assembly into nanostructures which have been reviewed in-depth (Lee et al., 2019; La Manna et al., 2021). A large number of SAPs are being explored for their potential in biomedical applications. In particular, MultiDomain Peptides (MDPs), Self-Assembling Amphiphilic Peptide Drug Conjugate (SAAPDC), RADARADARADARADA (RADA16), KLDLKLDLKLDL (KLD-12), SSGGPLGVRGKLVFFCAWSATWSNYWRH (LMY1), QAR-ILEADAEILRAYARILEAHAEILRAD (Coil-29), AEAEAKAKAEAEAKAK (EAK16-II), and QQKFQFQFEQQ (Q11) with their respective current immunotherapy strategies will be reviewed (Table 1) (Tripathi et al., 2015; Ding et al., 2016; Moore and Hartgerink, 2017; Mora-Solano et al., 2017; Ji et al., 2018; Wang et al., 2019; Lv et al., 2022; Wu et al., 2022).
As an example, MDPs are a class of SAP that form nanofibers consisting of a bilayer of β-sheet hydrogen bonded peptides. This organization is facilitated due to the amphiphilic nature of the MDP with hydrophilic amino acids facing the aqueous solution and hydrophobic amino acids facing the interior of the fiber bilayer. In this arrangement, the peptides form hydrogen bonds down the length of the fiber to further stabilize the assembly. Charged residues at the peptide’s termini are frequently designed to be negatively or positively charged, and this charge can be used to control assembly.
Recently four different charged MDPs were compared in vivo for their reaction with the host immune system (Moore et al., 2018; Lopez-Silva et al., 2020). In a murine subcutaneous injection model, the positively charged lysine-based MDP, K2(SL)6K2 (K2 MDP), and arginine-based MDP, R2(SL)6R2 (R2 MDP) promoted an inflammatory response compared to the negatively-charged E2(SL)6E2 (E2 MDP) and D2(SL)6D2 (D2 MDP). Through histological analysis, both amine groups K2 MDP and R2 MDP saw infiltration by host cells. K2 MDP was homogenously infiltrated by host cells, while R2 MDP had heterogeneous infiltration with a higher total number of cells and distinct cell pools. The SAPs with carboxylate side chains, E2 MDP and D2 MDP, had less infiltration. From early time points in flow cytometry and tSNE evaluations, K2 MDP had the presence of monocytes and macrophages, which resolved over time, indicating an acute inflammatory response. Meanwhile, R2 MDP had more polymorphonuclear myeloid-derived suppressor cells and, over time, did not resolve, implying chronic inflammatory reactions. These results provide a greater understanding of developing SAPs for particular purposes. In the case of tissue regeneration, K2 MDP would be a better SAP option as, over time, the material degrades, and inflammation declines. R2 MDP would be advantageous in cancer immunotherapy because having localized inflammation in the tumor immune microenvironment stimulates antitumor responses from macrophages that produce inflammatory cytokines like interleukin-1β (IL-1β), IL-6, and tumor necrosis factor-α (TNF-α) and lymphocytes such as natural killer cells, T cells, and B cells that induce cytotoxic activity (Chen et al., 2018b). E2 MDP and D2 MDP can be helpful in cases where the material does not provoke an immune response towards transplanted therapies such as mouse pancreatic-islet-derived cells for the treatment of diabetes (Su et al., 2010; Griffith et al., 2016). Thus, when developing SAPs, a significant consideration should be the hydrogel’s amino acid sequence to achieve the desired immune response.
SAPs can be chemically conjugated with small molecules and biologic recognition motifs to enhance cellular uptake (Hoffman, 2002; Wu et al., 2012; Fan et al., 2017; Levin et al., 2020). The alteration of the peptide primary sequence can include short bioactive amino acid sequences capable of initiating a biological response in the termini or, in some cases, in the middle of the sequence of SAPs. Cells can adhere to the nanostructures since most SAPs resemble the ECM. Cell adhesion-promoting bioactive amino acid sequences such as RGD can stimulate cell adherence (Cui et al., 2010). SAPs should be customized to the application and responsive to the environment. For instance, in bone defect repair, the rate of material degradation to the rate of bone regeneration is essential for developing new bone tissue (Dumas et al., 2014; Wei et al., 2020). SAPs can be remodeled by integrating sites in the material which are cleavable by enzymes such as matrix metalloproteinases-2 (MMP-2). These cleavable sites will allow healthy tissue to break down the material to make way for new tissue formation. In cancer applications, to prevent degradation of the ECM and metastasis, MMP-2 can be inhibited (Ji et al., 2018). SAPs can bind to cell surface receptors to activate or inhibit their signal. SAPs can include angiogenic cell surface receptor agonists to promote tissue development, such as VEGF mimic sequence (Siddiqui et al., 2021). Additionally, anti-angiogenic domains can prevent neovascularization (Nguyen et al., 2018). The signal is contained and localized in the gel by incorporating small molecules and biologic recognition domains in the hydrogel.
SAPs can load bioactive molecules for local delivery. During hydrogel formation, the SAPs can include the substances of interest. For instance, molecules such as drugs and cytokines can diffuse out of the gel. The rate at which the molecule diffuses out depends on the molecule’s identity and is not yet fully understood (Moore and Hartgerink, 2017). SAPs can load different polar molecules. For example, hydrophobic molecules are sequestered in the hydrophobic core of the SAP, while hydrophilic molecules are in the hydrophilic regions of the SAP. The ability to modify peptides and allow for the natural assembling process makes SAPs appealing for nanomaterial production.
3 Self-assembling peptide mimicking biological functions
Self-assembling peptides’ nanostructures resemble the ECM and can modify their peptide sequence for broad applications. Bioactive amino acid sequences, cleavable sites, and receptor agonists in SAPs can modulate the immune system (Hoffman, 2002; Wu et al., 2012; Fan et al., 2017). SAPs allow the signal to be contained and localized in the gel by incorporating small molecules and biologic recognition domains.
3.1 Small molecules
Engineered self-assembling peptides can deliver small molecules to targeted sites. Typically, small molecules are low in molecular weight and capable of modulating biochemical processes for diagnosis, treatment, or disease prevention (Lu and Atala, 2014). Most small molecules can cross the cell membrane allowing them to target intracellular proteins, and can be produced in large quantities, making them appealing for large-scale manufacture. However, most small molecules do not have targeting capability making it difficult to avoid off-target effects and maintain stability (Kidane and Bhatt, 2005; Gurevich and Gurevich, 2014). Researchers overcome this barrier by integrating small molecule mimics as a functional group in SAPs.
When designing biomaterials for biomedical applications, it is critical to tailor them to the disease. In the case of cancer, for example, there is an upregulation of inducible nitric oxide synthase (iNOS) in the tumor immune microenvironment (Jenkins et al., 1995; Zhang and Xu, 2001). Some researchers have used a small molecule inhibitor, N6-(1-iminoethyl)-L-lysine (L-NIL), to target iNOS to achieve tumor regression (Jenkins et al., 1995; Zhang and Xu, 2001; Fukumura et al., 2006; Jayaraman et al., 2012). An MDP with L-NIL-like functionality, KLNIL2(SL)6KLNIL2 (L-NIL-MDP), was developed to mimic a small-molecule inhibitor with immunomodulatory properties (Figure 2). L-NIL-MDP in vitro inhibited iNOS activity and nitrite production in cell populations interacting with the hydrogel material (Leach et al., 2019a). From a single subcutaneous injection of L-NIL-MDP, immunohistochemistry showed L-NIL maintained low nitrotyrosine levels in and around the implant over 7 days.
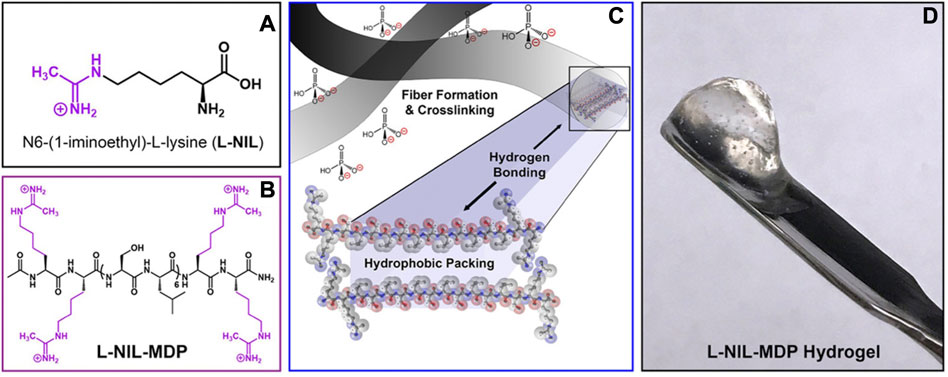
FIGURE 2. Self-assembling peptide chemical structure of N6-(1-iminoethyl)-L-lysine (A) Design of L-NIL-MDP. (B) Lysine side chains from starting material, K2(SL)6K2, converted to L-NIL functional group. (C) Schematic demonstrating the nanofibers formed from MDPs into antiparallel β-sheets. (D) Image of L-NIL-MDP formed at 1 wt% in a phosphate-containing buffer. Reprinted with permission from Leach et al. (2019b). Copyright 2019 American Chemical Society.
Additionally, the modified L-NIL-MDP with inherent bioactivity can be synergistically combined with a controlled released small molecule, cyclic dinucleotide (CDN). CDNs can induce antitumor responses in preclinical models through the Stimulator of Interferon Genes (STING) pathway (Corrales et al., 2015; Leach et al., 2018). The STING pathway links the detection of cytosolic tumor DNA through the enzyme cyclic GMP-AMP synthase, which activates STING resulting in the upregulation of type I interferons (Leach et al., 2019b; Jiang et al., 2020; Decout et al., 2021). This signaling allows for the crosstalk between tumor and immune cells, which promotes antitumor responses (Jiang et al., 2020). Clinical trials have evaluated intratumoral injections of CDN as a monotherapy, such as ADU-S1007 and MK14548 (ClinicalTrials.gov: NCT03172936, NCT03010176) and a modified CDN that forms a macrocycle-bridged STING agonist (Kim et al., 2021). These efforts may not be sufficient to localize the delivery of these small molecules. L-NIL-MDP was used to sequester CDN for controlled administration (Leach et al., 2021). Tumor-bearing mice treated with the L-NIL-MDP + CDN group showed decreased tumor growth compared with K2 MDP + CDN treatment. Survival increased with the L-NIL-MDP + CDN group compared to a saline control, L-NIL-MDP alone, and K2 MDP alone. Currently, the mechanism of action for L-NIL-MDP is being investigated.
Other SAPs have also been used for promoting antitumor activity. An SAAPDC was created containing amphiphilic peptide drug conjugate from oligomeric peptides (KGFRWR) and MMP-2 inhibitor doxorubicin (DOX) to treat hepatocellular carcinoma (Figure 3). DOX-KGFRWR strengthened the inhibition of MMP-2 activity and sustained the release of DOX in the DOX-KGFRWR group compared to DOX alone (Ji et al., 2018). The in vivo antitumor efficacy studies showed prolonged survival and therapeutic efficacy in the DOX-KGFRWR group. DOX-KGFRWR was able to control the release and retention of DOX without inducing severe systemic toxicity. The hexapeptide-based supramolecular system illustrated local and controlled drug release profiles. Researchers should explore the combination of embedding functional motifs and sequestering small molecules into SAPs in cases where synergy is required to improve response.
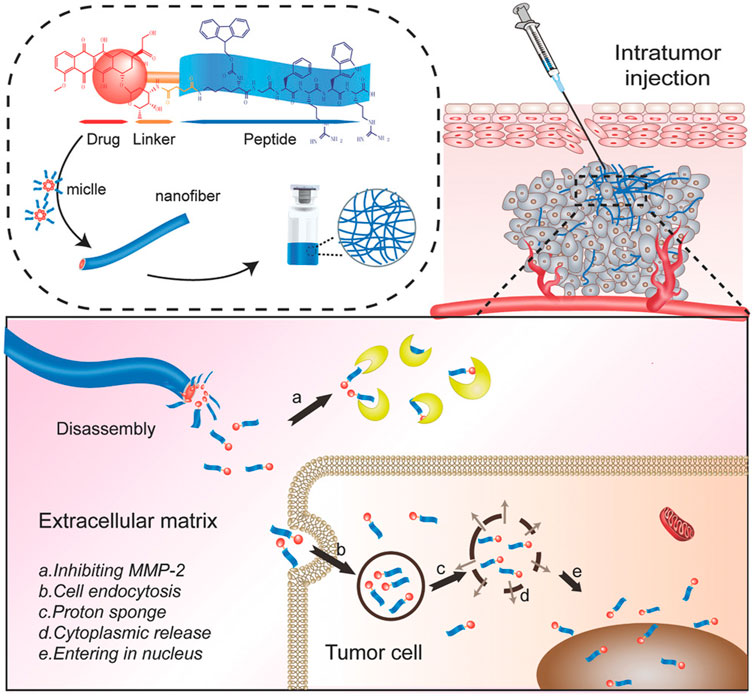
FIGURE 3. Self-assembling amphiphilic peptide drug containing amphiphilic peptide drug conjugate from oligomeric peptides (KGFRWR) and MMP-2 inhibitor doxorubicin (DOX). Reprinted from Ji et al. (2018).
3.2 Binding domains
Besides integrating small molecules, SAPs can incorporate binding domains to activate or inhibit cellular processes. Parallel and serial binding are two types of interactions common in receptor binding. In the case of parallel binding, coinciding binding occurs between multiple sites on the molecule and can have stronger total binding strength than monovalent interactions (Mammen et al., 1998; Varner et al., 2015). On the other hand, serial binding involves repeated weak-binding events that result in biological activities such as signal transduction (Ohlson et al., 1997; Ohlson, 2008). These binding activities play a fundamental role in intracellular and extracellular activity. Therefore, researchers can incorporate the binding domains in SAPs to get a biologic response. There are non-self-assembling therapeutic peptides that can deliver immunomodulatory agents. Such therapeutic peptides have been thoroughly reviewed and will not be discussed here (Lau and Dunn, 2018; Li et al., 2021; Thakur et al., 2022).
More than one binding domain can be incorporated into SAPs for vaccine applications. Particularly in active immunotherapy, the patient’s immune system is stimulated to produce a therapeutic response toward a disease or pathogen. The main goal of active immunotherapy is to generate a predictable B cell response without an autoreactive T cell response (Jia et al., 2013). Researchers have used B cell epitopes from the targeted protein, non-autologous T-helper epitopes incorporated in a carrier protein, and if the response is insufficient, adjuvants are added (Durez et al., 2014; Bavoso et al., 2015; Zhang et al., 2016). A supramolecular peptide system was created with exogenous T-cell epitopes and TNF B cell epitopes co-assembled into a nanofiber without additional adjuvants (Mora-Solano et al., 2017). Two T-cell epitopes were generated: high-affinity universal CD4+ T-cell epitope, PADRE, was used with Q11 to form PADREQ11, and T-cell epitope from Vaccinia I1L protein was used with Q11 to create VACQ11. A B cell epitope from mouse TNF was used with Q11 to form TNFQ11. In a TNF-mediated inflammation model, lipopolysaccharide was delivered intraperitoneally, and mice immunized with TNFQ11/PADREQ11 or TNFQ11/VACQ11 had improved survival. These findings indicate that a combination of B and T cell epitopes can produce immune cells’ specific activation towards a disease state.
Binding domains and small molecules can be included in SAPs. For instance, EAK16-II incorporated HIV-1 specific cytotoxic T-lymphocyte (CTL) epitope, SL9, to form SL9-EAK16-II (Ding et al., 2016). Additionally, toll-like receptor (TLR) agonists, R848 or R837, were included in SL9-EAK16-II as they have been shown to reduce the ability of lymphoid tissue to support HIV infection (Hofmann et al., 2016; Bam et al., 2017). In vitro and in vivo studies showed the co-delivery of CTL epitope and TLR7/8 agonist group SLP-EAK16-II/R848 had significantly strong SL9-specific CD8 T cell responses. A combination of known epitopes and agonists can be delivered through SAPs to obtain a desired immune response.
4 Self-assembling peptides releasing immunomodulators
Several new immunomodulators, such as immune checkpoint inhibitors (ICIs) and cytokines, have been used in a wide array of research areas. Immunomodulators can be divided into immunostimulators and immunosuppressants; depending on the context, they can activate or prevent immune cell activity. These modulators may face insufficient immune stimulation, off-target side effects, and bioactivity loss during circulation (Sathish et al., 2013; Feng et al., 2019). Many of these modulators, whether monotherapeutic or in combination, are administered systemically and require high doses to maintain modulators in circulation, ultimately resulting in high toxicities (Bast et al., 2019; Wang et al., 2022). Therefore, it is vital to have localized and sustained release of these immunomodulators. Many researchers have begun using self-assembling peptides to release immunomodulators, which have shown promise in several fields.
4.1 Antibodies
ICIs have succeeded in melanoma, renal cell carcinoma, and non-small cell lung cancer (Das and Johnson, 2019; Franzin et al., 2020). In head and neck squamous cell carcinoma (HNSCC), ICIs have shown some success. Particularly, anti-programmed death 1 (PD-1) was approved for treating recurrent/metastatic HNSCC (Burtness et al., 2019). Even though HNSCC has a similar mutational and immune profile as other solid cancers, anti-cytotoxic T lymphocyte-associated antigen 4 (CTLA-4) failed to demonstrate a benefit for HNSCC patients. Most of the HNSCC clinical trials testing anti-CTLA-4 recruited patients who previously received ablative locoregional therapies since HNSCC is prone to regional lymphatic metastasis (D'Cruz et al., 2015; Chow, 2020; Mody et al., 2021). Recently, it was found that by preserving the lymphatics, there is a robust immune response with anti-CTLA-4 in an HNSCC murine model (Saddawi-Konefka et al., 2022). Using a combination of anti-CTLA-4 and immunomodulators known to drive antigen processing and cross-presentation can help mount a robust antitumor response.
Tumor characteristics such as tumor immune infiltration and DNA damage pathways can influence immune checkpoint inhibitor efficacy (Bai et al., 2020; Meyers and Banerji, 2020). Tumor cells’ mutational burden can increase tumor antigenicity and enhance evasion strategies to targeted treatments (Seidel et al., 2018). Therefore, immunostimulatory substances are crucial for ICI combinations to have antigenicity and adjuvanticity (Galluzzi et al., 2020; Kwon et al., 2021). For instance, there have been early reports of combining anti-CTLA-4 with immunostimulatory substances such as GM-CSF, anti-CD25, and anti-CD40 (van Elsas et al., 1999; Sutmuller et al., 2001; Quezada et al., 2006; Sorensen et al., 2010). In an Oral Squamous Cell Carcinoma mouse model, anti-PD-1 with a STING agonist had improved tumor response compared to PBS + IgG2a (Shi et al., 2022). With these combinations, it was common to administer ICIs with their respective immunostimulant frequently. A biomaterial like SAPs can provide a controlled release strategy for ICIs and incorporate other motifs for targeting.
SAPs can load ICIs and other substances that elicit an antitumor response. As an illustration, a mixture of RADA16 peptide, anti-PD-1 antibodies, DCs, and tumor antigens was developed for cancer treatment (Figure 4). RADA16 peptide DC with model tumor antigen ovalbumin (OVA) and anti-PD-1 antibody (Gel-DC-OVA + anti-PD-1) increased the proportion of activated DCs in the draining lymph nodes and reduced regulatory T cells (T-regs) (Yang et al., 2018). Mice were rechallenged with, EG7-OVA cells, which are mouse lymphoma cells that exogenously expressed ovalbumin, and they found that Gel-DC-OVA and Gel-DC-OVA + anti-PD-1 delayed tumor growth. Although there were no significant differences in the addition of PD-1 blockade compared to Gel-DC-OVA, the PD-1 group saw improved tumor growth inhibition efficacy. In another study, DCs and whole tumor cell lysates (TCL) were loaded in the gels, and there was a significant efficiency in inhibiting tumor growth in the Gel-DC-TCL + anti-PD-1 group.
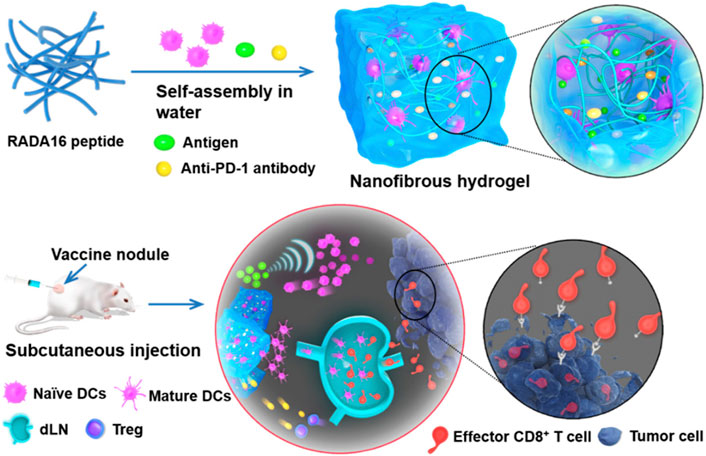
FIGURE 4. Delivery of exogenous dendritic cells and PD-1 in RADA16 for immunotherapy vaccine. Reprinted with permission from Yang et al. (2018). Copyright 2018 American Chemical Society.
Other cancer treatment strategies are looking at CD47 as a potential therapeutic target. CD47 is a transmembrane glycoprotein that emits a “do not eat” signal by binding to the signal regulatory protein α (SIRPα) on immune cells (Lian et al., 2019). Many cancers with poor prognosis express CD47 at high levels compared to normal cells (Chao et al., 2012; Lian et al., 2019). This signal is responsible for the escape of cancer cells from immune surveillance. Therefore, a self-assembling peptide LMY1 was created with a CD47 targeting motif, MMP-2 responsive peptide linker, self-assembly motif, and hydrophilic motif (Lv et al., 2022). The SAP first binds to CD47 on tumor cells followed by cleavage of the MMP-2 responsive peptide linker in the tumor immune microenvironment. This allows assembly into peptide-based nanofibers. The SAP nanofibers are then able to block subsequent interaction of CD47 and SIRPalpha, promoting the phagocytosis of tumor cells. In the subcutaneous Lewis lung carcinoma tumor model, combining LMY1 and anti-programmed death-ligand 1 (PD-L1) had the most significant antitumor efficacy and improved survival outcomes.
Sequestering immune checkpoint inhibitors in SAPs is a promising approach in cancer therapy. As illustrated by the previous example, combining multiple immunotherapeutic approaches is possible by using SAPs. A peptide nanofiber vaccine platform Coil29 was generated to include multiple epitopes to induce a coordinated antitumor immune response. Coil29 contains peptides with PEPvIII, B cell peptide epitope against EGFRvIII receptors, TRP2, a melanoma-associated CD8 T cell peptide antigen, and toxoid (Td) CD4 T cell epitope (Wu et al., 2022). After three subcutaneous immunizations with Coil29, the vaccine formulation containing three antigens PEPvIII, TRP2, and Toxoid (P/Tr/Td-fiber) and the Coil-29 with two antigens PEPvIII and Td (P/Td-fiber) both generated long-lasting PEPvIII-specific IgG responses compared to Complete Freund’s Adjuvant with the three antigens. The immunized mice were challenged with EGFRvIII-expressing B16vIII melanoma cells 2 weeks after final immunization. Tumor growth was significantly delayed in mice with P/Tr/Td-fibers and improved overall survival. When mice with B16vIII tumors were coadministered with P/Tr/Td-fibers and anti-PD-L1 and anti-CD47 there was significant tumor inhibition and long-term survival compared to the blockade group and the P/Tr/Td-fiber alone. Delivering multiple epitopes and immune checkpoint blockades in SAPs can aid in developing robust immune responses.
4.2 Cytokines and growth factors
Therapeutic strategies have also gone toward cytokine and growth factor interactions. Cytokines mediate the signaling among immune cells. There are different classes of cytokines, such as interleukins, interferons, and chemokines (Ramesh et al., 2013; Negishi et al., 2018). Some of the first approved cytokines were interferon α (IFN-α) as an adjuvant treatment for resected high-risk melanoma patients and high-dose IL-2 for metastatic renal cell cancer and melanoma. In cancer therapy, tumors can change their microenvironment by manipulating cellular and non-cellular components through complex signaling networks utilizing cytokines, chemokines, and growth factors to allow them to grow and spread (Baghban et al., 2020). In HNSCCs, the tumor immune microenvironment has been shown to impair tumor-infiltrating lymphocytes’ function and have immune-suppressive phenotypes, including myeloid-derived suppressive cells and T-regs (Chen et al., 2020). Dysfunctional tumor-infiltrating lymphocytes have decreased cytolytic activity and impaired production of effector cytokines such as IL-2, IFN-γ, and TNF-α (Zhang et al., 2020). Pro-inflammatory cytokines such as IL-2, IL-12, and IL-15 can improve antigen priming, increase the number of effector immune cells in the tumor immune microenvironment and enhance cytolytic activity (Berraondo et al., 2019). IL-2 can maintain T-regs to control the immune response and stimulate conventional T cells to promote the immune response (Abbas et al., 2018; Choudhry et al., 2018; Grasshoff et al., 2021). Unlike IL-2, IL-15 does not stimulate suppressive immunocytes such as T-regs (Saeed and Revell, 2001; Waldmann et al., 2020). IL-12 is crucial for the recruitment and effector functions of CD8 T cells and natural killer cells (Leonard et al., 1997; Del Vecchio et al., 2007; Nguyen et al., 2020; Mirlekar and Pylayeva-Gupta, 2021). As for growth factors, they stimulate cell proliferation, migration, and differentiation (Ren et al., 2019). These growth factors have been useful in regenerative medicine applications. Together, cytokines and growth factors can restore the intercellular communication needed to provide therapeutic potential.
Significant challenges arise when cytokines and growth factors are delivered with bolus or continuous administration (Pires et al., 2021). The small molecular size of cytokines and growth factors makes it difficult to localize them and can result in systemic toxicity outweighing their therapeutic efficacy (Baluna and Vitetta, 1997; Ren et al., 2019). Given the disadvantages of conventional systemic administration for cytokine therapy, a delivery vehicle capable of achieving the controlled release of cytokines would maximize their therapeutic potential while limiting the toxic systemic side effects and short half-life of these molecules (Berraondo et al., 2019; Conlon et al., 2019; Propper and Balkwill, 2022). Therefore, sequestering cytokines and growth factors in self-assembling peptides can mediate the release of these small signaling molecules.
Self-assembling peptides can be used to sequester cytokines. For instance, K-(SL)6-K-G-WKNFQTI (SLaM) was used to sequester chemokine (C-C) ligand 2 (CCL2), also known as monocyte chemoattractant protein-1 (MCP-1) from the extracellular matrix to reduce chemotaxis of monocytes and macrophages (Kim et al., 2020). Monocyte migration was reduced in the presence of SLaM hydrogel in contact with CCL2, and through ELISA found the hydrogel sequestered the majority of the CCL2 molecules in the solution. Using SAPs to sequester external signaling molecules can work as an adjunctive material for transplants or tissue regeneration without administering systemic immunosuppressants. Several cytokines can be delivered using SAPs. To demonstrate, a self-assembling domain and cell-adhesive fibronectin-derived RGDS sequence, also known as RGD, K(SL)2(SLRG)(SL)3K(GRGDS)(SLac) was used to release MCP-1 and IL-4 to foster a proangiogenic environment for tissue engineering applications (Kumar et al., 2015). MCP-1 had most of its release during the first 48 h, while IL-4 had prolonged release over 16 days. From the in vivo studies, SLac + MCP-1+IL-4 promoted infiltration, recruited macrophages, and polarized them to an M2 phenotype within and around hydrogel. This temporal release is not limited to proangiogenic cytokines; they can also be applied to pro-inflammatory applications such as cancer (Berraondo et al., 2019; Nash et al., 2021). The controlled release in SAPs can be beneficial in enhancing the half-life of cytokines and ensuring a robust immune response.
Spatiotemporal release can be achieved with the co-delivery of cytokines and growth factors in ischemic acute kidney injury (AKI). Patients with AKI have reduced kidney function and are at risk for chronic kidney disease. Many pro-inflammatory markers have been observed in AKI, including cytokines such as TNF-α and IL-1β, which can cause cell damage (Gao et al., 2013). Growth factors such as hepatocyte growth factor (HGF) have been reported to stimulate renal epithelial cell proliferation and induce tubular formation (Mao and Zhang, 2016). Therefore, the co-delivery of TNF-α neutralizing antibody and HGF in KLD2R/heparin hydrogel can enhance renal protective potential and reduce chronic renal fibrosis (Liu et al., 2020). A faster release of anti-TNF-α was achieved due to protein diffusion through the KLD2R hydrogel, while the slower release of HGF was due to a combination of heparin-binding affinity and molecular diffusion. This dual-drug delivery platform achieved sequential release kinetics of anti-TNF-α and HGF while promoting tissue repair. SAPs can allow for controlled spatiotemporal cytokine and growth factor release to augment an immune response.
5 Future directions
Self-assembling peptides can inherently contribute to therapeutics by integrating functional moieties. By knowing the disease pathology pathways SAPs can be modified to incorporate small molecules and receptor domains to improve disease state. Although functional moieties can be incorporated into SAPs, it is difficult to precisely control and predict secondary and supramolecular structures (Scanlon, 2008; Pitz et al., 2022). Therefore, more advanced computational modeling must be developed to accurately predict the structure of self-assembling peptides (Chang et al., 2022; Pitz et al., 2022).
The ability to modify peptides in SAPs makes it possible for large-scale production. In dental applications, it is common to treat early caries with fluoride to repair tooth enamel. A commercially available P11-4, Curodont™ Repair, was combined with fluoride for the non-invasive treatment of early occlusal enamel lesions (ClinicalTrials.gov: NCT02724592). They found the combination of P11-4 with fluoride facilitated biomimetic mineralization and was a safe and effective treatment for early carious lesions (Alkilzy et al., 2018). Current studies are looking into SAPs as an adjunct during surgery. There is a risk for hemorrhage by using Transoral Robotic Surgery for human papillomavirus positive early-stage oropharyngeal squamous cell carcinoma (Leonhardt et al., 2012; Moore et al., 2012; Chen et al., 2015). A hemostatic agent from the RADA16 family, PuraBond®, was used as an adjunct during Transoral Robotic Surgery (Gupta et al., 2022). Preliminary findings showed none of the patients developed primary or secondary hemorrhage post-transoral robotic surgery in conjunction with PuraBond®. These findings were based on a small cohort; further large-scale studies are needed to determine whether patients receive clinical benefit from the hemostatic and regenerative properties of PuraBond® (ClinicalTrials.gov: NCT05405907).
Immunomodulators such as cytokines traditionally have rapid clearance. The usual systemic delivery of ICIs have been associated with severe immune-related adverse events and even death; therefore, sequestering these modulators in SAPs can control drug release (Newton et al., 2019). Although maintaining modulators is important to sequester, it is imperative to consider the rate of degradation of the biomaterial. If a significant amount of cytokines are released at once, it can result in toxicity and, depending if there is a robust immune response, a cytokine storm (Baldo, 2014; Pires et al., 2021). Future work should consider having the temporal release of modulators that can strategically activate immune cells at certain times, which will be necessary with combination therapy.
There are commercially available SAPs being used as scaffolds and delivery vehicles. RADA16 has been commercialized as PuraMatrix™, which forms 3D hydrogel-forming nanofibrous structures to support the attachment of various cell types, tumor cell migration and invasion, and in vivo analysis of tissue regeneration. PuraMatrix™, in combination with mesenchymal stromal cells, was used as an epicardial coating of the heart and noted improved global cardiac function and decreased ventricular dilatation (Ichihara et al., 2018). Using these readily available SAPs allows for further modification in several other applications. To date, there are no clinical trials or FDA-approved self-assembling peptides for immunotherapy applications, although clinical trials of these materials for tissue regeneration are ongoing (ClinicalTrials.gov: NCT05127889, NCT05206539). Given the promise SAPs have shown for immunomodulation in the preclinical setting, we anticipate clinical applications of these materials in the future.
6 Conclusion
Self-assembling peptides are versatile materials that allow for tailoring peptide sequences to house antibodies, cytokines, and small molecules for applications in tissue engineering, immunotherapy, and drug delivery. SAPs can mimic the natural framework and are tunable to include biologically active systems. The adaptable nature of these peptides allows for the modification of their chemical composition to include modulators. A critical part of SAPs is maintaining biomaterial integrity to build the foundation of tissue growth or release immunomodulators for drug delivery and immunotherapy applications. To develop a precise SAP structure and targeting ability, advanced computational modeling is needed. The next-generation of SAPs should consider integration of spatiotemporal motifs for combination treatment.
Author contributions
AH designed the structure and wrote the first draft of the manuscript. AH, JH and SY corrected and edited the manuscript. All authors contributed to the article and approved the submitted version.
Funding
AH is a Ph.D. graduate student at The University of Texas MD Anderson Cancer Center UT Health Houston Graduate School of Biomedical Sciences and a recipient of a Diversity Supplement (R01DE030140) from the National Institutes of Health-National Institute of Dental and Craniofacial Research (NIH-NIDCR). This work was supported by the NIH-NIDCR grants R01DE030140 and R01DE021798.
Acknowledgments
We would like to thank Dr. Neeraja Dharmaraj for providing feedback.
Conflict of interest
The authors declare that the research was conducted in the absence of any commercial or financial relationships that could be construed as a potential conflict of interest.
Publisher’s note
All claims expressed in this article are solely those of the authors and do not necessarily represent those of their affiliated organizations, or those of the publisher, the editors and the reviewers. Any product that may be evaluated in this article, or claim that may be made by its manufacturer, is not guaranteed or endorsed by the publisher.
References
Abbas, A. K., Trotta, E., D, R. S., Marson, A., and Bluestone, J. A. (2018). Revisiting IL-2: Biology and therapeutic prospects. Sci. Immunol. 3 (25), eaat1482. doi:10.1126/sciimmunol.aat1482
Alkilzy, M., Tarabaih, A., Santamaria, R. M., and Splieth, C. H. (2018). Self-assembling peptide P(11)-4 and fluoride for regenerating enamel. J. Dent. Res. 97 (2), 148–154. doi:10.1177/0022034517730531
Andorko, J. I., and Jewell, C. M. (2017). Designing biomaterials with immunomodulatory properties for tissue engineering and regenerative medicine. Bioeng. Transl. Med. 2 (2), 139–155. doi:10.1002/btm2.10063
Baghban, R., Roshangar, L., Jahanban-Esfahlan, R., Seidi, K., Ebrahimi-Kalan, A., Jaymand, M., et al. (2020). Tumor microenvironment complexity and therapeutic implications at a glance. Cell Commun. Signal 18 (1), 59. doi:10.1186/s12964-020-0530-4
Bai, R., Lv, Z., Xu, D., and Cui, J. (2020). Predictive biomarkers for cancer immunotherapy with immune checkpoint inhibitors. Biomark. Res. 8, 34. doi:10.1186/s40364-020-00209-0
Baldo, B. A. (2014). Side effects of cytokines approved for therapy. Drug Saf. 37 (11), 921–943. doi:10.1007/s40264-014-0226-z
Baluna, R., and Vitetta, E. S. (1997). Vascular leak syndrome: A side effect of immunotherapy. Immunopharmacology 37 (2-3), 117–132. doi:10.1016/s0162-3109(97)00041-6
Bam, R. A., Hansen, D., Irrinki, A., Mulato, A., Jones, G. S., Hesselgesser, J., et al. (2017). TLR7 agonist GS-9620 is a potent inhibitor of acute HIV-1 infection in human peripheral blood mononuclear cells. Antimicrob. Agents Chemother. 61 (1), 013699–e1416. doi:10.1128/AAC.01369-16
Bast, R. C., Matulonis, U. A., Sood, A. K., Ahmed, A. A., Amobi, A. E., Balkwill, F. R., et al. (2019). Critical questions in ovarian cancer research and treatment: Report of an American association for cancer research special conference. Cancer 125 (12), 1963–1972. doi:10.1002/cncr.32004
Bavoso, A., Ostuni, A., De Vendel, J., Bracalello, A., Shcheglova, T., Makker, S., et al. (2015). Aldehyde modification and alum coadjuvancy enhance anti-TNF-alpha autovaccination and mitigate arthritis in rat. J. Pept. Sci. 21 (5), 400–407. doi:10.1002/psc.2718
Berraondo, P., Sanmamed, M. F., Ochoa, M. C., Etxeberria, I., Aznar, M. A., Perez-Gracia, J. L., et al. (2019). Cytokines in clinical cancer immunotherapy. Br. J. Cancer 120 (1), 6–15. doi:10.1038/s41416-018-0328-y
Berthiaume, F., Maguire, T. J., and Yarmush, M. L. (2011). Tissue engineering and regenerative medicine: History, progress, and challenges. Annu. Rev. Chem. Biomol. Eng. 2, 403–430. doi:10.1146/annurev-chembioeng-061010-114257
Burtness, B., Harrington, K. J., Greil, R., Soulieres, D., Tahara, M., de Castro, G., et al. (2019). Pembrolizumab alone or with chemotherapy versus cetuximab with chemotherapy for recurrent or metastatic squamous cell carcinoma of the head and neck (KEYNOTE-048): A randomised, open-label, phase 3 study. Lancet 394 (10212), 1915–1928. doi:10.1016/S0140-6736(19)32591-7
Chang, L., Mondal, A., and Perez, A. (2022). Towards rational computational peptide design. Front. Bioinform 2, 1046493. doi:10.3389/fbinf.2022.1046493
Chang, R., and Yan, X. (2020). Supramolecular immunotherapy of cancer based on the self-assembling peptide design. Small Struct. 1 (2), 2000068. doi:10.1002/sstr.202000068
Chao, M. P., Weissman, I. L., and Majeti, R. (2012). The CD47–SIRPα pathway in cancer immune evasion and potential therapeutic implications. Curr. Opin. Immunol. 24 (2), 225–232. doi:10.1016/j.coi.2012.01.010
Chen, A. M., Daly, M. E., Luu, Q., Donald, P. J., and Farwell, D. G. (2015). Comparison of functional outcomes and quality of life between transoral surgery and definitive chemoradiotherapy for oropharyngeal cancer. Head. Neck 37 (3), 381–385. doi:10.1002/hed.23610
Chen, J., Huang, Y., Ma, X., and Lei, Y. (2018a). Functional self-healing materials and their potential applications in biomedical engineering. Adv. Compos. Hybrid Mater. 1 (1), 94–113. doi:10.1007/s42114-017-0009-y
Chen, J., and Zou, X. (2019). Self-assemble peptide biomaterials and their biomedical applications. Bioact. Mater 4, 120–131. doi:10.1016/j.bioactmat.2019.01.002
Chen, L., Deng, H., Cui, H., Fang, J., Zuo, Z., Deng, J., et al. (2018b). Inflammatory responses and inflammation-associated diseases in organs. Oncotarget 9 (6), 7204–7218. doi:10.18632/oncotarget.23208
Chen, S. M. Y., Krinsky, A. L., Woolaver, R. A., Wang, X., Chen, Z., and Wang, J. H. (2020). Tumor immune microenvironment in head and neck cancers. Mol. Carcinog. 59 (7), 766–774. doi:10.1002/mc.23162
Choudhry, H., Helmi, N., Abdulaal, W. H., Zeyadi, M., Zamzami, M. A., Wu, W., et al. (2018). Prospects of IL-2 in cancer immunotherapy. Biomed. Res. Int. 2018, 1–7. doi:10.1155/2018/9056173
Chow, L. Q. M. (2020). Head and neck cancer. N. Engl. J. Med. 382 (1), 60–72. doi:10.1056/NEJMra1715715
Conlon, K. C., Miljkovic, M. D., and Waldmann, T. A. (2019). Cytokines in the treatment of cancer. J. Interferon Cytokine Res. 39 (1), 6–21. doi:10.1089/jir.2018.0019
Corrales, L., Glickman, L. H., McWhirter, S. M., Kanne, D. B., Sivick, K. E., Katibah, G. E., et al. (2015). Direct activation of STING in the tumor microenvironment leads to potent and systemic tumor regression and immunity. Cell Rep. 11 (7), 1018–1030. doi:10.1016/j.celrep.2015.04.031
Cui, H., Webber, M. J., and Stupp, S. I. (2010). Self-assembly of peptide amphiphiles: From molecules to nanostructures to biomaterials. Peptide Sci. 94 (1), 1–18. doi:10.1002/bip.21328
D'Cruz, A. K., Vaish, R., Kapre, N., Dandekar, M., Gupta, S., Hawaldar, R., et al. (2015). Elective versus therapeutic neck dissection in node-negative oral cancer. N. Engl. J. Med. 373 (6), 521–529. doi:10.1056/NEJMoa1506007
Das, S., and Johnson, D. B. (2019). Immune-related adverse events and anti-tumor efficacy of immune checkpoint inhibitors. J. Immunother. Cancer 7 (1), 306. doi:10.1186/s40425-019-0805-8
Davison, N. L., Barrère-de Groot, F., and Grijpma, D. W. (2014). Chapter 6 - degradation of biomaterials. Academic Press.
Decout, A., Katz, J. D., Venkatraman, S., and Ablasser, A. (2021). The cGAS-STING pathway as a therapeutic target in inflammatory diseases. Nat. Rev. Immunol. 21 (9), 548–569. doi:10.1038/s41577-021-00524-z
Del Vecchio, M., Bajetta, E., Canova, S., Lotze, M. T., Wesa, A., Parmiani, G., et al. (2007). Interleukin-12: Biological properties and clinical application. Clin. Cancer Res. 13 (16), 4677–4685. doi:10.1158/1078-0432.CCR-07-0776
Diesendruck, C. E., Sottos, N. R., Moore, J. S., and White, S. R. (2015). Biomimetic self-healing. Angew. Chem. Int. Ed. Engl. 54 (36), 10428–10447. doi:10.1002/anie.201500484
Ding, Y., Liu, J., Lu, S., Igweze, J., Xu, W., Kuang, D., et al. (2016). Self-assembling peptide for co-delivery of HIV-1 CD8+ T cells epitope and Toll-like receptor 7/8 agonists R848 to induce maturation of monocyte derived dendritic cell and augment polyfunctional cytotoxic T lymphocyte (CTL) response. J. Control Release 236, 22–30. doi:10.1016/j.jconrel.2016.06.019
Dumas, J. E., Prieto, E. M., Zienkiewicz, K. J., Guda, T., Wenke, J. C., Bible, J., et al. (2014). Balancing the rates of new bone formation and polymer degradation enhances healing of weight-bearing allograft/polyurethane composites in rabbit femoral defects. Tissue Eng. Part A 20 (1-2), 115–129. doi:10.1089/ten.TEA.2012.0762
Durez, P., Vandepapeliere, P., Miranda, P., Toncheva, A., Berman, A., Kehler, T., et al. (2014). Therapeutic vaccination with TNF-kinoid in TNF antagonist-resistant rheumatoid arthritis: A phase II randomized, controlled clinical trial. PLoS One 9 (12), e113465. doi:10.1371/journal.pone.0113465
Fan, T., Yu, X., Shen, B., and Sun, L. (2017). Peptide self-assembled nanostructures for drug delivery applications. J. Nanomater. 2017, 1–16. doi:10.1155/2017/4562474
Feng, X., Xu, W., Li, Z., Song, W., Ding, J., and Chen, X. (2019). Immunomodulatory nanosystems. Adv. Sci. (Weinh) 6 (17), 1900101. doi:10.1002/advs.201900101
Franzin, R., Netti, G. S., Spadaccino, F., Porta, C., Gesualdo, L., Stallone, G., et al. (2020). The use of immune checkpoint inhibitors in oncology and the occurrence of AKI: Where do we stand? Front. Immunol. 11, 574271. doi:10.3389/fimmu.2020.574271
Fukumura, D., Kashiwagi, S., and Jain, R. K. (2006). The role of nitric oxide in tumour progression. Nat. Rev. Cancer 6 (7), 521–534. doi:10.1038/nrc1910
Galluzzi, L., Humeau, J., Buque, A., Zitvogel, L., and Kroemer, G. (2020). Immunostimulation with chemotherapy in the era of immune checkpoint inhibitors. Nat. Rev. Clin. Oncol. 17 (12), 725–741. doi:10.1038/s41571-020-0413-z
Gao, G., Zhang, B., Ramesh, G., Betterly, D., Tadagavadi, R. K., Wang, W., et al. (2013). TNF-alpha mediates increased susceptibility to ischemic AKI in diabetes. Am. J. Physiol. Ren. Physiol. 304 (5), F515–F521. doi:10.1152/ajprenal.00533.2012
Grasshoff, H., Comduhr, S., Monne, L. R., Muller, A., Lamprecht, P., Riemekasten, G., et al. (2021). Low-dose IL-2 therapy in autoimmune and rheumatic diseases. Front. Immunol. 12, 648408. doi:10.3389/fimmu.2021.648408
Griffith, M., Islam, M. M., Edin, J., Papapavlou, G., Buznyk, O., and Patra, H. K. (2016). The quest for anti-inflammatory and anti-infective biomaterials in clinical translation. Front. Bioeng. Biotechnol. 4, 71. doi:10.3389/fbioe.2016.00071
Gupta, K. K., Garas, G., Idle, M., Germain, S., and De, M. (2022). Evaluating the role of the self-assembling topical haemostat PuraBond® in transoral robotic surgery (tors) for oropharyngeal cancer: A case series. Ann. Med. Surg. (Lond) 80, 104302. doi:10.1016/j.amsu.2022.104302
Gurevich, E. V., and Gurevich, V. V. (2014). Therapeutic potential of small molecules and engineered proteins. Handb. Exp. Pharmacol. 219, 1–12. doi:10.1007/978-3-642-41199-1_1
Habibi, N., Kamaly, N., Memic, A., and Shafiee, H. (2016). Self-assembled peptide-based nanostructures: Smart nanomaterials toward targeted drug delivery. Nano Today 11 (1), 41–60. doi:10.1016/j.nantod.2016.02.004
Han, C., Zhang, Z., Sun, J., Li, K., Li, Y., Ren, C., et al. (2020). <p>Self-Assembling Peptide-Based Hydrogels in Angiogenesis</p>. Int. J. Nanomedicine 15, 10257–10269. doi:10.2147/IJN.S277046
Herbst, F., Dohler, D., Michael, P., and Binder, W. H. (2013). Self-healing polymers via supramolecular forces. Macromol. Rapid Commun. 34 (3), 203–220. doi:10.1002/marc.201200675
Hoffman, A. S. (2002). Hydrogels for biomedical applications. Adv. Drug Deliv. Rev. 54 (1), 3–12. doi:10.1016/s0169-409x(01)00239-3
Hofmann, H., Vanwalscappel, B., Bloch, N., and Landau, N. R. (2016). TLR7/8 agonist induces a post-entry SAMHD1-independent block to HIV-1 infection of monocytes. Retrovirology 13 (1), 83. doi:10.1186/s12977-016-0316-3
Ichihara, Y., Kaneko, M., Yamahara, K., Koulouroudias, M., Sato, N., Uppal, R., et al. (2018). Self-assembling peptide hydrogel enables instant epicardial coating of the heart with mesenchymal stromal cells for the treatment of heart failure. Biomaterials 154, 12–23. doi:10.1016/j.biomaterials.2017.10.050
Jayaraman, P., Parikh, F., Lopez-Rivera, E., Hailemichael, Y., Clark, A., Ma, G., et al. (2012). Tumor-expressed inducible nitric oxide synthase controls induction of functional myeloid-derived suppressor cells through modulation of vascular endothelial growth factor release. J. Immunol. 188 (11), 5365–5376. doi:10.4049/jimmunol.1103553
Jenkins, D. C., Charles, I. G., Thomsen, L. L., Moss, D. W., Holmes, L. S., Baylis, S. A., et al. (1995). Roles of nitric oxide in tumor growth. Proc. Natl. Acad. Sci. U. S. A. 92 (10), 4392–4396. doi:10.1073/pnas.92.10.4392
Ji, Y., Xiao, Y., Xu, L., He, J., Qian, C., Li, W., et al. (2018). Drug-bearing supramolecular MMP inhibitor nanofibers for inhibition of metastasis and growth of liver cancer. Adv. Sci. (Weinh) 5 (8), 1700867. doi:10.1002/advs.201700867
Jia, T., Pan, Y., Li, J., and Wang, L. (2013). Strategies for active TNF-alpha vaccination in rheumatoid arthritis treatment. Vaccine 31 (38), 4063–4068. doi:10.1016/j.vaccine.2013.06.101
Jiang, M., Chen, P., Wang, L., Li, W., Chen, B., Liu, Y., et al. (2020). cGAS-STING, an important pathway in cancer immunotherapy. J. Hematol. Oncol. 13 (1), 81. doi:10.1186/s13045-020-00916-z
Kidane, A., and Bhatt, P. P. (2005). Recent advances in small molecule drug delivery. Curr. Opin. Chem. Biol. 9 (4), 347–351. doi:10.1016/j.cbpa.2005.06.006
Kim, D. S., Endo, A., Fang, F. G., Huang, K. C., Bao, X., Choi, H. W., et al. (2021). E7766, a macrocycle-bridged stimulator of interferon Genes (STING) agonist with potent pan-genotypic activity. ChemMedChem 16 (11), 1741–1744. doi:10.1002/cmdc.202100068
Kim, K. K., Siddiqui, Z., Patel, M., Sarkar, B., and Kumar, V. A. (2020). A self-assembled peptide hydrogel for cytokine sequestration. J. Mater Chem. B 8 (5), 945–950. doi:10.1039/c9tb02250c
Kumar, V. A., Taylor, N. L., Shi, S., Wickremasinghe, N. C., D'Souza, R. N., and Hartgerink, J. D. (2015). Self-assembling multidomain peptides tailor biological responses through biphasic release. Biomaterials 52, 71–78. doi:10.1016/j.biomaterials.2015.01.079
Kwon, M., Jung, H., Nam, G. H., and Kim, I. S. (2021). The right Timing, right combination, right sequence, and right delivery for Cancer immunotherapy. J. Control Release 331, 321–334. doi:10.1016/j.jconrel.2021.01.009
La Manna, S., Di Natale, C., Onesto, V., and Marasco, D. (2021). Self-assembling peptides: From design to biomedical applications. Int. J. Mol. Sci. 22 (23), 12662. doi:10.3390/ijms222312662
Langer, R., Cima, L. G., Tamada, J. A., and Wintermantel, E. (1990). Future directions in biomaterials. Biomaterials 11 (9), 738–745. doi:10.1016/0142-9612(90)90038-r
Lau, J. L., and Dunn, M. K. (2018). Therapeutic peptides: Historical perspectives, current development trends, and future directions. Bioorg Med. Chem. 26 (10), 2700–2707. doi:10.1016/j.bmc.2017.06.052
Leach, D. G., Dharmaraj, N., Lopez-Silva, T. L., Venzor, J. R., Pogostin, B. H., Sikora, A. G., et al. (2021). Biomaterial-facilitated immunotherapy for established oral cancers. ACS Biomater. Sci. Eng. 7 (2), 415–421. doi:10.1021/acsbiomaterials.0c01575
Leach, D. G., Dharmaraj, N., Piotrowski, S. L., Lopez-Silva, T. L., Lei, Y. L., Sikora, A. G., et al. (2018). STINGel: Controlled release of a cyclic dinucleotide for enhanced cancer immunotherapy. Biomaterials 163, 67–75. doi:10.1016/j.biomaterials.2018.01.035
Leach, D. G., Newton, J. M., Florez, M. A., Lopez-Silva, T. L., Jones, A. A., Young, S., et al. (2019a). Drug-mimicking nanofibrous peptide hydrogel for inhibition of inducible nitric oxide synthase. ACS Biomater. Sci. Eng. 5 (12), 6755–6765. doi:10.1021/acsbiomaterials.9b01447
Leach, D. G., Young, S., and Hartgerink, J. D. (2019b). Advances in immunotherapy delivery from implantable and injectable biomaterials. Acta Biomater. 88, 15–31. doi:10.1016/j.actbio.2019.02.016
Lee, S., Trinh, T. H. T., Yoo, M., Shin, J., Lee, H., Kim, J., et al. (2019). Self-assembling peptides and their application in the treatment of diseases. Int. J. Mol. Sci. 20 (23), 5850. doi:10.3390/ijms20235850
Leonard, J. P., Sherman, M. L., Fisher, G. L., Buchanan, L. J., Larsen, G., Atkins, M. B., et al. (1997). Effects of single-dose interleukin-12 exposure on interleukin-12–associated toxicity and interferon-γ production. Blood 90 (7), 2541–2548. doi:10.1182/blood.V90.7.2541
Leonhardt, F. D., Quon, H., Abrahao, M., O'Malley, B. W., and Weinstein, G. S. (2012). Transoral robotic surgery for oropharyngeal carcinoma and its impact on patient-reported quality of life and function. Head. Neck 34 (2), 146–154. doi:10.1002/hed.21688
Levin, A., Hakala, T. A., Schnaider, L., Bernardes, G. J. L., Gazit, E., and Knowles, T. P. J. (2020). Biomimetic peptide self-assembly for functional materials. Nat. Rev. Chem. 4 (11), 615–634. doi:10.1038/s41570-020-0215-y
Li, C. M., Haratipour, P., Lingeman, R. G., Perry, J. J. P., Gu, L., Hickey, R. J., et al. (2021). Novel peptide therapeutic approaches for cancer treatment. Cells 10 (11), 2908. doi:10.3390/cells10112908
Li, T., Lu, X. M., Zhang, M. R., Hu, K., and Li, Z. (2022). Peptide-based nanomaterials: Self-assembly, properties and applications. Bioact. Mater 11, 268–282. doi:10.1016/j.bioactmat.2021.09.029
Lian, S., Xie, X., Lu, Y., and Jia, L. (2019). <p>Checkpoint CD47 function on tumor metastasis and immune therapy</p>. Onco Targets Ther. 12, 9105–9114. doi:10.2147/OTT.S220196
Liu, S., Zhao, M., Zhou, Y., Li, L., Wang, C., Yuan, Y., et al. (2020). A self-assembling peptide hydrogel-based drug co-delivery platform to improve tissue repair after ischemia-reperfusion injury. Acta Biomater. 103, 102–114. doi:10.1016/j.actbio.2019.12.011
Loo, Y., Goktas, M., Tekinay, A. B., Guler, M. O., Hauser, C. A., and Mitraki, A. (2015). Self-assembled proteins and peptides as scaffolds for tissue regeneration. Adv. Healthc. Mater 4 (16), 2557–2586. doi:10.1002/adhm.201500402
Lopez-Silva, T. L., Leach, D. G., Azares, A., Li, I. C., Woodside, D. G., and Hartgerink, J. D. (2020). Chemical functionality of multidomain peptide hydrogels governs early host immune response. Biomaterials 231, 119667. doi:10.1016/j.biomaterials.2019.119667
Lopez-Silva, T. L., Leach, D. G., Li, I. C., Wang, X., and Hartgerink, J. D. (2019). Self-assembling multidomain peptides: Design and characterization of neutral peptide-based materials with pH and ionic strength independent self-assembly. ACS Biomater. Sci. Eng. 5 (2), 977–985. doi:10.1021/acsbiomaterials.8b01348
Lu, B., and Atala, A. (2014). Small molecules and small molecule drugs in regenerative medicine. Drug Discov. Today 19 (6), 801–808. doi:10.1016/j.drudis.2013.11.011
Lv, M. Y., Xiao, W. Y., Zhang, Y. P., Jin, L. L., Li, Z. H., Lei, Z., et al. (2022). In situ self-assembled peptide enables effective cancer immunotherapy by blockage of CD47. Colloids Surf. B Biointerfaces 217, 112655. doi:10.1016/j.colsurfb.2022.112655
Mammen, M., Choi, S.-K., and Whitesides, G. M. (1998). Polyvalent interactions in biological systems: Implications for design and use of multivalent ligands and inhibitors. Angew. Chem. Int. Ed. 37 (20), 2754–2794. doi:10.1002/(SICI)1521-3773(19981102)37:20<2754::AID-ANIE2754>3.0.CO;2-3
Mao, S., and Zhang, J. (2016). The emerging role of hepatocyte growth factor in renal diseases. J. Recept Signal Transduct. Res. 36 (3), 303–309. doi:10.3109/10799893.2015.1080275
Meyers, D. E., and Banerji, S. (2020). Biomarkers of immune checkpoint inhibitor efficacy in cancer. Curr. Oncol. 27 (2), S106–S114. doi:10.3747/co.27.5549
Mirlekar, B., and Pylayeva-Gupta, Y. (2021). IL-12 family cytokines in cancer and immunotherapy. Cancers (Basel) 13 (2), 167. doi:10.3390/cancers13020167
Mody, M. D., Rocco, J. W., Yom, S. S., Haddad, R. I., and Saba, N. F. (2021). Head and neck cancer. Lancet 398 (10318), 2289–2299. doi:10.1016/S0140-6736(21)01550-6
Moore, A. N., and Hartgerink, J. D. (2017). Self-assembling multidomain peptide nanofibers for delivery of bioactive molecules and tissue regeneration. Acc. Chem. Res. 50 (4), 714–722. doi:10.1021/acs.accounts.6b00553
Moore, A. N., Lopez Silva, T. L., Carrejo, N. C., Origel Marmolejo, C. A., Li, I. C., and Hartgerink, J. D. (2018). Nanofibrous peptide hydrogel elicits angiogenesis and neurogenesis without drugs, proteins, or cells. Biomaterials 161, 154–163. doi:10.1016/j.biomaterials.2018.01.033
Moore, E. J., Olsen, S. M., Laborde, R. R., Garcia, J. J., Walsh, F. J., Price, D. L., et al. (2012). Long-term functional and oncologic results of transoral robotic surgery for oropharyngeal squamous cell carcinoma. Mayo Clin. Proc. 87 (3), 219–225. doi:10.1016/j.mayocp.2011.10.007
Mora-Solano, C., Wen, Y., Han, H., Chen, J., Chong, A. S., Miller, M. L., et al. (2017). Active immunotherapy for TNF-mediated inflammation using self-assembled peptide nanofibers. Biomaterials 149, 1–11. doi:10.1016/j.biomaterials.2017.09.031
Nash, A., Aghlara-Fotovat, S., Hernandez, A., Scull, C., and Veiseh, O. (2021). Clinical translation of immunomodulatory therapeutics. Adv. Drug Deliv. Rev. 176, 113896. doi:10.1016/j.addr.2021.113896
Negishi, H., Taniguchi, T., and Yanai, H. (2018). The interferon (IFN) class of cytokines and the IFN regulatory factor (IRF) transcription factor family. Cold Spring Harb. Perspect. Biol. 10 (11), a028423. doi:10.1101/cshperspect.a028423
Newton, J. M., Sikora, A. G., and Young, S. (2019). “Chapter 41 - materials-based cancer immunotherapies,” in Principles of regenerative medicine. Editors A. Atala, R. Lanza, A. G. Mikos, and R. Nerem Third Edition (Boston: Academic Press), 715–739.
Nguyen, K. G., Vrabel, M. R., Mantooth, S. M., Hopkins, J. J., Wagner, E. S., Gabaldon, T. A., et al. (2020). Localized interleukin-12 for cancer immunotherapy. Front. Immunol. 11, 575597. doi:10.3389/fimmu.2020.575597
Nguyen, P. K., Sarkar, B., Siddiqui, Z., McGowan, M., Iglesias-Montoro, P., Rachapudi, S., et al. (2018). Self-assembly of an antiangiogenic nanofibrous peptide hydrogel. ACS Appl. Bio Mater 1 (3), 865–870. doi:10.1021/acsabm.8b00283
O'Brien, F. J. (2011). Biomaterials & scaffolds for tissue engineering. Mater. Today 14 (3), 88–95. doi:10.1016/S1369-7021(11)70058-X
Ohlson, S. (2008). Designing transient binding drugs: A new concept for drug discovery. Drug Discov. Today 13 (9-10), 433–439. doi:10.1016/j.drudis.2008.02.001
Ohlson, S., Strandh, M., and Nilshans, H. (1997). Detection and characterization of weak affinity antibody antigen recognition with biomolecular interaction analysis. J. Mol. Recognit. 10 (3), 135–138. doi:10.1002/(SICI)1099-1352(199705/06)10:3<135::AID-JMR355>3.0.CO;2-B
Overstreet, D. J., Dutta, D., Stabenfeldt, S. E., and Vernon, B. L. (2012). Injectable hydrogels. J. Polym. Sci. Part B Polym. Phys. 50 (13), 881–903. doi:10.1002/polb.23081
Peng, W., Li, D., Dai, K., Wang, Y., Song, P., Li, H., et al. (2022). Recent progress of collagen, chitosan, alginate and other hydrogels in skin repair and wound dressing applications. Int. J. Biol. Macromol. 208, 400–408. doi:10.1016/j.ijbiomac.2022.03.002
Pires, I. S., Hammond, P. T., and Irvine, D. J. (2021). Engineering strategies for immunomodulatory cytokine therapies - challenges and clinical progress. Adv. Ther. (Weinh) 4 (8), 2100035. doi:10.1002/adtp.202100035
Pitz, M. E., Nukovic, A. M., Elpers, M. A., and Alexander-Bryant, A. A. (2022). Factors affecting secondary and supramolecular structures of self-assembling peptide nanocarriers. Macromol. Biosci. 22 (2), e2100347. doi:10.1002/mabi.202100347
Propper, D. J., and Balkwill, F. R. (2022). Harnessing cytokines and chemokines for cancer therapy. Nat. Rev. Clin. Oncol. 19 (4), 237–253. doi:10.1038/s41571-021-00588-9
Quezada, S. A., Peggs, K. S., Curran, M. A., and Allison, J. P. (2006). CTLA4 blockade and GM-CSF combination immunotherapy alters the intratumor balance of effector and regulatory T cells. J. Clin. Invest. 116 (7), 1935–1945. doi:10.1172/JCI27745
Ramesh, G., MacLean, A. G., and Philipp, M. T. (2013). Cytokines and chemokines at the crossroads of neuroinflammation, neurodegeneration, and neuropathic pain. Mediat. Inflamm. 2013, 1–20. doi:10.1155/2013/480739
Ren, X., Zhao, M., Lash, B., Martino, M. M., and Julier, Z. (2019). Growth factor engineering strategies for regenerative medicine applications. Front. Bioeng. Biotechnol. 7, 469. doi:10.3389/fbioe.2019.00469
Saddawi-Konefka, R., O'Farrell, A., Faraji, F., Clubb, L., Allevato, M. M., Jensen, S. M., et al. (2022). Lymphatic-preserving treatment sequencing with immune checkpoint inhibition unleashes cDC1-dependent antitumor immunity in HNSCC. Nat. Commun. 13 (1), 4298. doi:10.1038/s41467-022-31941-w
Saeed, S., and Revell, P. A. (2001). Production and distribution of interleukin 15 and its receptors (IL-15Rα and IL-R2β) in the implant interface tissues obtained during revision of failed total joint replacement. Int. J. Exp. Pathol. 82 (3), 201–209. doi:10.1111/j.1365-2613.2001.iep185.x
Sankar, S., O'Neill, K., Bagot D'Arc, M., Rebeca, F., Buffier, M., Aleksi, E., et al. (2021). Clinical use of the self-assembling peptide RADA16: A review of current and future trends in biomedicine. Front. Bioeng. Biotechnol. 9, 679525. doi:10.3389/fbioe.2021.679525
Sathish, J. G., Sethu, S., Bielsky, M. C., de Haan, L., French, N. S., Govindappa, K., et al. (2013). Challenges and approaches for the development of safer immunomodulatory biologics. Nat. Rev. Drug Discov. 12 (4), 306–324. doi:10.1038/nrd3974
Scanlon, S. A. A. (2008). Self-assembling peptide nanotubes. Nano Today 3 (3-4), 22–30. doi:10.1016/S1748-0132(08)70041-0
Seidel, J. A., Otsuka, A., and Kabashima, K. (2018). Anti-PD-1 and anti-CTLA-4 therapies in cancer: Mechanisms of action, efficacy, and limitations. Front. Oncol. 8, 86. doi:10.3389/fonc.2018.00086
Shi, Y., Xie, T., Wang, B., Wang, R., Cai, Y., Yuan, B., et al. (2022). Mutant p53 drives an immune cold tumor immune microenvironment in oral squamous cell carcinoma. Commun. Biol. 5 (1), 757. doi:10.1038/s42003-022-03675-4
Siddiqui, Z., Sarkar, B., Kim, K. K., Kadincesme, N., Paul, R., Kumar, A., et al. (2021). Angiogenic hydrogels for dental pulp revascularization. Acta Biomater. 126, 109–118. doi:10.1016/j.actbio.2021.03.001
Sinha, N. J., Langenstein, M. G., Pochan, D. J., Kloxin, C. J., and Saven, J. G. (2021). Peptide design and self-assembly into targeted nanostructure and functional materials. Chem. Rev. 121 (22), 13915–13935. doi:10.1021/acs.chemrev.1c00712
Sorensen, M. R., Holst, P. J., Steffensen, M. A., Christensen, J. P., and Thomsen, A. R. (2010). Adenoviral vaccination combined with CD40 stimulation and CTLA-4 blockage can lead to complete tumor regression in a murine melanoma model. Vaccine 28 (41), 6757–6764. doi:10.1016/j.vaccine.2010.07.066
Su, J., Hu, B. H., Lowe, W. L., Kaufman, D. B., and Messersmith, P. B. (2010). Anti-inflammatory peptide-functionalized hydrogels for insulin-secreting cell encapsulation. Biomaterials 31 (2), 308–314. doi:10.1016/j.biomaterials.2009.09.045
Sutmuller, R. P., van Duivenvoorde, L. M., van Elsas, A., Schumacher, T. N., Wildenberg, M. E., Allison, J. P., et al. (2001). Synergism of cytotoxic T lymphocyte-associated antigen 4 blockade and depletion of CD25(+) regulatory T cells in antitumor therapy reveals alternative pathways for suppression of autoreactive cytotoxic T lymphocyte responses. J. Exp. Med. 194 (6), 823–832. doi:10.1084/jem.194.6.823
Thakur, R., Suri, C. R., Kaur, I. P., and Rishi, P. (2022). Peptides as diagnostic, therapeutic, and theranostic tools: Progress and future challenges. Crit. Rev. Ther. Drug Carr. Syst. 40 (1), 49–100. doi:10.1615/CritRevTherDrugCarrierSyst.2022040322
Tripathi, J. K., Pal, S., Awasthi, B., Kumar, A., Tandon, A., Mitra, K., et al. (2015). Variants of self-assembling peptide, KLD-12 that show both rapid fracture healing and antimicrobial properties. Biomaterials 56, 92–103. doi:10.1016/j.biomaterials.2015.03.046
Uman, S., Dhand, A., and Burdick, J. A. (2020). Recent advances in shear-thinning and self-healing hydrogels for biomedical applications. J. Appl. Polym. Sci. 137 (25), 48668. doi:10.1002/app.48668
van Elsas, A., Hurwitz, A. A., and Allison, J. P. (1999). Combination immunotherapy of B16 melanoma using anti-cytotoxic T lymphocyte-associated antigen 4 (CTLA-4) and granulocyte/macrophage colony-stimulating factor (GM-CSF)-producing vaccines induces rejection of subcutaneous and metastatic tumors accompanied by autoimmune depigmentation. J. Exp. Med. 190 (3), 355–366. doi:10.1084/jem.190.3.355
Varner, C. T., Rosen, T., Martin, J. T., and Kane, R. S. (2015). Recent advances in engineering polyvalent biological interactions. Biomacromolecules 16 (1), 43–55. doi:10.1021/bm5014469
Waldmann, T. A., Dubois, S., Miljkovic, M. D., and Conlon, K. C. (2020). IL-15 in the combination immunotherapy of cancer. Front. Immunol. 11, 868. doi:10.3389/fimmu.2020.00868
Wang, R., Wang, Z., Guo, Y., Li, H., and Chen, Z. (2019). Design of a RADA16-based self-assembling peptide nanofiber scaffold for biomedical applications. J. Biomater. Sci. Polym. Ed. 30 (9), 713–736. doi:10.1080/09205063.2019.1605868
Wang, X. J., Cheng, J., Zhang, L. Y., and Zhang, J. G. (2022). Self-assembling peptides-based nano-cargos for targeted chemotherapy and immunotherapy of tumors: Recent developments, challenges, and future perspectives. Drug Deliv. 29 (1), 1184–1200. doi:10.1080/10717544.2022.2058647
Wei, S., Ma, J. X., Xu, L., Gu, X. S., and Ma, X. L. (2020). Biodegradable materials for bone defect repair. Mil. Med. Res. 7 (1), 54. doi:10.1186/s40779-020-00280-6
Williams, D. F. (2019). Challenges with the development of biomaterials for sustainable tissue engineering. Front. Bioeng. Biotechnol. 7, 127. doi:10.3389/fbioe.2019.00127
Wu, E. C., Zhang, S., and Hauser, C. A. E. (2012). Self-assembling peptides as cell-interactive scaffolds. Adv. Funct. Mater. 22 (3), 456–468. doi:10.1002/adfm.201101905
Wu, Y., Wen, H., Bernstein, Z. J., Hainline, K. M., Blakney, T. S., Congdon, K. L., et al. (2022). Multiepitope supramolecular peptide nanofibers eliciting coordinated humoral and cellular antitumor immune responses. Sci. Adv. 8 (29), eabm7833. doi:10.1126/sciadv.abm7833
Yang, P., Song, H., Qin, Y., Huang, P., Zhang, C., Kong, D., et al. (2018). Engineering dendritic-cell-based vaccines and PD-1 blockade in self-assembled peptide nanofibrous hydrogel to amplify antitumor T-cell immunity. Nano Lett. 18 (7), 4377–4385. doi:10.1021/acs.nanolett.8b01406
Zhang, L., Wang, J., Xu, A., Zhong, C., Lu, W., Deng, L., et al. (2016). A rationally designed TNF-alpha epitope-scaffold immunogen induces sustained antibody response and alleviates collagen-induced arthritis in mice. PLoS One 11 (9), e0163080. doi:10.1371/journal.pone.0163080
Zhang, R., Billingsley, M. M., and Mitchell, M. J. (2018). Biomaterials for vaccine-based cancer immunotherapy. J. Control Release 292, 256–276. doi:10.1016/j.jconrel.2018.10.008
Zhang, X. M., and Xu, Q. (2001). Metastatic melanoma cells escape from immunosurveillance through the novel mechanism of releasing nitric oxide to induce dysfunction of immunocytes. Melanoma Res. 11 (6), 559–567. doi:10.1097/00008390-200112000-00002
Keywords: biomaterials, self-assembling peptides (SAP), cancer, immunotherapy, localized delivery
Citation: Hernandez A, Hartgerink JD and Young S (2023) Self-assembling peptides as immunomodulatory biomaterials. Front. Bioeng. Biotechnol. 11:1139782. doi: 10.3389/fbioe.2023.1139782
Received: 07 January 2023; Accepted: 20 February 2023;
Published: 01 March 2023.
Edited by:
Amanda Acevedo-Jake, University of Leeds, United KingdomReviewed by:
Nikhil Pandey, University of Maryland, United StatesSiyu Shi, Stanford Healthcare, United States
Copyright © 2023 Hernandez, Hartgerink and Young. This is an open-access article distributed under the terms of the Creative Commons Attribution License (CC BY). The use, distribution or reproduction in other forums is permitted, provided the original author(s) and the copyright owner(s) are credited and that the original publication in this journal is cited, in accordance with accepted academic practice. No use, distribution or reproduction is permitted which does not comply with these terms.
*Correspondence: Simon Young, c2ltb24ueW91bmdAdXRoLnRtYy5lZHU=