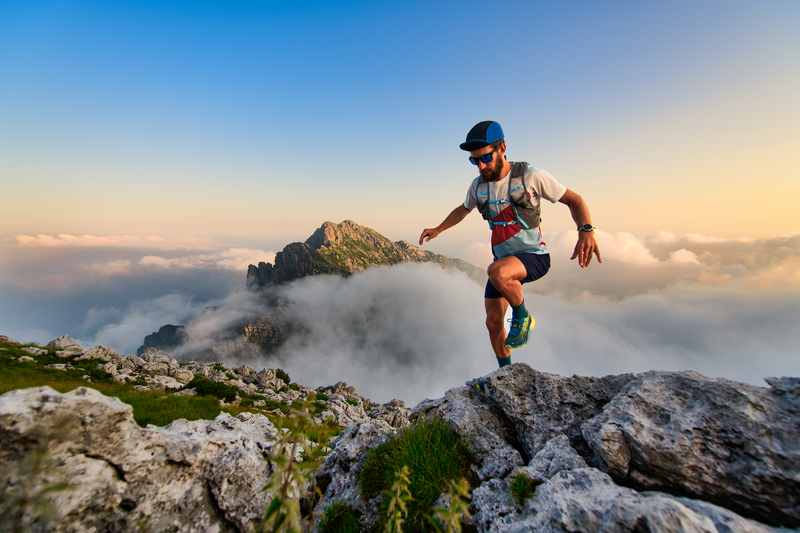
95% of researchers rate our articles as excellent or good
Learn more about the work of our research integrity team to safeguard the quality of each article we publish.
Find out more
ORIGINAL RESEARCH article
Front. Bioeng. Biotechnol. , 24 August 2022
Sec. Biosensors and Biomolecular Electronics
Volume 10 - 2022 | https://doi.org/10.3389/fbioe.2022.969870
This article is part of the Research Topic Nanomaterials-Based Electrochemical Biosensors View all 6 articles
The H2S concentration in exhaled breath increases marginally with the progress of periodontal disease, and H2S is considered to be one of the most important gases related to meat and seafood decomposition; however, the concentration of H2S is low and difficult to detect in such scenarios. In this study, Au–PrFeO3 nanocrystalline powders with high specific surface areas and porosities were prepared using an electrospinning method. Our experimental results show that loading Au on the material provides an effective way to increase its gas sensitivity. Au doping can decrease the material’s resistance by adjusting its energy band, allowing more oxygen ions to be adsorbed onto the material’s surface due to a spillover effect. Compared with pure PrFeO3, the response of 3 wt% Au–PrFeO3 is improved by more than 10 times, and the response time is more than 10 s shorter. In addition, the concentration of H2S due to the decomposition of shrimp was detected using the designed gas sensor, where the error was less than 15%, compared with that obtained using a GC-MS method. This study fully demonstrates the potential of Au–PrFeO3 for H2S concentration detection.
H2S is a colorless, highly toxic, and acidic gas. It has a particular rotten egg smell, and even low concentrations of H2S can impair the human sense of smell. In high concentrations, it has no smell (as high concentrations paralyze the olfactory nerve). In addition, H2S is flammable and is typically considered a dangerous gas (Ethiraj et al., 2015; Wu et al., 2019; Kumar et al., 2021; Liu et al., 2021; Priya et al., 2021; Zheng et al., 2021; Zuo et al., 2021; Li et al., 2022a). H2S gas is released during the breakdown of food, and is also responsible for the bad breath caused by periodontitis (Chen et al., 2017; Wu et al., 2019; Hsu et al., 2021; Lopez et al., 2021): about 0.195 ppm H2S can be detected in the exhaled breath of periodontitis patients, while 0.105 ppm is a typical concentration in the exhaled breath of healthy individuals (Yaegaki and Sanada, 1992). Using the nose as a means of detecting H2S can be fatal. Therefore, the timely detection of very low concentrations of H2S gas is very necessary and important.
In recent years, the use of MOS (metal oxide semiconductor) gas sensors to detect the concentration of target gases has become increasingly popular, such as smoke sensors in hotels, natural gas alarms in homes, and so on. It has been reported that some MOSs, as gas-sensing materials, show excellent response to gases, such as LaFeO3 (Xiangfeng and Siciliano, 2003; Song et al., 2014; Jaouali et al., 2018; Ma et al., 2021), SmFeO3 (Tomoda et al., 2004; Hosoya et al., 2005; Huang et al., 2018; Han et al., 2020), PrFeO3 (Ma et al., 2018), HoFeO3 (Song et al., 2020), NdFeO3 (Sheng et al., 2022), YCoO3 (Addabbo et al., 2015), BaSnO3 (Cerdà et al., 2002), ZnSnO3 (Yin et al., 2020), and YMnO3 (Balamurugan and Lee, 2015). For H2S, commonly used gas-sensing materials include Pt–ZnO (Zhou et al., 2022), Pd–ZnO (Bae et al., 2022), CuO/SnO2 (Fan et al., 2019), Pt–WO3 (Yao et al., 2022), WO3 (Wang et al., 2018; Akamatsu et al., 2021; Li et al., 2022b), Pt–Fe2O3 (Guo et al., 2018), CuO/CuFe2O4(Lim et al., 2021), Ag–SnO2 (Senapati and Sahu, 2020), LaFeO3 (Xiangfeng and Siciliano, 2003), YMnO3 (Balamurugan and Lee, 2015), and Sn–NiO (Gao et al., 2017), among others. MOSs—especially ABO3 perovskite materials—have the unique advantages of large specific surface area and abundant active sites, which can promote the diffusion path and increase the adsorption of target gas molecules, thus enhancing the sensing ability. There are other ways to detect a target gas, such as Tamm plasmon resonance (Mehaney et al., 2021) and photonic crystal (Ahmed et al., 2021; Ameen et al., 2021; Alrowaili et al., 2022). In particular, these two methods have high accuracy in detecting the gases in exhaled breath, and have good development prospect.
In recent years, sensors based on Graphene and MWCNT have been widely reported, especially for gases in exhaled breath or aromatic gases (Behi et al., 2020, 2022; Thamri et al., 2021). Such sensors display high response and selectivity to target gases; moreover, they have good development prospect due to their low preparation costs.
The aim of this study is to obtain a gas-sensing material with high response, high selectivity, low detection limit, and high long-term stability. PrFeO3 with different Au doping levels was synthesized using an electrospinning method and sintered at 800°C. It has high specific surface area and high porosity, which are two important factors for improving the gas response of gas-sensing materials. Compared with PrFeO3, Au–PrFeO3 shows a higher response and high selectivity for H2S. In addition, Au doping, as a catalyst, can greatly enhance the surface activity of gas-sensing materials, thus shortening the response-recovery time. Finally, the H2S concentration in the air around shrimp is detected using the gas sensor designed in this study, which was compared with the data obtained by GC-MS, showing that the error was within 15%. The experimental results prove that Au doping can greatly improve the response of PrFeO3 to H2S gas, providing a feasible and effective way to detect H2S gas using a gas sensor.
First, samarium oxide, ferric nitrate, palladium chloride, DMF (99.5%), PVP (Mw = 1,300,000), C2H5OH (99.7%), and HNO3 in a stoichiometric ratio were weighed and mixed in deionized water (Figure 1A). The mixed solution was heated in the water bath at 60°C with magnetic stirring until it became transparent, in order to obtain the electrospinning precursor solution (Figure 1B). Then, the as-prepared precursor solution was transferred into a 10 mL syringe. The voltage was maintained at 12 kV during the spinning process, the distance between needle tip and the collector was about 20 cm, and the injection rate of the syringe was 0.4 mL/h (Figure 1C). The obtained nanofibers were sintered at 800°C for 6 h in a muffle furnace (Figure 1D). Finally, the X (0, 1, 3, 5) wt% Au–PrFeO3 powder was obtained.
The Au–PrFeO3 powder was mixed with deionized water to make a paste, which was then placed on gas-sensing film (Figure 1E). The as-prepared plane electrode plate was aged on an aging platform for 48 h, in order to dry it out. At this time, a qualified sensor was ready. The front side of the electrode plate has two electrodes, which are used to detect the resistance value of the gas-sensing material. On the back of electrode plate is a heating plate, which enables the gas-sensing material to reach a higher operating temperature.
The gas sensor structure diagram is shown in Figure 1E. The Au–PrFeO3 is coated onto the sensing film. The
The gas-sensing response, S, is defined as
The whole experiment was carried out in a closed glass chamber, into which H2S gas was injected with a microinjector. The injection amount of H2S liquid was calculated as follows (Deng et al., 2013):
where
Figure 2A shows the X-ray diffraction analysis (XRD; Bruker D8 ADVANCE with the CuKα amount of 1.5405 Å at 40 kV and 40 mA) results of X (0, 1, 3, 5) wt% Au–PrFeO3. Compared with the standard card (PDF card: 37-1493), it shows a single-phase. The average particle size can be calculated using the Scherrer method. The Scherrer equation is as follows:
where
FIGURE 2. (A) The XRD pattern of X (0–5) wt% Au-PrFeO3; (B) The XPS survey of 3 wt% Au-PrFeO3; (C–F) The fine spectra of XPS analysis for each element (Au, Pr, Fe, O).
Figures 3A,B shows the Scanning Electron Microscope (SEM; Japan HITACHI SU8010, 8.0 kV) images of 3 wt% Au–PrFeO3 under different magnifications (PrFeO3 was synthesized by a sol-gel method and sintered at 800°C; Supplementary Figure S1). The pure PrFeO3 presented a common perovskite structure, while 3 wt% Au–PrFeO3 presented a nanotube-like microstructure. In the material preparation stage, after sintering, the surface of the material becomes rough and the nanotubes become hollow as the PVP decomposes at high temperature.
FIGURE 3. (A,B) The SEM of 3 wt% Au-PrFeO3; (C) N2 adsorption–desorption isotherms and pore size distributions (the inset) for Au-PrFeO3 nanocomposite; (D) The surface area of PrFeO3 with different amount of Au doping.
In order to understand which microstructure provides more favorable properties to the gas-sensing material, it is necessary to figure out which structure has higher specific surface area and porosity. The specific surface area and porosity of the 3 wt% Au–PrFeO3 hollow nanofibers were further analyzed by nitrogen adsorption–desorption analysis. Figure 3C shows the BET curves for 3 wt% Au–PrFeO3 and the corresponding Barrett–Joyner–Halenda (BJH) pore size distribution (inset). The specific surface area of 3 wt% Au–PrFeO3 was 23.67 m2/g and the average pore size was 10.2 nm. The specific surface areas of PrFeO3 with different amounts of Au element doping are shown in Figure 3D. It can be seen that, when the doping amount of Au element was 3 wt%, the composite powder presented the largest specific surface area. This occurred as Au doping can inhibit the growth of MOS grains (the smaller the grain size, the larger the specific surface area); however, when the Au doping amount is too high, the particles will appear in a small range of agglomeration, and the specific surface area of the material will decreased. Considering the sensing properties of materials, the specific surface area is an important factor. A high specific surface area can provide more adsorption sites, which can enhance the reactions between the sensing material and gas molecules, leading to a high response to the test gas.
Figure 4A and Supplementary Figure S2 show the response curves of PrFeO3 with different amount of Au doping to 1 ppm H2S at various operating temperatures. For all samples, the highest responses were obtained at 120°C. The highest responses to 1 ppm H2S were 6.93 (0 wt% Au), 38.16 (1 wt% Au), 72.86 (3 wt% Au), and 56.29 (5 wt% Au). It can be seen that the response was more than 10 times higher when using the best Au-doped sample, compared with the pure sample. Moreover, Table 1 shows the H2S sensing properties of some typical gas-sensing materials for reference. By comparison, 3 wt% Au–PrFeO3 exhibited an extremely high response value while ensuring a short response–recovery time.
FIGURE 4. (A) The response of PrFeO3 with Au doping; (B) The relationship between the response of Au-PrFeO3 and multiple H2S concentration.
The relationship between the material’s sensitivity and the gas concentration is very important, and a good fitting relationship can be used to predict the response value at a given gas concentration. Figure 4B and Supplementary Figure S3 show the relationship between the response of Au–PrFeO3 and multiple H2S concentrations. It can be seen that, for both undoped and Au–doped PrFeO3, the response had a good linear relationship with the gas concentration, with all
Repeatability is another important property that determines whether a gas-sensing material is excellent or not. For Au–PrFeO3, the repeatability of responses to different concentrations of H2S gas are shown in Figures 5A–C and Supplementary Figure S4. The repeated processes were carried out as follows: when the resistance value of the gas-sensing material had stabilized, the H2S gas was injected into the reaction chamber, and the resistance of the material increased immediately. After a period of time, the resistance stabilized, following which the H2S gas is removed and the resistance of the material decreased immediately, restoring it to the initial state. It can be seen that, for H2S gas at different concentrations, the resistance of the gas-sensing material could be restored to the initial value every time after the H2S gas was removed, indicating that the material has excellent repeatability. Additionally, the response of all samples changed upon exposure to 1 ppm H2S gas, as shown in Figures 5D–F. It can be seen that the gas response of samples had no obvious change after a 3-cycle response–recovery test, indicating the high operating stability of the designed Au–PrFeO3 sensor. Additionally, the gas-sensing reproducibility of Au-PrFeO3 is about 38.16
FIGURE 5. (A–C) The repeatability of responses of PrFeO3 with Au doping to different concentration of H2S gas; (D–F); The repeatability of responses of PrFeO3 with Au doping to 1 ppm H2S gas; (G–I) The response-recovery time of Au-PrFeO3 to 1 ppm H2S.
The response–recovery time of all samples differed at different operating temperatures, indicating that the operating temperature affects the chemical reaction on the material’s surface. The response–recovery times of all samples are shown in Supplementary Table S1, Figures 5G–I, and Supplementary Figure S5. It can be seen that the response–recovery time increased with the operating temperature up to 120°C; then, after 120°C, the response–recovery time decreased with any further increase in the operating temperature. This may be due to the fact that, before the optimum operating temperature, the adsorption rate of gas molecules is higher than the desorption rate, and the number of oxygen ions and H2S gas molecules adsorbed on the material’s surface are increased, leading to an increased reaction time. With an increase in the operating temperature, the adsorption and desorption rates are balanced at the optimum operating temperature, and the number of H2S gas molecules and adsorbed oxygen ions on the material’s surface reach a maximum. At this operating temperature, the reaction time also reaches its maximum. With a further increase in operating temperature, the desorption rate of gas molecules is higher than the adsorption rate, the reaction reactants become less, and the reaction time is shortened. In addition, Au doping can increase the surface activity of the material and improve the reaction rate; therefore, the response–recovery time of Au–PrFeO3 was shorter than that of pure PrFeO3.
In practical application, it is very common to detect a certain gas in a mixture, such as H2S gas in an individual’s exhaled breath. Therefore, the selectivity of a gas-sensing material to a certain gas determines its practical application value. The selectivity comparison of Au–PrFeO3 to 1 ppm H2S and several other common gases in a person’s exhaled breath is shown in Figures 6A–C and Supplementary Figure S6. It can be seen that, compared with other gases, Au–PrFeO3 presented high selectivity for H2S gas. In particular, for N2, O2, NO, CO2, CO, and other common gases present in exhaled breath, the response was negligible, such that the H2S in the exhaled breath can be detected more accurately.
FIGURE 6. (A–C) The selectivity comparison of Au-PrFeO3 to 1 ppm H2S and several other common gases.
The relative humidity (RH) in the environment is also a factor that cannot be ignored in the application of gas sensors. Figures 7A and Supplementary Figure S7 show the responses of Au–PrFeO3 to 1 ppm H2S with varying RH. It can be seen that the response decreased with increasing RH: before 50% RH, the response was little affected by it; however, above 50% RH, the responses decreased sharply. This means that the gas sensor in this study can be used in a low-RH environment without considering the influence of RH. This will greatly expand its practical application field. Figures 7B and Supplementary Figure S8 show the resistance change of Au–PrFeO3 with RH. For Au–PrFeO3, the resistance decreased with RH, but the proportion of decrease differed. In the 20–90% RH range, the proportion of decreases were 53.21% (0 wt% Au), 48.6% (1 wt% Au), 41.8% (3 wt% Au), and 47.09% (5 wt% Au). Thus, the resistance of 3 wt% Au–PrFeO3 presented the highest RH adaptability.
FIGURE 7. (A) The response changing of Au-PrFeO3 with RH; (B) The resistance changing of Au-PrFeO3 with RH; (C–F) The long-term stability of responses of Au-PrFeO3 under different RH.
Long-term stability is another important property for gas-sensing materials. The higher the long-term stability, the longer the replacement cycle of the gas-sensing material and, so, the more economic and energy advantages it has. Figures 7C–F show the long-term stability of Au–PrFeO3 under different RH over 30 days. The experimental data were obtained every 2 days. It can be seen that all of the responses decreased slightly with time, but the proportion of decrease was lowest when the sensor was kept at under 20% RH. The proportions of decrease when the sensor was kept at under 20% RH were 34.9% (0 wt% Au), 13.3% (1 wt% Au), 3.7% (3 wt% Au), and 5.7% (5 wt% Au). It can be seen that the long-term stability of 3 wt% Au–PrFeO3 was more than 9 times that of pure PrFeO3. Therefore,Au-doped PrFeO3 demonstrated advantages, in terms of long-term stability. Other types of sensors, such as MOX (Pashami et al., 2012; Li et al., 2021) and MWCNT (Pistone et al., 2013; Barthwal and Singh, 2020) have been shown to have good stability under high RH environments. However, MOS, MOX, and MWCNT gas sensors are affected by RH in practical applications; therefore, improving their RH adaptability is a keyway to broaden their application field.
Figure 8 shows the reaction mechanism for the experiment conducted in this work. At room temperature (20°C), for a p-type semiconductor, the main carrier of Au–PrFeO3 is the hole (
FIGURE 8. The reaction mechanism of the whole experiment in this work. (A) The hole is the main carriers in Au-PrFeO3; (B) At high operating temperatures, the oxygen molecules capture electrons from the surface of the Au-PrFeO3; (C) The resistance changing of Au-PrFeO3 at any operating temperature; (D) At high operating temperatures, the H2S gas molecules react with oxygen ions on the surface of the Au-PrFeO3; (E) At high operating temperatures, the response increase with the concentration of H2S gas molecules.
Before Au doping, few oxygen molecules capture the free electrons from the material, resulting in the formation of oxygen ions on the material’s surface and few holes are created in this process at the same time. As the work function of Au is larger than that of PrFeO3, electrons will transfer from PrFeO3 to the surrounding Au nanoparticles after Au doping, resulting in an increase in the number of holes in PrFeO3 (Figure 8B). This reaction may look like:
where ads denote the state where oxygen is adsorbed on the material surface.
In order to verify this theoretical assumption, the resistances of pure PrFeO3 and 3 wt% Au–PrFeO3 were tested, and the results are shown in Figure 8C. It can be seen that the resistance of 3 wt% Au–PrFeO3 was lower than that of PrFeO3 at any operating temperature, consistent with the above theoretical assumption.
When the H2S gas molecule is introduced, it will be adsorbed onto the surface of the PrFeO3 to react with the oxygen ions (Figure 8D). The adsorption and desorption on the surface of Au–PrFeO3 of H2S gas molecules exist simultaneously. The rates of adsorption and desorption increase with the operating temperature, where the rate of adsorption is greater than the rate of desorption before the operating temperature reaches the optimum temperature. Therefore, the count of adsorbed H2S molecules on the surface of the material increases, and the reaction between H2S molecules and oxygen ions is more intense, resulting in an increased response. When the operating temperature exceeds the optimum temperature, the rate of adsorption of Au–PrFeO3 with respect to H2S molecules is lower than the rate of desorption and the intensity of the reaction between H2S molecule and oxygen ions is reduced, causing the response to decrease. Furthermore, at the optimum temperature, as the concentration of H2S gas molecule increases, the number of H2S molecules adsorbed on the surface of the Au–PrFeO3 will increase, causing the response to increase (Figure 8E). However, the number of free electrons on the surface of the Au–PrFeO3 is not infinite, and the energy required to make an electronic transition within Au–PrFeO3 is also increasing. Therefore, the response (
The reaction between H2S molecules and oxygen ions may as follows:
Additionally, it is well-known that the oxygen in air can be adsorbed onto the surface of semiconductor metal oxides to become oxygen ions, for which Au is a good catalyst. In this work, with the assistance of Au, oxygen molecules can more easily be adsorbed onto the surface of PrFeO3, due to the spillover effect (Kung et al., 2007; Wang et al., 2013). For this reason, more oxygen gets adsorbed and captures free electrons to form oxygen ionic species (Liu et al., 2011). This process increases both the quantity of adsorbed oxygen and the molecule–ion conversion rate, resulting in a high gas response (Wang et al., 2012).
Accurately and quickly assessing whether meat and seafood have decomposed or not is very important. H2S is thought to be one of the most important gases released in the decomposition of food. The H2S concentration around shrimp with time was detected using the gas sensor designed in this study and GC-MS, as shown in Figure 9. Eight shrimps were placed in the experimental apparatus, each about 10–16 cm in length. It can be seen that the concentration of H2S increased with death-time, and the concentration of H2S measured by the designed gas sensor was greater than that measured by GC-MS at any time, which indicates that there were other gases in the surrounding air of the shrimp, which can have an effect on the gas sensor; however, this effect was very small. By comparing the H2S concentrations measured by the two methods, the error was within 10%. The results are provided in Table 3.
In this study, Au-modified PrFeO3 was synthesized using an electrospinning method. It has a large specific surface area and high porosity, which improved the response to a certain extent. Our experimental results demonstrated that the optimum Au doping content was 3 wt%. The response of 3 wt% Au–PrFeO3 to H2S was more than 10 times higher, and its long-term stability was more than 9 times that of pure PrFeO3. Moreover, the response–recovery time of 3 wt% Au–PrFeO3 was more than 10 s shorter than that of the pure PrFeO3. In addition, the doping of Au, as a catalyst, greatly improved the RH adaptability and selectivity of the material. Finally, the designed Au–PrFeO3 was shown to be very accurate for detecting the concentration of H2S gas in the air around shrimp, with an error of less than 15%, when compared to the results obtained by GC-MS. Our experimental results fully demonstrate the advantages of noble metal doping in improving the performance of gas-sensing materials and the great potential of Au–PrFeO3 in H2S detection.
The raw data supporting the conclusions of this article will be made available by the authors, without undue reservation.
HZ, YZ, LZ, JC, and JX designed the study. HZ performed experiments and analyzed data. HZ, JC, JX, and PJ wrote and revised the manuscript. HZ, JC, JX, and PJ provided an experimental resource.
This work was supported by National Natural Science Foundation of China (81860386), Guangxi Natural Science Foundation (2020GXNSFBA238016), Shandong Natural Science Foundation (No. ZR2021QE265), the Fundamental Research Funds of Taishan University (No. Y-01-2020015), National Natural Science Foundation of China (Nos. 61574098 and 61204051), the Shandong Province Key Research and Development Program (No. 2019GGX101016), Yangtze University Medical Innovation Fund (2022MIF03), and Innovation and Entrepreneurship Training program for university students of Yangtze University (Yz2021292).
The authors declare that the research was conducted in the absence of any commercial or financial relationships that could be construed as a potential conflict of interest.
All claims expressed in this article are solely those of the authors and do not necessarily represent those of their affiliated organizations, or those of the publisher, the editors and the reviewers. Any product that may be evaluated in this article, or claim that may be made by its manufacturer, is not guaranteed or endorsed by the publisher.
The Supplementary Material for this article can be found online at: https://www.frontiersin.org/articles/10.3389/fbioe.2022.969870/full#supplementary-material
Addabbo, T., Bertocci, F., Fort, A., Gregorkiewitz, M., Mugnaini, M., Spinicci, R., et al. (2015). Gas sensing properties and modeling of YCoO3 based perovskite materials. Sensors Actuators B Chem. 221, 1137–1155. doi:10.1016/j.snb.2015.07.079
Ahmed, A. M., Mehaney, A., and Elsayed, H. A. (2021). Detection of toluene traces in exhaled breath by using a 1D PC as a biomarker for lung cancer diagnosis. Eur. Phys. J. Plus 136, 626. doi:10.1140/epjp/s13360-021-01621-7
Akamatsu, T., Itoh, T., Tsuruta, A., and Masuda, Y. (2021). CH3SH and H2S sensing properties of V2O5/WO3/TiO2 gas sensor. Chemosensors 9, 113. doi:10.3390/chemosensors9050113
Alrowaili, Z. A., Elsayed, H. A., Ahmed, A. M., Taha, T. A., and Mehaney, A. (2022). Simple, efficient and accurate method toward the monitoring of ethyl butanoate traces. Opt. Quantum Electron. 54, 126. doi:10.1007/s11082-021-03497-4
Ameen, A. A., Elsayed, H., and Aly, A. H. (2021). Towards a highly efficient air purifier using annular photonic crystals in UV regimes. RSC Adv. 11, 14915–14921. doi:10.1039/d1ra00991e
Bae, G., Kim, M., Lee, A., Ji, S., Jang, M., Yim, S., et al. (2022). Nanometric lamination of zinc oxide nanofilms with gold nanoparticles for self-perceived periodontal disease sensors. Compos. Part B Eng. 230, 109490. doi:10.1016/j.compositesb.2021.109490
Balamurugan, C., and Lee, D.-W. (2015). Perovskite hexagonal YMnO3 nanopowder as p-type semiconductor gas sensor for H2S detection. Sensors Actuators B Chem. 221, 857–866. doi:10.1016/j.snb.2015.07.018
Barthwal, S., and Singh, N. B. (2020). Urea detection by ZnO-MWCNT nanocomposite sensor. Mater. Today Proc. 29, 749–752. doi:10.1016/j.matpr.2020.04.514
Behi, S., Bohli, N., Casanova-Cháfer, J., Llobet, E., and Abdelghani, A. (2020). Metal oxide nanoparticle-decorated few layer Graphene nanoflake chemoresistors for the detection of aromatic volatile organic compounds. Sensors 20, 3413. doi:10.3390/s20123413
Behi, S., Casanova-Chafer, J., González, E., Bohli, N., Llobet, E., and Abdelghani, A. (2022). Metal loaded nano-carbon gas sensor array for pollutant detection. Nanotechnology 33, 195501. doi:10.1088/1361-6528/ac4e43
Cerdà, J., Arbiol, J., Dezanneau, G., Dı́az, R., and Morante, J. R. (2002). Perovskite-type BaSnO3 powders for high temperature gas sensor applications. Sensors Actuators B Chem. 84, 21–25. doi:10.1016/S0925-4005(02)00005-9
Chen, L., Luque, R., and Li, Y. (2017). Controllable design of tunable nanostructures inside metal–organic frameworks. Chem. Soc. Rev. 46, 4614–4630. doi:10.1039/C6CS00537C
Deng, X., Sang, S., Li, P., Li, G., Gao, F., Sun, Y., et al. (2013). Preparation, characterization, and mechanistic understanding of Pd-decorated ZnO nanowires for ethanol sensing. J. Nanomater. 2013, 1–8. doi:10.1155/2013/297676
Ethiraj, J., Bonino, F., Lamberti, C., and Bordiga, S. (2015). H2S interaction with HKUST-1 and ZIF-8 MOFs: A multitechnique study. Microporous Mesoporous Mat. 207, 90–94. doi:10.1016/j.micromeso.2014.12.034
Fan, J., Liu, P., Chen, X., Zhou, H., Fu, S., and Wu, W. (2019). Carbon nanotubes-CuO/SnO2 based gas sensor for detecting H2S in low concentration. Nanotechnology 30, 475501. doi:10.1088/1361-6528/ab3cb3
Gao, H., Wei, D., Lin, P., Liu, C., Sun, P., Shimanoe, K., et al. (2017). The design of excellent xylene gas sensor using Sn-doped NiO hierarchical nanostructure. Sensors Actuators B Chem. 253, 1152–1162. doi:10.1016/j.snb.2017.06.177
Guo, L., Xie, N., Wang, C., Kou, X., Ding, M., Zhang, H., et al. (2018). Enhanced hydrogen sulfide sensing properties of Pt-functionalized α-Fe2O3 nanowires prepared by one-step electrospinning. Sensors Actuators B Chem. 255, 1015–1023. doi:10.1016/j.snb.2017.07.055
Han, T., Ma, S. Y., Xu, X. L., Xu, X. H., Pei, S. T., Tie, Y., et al. (2020). Rough SmFeO3 nanofibers as an optimization ethylene glycol gas sensor prepared by electrospinning. Mat. Lett. 268, 127575. doi:10.1016/j.matlet.2020.127575
Hosoya, Y., Itagaki, Y., Aono, H., and Sadaoka, Y. (2005). Ozone detection in air using SmFeO3 gas sensor. Sensors Actuators B Chem. 108, 198–201. doi:10.1016/j.snb.2004.10.059
Hsu, K.-C., Fang, T.-H., Hsiao, Y.-J., and Li, Z.-J. (2021). Rapid detection of low concentrations of H2S using CuO-doped ZnO nanofibers. J. Alloys Compd. 852, 157014. doi:10.1016/j.jallcom.2020.157014
Huang, H. T., Zhang, W. L., Zhang, X. D., and Guo, X. (2018). NO2 sensing properties of SmFeO3 porous hollow microspheres. Sensors Actuators B Chem. 265, 443–451. doi:10.1016/j.snb.2018.03.073
Jaouali, I., Hamrouni, H., Moussa, N., Nsib, M. F., Centeno, M. A., Bonavita, A., et al. (2018). LaFeO3 ceramics as selective oxygen sensors at mild temperature. Ceram. Int. 44, 4183–4189. doi:10.1016/j.ceramint.2017.11.221
Kumar, V., Majhi, S. M., Kim, K.-H., Kim, H. W., and Kwon, E. E. (2021). Advances in In2O3-based materials for the development of hydrogen sulfide sensors. Chem. Eng. J. 404, 126472. doi:10.1016/j.cej.2020.126472
Kung, M. C., Davis, R. J., and Kung, H. H. (2007). Understanding Au-catalyzed low-temperature CO oxidation. J. Phys. Chem. C 111, 11767–11775. doi:10.1021/jp072102i
Li, W., Huang, L., Wang, T., Hao, X., Wang, B., Lu, Q., et al. (2021). Based Nafion gas sensor utilizing Pt-MOx (MOx = SnO2, In2O3, CuO) sensing electrode for CH3OH detection at room temperature in FCVs. Sensors Actuators B Chem. 346, 130543. doi:10.1016/j.snb.2021.130543
Li, X., Hu, J., Ban, J., He, S., Zheng, N., Shao, G., et al. (2022a). Mechanism of enhanced H2S sensor ability based on emerging Li0.5La0.5TiO3-SnO2 core-shell structure. Sensors Actuators B Chem. 352, 131054. doi:10.1016/j.snb.2021.131054
Li, X., Yang, H., Wang, X., Qin, Z., Hu, X., Wang, X., et al. (2022b). Exposed edges of porous ultrathin WO3 nanosheets determined High-performance sensing for hydrogen sulfide. Appl. Surf. Sci. 571, 151327. doi:10.1016/j.apsusc.2021.151327
Lim, K., Jo, Y. M., Kim, S., Yoon, J. W., Jeong, S. Y., Kim, J. S., et al. (2021). Selective dual detection of hydrogen sulfide and methyl mercaptan using CuO/CuFe2O4 nanopattern chemiresistors. Sensors Actuators B Chem. 348, 130665. doi:10.1016/j.snb.2021.130665
Liu, X., Zhang, J., Wang, L., Yang, T., Guo, X., Wu, S., et al. (2011). 3D hierarchically porous ZnO structures and their functionalization by Au nanoparticles for gas sensors. J. Mat. Chem. 21, 349–356. doi:10.1039/c0jm01800g
Liu, B., Zhang, L., Luo, Y., Gao, L., and Duan, G. (2021). The dehydrogenation of HS bond into sulfur species on supported Pd single atoms allows highly selective and sensitive hydrogen sulfide detection. Small 17, 2105643. doi:10.1002/smll.202105643
Lopez, J. D., Keley, M., Dante, A., and Werneck, M. M. (2021). Optical fiber sensor coated with copper and iron oxide nanoparticles for hydrogen sulfide sensing. Opt. Fiber Technol. 67, 102731. doi:10.1016/j.yofte.2021.102731
Ma, L., Ma, S. Y., Shen, X. F., Wang, T. T., Jiang, X. H., Chen, Q., et al. (2018). PrFeO3 hollow nanofibers as a highly efficient gas sensor for acetone detection. Sensors Actuators B Chem. 255, 2546–2554. doi:10.1016/j.snb.2017.09.060
Ma, Z., Yang, K., Xiao, C., and Jia, L. (2021). C-doped LaFeO3 porous nanostructures for highly selective detection of formaldehyde. Sensors Actuators B Chem. 347, 130550. doi:10.1016/j.snb.2021.130550
Mehaney, A., Alrowaili, Z. A., Elsayed, H. A., Taha, T. A., and Ahmed, A. M. (2021). Theoretical investigations of Tamm plasmon resonance for monitoring of isoprene traces in the exhaled breath: Towards chronic liver fibrosis disease biomarkers. Phys. Lett. A 413, 127610. doi:10.1016/j.physleta.2021.127610
Mehta, S. S., Nadargi, D. Y., Tamboli, M. S., Alshahrani, T., Minnam Reddy, V. R., Kim, E. S., et al. (2021). RGO/WO3 hierarchical architectures for improved H2S sensing and highly efficient solar-driving photo-degradation of RhB dye. Sci. Rep. 11, 5023. doi:10.1038/s41598-021-84416-1
Pan, W., Zhang, Y., Yu, S., Liu, X., and Zhang, D. (2021). Hydrogen sulfide gas sensing properties of metal organic framework-derived α-Fe2O3 hollow nanospheres decorated with MoSe2 nanoflowers. Sensors Actuators B Chem. 344, 130221. doi:10.1016/j.snb.2021.130221
Pashami, S., Lilienthal, A., and Trincavelli, M. (2012). Detecting changes of a distant gas source with an array of MOX gas sensors. Sensors 12, 16404–16419. doi:10.3390/s121216404
Peng, F., Yu, W., Lu, Y., Sun, Y., Fu, X., Hao, J. M., et al. (2020). Enhancement of low-temperature gas-sensing performance using substoichiometric WO3–x modified with CuO. ACS Appl. Mat. Interfaces 12, 41230–41238. doi:10.1021/acsami.0c09213
Pistone, A., Piperno, A., Iannazzo, D., Donato, N., Latino, M., Spadaro, D., et al. (2013). Fe3O4–MWCNTPhCOOH composites for ammonia resistive sensors. Sensors Actuators B Chem. 186, 333–342. doi:10.1016/j.snb.2013.06.027
Priya, A. K., Suresh, R., Kumar, P. S., Rajendran, S., Vo, D.-V. N., and Soto-Moscoso, M. (2021). A review on recent advancements in photocatalytic remediation for harmful inorganic and organic gases. Chemosphere 284, 131344. doi:10.1016/j.chemosphere.2021.131344
Senapati, M., and Sahu, P. P. (2020). Meat quality assessment using Au patch electrode Ag-SnO2/SiO2/Si MIS capacitive gas sensor at room temperature. Food Chem. 324, 126893. doi:10.1016/j.foodchem.2020.126893
Sheng, H., Ma, S., Han, T., Yun, P., Yang, T., and Ren, J. (2022). A highly sensitivity and anti-humidity gas sensor for ethanol detection with NdFeO3 nano-coral granules. Vacuum 195, 110642. doi:10.1016/j.vacuum.2021.110642
Song, P., Zhang, H., Han, D., Li, J., Yang, Z., and Wang, Q. (2014). Preparation of biomorphic porous LaFeO3 by sorghum straw biotemplate method and its acetone sensing properties. Sensors Actuators B Chem. 196, 140–146. doi:10.1016/j.snb.2014.02.006
Song, Y., Zhang, Y., Ma, M., Ren, J., Liu, C., and Tan, J. (2020). Visible light-assisted formaldehyde sensor based on HoFeO3 nanoparticles with sub-ppm detection limit. Ceram. Int. 46, 16337–16344. doi:10.1016/j.ceramint.2020.03.191
Thamri, A., Baccar, H., Casanova-Chafer, J., Mejri, M. B., Llobet, E., and Abdelghani, A. (2021). Thiol-amine functionalized decorated carbon nanotubes for biomarker gases detection. Chemosensors 9, 87. doi:10.3390/chemosensors9050087
Tomoda, M., Okano, S., Itagaki, Y., Aono, H., and Sadaoka, Y. (2004). Air quality prediction by using semiconducting gas sensor with newly fabricated SmFeO3 film. Sensors Actuators B Chem. 97, 190–197. doi:10.1016/j.snb.2003.08.013
Wang, L., Lou, Z., Fei, T., and Zhang, T. (2012). Templating synthesis of ZnO hollow nanospheres loaded with Au nanoparticles and their enhanced gas sensing properties. J. Mat. Chem. 22, 4767–4771. doi:10.1039/c2jm15342d
Wang, L., Dou, H., Lou, Z., and Zhang, T. (2013). Encapsuled nanoreactors (Au@SnO2): a new sensing material for chemical sensors. Nanoscale 5, 2686. doi:10.1039/c2nr33088a
Wang, Q., Huang, J., Zhou, J., Liu, Z., Geng, Y., Liang, Z., et al. (2018). Different nanostructured tungsten oxides synthesized by facile solvothermal route for chlorine gas sensing. Sensors Actuators B Chem. 275, 306–311. doi:10.1016/j.snb.2018.08.047
Wang, X., Lu, J., Han, W., Yang, J., Jiang, B., Sun, Y., et al. (2021). Co-PBA MOF-derived hierarchical hollow Co3O4@NiO microcubes functionalized with Pt for superior H2S sensing. Sensors Actuators B Chem. 342, 130028. doi:10.1016/j.snb.2021.130028
Wu, X., Xiong, S., Gong, Y., Gong, Y., Wu, W., Mao, Z., et al. (2019). MOF-SMO hybrids as a H2S sensor with superior sensitivity and selectivity. Sensors Actuators B Chem. 292, 32–39. doi:10.1016/j.snb.2019.04.076
Xiangfeng, C., and Siciliano, P. (2003). CH3SH-sensing characteristics of LaFeO3 thick-film prepared by co-precipitation method. Sensors Actuators B Chem. 94, 197–200. doi:10.1016/S0925-4005(03)00340-X
Yaegaki, K., and Sanada, K. (1992). Volatile sulfur compounds in mouth air from clinically healthy subjects and patients with periodontal disease. J. Periodontal Res. 27, 233–238. doi:10.1111/j.1600-0765.1992.tb01673.x
Yao, X., Zhao, J., Liu, J., Wang, F., Wu, L., Meng, F., et al. (2022). H2S sensing material Pt-WO3 nanorods with excellent comprehensive performance. J. Alloys Compd. 900, 163398. doi:10.1016/j.jallcom.2021.163398
Yin, Y., Shen, Y., Zhou, P., Lu, R., Li, A., Zhao, S., et al. (2020). Fabrication, characterization and n-propanol sensing properties of perovskite-type ZnSnO3 nanospheres based gas sensor. Appl. Surf. Sci. 509, 145335. doi:10.1016/j.apsusc.2020.145335
Zhang, Y., Zhang, X., Guo, C., Xu, Y., Cheng, X., Zhang, F., et al. (2020). Novel two-dimensional WO 3/Bi 2 W 2 O 9 nanocomposites for rapid H 2 S detection at low temperatures. ACS Appl. Mat. Interfaces 12, 54946–54954. doi:10.1021/acsami.0c15611
Zheng, X., Zhang, G., Yao, Z., Zheng, Y., Shen, L., Liu, F., et al. (2021). Engineering of crystal phase over porous MnO2 with 3D morphology for highly efficient elimination of H2S. J. Hazard. Mat. 411, 125180. doi:10.1016/j.jhazmat.2021.125180
Zhou, Q., Xu, L., Kan, Z., Yang, L., Chang, Z., Dong, B., et al. (2022). A multi-platform sensor for selective and sensitive H2S monitoring: Three-dimensional macroporous ZnO encapsulated by MOFs with small Pt nanoparticles. J. Hazard. Mat. 426, 128075. doi:10.1016/j.jhazmat.2021.128075
Keywords: gas sensor, Au-PrFeO3, H2S, moss, gas-sensing
Citation: Zhang H, Xiao J, Chen J, Zhang L, Zhang Y and Jin P (2022) Au modified PrFeO3 with hollow tubular structure can be efficient sensing material for H2S detection. Front. Bioeng. Biotechnol. 10:969870. doi: 10.3389/fbioe.2022.969870
Received: 15 June 2022; Accepted: 27 July 2022;
Published: 24 August 2022.
Edited by:
Ben Ali Mounir, University of Sousse, TunisiaReviewed by:
Abdelghani Adnane, National Institute of Applied Science and Technology, TunisiaCopyright © 2022 Zhang, Xiao, Chen, Zhang, Zhang and Jin. This is an open-access article distributed under the terms of the Creative Commons Attribution License (CC BY). The use, distribution or reproduction in other forums is permitted, provided the original author(s) and the copyright owner(s) are credited and that the original publication in this journal is cited, in accordance with accepted academic practice. No use, distribution or reproduction is permitted which does not comply with these terms.
*Correspondence: Jing Xiao, eGlhb2ppbmd6eEAxNjMuY29t; Pan Jin, amlucGFubW91bnRhaW5AMTYzLmNvbQ==
Disclaimer: All claims expressed in this article are solely those of the authors and do not necessarily represent those of their affiliated organizations, or those of the publisher, the editors and the reviewers. Any product that may be evaluated in this article or claim that may be made by its manufacturer is not guaranteed or endorsed by the publisher.
Research integrity at Frontiers
Learn more about the work of our research integrity team to safeguard the quality of each article we publish.