- 1Department of Women’s and Children’s Health, University of Padova, Padova, Italy
- 2Laboratory of Biology, Medical School of Athens, National and Kapodistrian University of Athens, Athens, Greece
- 3Centro di Ricerca E. Menni, Fondazione Poliambulanza Istituto Ospedaliero, Brescia, Italy
- 4Department of Laboratory Medicine, Division of Pathology, Karolinska Institutet, Stockholm, Sweden
- 5Ludwig Boltzmann Institute for Experimental and Clinical Traumatology, The Research Center in Cooperation with AUVA Trauma Research Center, Austrian Cluster for Tissue Regeneration, Vienna, Austria
- 6Service de Chirurgie Orthopédique, Traumatologique et plastique, CHU Besançon, Laboratoire de Nanomédecine, Imagerie, Thérapeutique EA 4662, University Bourgogne Franche-Comté, Besançon, France
- 7Department of Experimental Medicine (DIMES), School of Medical and Pharmaceutical Sciences, University of Genova, Genova, Italy
- 8University of Reims Champagne Ardenne, EA 4691 BIOS “Biomatériaux et Inflammation en Site Osseux”, UFR d’Odontologie, Reims, France
- 9University of Bordeaux, INSERM, BIOTIS, U1026, Bordeaux, France
- 10Department of Medicine and Ageing Sciences, Section of Biomorphology, G. d'Annunzio University of Chieti-Pescara, Chieti, Italy
- 11Department of Molecular Cell Biology, Institute of Biochemistry, Vilnius University, Vilnius, Lithuania
- 12Department of Neuroscience and Rehabilitation, University of Ferrara, Ferrara, Italy
- 13Department of Obstetrics and Feto-maternal Medicine, Inselspital, Bern University Hospital, Department for BioMedical Research (DBMR), University of Bern, Bern, Switzerland
- 14Systems Biology Ireland, School of Medicine, Conway Institute, University College Dublin, Dublin, Ireland
- 15Institute for Transfusion Medicine, University Hospital Essen, University of Duisburg-Essen, Essen, Germany
- 16Laboratory of Experimental Hematology, Vaccine and Infectious Disease Institute (Vaxinfectio), University of Antwerp, Antwerp, Belgium
Perinatal tissues, such as placenta and umbilical cord contain a variety of somatic stem cell types, spanning from the largely used hematopoietic stem and progenitor cells to the most recently described broadly multipotent epithelial and stromal cells. As perinatal derivatives (PnD), several of these cell types and related products provide an interesting regenerative potential for a variety of diseases. Within COST SPRINT Action, we continue our review series, revising and summarizing the modalities of action and proposed medical approaches using PnD products: cells, secretome, extracellular vesicles, and decellularized tissues. Focusing on the brain, bone, skeletal muscle, heart, intestinal, liver, and lung pathologies, we discuss the importance of potency testing in validating PnD therapeutics, and critically evaluate the concept of PnD application in the field of tissue regeneration. Hereby we aim to shed light on the actual therapeutic properties of PnD, with an open eye for future clinical application. This review is part of a quadrinomial series on functional/potency assays for validation of PnD, spanning biological functions, such as immunomodulation, anti-microbial/anti-cancer, anti-inflammation, wound healing, angiogenesis, and regeneration.
1 Introduction
Stem and progenitor cells, including their cellular and acellular derivatives, are increasingly applied as therapeutic agents for a variety of pathologies, not only in preclinical animal studies but—more importantly—in human clinical trials also. However, the field of regenerative medicine for non-hematopoietic diseases has evolved over the past 20 years from direct cell replacement to trophic support exerted by administered cell populations or their derivatives in order to induce tissue repair (i.e., improve the functionality of damaged/diseased cells/tissues) and/or regeneration (i.e., stimulate the growth of new cells replacing diseased cells/tissues). Nevertheless, many questions remain regarding the type of stem cell (product) needed, the minimal criteria such a product needs to comply with, and appropriate guidelines for determining the success of an applied therapeutic intervention, both in preclinical animal studies and human clinical trials. While this field has been dominated by studies using tissue and cell products obtained from adult stem cell sources, and besides pluripotent stem cell derivatives (embryonic stem/induced pluripotent stem cells, ES/iPSC), emerging alternatives can be found in perinatal tissues. Due to their naïvity and easy access, perinatal tissues and cells collected during pregnancy (AFS) and at full-term pregnancy, here referred to as Perinatal Derivatives (PnD), may provide important advantages over adult stem cell products, especially for non-hematopoietic (stem) cell products. The main non-hematopoietic PnD currently used in pre-clinical and clinical applications are amniotic fluid/tissue and cells isolated from the placenta, including the amniotic membrane and the umbilical cord. For a detailed description and correct nomenclature of the different perinatal cell types of therapeutic interest, we refer to a recent SPRINT-COST supported review manuscript by Silini and colleagues, in which a proposed consensus nomenclature for human perinatal tissues and cells has been published (Silini et al., 2020). Here we will further elaborate on the most frequently studied PnD, their phenotypic characteristics, and suggested therapeutic efficacy in different pathologies, including various tools to determine their potency, by means of functional assays and before (and after) administration. A general overview of the manuscript organization is provided in Figure 1.
More specifically, with this manuscript, a team composed of experts and pioneers in using PnD products in clinical and veterinarian treatments, afferent to the COST SPRINT Action (CA17116), aimed to offer a guide on when and how it may be advantageous to the employment of placenta-derived cells or scaffolds. We will consider the applications of different sources of PnD with a focus on the functional/potency assays for the regeneration of different affected organs (Figure 2).
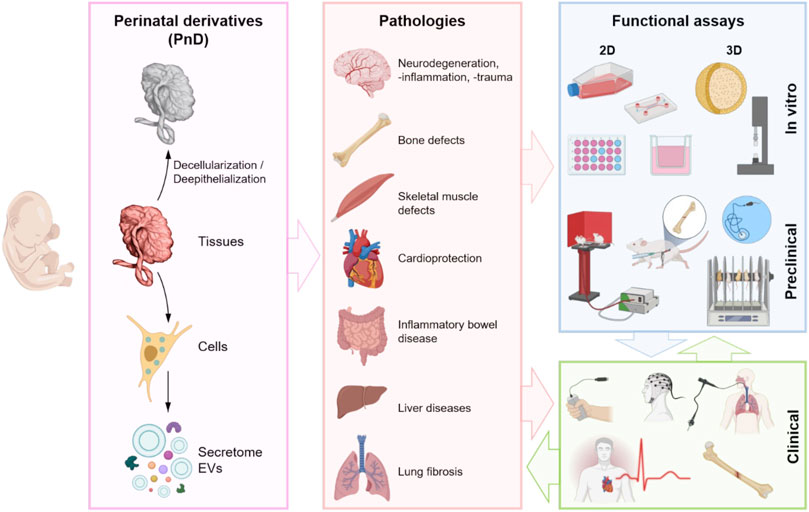
FIGURE 2. Schematic representation of the use of different PnD (tissues, cells, and paracrine derivatives—secretome (whole soluble factors including EVs) and isolated EVs), their application in different diseased organs and the functional assays. Created with BioRender.com.
Based on the unique ontogenetical and biological properties that characterize perinatal cells, different cells, spanning from infiltrating (maternal) immune cells to endothelial, epithelial, or mesenchymal stromal cells (MSC), have been isolated and studied during the last decades (Silini et al., 2020). These multipotent stem cells have been offering new, promising experimental applications for regenerative medicine and tissue engineering approaches. With its immunologically-privileged status (Zhang et al., 2017a), the PnD lends credence to its use as an allograft scaffold in tissue regenerative medicine. However, to widen a safe application, different strategies of nuclei depletion (decellularization or de-epithelization process) have been assessed to give rise to decellularized PnD (Gilbert et al., 2006; Crapo et al., 2011). After checking the quality of the decellularization process following standardized procedures of nuclei and DNA quantification (Gilbert et al., 2006; Crapo et al., 2011), naturally derived scaffolds, as from PnD, are thought to be an ideal and safe system to deliver chemokines and growth factors, to provide adequate structural and biomechanical microarchitecture in the damaged microenvironment (Jadalannagari et al., 2017; Dubus et al., 2022), with attention to avoid foreign body reaction and formation of the fibrous capsule (Keane et al., 2012; Hussein et al., 2016).
The development of a validation system designed according to international reference standards is an ambitious goal whose achievement still requires considerable effort toward the 1) cell-based strategies and 2) the cell-free strategies. Regarding the first, the efficacy of decellularized PnD (dP) combined with cells requires the employment of a conventional functional assay aimed at measuring the biochemical and mechanical properties of the dP on cell growth, differentiation, and functionality. In this scenario, the dP may act both as 1) a stem/progenitor-cell-like niche with key elements to control the regulation of stem cell fate and function, or 2) a platform for mature cells with the signals needed to initiate and maintain the differentiation of tissue-specific lineages and to support the cell repopulation after implantation. This approach relies on the ability of dP to promote the growth of cells with the enhanced potential to regenerate tissue damaged by injury, disease, or aging. This is the case of decellularized amniotic membrane (AM) and ultra-thin AM (Zhang et al., 2016) or Wharton’s jelly (Penolazzi et al., 2020; Dubus et al., 2022; Penolazzi et al., 2022) which have been produced as a suitable scaffold for the ex vivo expansion and delivery of stem and progenitor cells.
Regarding the cell-free strategies, the use of in vivo biological assays will allow testing the efficacy of dP extracellular matrix (dP-ECM) as a cell-free scaffold to produce a good regeneration guide.
A wide variety of clinical applications, after biocompatibility evaluation by subcutaneous implantation tests according to ISO/EN 10993 part 6 guidelines, have been reported for human amniotic membrane (hAM) / human amnio-chorionic membrane (hACM (Fenelon et al., 2019; Fénelon et al., 2021). In vitro cytocompatibility was assessed by extract cytotoxicity assay and contact cytotoxic assay according to the ISO/EN 10993 part 5 guideline.
Multicellular potency has been found critical in preclinical/clinical transplantation as much as PnD paracrine effects, subsidiary to support engraftment and long-term survival for donor cells.
It has been largely described as dP or perinatal cells have constitutive angiogenic and anti-inflammatory properties (C hoi et al., 2012) carried out by release mediators such as transforming growth factor beta 1 (TGF- β1), basic fibroblast growth factor (bFGF), epidermal growth factor (EGF), platelet-derived growth factor (PDGF), insulin-like growth factor 1 (IGF-1), vascular endothelial growth factor (VEGF), anti-inflammatory cytokines (i.e. interleukin (IL)-10), antimicrobial peptides (β-defensins, elafin), and tissue inhibitor of metalloproteinases (TIMP-1, 2, 3 and 4) (Hao et al., 2000; Mao et al., 2017a).
A remarkable property of natural scaffolds and more consistent in progenitor/stem cells is to secrete extracellular vesicles (EVs) commonly distinguished as: 1) macrovesicles (MVs) and 2) exosomes (Rani et al., 2015) vehiculating immunomodulatory or anti-inflammatory molecules in the form of miRNA, mRNA, proteins characteristic of cells of origin (Chen et al., 2010; Rani et al., 2015). Moreover, EVs may have a superior safety profile as compared to the cells they derived from since they do not replicate (Reis et al., 2016) nor cause microvascular embolism (Xiong et al., 2017) and can be stored without losing their properties (Wen et al., 2016). Thus, extensive research is currently underway to establish the potential use of mesenchymal stromal cells extracellular vesicles (MSC-EVs) for cell-free therapeutic applications in diseases, such as cardiovascular diseases (Fleury et al., 2014), liver failure (Maji et al., 2017), and respiratory diseases (Abreu et al., 2016). In addition, animal model-based studies suggest that exosome-sized or small EVs (sEVs), compared to their parental cells, may represent a novel cell-free medicinal product that is effective and can be safely stored without loss of function, as an alternative to cell-based therapies (El Andaloussi et al., 2013; Masyuk et al., 2013).
The role of PnD has been considered paramount in specific environments where support or corrective effects are needed, such as neurodegenerative diseases, skeletal muscle and bone repair, cardioprotection, intestine inflammation, and liver and lung chronic or congenital disorders. The use of either tissue patches, depleted from cells (decellularized hAM or umbilical cord), or perinatal cells/EVs have been validated in preclinical models and translated to the clinic (Figure 2). During the past year, our team composed of international experts on PnD technologies revised and compared existing standardized procedures validated to produce PnD medical products. The present work compiles minimum requirements and provides an overview of the minimal measures needed to translate PnD therapies to the clinic. Being supportive, Table 1 summarizes the applications of functional/potency assays of PnD in research and the clinic (also see Table 1 for more details).
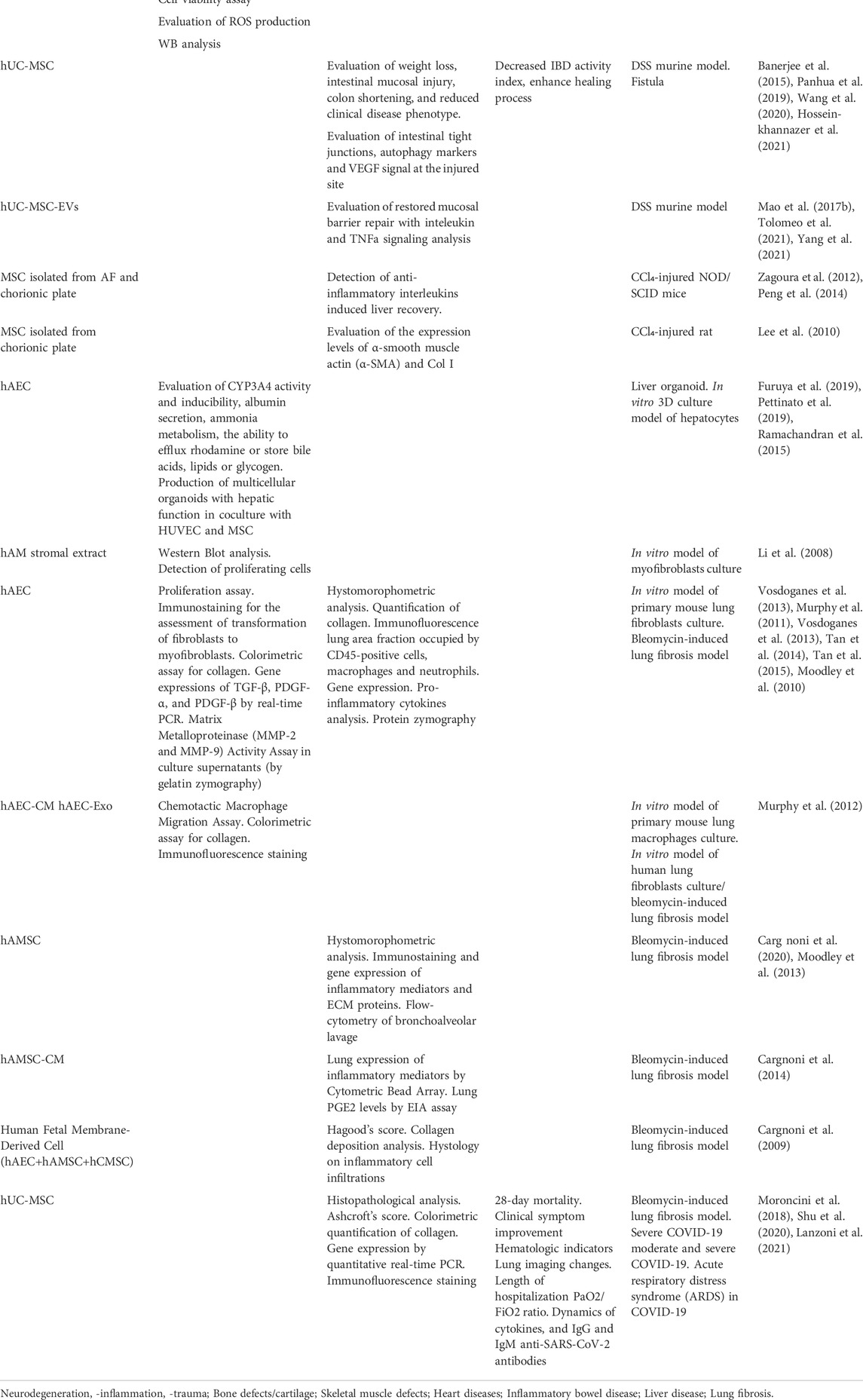
TABLE 1. Overview of the functional assays for the different compartments analyzed in the present manuscript.
Crucial aspects of qualification and functional characterization of the final products will be described and discussed. Within the next paragraphs, we aim to bring to light the standardized procedures used to produce a specific regenerative effect. Table 2 provides an overall conclusion for each of the compartments discussed below.
2 Specific sections dedicated to PnD applications
2.1 Role of perinatal derivatives in treatment of neurodegenerative diseases
Novel treatment suggestions for neurodegenerative disorders applying cell or cell-derived products, including those from perinatal sources, primarily rely on the beneficial paracrine effects of the applied products. Although PnD have been shown to display trans-differentiation capacity into neural-like cells in vitro, or shown to home to the regions of interest upon transplantation, their differentiation capacity into neural cell types and the replacement of damaged neural cells in vivo following grafting plays only a minor (if after all) contribution to the beneficial effect PnD can display as a treatment modality for neurodegenerative disorders. More likely, as neurodegeneration is often accompanied by out-of-balance neuroinflammation, PnD interventions aid in resolving chronic inflammatory processes, induction of neuroprotection by the release of neurotrophic factors, and by stimulation of endogenous neurogenesis, with all three contributing to a successful neuro-regenerative potential (Volkman and Offen, 2017).
2.1.1 Functional assays to demonstrate a neuroprotective effect of perinatal derivatives on neural stem/progenitor cells and neuronal cells after oxidative stress
Oxidative stress following acute or chronic hypoxic-ischemic insults, such as ischemic stroke, hemorrhagic stroke, or hypoxic-ischemic encephalopathy, is a common hallmark of many neurodegenerative diseases. A number of assays have been developed to assess the potential of PnD to reduce or resolve stress responses during/after an ischemic insult. Hereto, neural stem (NSC) or progenitor cells (NPC), neuronal primary cells, or cell lines (such as the mouse neuroblastoma cell line Neuro 2a) are exposed to conditions mimicking a hypoxic-ischemic insult, such as oxygen-glucose deprivation (OGD), possibly followed by reoxygenation or the exposure to H2O2 (Petrenko et al., 2020). Different types of PnD may then be added in direct or in indirect co-culture (transwell culture system) with the neural cells. Alternatively, the neural cells’ culture medium can be supplemented or replaced with a conditioned medium containing the PnD’s secretome (fractions). Since NSC/NPC have the potential to differentiate into all neural cell types, the effects of the PnD treatment on the cell fate specification and differentiation can be assessed by measuring the expression of cell type-specific markers along with the documentation of nuclear and cytoplasmic morphological changes in cells undergoing differentiation. With neuronal cell death being a hallmark of hypoxic-ischemic insults, in the first instance changes in neuronal apoptotic and necrotic outcome measures, such as standard viability assays as well as DNA fragmentation or the expression of molecules associated with apoptotic pathways (e.g., caspases), are valid indicators of the neuroprotective potential of PnD. Depending on the disease model, more specific assays such as the effect of PnD on neurite outgrowth (measured as the total area of neurites) of dorsal root ganglion (Petrenko et al., 2020) or other neurons will complement these outcome measures.
2.1.2 Functional assays to demonstrate a pro-myelination effect of perinatal derivatives on oligodendrocyte (progenitor) cells
Myelination of neuronal axons is a prerequisite for the efficient transmission of electrical signals in the central nervous system (CNS). Many neurodevelopmental or degenerative disorders disrupt myelination or myelin homeostasis. Oligodendrocytes in the CNS and Schwann cells in the peripheral nervous system (PNS) are the cells that build the myelin sheath enwrapping the axons. Oligodendrocytes differentiate from NSC/NPC into oligodendrocyte progenitor cells (OPC), differentiation-committed precursors, newly formed oligodendrocytes, myelin-forming oligodendrocytes, and finally mature oligodendrocytes. This oligodendrocyte fate specification is used to model the potential of PnD to support processes involved in myelination. Hereto, NSC/NPC are directly or indirectly co-cultured with PnD or the culture medium supplemented with a conditioned medium derived from PnD cultures. Differentiation into the different stages of oligodendrocyte development is observed by morphological changes and the expression of stage-specific proteins such as chondroitin sulfate proteoglycan 4 (CSPG4) and platelet-derived growth factor receptor alpha (PDGFRA), markers of oligodendrocyte progenitor cells, galactosylceramidase (GALC), a marker of immature oligodendrocytes, or the mature oligodendroglial marker myelin basic protein (MBP) (Oppliger et al., 2017). Improvement of damage or developmental arrest can also be assessed by co-culturing PnD or administering their secretome to oligodendrocyte cell lines (e.g., MO3.13, (Joerger-Messerli et al., 2021)) or primary OPC after lipopolysaccharide (LPS)- and/or OGD-mediated injury.
2.1.3 Functional assays to demonstrate anti-inflammatory effects of perinatal derivatives on microglia and astrocytes
Microglia are the resident immune cells of the CNS and act as scavengers to remove damaged cells or pathogens. Under pathological conditions, microglia react to danger or pathogen-associated molecular patterns (DAMP/PAMP), proliferate, migrate, undergo morphological changes, and secrete inflammatory molecules such as cytokines, chemokines, and neurotoxic factors. This stimulation can be mimicked in vitro by the exposure of primary microglia (Stansley et al., 2012), mixed glial cells (Thomi et al., 2019), immortalized (Stansley et al., 2012; Thomi et al., 2019; Spinelli et al., 2020), or iPSC-derived (Hasselmann and Blurton-Jones, 2020) microglial cell lines to LPS or a combination of interferon-gamma and tumor necrosis factor-alpha (IFNγ+TNFα) (Lively and Schlichter, 2018), with or without hypoxia. Indirect co-cultures with the microglial cells in the bottom and the PnD in transwells, or the addition of PnD-conditioned medium to microglial cells are used to examine the PnD immunoregulatory effects on microglial activation by measuring microglial proliferation, the expression or secretion of inflammatory molecules and by documenting morphological changes (Cao et al., 2019). Alternatively, organotypic brain explant models allow for stimulating glial cells in their niche and the context of pathological cell interactions (Holloway et al., 2021). Furthermore, the recent development of neuro-immune competent organoid models may even shed further light on the diffusion properties of PnD-derived therapeutic actors within a brain-like environment.
Astrocyte activation is a second important hallmark in many neuroinflammatory and degenerative diseases. Primary astrocytes or glial cell cultures enriched in astrocytes (Gorina et al., 2009) can be activated by the treatment with LPS (Roboon et al., 2021) or by OGD (ischemia-reperfusion (Wu et al., 2021)) or H2O2 (Wen et al., 2021) in indirect (transwell) co-culture systems. Outcome measures include the production of reactive oxygen species (ROS), the upregulation of the intermediate filament proteins glial fibrillary acidic protein (GFAP) and vimentin, and the presence of complement C3+ astrocytes or reactive astrocyte-induced neuronal cell death (Liddelow et al., 2017).
2.1.4 Functional assays to demonstrate the influence of perinatal derivatives on blood-brain barrier integrity
CNS injury or hypoxic-ischemic and inflammatory insults are accompanied by an increase in the blood-brain barrier (BBB) permeability, resulting in the extravasation of proteins and uncontrolled immune cell trafficking (Cecchelli et al., 2014). Advanced in vitro BBB models include brain microvessel endothelial cells, pericytes, and astrocytes (Jamieson et al., 2019) grown in transwell cell cultures or microfluidic devices (Campisi et al., 2018). Small molecule tracers, such as the fluorescent FITC-dextran (Campisi et al., 2018), are added into the upper chamber or outer compartment and are used to monitor the permeability of the BBB model upon stimulation with inflammatory molecules such as TNFα (Chen et al., 2016). The influence on BBB permeability by PnD secretomes can be tested by adding a PnD-conditioned medium to the culture system. Additionally, readout systems include the expression of adhesion molecules and tight junction proteins on the endothelial cells that are involved in the rolling, tethering, and extravasation of peripheral immune cells from the bloodstream to the brain. Transendothelial electric resistance (TEER) is used to measure the integrity of tight junctions in transwell BBB culture models (Srinivasan et al., 2015).
2.1.5 Functional assays to demonstrate the influence of perinatal derivatives on peripheral nerve repair
hAM has been evaluated as nerve wraps or conduits for the repair of peripheral nerve injuries in vitro in human and pre-clinical models (Wolfe et al., 2022) and humans (Fénelon et al., 20212021). hAM alone or associated with adult or perinatal cells (Fénelon et al., 2021), such as allogeneic hUC-MSC (Li et al., 2013), has been evaluated. In animal models, allogeneic hUC-MSC alone or coupled to additional scaffolds like PCL polycaprolactone (PCL) or chitosan were delivered at the site of injury (Bojanic et al., 2020), supplemented with systemic erythropoietin injection (Ülger et al., 2021).
In addition, human amniotic membrane epithelial cells (hAEC) (Jin et al., 2015), hAMSC (Chen et al., 2022), hAF stem cells (Su et al., 2018), or hUC-MSC (Bojanic et al., 2020) have been tested alone or coupled to additional scaffolds like PCL or chitosan or embedded in fibrin hydrogel-containing polylactate (PLA) nerve conduits (Su et al., 2018). For sciatic nerve injury models, Schwann cell proliferation and migration were used as in vitro assays (Chen et al., 2022). Non-invasive, conventional magnetic resonance imaging (MRI) coupled to a diffusion tensor MRI (DTI)-based fiber tractography assay has been used in the minipig model to follow the repair of sciatic nerve injury after hAF stem cell grafting (Su et al., 2018). Stress relaxation and creep testing were performed to assess the viscoelastic behavior of strained brachial plexus samples, and grooming behavior was scored as an indicator of forepaw motor function in a rabbit model (Jin et al., 2015).
Animal studies and clinical trials have highlighted their role in preventing the recurrence of perineural adhesions, reducing extra- and intra-neural fibrosis, accelerating nerve repair/regeneration, and improving nerve function (Lemke et al., 2018; Bour geois et al., 2019; Fénelon et al., 2021; Mirzayan et al., 2021). Different types of nerve injuries, including those affecting lower or upper extremity and thorax were assessed for: gap, transection, neurotmesis, crush, epineurectomy, induction of chemical fibrosis, and partial transection.
Histological (HE, Toluidine Blue, Oil Red O staining) or immunohistological analysis have allowed qualitative/stereological analysis of the gross morphology or microstructure of: 1) nerve parameters such as axon regeneration—increase of axon size—number—diameter—density, axonal migration, myelin sheath thickness or nerve fiber diameter; 2) muscle mass innervated by the transected then repaired nerve and 3) muscle atrophy attenuation (Bour geois et al., 2019; Bojanic et al., 2020; Ülger et al., 2021; Wolfe et al., 2022). Retrograde axonal transport was estimated through fluorogold-labeled neuron counts after hUC-MSC grafting (Bojanic et al., 2020).
Neuromorphometry employs transmission electron microscopy or high-resolution light microscopy in which the number of myelinated fibers, the total number of nerve fibers, axon diameter, myelin thickness, G-ratio, and N-ratio were the most commonly reported outcomes (Marchesini et al., 2018; Wolfe et al., 2022).
In a rat scarring model for recurrent adhesions in the peripheral sciatic nerve, a vital hAM has been demonstrated to prevent adhesion, improve the level of extra- and intra-neural fibrosis, and support nerve regeneration, and also reflected in the sciatic functional index (Lemke et al., 2018).
Functional motor assessments included various types of static or dynamic gait analysis, strength measurements, or analysis of other specific behavior (Su et al., 2018; Bour geois et al., 2019; Ülger et al., 2021; Wolfe et al., 2022). The sciatic functional index (SFI) is an index of the functional condition of rat sciatic nerve based on measurements made from walking tracks (Ülger et al., 2021). Functional sensory outcomes measures also included withdrawal latency reflex (WRL) tests, tactile tests, or heat cutaneous allodynia (Wolfe et al., 2022).
Electrophysiological tests were performed in the pre-clinical model using a bipolar stimulating electrode placed under the nerve proximal to the hAM graft. Recordings were conducted using superficial electrodes and performed with an electromyogram (EMG). The peak amplitude of the compound muscle action potential (CMAP), CMAP latency of onset, and nerve conduction velocity (NCV) values were calculated (Su et al., 2018; Bour geois et al., 2019; Wolfe et al., 2022).
In humans, non-invasive ultrasound was used to determine neuroma formation and nerve regeneration determined based on EMG (Li et al., 2013).
After hAM grafting, sometimes associated with the hUC-MSC application, sensory and motor function restoration was evaluated based on the Modified Medical Research Council classification (MRCC), categorizing superficial cutaneous pain and tactile sensibility (Li et al., 2013). Additional tests include the Lister test for the intrinsic muscles of the hand, grip power and pinch strength measurements, and Jamar or Sakellarides tests (Riccio et al., 2014).
Finally, satisfaction of patients was assessed by means of the pain disappearance and the Quick Disabilities of the Arm, Shoulder, and Hand (Quick DASH) which evaluates 30 items that measure: 1) physical abilities (21 items); 2) severity of symptoms (5 items), and 3) social skills or roles (4 items) (Marchesini et al., 2018).
2.1.6 Conclusion
The advantage of CNS in vitro cell culture systems, compared to animal models, is the relative ease of use, their potential for standardization, and the comparatively short interval from the models’ onset to the collection of data. Unfortunately, these assays either alone or in combination are not uniformly used throughout various studies evaluating novel therapeutic strategies for CNS disorders. Therefore, this field may majorly benefit from defining a standardized approach to select a number of appropriate elementary in vitro assays for which PnD should pass before proceeding to animal studies and/or human clinical trials. Furthermore, in line with the view of in vitro models contributing to the 3R principles in animal experimentation, the development of 3D models and organoids for in vitro CNS research may be a major step forward toward clinically meaningful readouts. Once established, this may increase the predictivity of therapeutic success of various PnD.
2.2 Role of perinatal derivatives in treatment of the skeletal-muscle injury
The most challenging aspect of skeletal muscle regeneration is the need for reconstruction of muscle fiber architecture in order to restore tensile force and function. With this in mind, various kinds of supportive scaffolds have been employed for tissue engineering purposes, to guarantee correct myoblast alignment and to induce their fusion. Several approaches already involved the use of scaffolds of natural origins like decellularized PnD to drive myofiber production in damaged muscle.
2.2.1 Functional assays to demonstrate the influence of perinatal derivatives-derived scaffolds on skeletal muscle repair
Experimentally, decellularized scaffolds produced from human amniotic membrane have been proven effective in muscle repair and force regain when combined with training in a rat volumetric muscle loss (VML) model (Izadi et al., 2021). This approach furthermore led to a significant increase in the number of the newly formed centrally nucleated myofibers compared to untreated muscles, an important parameter often evaluated in such models because it is directly correlated to the fusion of myoblasts occurring during muscle regeneration (Wang et al., 2018; Hadipour et al., 2021; Izadi et al., 2021; Magarotto et al., 2021). Moreover, tissue regeneration after scaffold implantation was not only visible by histological methods, but at a functional level. Here electrophysiological methods allowed to demonstrate increased isometric contraction force of the muscle after nerve stimulation (Qiu et al., 2018; Izadi et al., 2021; Magarotto et al., 2021). In an alternative approach, the peculiar tissue architecture was reproduced in artificial implantable scaffolds with the addition of electrospun fibers of polymers like poly(lactic-co-glycolic acid) PLGA or PCL onto the decellularized amniotic membrane. This guaranteed optimal mechanical properties (strength and elasticity) and cell orientation, as evaluated by measuring the angle of the generated fibers or with live imaging techniques to track cell motility (Hasmad et al., 2018). Furthermore, stem cell differentiation was favored toward a myogenic phenotype, defined by the expression of regenerating muscle markers like MyoD, MyoG, Mhc (Hadipour et al., 2021; Izadi et al., 2021; Magarotto et al., 2021; Park et al., 2021).
2.2.2 Functional assays to demonstrate the influence of perinatal derivatives on skeletal muscle repair
The employment of a scaffold is often not sufficient for complete regeneration in case of extensive tissue damage. In this situation, an effective approach is the administration of cell types that show myogenic potential (Mishra et al., 2020) or are active in the crosstalk with the other cell types that reside in the muscle niche. UC-MSC are active in muscle repair thanks to the interaction with the inflammatory compartment, which triggers and supports regenerative mechanisms. Importantly, they are not only involved in the production of myogenic miRNAs (Mishra et al., 2020; Sun and Karaoz, 2020; Park et al., 2021), but they are also capable to 1) promote macrophage polarization toward an M2 pro-regenerative phenotype, as characterized by the expression of both CD68 and CD206 (Qiu et al., 2018; Arutyunyan et al., 2019), and 2) reduce neutrophil-mediated acute inflammation, indicated by reduced infiltration of Ly6G+ cells at the site of injury (Su et al., 2019). Mechanistically, MSC were found to reduce chronic inflammation in sarcopenia through TNFα and IL-6 downregulation (reducing M1-oriented pro-inflammatory signals) and IL-4 and IL-10 upregulation (stimulating M2-oriented anti-inflammatory signals (Wang et al., 2018). For their immunomodulatory and antifibrotic properties, they were also proposed for the treatment of Duchenne’s Muscular Dystrophy (Sun and Karaoz, 2020; Park et al., 2021). Moving from cell-based interventions to acellular therapeutics, similar immunomodulatory and/or anti-fibrotic effects were also exerted by their secretome. The mechanism of action of MSC-EVs in muscle regeneration is still under investigation. However, evidence exists that they can modulate the inflammatory response and direct it toward regeneration with the reduction of fibrosis, a parameter quantified by the histological analysis of collagen deposition in the regenerating tissue (Bier et al., 2018; Magarotto et al., 2021). MSC-EVs also act on neuromuscular junctions, repairing axons and restoring nerve physiology (Ma et al., 2019; Magarotto et al., 2021), which is paramount for muscle function, evaluated in vivo by grip or gait analysis (Ma et al., 2019; Su et al., 2019).
2.2.3 Conclusion
The multifaceted properties of PnD derivatives are attracting scientific interest for the development of new therapeutic applications in the regenerative medicine field of muscle regeneration. Besides the structural and mechanical support, it appears evident that the paracrine factors released by MSC such as the EVs are the executive of the mechanism of action of PnD in skeletal muscle repair. However, this complex aspect is still under investigation.
When skeletal tissues are damaged, natural scaffolds with their own growth factors and cytokines but also adjuvanted with MSC and EVs stimulate a favorable microenvironment. The promoted regeneration by the induction of pleiotropic effects such as, among others, immune-modulating and pro angiogenic can be validated in vivo. In this paragraph indeed, we underlined that in vivo functional assays are the best way to prove the PnD derivatives effects.
2.3 Role of perinatal derivatives for bone repair
Bone tissue engineering has emerged as an interdisciplinary strategy combining biomaterials, cells, and/or biologically active molecules, aiming to reconstruct injured or lost bone. PnD and in particular, perinatal tissues and derived cells have been used for that purpose. Orthopedic surgeons successfully apply cryopreserved or decellularized and lyophilized bone allografts. Thus, de-epithelialized or decellularization and/or lyophilization have been used accordingly for perinatal tissues to improve their storage, their biocompatibility, and their capacities to act as a scaffold.
2.3.1 Functional assays to demonstrate the osteogenic potential of the amniotic membrane
Both human amniotic membrane MSC (AMSC) and hAECs display in vitro and in vivo osteogenic differentiation potential (Kmiecik et al., 2015). It was reported that under osteogenic conditions in vitro, intact hAM became mineralized and expressed markers of early and late osteoblastic differentiation, as demonstrated by a significant rise in calcium contents, induction of alkaline phosphatase (AP) activity, RUNX2, AP, BMP-2, and BMP-4 mRNA expression and osteocalcin staining colocalizing with von Kossa/alizarin red staining (Lindenmair et al., 2010).
Similarly, anti-collagen I immunohistochemistry was carried out on intact osteodifferentiated hAM to highlight osteoblastic differentiation potential (Gualdi et al., 2019). Furthermore, Energy dispersive X-ray (EDX) and X-ray diffraction (XRD) were used as a novel approach to qualify the mineralization of amniotic cells in intact osteodifferentiated hAM and derived amniotic cells (Gualdi et al., 2019). Here, it was reported that, in osteogenic conditions, hAEC had a mesenchymal phenotype with osteocyte function, and even native synthesis of hydroxyapatite, focusing osteogenic potential mainly in this epithelial layer. The whole hAM is characterized using silver nitrate and Alizarin red which are typically used to label the mineralization of isolated cells. Both assays would be easy to implement in a tissue bank.
2.3.2 Functional assays to demonstrate the potential of perinatal derivatives in bone repair
In vitro, the ability of fresh and preserved AM to act as a scaffold upon which adult human bone marrow MSC (hBMSC) can proliferate and differentiate into osteogenic lineage has been assessed using metabolic activity assays, alkaline phosphatase staining, and alizarin red quantification (Fenelon et al., 2019; F enelon et al., 2020). In vivo, the efficiency of hUC-MSC to promote bone regeneration can be assessed using ectopic or orthotopic models to evaluate their osteoinductive and/or osteoconductive properties. Representative 2D or 3D-radiography followed by histology (Masson’s trichrome and HES staining) are the most commonly performed analysis to evaluate bone regeneration in animal models (Etchebarne et al., 2021; Fenelon et al., 2021). Immunohistochemistry (type I collagen, osteocalcin, AP) brings additional information (Laurent et al., 2017). As an example, it has been investigated the in vitro and in vivo bone regenerative performance of hUC-MSC and Arginyl-glycyl-aspartic acid (RGD) modified macroporous calcium phosphate cements (CPC). Similarly, it has been observed high expressions of osteogenic specific genes on CPC seeded with hUC-MSC. After implantation for 24 weeks in the rat calvaria bone defect model, the newly formed bone tissue exhibited a higher bone mineral density, new bone amount, and vessel density versus CPC control group (Chen et al., 2013). These observations are in agreement with a study combining blood umbilical cord-derived stem cells with a partially demineralized bone matrix (Liu et al., 2010). Calcium phosphate synthetic bone substitutes (Barboni et al., 2013) and magnesium-enriched hydroxyapatite (MgHA)/collagen-based scaffold (Riccio et al., 2012) were also combined with ovine amniotic fluid PnD. Implanted in an ovine maxillary sinus augmentation model, this study demonstrated a reduced fibrotic reaction, a limited inflammatory response, and an accelerated process of angiogenesis for Sinus explants derived from the PnD-scaffold group compared with the control group (without PnD) (Riccio et al., 2012; Barboni et al., 2013).
Bone develops through a tightly regulated process leading to a hierarchically ordered three-dimensional structure, described in the literature as a bone nodule (Mechiche Alami et al., 2016). UC-WJ-MSC are able to form these 3D structures in vitro (Rammal et al., 2017). However, the nodule density of around 9 ± 2 nodules in the cultured area suggests a low commitment of UC-WJ-MSC into osteoprogenitor cells (Rammal et al., 2019). These observations are consistent with other studies highlighting a very low yield of UC-WJ-MSC able to differentiate into osteoblastic lineage (Jin and Lee, 2018; Golchin and Farahany, 2019). Once injected into damaged tissue, UC-WJ-MSC showed a relatively poor rate of cell engraftment and engrafted ones are rather to be short-lived (Wang et al., 2014). The low rate of cell differentiation is in favor of a paracrine mechanism. Despite the small fraction of committed cells, the osteoinductive (i.e., able to induce cell differentiation without adding chemical inductors) scaffold decreased the production of IL-1β, IL-6, and IL-8 inflammatory mediators and increased the release of b-FGF, VEGF angiogenic growth factors by cells in comparison with the inert scaffold. Furthermore, this paracrine action of PnD’s cells enhanced the migration of endothelial cells, the neutrophil diapedesis via ICAM1 up-regulation and the osteogenic potential of endothelial cells (e.g. overproduction of BMP-2) (Rammal et al., 2019).
2.3.3 Clinical assays to demonstrate the influence of PnD on bone repair
Usually, in the clinic, bone union and failure were assessed every 6 months during the first year as follows: first X-ray (or Computed Tomography (CT) scan if doubt) showing 3 of 4 continuous cortices (united) (Moris et al., 2016). Clinically, healing is assessed by the absence of pain and absence of mobility at the fracture site and, in the case of the lower limb, weight-bearing (Gindraux et al., 2020). Some patients could also benefit from the Position–Emission Tomography. In bone repair, osseous biopsies for histological or immuno-histochemistry analysis are often too invasive and usually not performed. When used for example to evaluate the benefit of a specific treatment, markers are comparable to those used in vivo (Etchebarne et al., 2021).
2.3.4 Conclusion
In conclusion, for bone regenerative approaches, PnD have been considered in different formats. Although PnD may contribute to bone formation directly, their major value may rely rather on their paracrine activity. The amniotic membrane may act as a collagenous niche for tissue deposition and ingrowth. In consequence, in vitro functional assays identifying activities such as immunomodulation, proangiogenic and trophic factor release may represent suitable surrogates for these properties.
2.4 Potential role of perinatal derivatives in cardioprotection
Cardiovascular disease and heart failure represent major social and economic burdens as being the leading cause of mortality and morbidity worldwide, with ischemic myocardial infarction the most common event underlying the development of cardiac dysfunction and heart failure (Segers and Lee, 2008; Roger, 2013). When facing prolonged ischemia, the adult mammalian heart can only activate a defective repair mechanism aiming at replacing cardiomyocytes lost during the hypoxic injury with a non-contractile fibrotic collagen scar, leading over time to the detrimental remodeling of the left ventricle, with progression toward myocardial dysfunction and heart failure (Madonna et al., 2016). Despite the remarkable progress achieved by pharmacological and interventional cardiology in the last 20 years, heart transplantation still represents the ultimate therapeutic option for heart failure. Therefore, preclinical research in cardiac regenerative medicine has been recently developed to define innovative therapeutic strategies to enhance cardiac repair mechanisms.
2.4.1 Emerging regenerative approaches for heart repair
Bona fide myocardial regeneration can only be obtained by structural and functional reconstitution of compromised/lost cardiomyocytes following injury, via the active renewal of surviving resident ones (Eschenhagen et al., 2017). Such regenerative mechanisms have been extensively described in lower vertebrates. Notably, the neonatal rodent heart has recently been shown to promote proficient myocardial renewal as well, although this response is strictly limited to the very first week after birth, with a clear transition to fibrosis and defective repair soon afterward (Foglia and Poss, 2016). True cardiac regeneration and active myocardial renewal are thus very challenging to be accomplished in the mammalian adult heart; therefore, increasing efforts have recently been focused on optimizing endogenous mechanisms of cardiac repair. These directions focus on enhancing cardio-protection and supporting local angiogenesis, in order to preserve as much viable myocardium as possible during injury/disease. In such a perspective, high expectations have been allocated to paracrine effects from stem/progenitor cells and MSC for cardiac repair (Gnecchi et al., 2012; Hodgkinson et al., 2016; Bollini et al., 2018; Menasché, 2018; Balbi et al., 2020; Magh in et al., 2020). Indeed, the secretome isolated from MSC of different PnD origin has widely been investigated in preclinical cell-free strategies for implementing endogenous myocardial repair as tentative proof-of-principle for future therapy. As a matter of fact, endogenous cardiac repair should be promptly targeted during the early phase following acute heart injury (i.e., following myocardial infarction, at time of reperfusion) or improved by additional therapy in case of off-target cardiotoxic effects due to chronic pharmacological treatment (i.e., chemotherapy).
2.4.2 Functional assays to validate perinatal derivatives in cardioprotection/repair
In vitro cardioprotective and pro-angiogenic assays are broadly used to define the paracrine effects of stem/progenitor cell secretomes on supporting cardiomyocyte survival and triggering new vessel development. The in vitro mimicking of the altered/injured myocardial conditions can be obtained by exposing cardiomyocytes to severe stress/damage to assess their apoptosis and necrosis, as it has been previously and comprehensively shown (Lazzarini et al., 2016; Balbi et al., 2019a; Balbi et al., 2019b; Takov et al., 2020).
Cardioprotection is usually assessed by evaluating the level of intracellular ROS and metabolism impairment, along with activation of apoptotic pathways and DNA fragmentation in target cardiac cells undergoing hypoxic and/or oxidative stress insult/injury. Cardiomyocyte cell viability is usually evaluated by annexin V and propidium iodide (AnnV/PI) staining, and by MTT/MTS and lactate dehydrogenase activity assay, thus measuring metabolic activity. Angiogenic analyses are generally based on evaluating endothelial progenitor or endothelial-like cell migration and capacity to form tube structures and capillary configurations on Matrigel, following specific stimuli.
The cardio-active and pro-vasculogenic potential of different secretome formulations (the total cell-conditioned medium or its separated and concentrated EV subfraction) from human PnD (i.e., AM-derived progenitors, amniotic fluid stem cells, and umbilical cord stromal mesenchymal cells) have been intensely scrutinized in the last years. The human amniotic fluid progenitor cell secretome and EVs separated and concentrated from that have been recently described to counteract cell death and premature senescence in rodent cardiomyocytes exposed to severe hypoxic stress and cardiotoxic exposure to doxorubicin in vitro; likewise, human endothelial colony-forming cells (ECFC) and human umbilical vein endothelial cells (HUVEC) have been shown to respond to the secretome paracrine stimulation by improving their migratory and formation of capillary-like structure in vitro (Lazzarini et al., 2016; Balbi et al., 2019a; Balbi et al., 2019b; Takov et al., 2020). Similar promising cardioprotective results have also been obtained with a human amniotic membrane MSC-conditioned medium on the rat embryonic cardiomyoblast-like H9c2 cell line in a model of hypoxia and reoxygenation injury in vitro (Danieli et al., 2015). The therapeutic paracrine relevance of human PnD has further been reported in a study demonstrating that human amniotic membrane proteins (AMPs) extracted from the amniotic membrane can antagonize doxorubicin-elicited detrimental effects on H9c2 via the inhibition of oxidative stress and apoptosis. The whole secretome of independent formulation of human umbilical cord mesenchymal stromal cells (UC-MSC), and the EVs obtained from it, has been also proven to be effective in preserving rodent cardiomyocyte cell lines from apoptosis, while stimulating the pro-angiogenic potential HUVEC (Santos Nascimento et al., 2014; Huang et al., 2020).
2.4.3 Conclusion
The majority of studies, reporting in vivo validation of PnD therapeutic efficacy (by assessing cardiac function via ultrasound analysis in preclinical animal models of myocardial injury, as extensively reviewed and discussed elsewhere (Magh in et al., 2020)), are to a certain degree supplemented with in vitro data describing cardioprotective effects. Some mechanistic studies have been reported, showing as human amniotic fluid stem cell secretome may preserve doxorubicin-stressed murine primary neonatal cardiomyocytes by activating the PI3K/Akt signaling cascade, and by upregulating the NF-κB controlled genes, Il6 and Cxcl1 (Lazzarini et al., 2016). Moreover, human umbilical cord MSC-EVs have been shown to exert beneficial effects as mediating the horizontal transfer of miR-19a, targeting Sox6 in hypoxic H9c2 cells in vitro; such candidate mechanism of action was further assessed by means of Sox6 knockdown together with miR-19a inhibition within MSC-EVs (Huang et al., 2020). In conclusion, while most of the studies published so far have reported beneficial effects by using different PnD, consensus on a uniform method for standardized dosing and protocol of administration has not been reached yet.
2.5 Potential role of perinatal derivatives in bowel inflammation/intestinal regeneration
Inflammatory Bowel Diseases (IBD) such as Ulcerative Colitis and Crohn’s Disease are chronic inflammatory diseases of unknown etiology. The majority of patients are diagnosed early in life and the incidence is increasing; therefore, the effect of IBD on healthcare systems will rise exponentially.
In the last decade, numerous studies demonstrated as perinatal cells can modulate the activity of inflammatory cells such as macrophages, T and B lymphocytes. The easy cell availability and lack of ethical concerns yield PnD a promising tool in regenerative medicine (Zhang et al., 2017a).
The factors involved in the immunomodulatory actions of perinatal cells have not been fully identified but have been suggested to rise from factors secreted either as soluble proteins (secretome) or entrapped in EVs. Perinatal-derived EVs contribute to modulating the immune response in inflammatory bowel diseases (IBD) by directly targeting different elements of the inflammatory microenvironment, ultimately leading to the repair and regeneration of damaged tissues (Tolomeo et al., 2021).
2.5.1 Functional assays to support perinatal derivatives in modulating inflammatory bowel diseases
The efficiency of MSC derived from PnD as well as their secretome (conditioned media or purified EVs) have been tested in chemically-induced experimental colitis mouse models, suggesting as a new and innovative approach for IBD cell-based therapy.
Initial studies have shown that UC-MSC modulate dextran sulfate sodium (DSS) induced acute colitis in NOD.CB17-Prkdc scid/J immunodeficient mice (Banerjee et al., 2015). Recently, human UC-MSC effectively alleviated weight loss, intestinal mucosal injury, colon shortening, and reduced clinical disease phenotype in the DSS-induced mouse IBD model by inhibiting ERK phosphorylation in neutrophils (Wang et al., 2020). In a similar experimental approach, both efficacy and mechanism of action of UC-MSC were further evaluated in acute and chronic mouse models of IBD, established by using a DSS solution. Findings of this study indicated an enhanced expression of the intestinal tight junction protein occludin, downregulation of the expression of the autophagy marker LC3A/B in colon tissue, and upregulated the expression of VEGF-A and VEGFR-1 at the injured site (Panhua et al., 2019). In addition, in a DSS-induced colitis model UC-MSC, when administered with MIS416, a novel microparticle that activates NOD2 and TLR9 signaling was found to improve the pathological phenotype by systemically regulating the immune response, (Lee et al., 2018). In this context, treatment with hAFSC resulted in preventing colon shortening after induction of colitis and dramatically ameliorated the body weight loss induced by the DSS treatment
Similar results were obtained when UC-MSC were administered in a trinitrobenzene sulfonate (TNBS)-induced mouse model of colitis (Liang et al., 2011). More specifically, the administration of UC-MSC reduced the severity of TNBS-induced colitis in mice by increasing anti-inflammatory responses in colons of mice, such as the production of IL-10 and reduced production of inflammatory cytokines (Kim et al., 2013). Moreover, preconditioning of UC-MSC with polyribocytidylic acid enhanced the therapeutic effects of UC-MSC in TNBS-induced colitis (Qiu et al., 2017).
Notably, a number of studies showed as AF-MSC and UC-MSC-derived secreted molecules had a significant therapeutic effect, suggesting a paracrine anti-inflammatory mode of action. Indeed, secretome studies on AF-MSC indicated the presence of anti-inflammatory molecules such as interleukins IL-10, IL-1ra, IL-13, and IL-27; and angiogenic factors like angiopoietin-1, PD-ECGF, uPA that can contribute to the functional improvement of DSS-induced colitis in immunodeficient colitis mouse model phenotype (Legaki et al., 2016). Similarly, intraperitoneal injection of MSC extracts in the DSS-C57BL/6 mouse model reduced colitis, disease activity index, histological colitis scores, and increased body weight (Song et al., 2017). EV-based strategies, including UC-MSC-EVs exerted profound effects on alleviating DSS-induced IBD through the modulation of IL-7 expression in macrophages (Mao et al., 2017b) and induction of MUC2 expression (Tolomeo et al., 2021). Furthermore, recent studies indicated that UC-MSC-EV samples containing miRNA-378a inhibited NLR family pyrin domain containing 3 (NLRP3) inflammasomes and abrogated cell pyroptosis, (Cai et al., 2021) and also restored mucosal barrier repair and intestinal immune homeostasis via TNF-α stimulated gene 6 (Tsg6), to protect against DSS-induced colitis (Yang et al., 2021).
2.5.2 Functional assays to demonstrate the influence of perinatal derivatives in clinic for inflammatory bowel diseases
Experimental approaches have been translated into clinical settings by using stem cell-based therapies for IBDs. Recent data showed that local administration of autologous MSC into perianal fistula leads to an enhanced healing process, promoted fistula relief, and ameliorated clinical complications (Hossein-khannazer et al., 2021).
Interestingly, administration of perinatal MSC to patients with Crohn’s disease was proved safe and well-tolerated, generating decreased Crohn’s disease activity index score and complete remission. Similarly, the application of allogeneic UC-MSC exhibited safety, feasibility, limited or no adverse effects, and led to the improvement of clinical symptoms. However, no significant changes in the levels of inflammatory cytokines in blood serum were detected (Hossein-khannazer et al., 2021; Vieujean et al., 2022).
2.5.3 Conclusion
Overall, cell-based therapy in IBDs demonstrated that MSC are safe and beneficial following anti-inflammatory activity. However, cell-free products (EVs or secretomes in general) may represent an ideal source of anti-inflammatory agents, solving the problem of cell safety and toxicity (Villa et al., 2019; Herrmann et al., 2021). Notwithstanding, additional studies are ongoing to optimize and standardize preparation, administration, and dosage. Accordingly, the development of functional assays to validate PnD products is a prospective direction to modulate microbial structure or intestinal homeostasis.
2.6 Potential role of perinatal derivatives in liver regeneration
Liver dysfunction comprises heterogeneous malfunctions that could potentially lead to acute or chronic liver disease, such as fulminant hepatitis or acute liver disease, advanced fibrosis/cirrhosis, congenital metabolic disorders, or fatty liver disease (Psaraki et al., 2021). Liver transplantation is the gold-standard therapy for many of the afore disorders, limited by organ shortage and the need for a lifelong immunosuppressive regimen. Recent refinements and preclinical/clinical studies have validated alternative approaches where fetal or neonatal liver cells have been infused in patients with chronic or congenital liver disorders (Gramignoli et al., 2015; Bluhme et al., 2022).
Despite innate proliferative capacities, these progenitor cells have shown limited metabolic and synthetic strength, with reduced clinical outcomes. Furthermore, the limited availability of adult human hepatocytes for clinical treatments, in addition to the immunosuppressant regiment required to sustain cellular long-term engraftment have encouraged the use of different cell sources. Over the past years, several groups and companies have been proposing stem cells as an alternative solution, bounded by genetic and epigenetic instabilities, and limited by hepatic maturation level (Bluhme et al., 2022). The additional negative caveat is represented by the chronic inflammatory milieu in response to allograft, which may favor the oncogenic transformation of transplanted cells. Immune-privileged cells such as perinatal stem cells have attracted attention in preclinical studies. Cells isolated from AM have satisfied safety and efficacy requirements, overcoming all the aforementioned risks, and reverting life-threatening acute and congenital liver diseases. Encouraged by lack of tumorigenicity and multipotency potential, hAEC have been transplanted and corrected inborn errors of metabolism such as maple syrup urine disease (MSUD) (Skvorak et al., 2013a; Skvorak et al., 2013b). Phenylketonuria (Strom et al., 2013), and another liver-based disease, is associated with end-stage renal pathologies (atypical hemolytic uraemic syndrome) (Casiraghi et al., 2021). Preclinical analysis revealed correction or normalization in amino acid and neurotransmitter imbalances in human perinatal treated animals, to a superior level reached by syngeneic murine hepatocytes. And the lack of immuno-recognition in xenogenic settings raised attention to the immune-evasive capacity amniotic cells have, rather than an immuno-privileged phenotype.
Allogeneic perinatal cells have been infused to rescue livers in acute failure, supporting parenchymal cell survival and hepatic functions, highlighting remarkable engraftment and anti-inflammatory effects. Both MSC isolated from amniotic fluid and chorionic plate have proved their potential in rescuing intoxicated livers (Lee et al., 2010; Zagoura et al., 2012; Peng et al., 2014).
Xenotransplantation of perinatal cells into immune-competent animals has proved long-term acceptance without the administration of immunosuppressive drugs and resulted in hepatoprotection and regulation of the inflammatory process (Milosavljevic et al., 2017; Shi et al., 2017). However, in clinical situations, liver treatments by allogeneic cells have been proved to require immediate availability (in fulminant hepatitis) and a high number of cells (up to 2 × 108 cells per kg of body weight in metabolic disorders) (Gramignoli et al., 2015). Such challenges have been frequently hampered by limited engraftment capacity by donor cells, as well as lack of selective advantage. Recent clinical trials have tested the supportive role offered by UC-MSC to allogeneic human hepatocytes for the treatment of liver disease (Iansante et al., 2018). Different reports highlighted as injection of UC-WJ-MSC in patients with chronic liver disorders or steatohepatitis ameliorate transaminase levels and lipid profile, reduces bilirubin and hyperglycemic profile, resulting in improved MELD score and overall survival (see (Shahrbaf et al., 2022) for revision of such clinical studies). Beneficial clinical support offered by perinatal cells has been ascribed to repeated infusion rather than one single infusion (Jia et al., 2020), in line with results offered by 3 decades of hepatocyte transplantation (Gramignoli et al., 2015). Another source of perinatal MSC, amniotic membrane, has been described as instrumental to attenuate Kupffer autophagy and prevent Stellate activation (Shahrbaf et al., 2022). Recently, it has been reported the therapeutic effects of intact AMSC and secreted factors in reversing sclerosing cholangitis (Sugiura et al., 2018). The pro-regenerative effect offered by perinatal MSC in advanced fibrotic/cirrhotic conditions has been ascribed to ECM remodeling factors, such as secreted MMPs (Fu et al., 2018), or angiogenic and hepatogenic mediators (Ding et al., 2018; Hoffmann et al., 2020). Indeed, perinatal cells actively interact and crosstalk with (innate and adaptive) immune cells or support hepatic regeneration not only by cell-to-cell interactions but also through paracrine mediators. Several studies suggest as perinatal therapies, adequate for patients with liver diseases exacerbated by deranged inflammation, will most likely benefit from PnD secretome rather than cellular hepatic maturation (Nagaishi et al., 2014; Liu et al., 2015; Trohatou and Ro ubelakis, 2017). The clinical efficacy of CP-MSC or AF-MSC injections has been described in relation to secreted anti-inflammatory (i.e., TGF-β1 and IL-10) or anti-fibrotic (i.e., MMP-2 and MMP-9) mediators (Shahrbaf et al., 2022). But soluble mediators are characterized by shorter half-life and limited paracrine distribution compared to active molecules (surface-bound or miRNA) embedded in EVs.
2.6.1 Hepato-specific functional assays to validate perinatal derivatives in liver disease
In the majority of the published reports, the cell viability test has been the sole evaluation for donor quality before infusion. Viability quantification has been routinely offered and used to validate cell transplantation procedures. Regulatory Agencies, such as FDA or EMA, mandate parameters such as product sterility, cell viability, and identity to be included to validate cellular biopharmaceutical products. PnD products are not relieved by such qualifications. While product sterility (bacteria- or mycoplasma-free) is quite a standardized procedure, routinely performed in every manufacturing site, currently, no consensus on the level of viability for the cell product is widely accepted. In many phase II trials, MSC viability between 70% and 90% is commonly required (Matthay et al., 2019), while primary liver cell preparation cannot be inferior to 60% in viability to allow injection in patients (Gramignoli et al., 2015). Furthermore, cell viability is seldom performed to determine the percentage of viable cells versus death (necrotic) cells, with additional detection of cells in the early stages of the cell death cascade (i.e., apoptosis).
The International committee described epithelial cells isolated from the fetal side of the amniotic membrane as positive for CD49f and CD326 (ISCT committee; (Dominici et al., 2006)) and published a position paper recommending the inclusion of functional assays to couple with identity validation for therapeutic cell products (Viswanathan et al., 2019). Nevertheless, current biosynthetic analysis or quantification of secreted anti-inflammatory/anti-fibrotic mediators can be instrumental only for a therapeutic approach where no stem cell maturation/transdifferentiation is required. Hepato-specific phase I and II activities, bile acid transporters, and urea cycle enzymes are critical to determining long/term correction of congenital liver disorders. In hepatocyte transplant programs, such a multiparametric functional analysis is commonly the minimum criteria in order to confirm cell function (Donato et al., 2008; Bluhme et al., 2022).
Between perinatal cells, hAEC have attracted particular attention for their hepatocyte-like features and can acquire hepatic functions upon transplantation. Epithelial cells released by the amniotic membrane have been proved to secrete low levels of albumin, and upon engraftment in liver parenchyma mature into functional hepatocyte-like cells expressing Cytochrome P450 (CYP) enzymes (as CYP3A4) or secreting proteinases (as A1AT) (Takashima et al., 2004). Ex vivo maturation of stem or progenitor cells into a functional hepatic phenotype is still debated. During the past years, revisional studies elegantly proved as the generation of hepatocyte-like cells starting from somatic or perinatal MSC was not a direct consequence of trans-differentiation into endoderm-like cells, but rather resulting from the fusion between donor cells and recipient’s hepatocytes (Camargo et al., 2004; Willenbring et al., 2004). Differently, epithelial cells isolated from the amniotic membrane have been proved capable to mature into functional, adult parenchymal cells upon being implanted in the liver, precluding any fusion event between donor and recipient cells (Marongiu et al., 2015). Such hepatic maturation has only been partially achieved in 2D culture (M iki et al., 2005; Marongiu et al., 2011; Fanti et al., 2017; Maymó et al., 2018; Passaretta et al., 2020).
Metabolic activities in human hepatocytes or stem cell-derived hepatocyte-like cells can be assessed by incubation with drugs or chemical substrates and analyzed with unexpensive and widely available lab equipment (such as spectrophotometers) (Kim et al., 2005; Gramignoli et al., 2012). Release criteria and a battery of potency assays have been developed and described, where up to eleven different hepatic functions can be simultaneously measured to validate donor cells transplanted to offer support or replace liver cells (Gramignoli et al., 2013; Gramignoli et al., 2014). Indeed, in order to validate multi-functional cells, such as liver cells, a matrix of functional assays has been recommended to support injection of multipotent PnD cells and confirm maturation capacity into periportal or centro-lobular hepatocytes and cholangiocytes. Bio-synthetic and metabolic activities have been frequently coupled with selective transcriptome analysis. Hepatic maturation can be monitored by transcriptomic assays specifically designed to validate stem/progenitor cell transplantations and match patients’ needs (Zabulica et al., 2019).
2.6.2 Liver organoid as novel perinatal derivatives maturation potency assay for liver disease
The therapeutic potential of liver organoids represents an alternative of functional and expandable cells for transplantation, exceeding the current limitations (Prior et al., 2019). Liver organoids have been recently established from different cell sources among which hAEC have currently gained particular attention.
Some authors demonstrated that the combination of different cell types (endothelial, mesenchymal) in a 3D culture system may promote and enhance stem cell differentiation, physiological function, and proliferation ability, providing a tissue engineering strategy, referred to as “building blocks”, for larger tissue constructs (Takebe et al., 2013; Laschke and Menger, 2017; Nie et al., 2018; Lebreton et al., 2019). Recently, multicellular organoids have been generated with hepatic features and functions by combining hAEC with HUVEC and MSC (Furuya et al., 2019). Besides obtaining functional organoids, they evaluated cellular differentiation within organoids through a bioinformatics approach demonstrating a higher level of cell differentiation in comparison with 2D cell cultures.
Additional studies have been conducted to evaluate liver organoids for hepato-specific gene expression and functions, such as CYP3A4 activity and inducibility, albumin secretion, ammonia and metabolism, the ability to efflux rhodamine or store bile acids, lipids, or glycogen (Ramachandran et al., 2015; Pettinato et al., 2019; Wu et al., 2019).
Based on these results, liver organoids could represent a novel in vitro model to study novel therapeutic interventions, but may become important an alternative strategy to organ transplantation for liver failure (Furuya et al., 2019). However, implantation strategies and high engraftment efficiency are crucial to rescue metabolic defects. The percentage of organoid engraftment reported is extremely low (1%) (Huch et al., 2013) and restoring enzyme or protein deficiency requires several billion proficient cells, quantified in approx. 5–10% of total liver mass according to missing enzyme (Gramignoli et al., 2015). It has been reported as liver organoids can mimic structures and genetic signatures, although their biomedical use on a large scale is currently limited to the lack in control of cell size, shape, and composition (Rossi et al., 2018). In this regard, it appears crucial to optimize long-term cultures and differentiation characteristics of liver organoids aimed at generating a tissue architecture closer to the in vivo conditions (Ramachandran et al., 2015). In vitro refinements as well as in vivo validation are in progress.
2.6.3 Conclusion
The liver, with its unique regenerative capacity and plethora of functions critical for life, is most likely one of the organs that can largely benefit from PnD supportive strategies to enhance regeneration rather than organ replacement.
Cell-based therapies have proved safety and efficacy for liver regeneration or inborn errors of metabolism correction. However, a reliable, stable source of functional hepatocyte-like cells is still pending to offer such a strategy to millions of patients with congenital or chronic liver disease. Perinatal cells such as MSC or hAEC have been recognized with constitutive and robust anti-inflammatory and anti-fibrotic properties, qualifying these PnD as suitable bioproducts for inflammatory conditions such as hepatitis, fibrosis, and cirrhosis. However, to correct enzymatic defects, a large amount of proficient and metabolic active cells is needed. Convincing evidence in support of the maturation of epithelial cells isolated from AM is encouraging. Moving from the fact that hAEC originates from epiblast during the second week of embryo development and display the presence of early-phase hepatic markers, such PnD product is motivating translation into phase I and II clinical trials. Conversely, transdifferentiation of MSC into functional hepatocyte-like cells has raised more concerns than solid evidence and will most likely restrict their use to paracrine effects or secretome approaches in the near future.
Major challenge in many published studies is validating progenitor/stem cell maturation into functional hepatocytes. Transcriptomic analysis has represented an easy and relatively cheap method to initially test PnD, but such analysis needs to be coupled with real metabolic activity and performed in direct comparison with primary human hepatocytes.
The involvement of metabolic enzymes characteristic of mature, functional somatic cells and the current lack of standardized and reproducible protocols able to induce hepatic maturation on any progenitor or stem cell in vitro to a level that corresponds to postnatal liver cells (Zabulica et al., 2019) makes hepatic potency assay refinement even more complex than any other organ, where immunomodulation or inflammatory governance is the sole or major goals.
Finally, concerning the liver organoid approach, whilst many 3D liver models are described as organoids, not all of them are equally organotypic since in most cases they are aggregates of a single cell type or mixed cell types rather than containing both parenchymal and non-parenchymal cells of the liver necessary for modeling inflammation and liver disease. Although this approach still needs further validation in vitro and in vivo, to circumvent the issue of angiogenesis, innervation or survival of encapsulated hepatocytes, PnD-derived organoids deserve much attention in comparison both with iPSC-derived organoids, which imply the risk to develop teratomas and with adult stem cells-derived organoids which show a lack of heterogeneity in the starting population.
2.7 Potential role of perinatal derivatives on lung fibrosis
Idiopathic pulmonary fibrosis (IPF) is a progressive, fatal, chronic interstitial lung disease characterized by aberrant extracellular matrix deposition leading to loss of normal lung architecture and respiratory capacity. In Europe and North America, IPF has an incidence of 3–9 cases per 100,000 people and is increasing worldwide (Scelfo et al., 2017).
Although IPF etiology remains unknown, it is generally considered a dysregulated wound healing process mediated by resident fibroblasts and recruited fibrocytes which are activated to myofibroblasts (the latter of which are mostly responsible for extracellular matrix production) by repetitive alveolar injuries (induced for example by environmental exposures, smoking, viral infections and by genetic predispositions). However, more recent findings evidenced the contribution of diverse immune cell types, belonging to both innate (neutrophils, monocytes, macrophages) and adaptative (T and B lymphocytes) immune systems, in the initiation and progression of fibrotic lesion in IPF patients (Desai et al., 2018; Heukels et al., 2019).
In this context, PnD have been applied in lung fibrosis models with the purpose either to counteract the proliferation and collagen production of lung myofibroblasts and also to modulate the inflammatory response involved in fibrosis initiation and progression.
2.7.1 Lung-specific functional assays to validate perinatal derivatives in lung fibrosis
Due to the multiple cellular actors involved in the pathogenesis of IPF and the consequent implication of different pathways contributing either to fibrogenesis and fibrolysis, in vitro models of fibrosis do not resemble the complex conditions of in vivo fibrotic tissues. The general in vitro approach is to analyze the effects of PnD on in vitro models of inflammation-independent fibrosis, for example, the assessment of their direct effects on the proliferation, collagen production, and the secretion of profibrotic cytokines (e.g., TGF-β, PDGF-a, and PDGF-b), of lung fibroblasts activated to myofibroblasts with TGF-β (Li et al., 2008; Vosdoganes et al., 2013).
Recently, 3D in vitro lung models have been developed in the effort to reproduce the cellular and functional complexity of pulmonary tissue and possibly recapitulate the cellular and structural alterations present in the lungs of patients with IPF. Lung organoids have been developed by combining collagen-functionalized alginate beads and induced pluripotent stem cell (iPSC) derived from human lung fibroblasts in a rotational bioreactor (Wilkinson et al., 2017). To generate a model of progressive scarring, as found in IPF patients, organoids have been treated with exogenous TGF-β1 and observed increased expression of collagen 1, α-SMA, and the emergence of fibroblastic foci confirming the possibility to model lung fibrosis in vitro.
Lung spheroids (called pulmospheres) have been developed using cells and extracellular matrix components isolated from lung biopsies obtained from IPF patients (Surolia et al., 2017) and they have been applied to evaluate the responsiveness of individual patients to antifibrotic drugs.
The limitations of the in vitro lung disease modeling make it so that studies focused on establishing the potential anti-fibrotic effects of PnD have been performed on animal models evaluating a series of cellular/humoral/tissue parameters. Specifically, PnDs, and mainly hAEC and hAMSC, have been administered in animals with bleomycin-induced pulmonary fibrosis to evaluate either their ability to reduce lung inflammation and their potential to slow down the progression and even to reverse the fibrotic lesion.
In these studies, the anti-inflammatory ability of PnD was evaluated in terms of reduction of inflammation and immune cell infiltration in lung parenchyma and broncho-alveolar lavage. Specifically, these were observed as: 1) a reduction of neutrophils (Cargnoni et al., 2009; Murphy et al., 2011) and macrophages in the lungs (Tan et al., 2014); 2) increased lung levels of regulatory T cells (Treg), able to suppress T cell activation (Tan et al., 2015); 3) promotion of macrophage polarization toward an anti-inflammatory phenotype (M2 macrophages) (Murphy et al., 2012; Tan et al., 2014; Tan et al., 2018); 4) reduction of lung levels of proinflammatory cytokines (MCP-1, TNF-a, IL-1, IL-6) (Moodley et al., 2010; Murphy et al., 2011) and chemokines (CXCL12, CXCL13, BAFF (Carg noni et al., 2020)); 5) increase of tissue levels of cytokines and molecules with anti-inflammatory activities, such as IL-10 (Carg noni et al., 2020) and TSG-6 (Moodley et al., 2013); 6) reduction in the number and in the maturation ability of B cells recruited in lung parenchyma (Carg noni et al., 2020); and 7) reduction in the number and size of Tertiary Lymphoid Organs (TLO) (Carg noni et al., 2020), T and B cell aggregates found in the lungs of IPF patients and that correlate with inflammation chronicization and severity of lung fibrotic lesions (Todd et al., 2013).
Associated and possibly mediated by the ability to counteract the inflammatory response and the chronicization of lung inflammatory processes, PnD (hAEC and hAMSC) exhibited the ability to interfere with the progression of lung fibrotic lesions. Lung fibrosis has been evaluated through the assessment of parameters/factors involved either in fibrogenesis or in fibrolysis. Indeed, it has been observed that PnD decreases lung levels of TGF-β1 (Moodley et al., 2010), a pro-fibrotic growth factor with a crucial role in triggering the activation of lung fibroblasts to myofibroblasts (α-SMA positive cells) (Murphy et al., 2012; Vosdoganes et al., 2013; Carg noni et al., 2020) and lung accumulation of collagen and fibronectin (Vosdoganes et al., 2013; Carg noni et al., 2020). Instead, PnD appears to promote fibrolysis by increasing lung levels of MMPs and by decreasing their inhibitors TIMPs (Moodley et al., 2010).
UC-MSC, similar to hAEC and hAMSC, were recently reported to reduce collagen deposition and lung IL-6 expression in bleomycin-challenged mice when administered twice (24 h and 7 days post-bleomycin) (Moroncini et al., 2018). However, differently from the other PnD, hUC-MSC treatment reduced lung levels of M2 macrophages.
It must be underlined that the anti-inflammatory and anti-fibrotic effects of perinatal cells were observed regardless of any significant engraftment of these cells in the injured lung after administration (Cargnoni et al., 2009; Murphy et al., 2011), suggesting that secreted factors, rather than cells per sé, act via a paracrine mechanism on host cells. Indeed, both conditioned medium generated from the in vitro culture of hAMSC (hAMSC-CM) and EVs from hAEC (hAEC-EVs), have been shown to exert beneficial actions in a bleomycin-induced lung fibrosis model. The treatment with hAMSC-CM preserved blood gas parameters of bleomycin-challenged mice and reduced lung levels of pro-inflammatory and pro-fibrotic cytokines (IL-6, TNF-α, MIP-1α, MCP-1 TGF-β) associated with reduced lung macrophage levels (Cargnoni et al., 2014). Intranasal instillation of hAEC-Exo administered early, on day 1 post bleomycin challenge, reduced lung inflammation while treatment on day 7 improved tissue-to-airspace ratio and reduced fibrosis (Tan et al., 2018).
2.7.2 Conclusion
The experimental evidence supporting the anti-inflammatory and anti-fibrotic actions of PnD (cells and derived secretome and EV) has encouraged their application in clinical studies. In particular, placental stromal cells (Chambers et al., 2014) and hUC-MSC (Zhang et al., 2017b) were transplanted in IPF patients with null/mild side effects. More recently, to limit the immunopathogenic effects of cytokine storm, hUC-MSC were used, in combination with the standard optimal care, to treat COVID-19 patients with ARDS (Meng et al., 2020; Shu et al., 2020). The studies performed until now indicated that PnD administration is feasible and safe (Lanzoni et al., 2021), however, clinical trials with a large population of patients are needed to demonstrate the potential efficacy of PnD in human diseases.
3 Overall message
The use of PnD in regenerative medicine covers the employment of scaffolds after decellularization or de-epithelization, stem cells, and secretome including EVs. Recent preclinical and clinical results propose PnD derivatives as safe and effective biological products, whereas their therapeutic application and effectiveness remain challenged. Potency assays are designed to certify the mechanism of action of starting material, pre- or post-commitment.
Any PnD technology is required to satisfy strict release criteria, based on the quality of cell products as well as potency measurements validating specific capacity. Unlike conventional release criteria based on static marker qualification (immune-cytological analysis), specific potency assays are less standardized and cannot be performed on donor cells inefficiently or partially committed over a specific phenotype.
Multiple factors influence treatment outcomes and the proposed potency assays aim to mark a new path to standardize PnD application. This manuscript aimed at offering a guide on when and how it may be advantageous for the employment of placenta-derived cells or scaffolds. The described potency assays can serve as the first tool to standardize PnD derivation and usage, and the second instance may contribute to match recipients’ needs with donor characteristics. We are aware that standardization in manufacturing and qualification represents the main limitations hampering a more intensive and extensive use of PnD in clinical trials, so far. A current lack of consensus in the scientific community has limited PnD use in clinical settings, although such derivatives have been reported well-tolerated and refractory to the host’s immunorecognition and rejection. To establish and predict PnD functionality, COST working group of experts has been actively recruited and worked to establish a clear set of laboratory tests that can be conducted in every research or transplant centers, using common laboratory equipment and fulfilling regulatory agencies’ requirements. Different validation analyses will likely require both in vitro 2D/3D tests to refine activity and modalities, correlated by in vivo validations using human-related animal models. As result, we can envision in the near future, a guide compiled by the international community that will standardize the assays, detail advantages and disadvantages, and tailor the implementation of PnD according to patient’s needs. Any ethical or religious issues have been depicted during the past years, since perinatal cells and tissue are commonly considered waste material (at least in the vast majority of medical centers) and may represent a new perspective in regenerative and interventional medicine, upon standardization and validation of the afore processes.
Data availability statement
The original contributions presented in the study are included in the article/Supplementary Material, and further inquiries can be directed to the corresponding authors.
Author contributions
SD, MR, AC, RG, SW, FG, SB, HK, MF, RD, MB, VB, RDP, AS, MP, wrote the specific parts of the manuscript and the table. AS draw the figure. MP, PP, coordinated the work and compiled the manuscript. All authors contributed to revising and editing the manuscript and approved the manuscript.
Funding
This work contributes to the COST Action CA17116 International Network for Translating Research on Perinatal Derivatives into Therapeutic Approaches (SPRINT), supported by COST (European Cooperation in Science and Technology). SDA is supported by the Grant 27/01, Foundation Institute of Pediatric Research Città della Speranza, Italy, PI: MP.
Conflict of interest
The authors declare that the research was conducted in the absence of any commercial or financial relationships that could be construed as a potential conflict of interest.
Publisher’s note
All claims expressed in this article are solely those of the authors and do not necessarily represent those of their affiliated organizations, or those of the publisher, the editors and the reviewers. Any product that may be evaluated in this article, or claim that may be made by its manufacturer, is not guaranteed or endorsed by the publisher.
References
Abreu, S. C., Weiss, D. J., and Rocco, P. R. M. (2016). Extracellular vesicles derived from mesenchymal stromal cells: A therapeutic option in respiratory diseases? Stem Cell Res. Ther. 7 (1), 53. doi:10.1186/s13287-016-0317-0
Arutyunyan, I., Fatkhudinov, T., Elchaninov, A., Vasyukova, O., Makarov, A., Usman, N., et al. (2019). Umbilical cord-derived mesenchymal stromal/stem cells enhance recovery of surgically induced skeletal muscle ischemia in a rat model. Histol. Histopathol. 34 (5), 513–523. doi:10.14670/HH-18-057
Balbi, C., Costa, A., Barile, L., and Bollini, S. (2020). Message in a bottle: Upgrading cardiac repair into rejuvenation. Cells 9 (3), 724. doi:10.3390/cells9030724
Balbi, C., Lodder, K., Costa, A., Moimas, S., Moccia, F., van Herwaarden, T., et al. (2019). Reactivating endogenous mechanisms of cardiac regeneration via paracrine boosting using the human amniotic fluid stem cell secretome. Int. J. Cardiol. 287, 87–95. doi:10.1016/j.ijcard.2019.04.011
Balbi, C., Lodder, K., Costa, A., Moimas, S., Moccia, F., van Herwaarden, T., et al. (2019). Supporting data on in vitro cardioprotective and proliferative paracrine effects by the human amniotic fluid stem cell secretome. Data Brief. 25, 104324. doi:10.1016/j.dib.2019.104324
Banerjee, A., Bizzaro, D., Burra, P., Di Liddo, R., Pathak, S., Arcidiacono, D., et al. (2015). Umbilical cord mesenchymal stem cells modulate dextran sulfate sodium induced acute colitis in immunodeficient mice. Stem Cell Res. Ther. 6 (1), 79. doi:10.1186/s13287-015-0073-6
Barboni, B., Mangano, C., Valbonetti, L., Marruchella, G., Berardinelli, P., Martelli, A., et al. (2013). Synthetic bone substitute engineered with amniotic epithelial cells enhances bone regeneration after maxillary sinus augmentation. PLoS One 8 (5), e63256. doi:10.1371/journal.pone.0063256
Bier, A., Berenstein, P., Kronfeld, N., Morgoulis, D., Ziv-Av, A., Goldstein, H., et al. (2018). Placenta-derived mesenchymal stromal cells and their exosomes exert therapeutic effects in Duchenne muscular dystrophy. Biomaterials 174, 67–78. doi:10.1016/j.biomaterials.2018.04.055
Bluhme, E., Henckel, E., Gramignoli, R., Kjellin, T., Hammarstedt, C., Nowak, G., et al. (2022). Procurement and evaluation of hepatocytes for transplantation from neonatal donors after circulatory death. Cell Transpl. 31, 096368972110699. doi:10.1177/09636897211069900
Bojanic, C., To, K., Zhang, B., Mak, C., and Khan, W. S. (2020). Human umbilical cord derived mesenchymal stem cells in peripheral nerve regeneration. World J. Stem Cells 12 (4), 288–302. doi:10.4252/wjsc.v12.i4.288
Bollini, S., Smits, A. M., Balbi, C., Lazzarini, E., and Ameri, P. (2018). Triggering endogenous cardiac repair and regeneration via extracellular vesicle-mediated communication. Front. Physiol. 9, 1497. doi:10.3389/fphys.2018.01497
Bourgeois, M., Loisel, F., Obert, L., Pluvy, I., and Gindraux, F. (2019). Can the amniotic membrane be used to treat peripheral nerve defects? A review of literature. Hand Surg. Rehabil. 38 (4), 223–232. doi:10.1016/j.hansur.2019.05.006
Choi, J. S., Kim, J. D., Yoon, H. S., and Cho, Y. W. (2012). Full-thickness skin wound healing using human placenta-derived extracellular matrix containing bioactive molecules. Tissue Eng. Part A 19 (3–4), 329–339. doi:10.1089/ten.tea.2011.0738
Cai, X., Zhangyu, Z., Yuantao, J., Ocansey, D. K. W., Tu, Q., Zhang, X., et al. (2021). hucMSC-derived exosomes attenuate colitis by regulating macrophage pyroptosis via the miR-378a-5p/NLRP3 axis. Stem Cell Res. Ther. 12 (1), 416. doi:10.1186/s13287-021-02492-6
Camargo, F. D., Finegold, M., and Goodell, M. A. (2004). Hematopoietic myelomonocytic cells are the major source of hepatocyte fusion partners. J. Clin. Invest. 113 (9), 1266–1270. doi:10.1172/jci21301
Campisi, M., Shin, Y., Osaki, T., Hajal, C., Chiono, V., Kamm, R. D., et al. (2018). 3D self-organized microvascular model of the human blood-brain barrier with endothelial cells, pericytes and astrocytes. Biomaterials 180, 117–129. doi:10.1016/j.biomaterials.2018.07.014
Cao, D., Qiao, H., He, D., Qin, X., Zhang, Q., Zhou, Y., et al. (2019). Mesenchymal stem cells inhibited the inflammation and oxidative stress in LPS-activated microglial cells through AMPK pathway. J. Neural Transm. (Vienna). 126 (12), 1589–1597. doi:10.1007/s00702-019-02102-z
Cargnoni, A., Romele, P., Bonassi Signoroni, P., Farigu, S., Magatti, M., Vertua, E., et al. (2020). Amniotic MSCs reduce pulmonary fibrosis by hampering lung B-cell recruitment, retention, and maturation. Stem Cells Transl. Med. 9 (9), 1023–1035. doi:10.1002/sctm.20-0068
Cargnoni, A., Gibelli, L., Tosini, A., Signoroni, P. B., Nassuato, C., Arienti, D., et al. (2009). Transplantation of allogeneic and xenogeneic placenta-derived cells reduces bleomycin-induced lung fibrosis. Cell Transpl. 18 (4), 405–422. doi:10.3727/096368909788809857
Cargnoni, A., Piccinelli, E. C., Ressel, L., Rossi, D., Magatti, M., Toschi, I., et al. (2014). Conditioned medium from amniotic membrane-derived cells prevents lung fibrosis and preserves blood gas exchanges in bleomycin-injured mice-specificity of the effects and insights into possible mechanisms. Cytotherapy 16 (1), 17–32. doi:10.1016/j.jcyt.2013.07.002
Casiraghi, F., Ordonez, P. Y. R., Azzollini, N., Todeschini, M., Rottoli, D., Donadelli, R., et al. (2021). Amnion epithelial cells are an effective source of factor H and prevent kidney complement deposition in factor H-deficient mice. Stem Cell Res. Ther. 12 (1), 332. doi:10.1186/s13287-021-02386-7
Cecchelli, R., Aday, S., Sevin, E., Almeida, C., Culot, M., Dehouck, L., et al. (2014). A stable and reproducible human blood-brain barrier model derived from hematopoietic stem cells. PLoS One 9 (6), e99733. doi:10.1371/journal.pone.0099733
Chambers, D. C., Enever, D., Ilic, N., Sparks, L., Whitelaw, K., Ayres, J., et al. (2014). A phase 1b study of placenta-derived mesenchymal stromal cells in patients with idiopathic pulmonary fibrosis. Respirology 19 (7), 1013–1018. doi:10.1111/resp.12343
Chen, C. C., Liu, L., Ma, F., Wong, C. W., Guo, X. E., Chacko, J. V., et al. (2016). Elucidation of exosome migration across the blood–brain barrier model in vitro. Cell. Mol. Bioeng. 9 (4), 509–529. doi:10.1007/s12195-016-0458-3
Chen, T. S., Lai, R. C., Lee, M. M., Choo, A. B. H., Lee, C. N., Lim, S. K., et al. (2010). Mesenchymal stem cell secretes microparticles enriched in pre-microRNAs. Nucleic Acids Res. 38 (1), 215–224. doi:10.1093/nar/gkp857
Chen, W., Chang, S., Yang, C., Zhou, J., Zhang, H., Nie, K., et al. (2022). Schwann cell-like cells derived from human amniotic mesenchymal stem cells promote sciatic nerve repair through an exosome-induced SOX2/FN1 pathway in vitro. Int. J. Mol. Med. 49 (6), 80. doi:10.3892/ijmm.2022.5136
Chen, W., Liu, J., Manuchehrabadi, N., Weir, M. D., Zhu, Z., Xu, H. H. K., et al. (2013). Umbilical cord and bone marrow mesenchymal stem cell seeding on macroporous calcium phosphate for bone regeneration in rat cranial defects. Biomaterials 34 (38), 9917–9925. doi:10.1016/j.biomaterials.2013.09.002
Crapo, P. M., Gilbert, T. W., and Badylak, D. V. M. (2011). An overview of tissue and whole organ decellularization processes. Biomaterials 32 (12), 3233–3243. doi:10.1016/j.biomaterials.2011.01.057
Danieli, P., Malpasso, G., Ciuffreda, M. C., Cervio, E., Calvillo, L., Copes, F., et al. (2015). Conditioned medium from human amniotic mesenchymal stromal cells limits infarct size and enhances angiogenesis. Stem Cells Transl. Med. 4 (5), 448–458. doi:10.5966/sctm.2014-0253
Desai, O., Winkler, J., Minasyan, M., and Herzog, E. L. (2018). The role of immune and inflammatory cells in idiopathic pulmonary fibrosis. Front. Med. 5, 43. doi:10.3389/fmed.2018.00043
Ding, C., Zou, Q., Wang, F., Wu, H., Chen, R., Lv, J., et al. (2018). Human amniotic mesenchymal stem cells improve ovarian function in natural aging through secreting hepatocyte growth factor and epidermal growth factor. Stem Cell Res. Ther. 9 (1), 55. doi:10.1186/s13287-018-0781-9
Dominici, M., Le Blanc, K., Mueller, I., Slaper-Cortenbach, I., Marini, F. C., Krause, D. S., et al. (2006). Minimal criteria for defining multipotent mesenchymal stromal cells. The International Society for Cellular Therapy position statement. Cytotherapy 8 (4), 315–317. doi:10.1080/14653240600855905
Donato, M. T., Lahoz, A., Montero, S., Bonora, A., Pareja, E., Mir, J., et al. (2008). Functional assessment of the quality of human hepatocyte preparations for cell transplantation. Cell Transpl. 17 (10–11), 1211–1219. doi:10.3727/096368908787236620
Dubus, M., Scomazzon, L., Chevrier, J., Ledouble, C., Baldit, A., Braux, J., et al. (2022). Antibacterial and immunomodulatory properties of acellular wharton’s jelly matrix. Biomedicines 10 (2), 227. doi:10.3390/biomedicines10020227
El Andaloussi, S., Mäger, I., Breakefield, X. O., and Wood, M. J. A. (2013). Extracellular vesicles: Biology and emerging therapeutic opportunities. Nat. Rev. Drug Discov. 12 (5), 347–357. doi:10.1038/nrd3978
Eschenhagen, T., Bolli, R., Braun, T., Field, L. J., Fleischmann, B. K., Frisén, J., et al. (2017). Cardiomyocyte regeneration. Circulation 136 (7), 680–686. doi:10.1161/circulationaha.117.029343
Etchebarne, M., Fricain, J-C., Kerdjoudj, H., Di Pietro, R., Wolbank, S., Gindraux, F., et al. (2021). Use of amniotic membrane and its derived products for bone regeneration: A systematic review. Front. Bioeng. Biotechnol. 9, 661332. doi:10.3389/fbioe.2021.661332
Fenelon, M., Etchebarne, M., Siadous, R., Grémare, A., Durand, M., Sentilhes, L., et al. (2020). Assessment of fresh and preserved amniotic membrane for guided bone regeneration in mice. J. Biomed. Mater. Res. A 108 (10), 2044–2056. doi:10.1002/jbm.a.36964
Fanti, M., Gramignoli, R., Serra, M., Cadoni, E., Strom, S. C., Marongiu, F., et al. (2017). Differentiation of amniotic epithelial cells into various liver cell types and potential therapeutic applications. Placenta 59, 139–145. doi:10.1016/j.placenta.2017.03.020
Fenelon, M., B Maurel, D., Siadous, R., Gremare, A., Delmond, S., Durand, M., et al. (2019). Comparison of the impact of preservation methods on amniotic membrane properties for tissue engineering applications. Mater Sci. Eng. C Mater Biol. Appl. 104, 109903. doi:10.1016/j.msec.2019.109903
Fénelon, M., Catros, S., Meyer, C., Fricain, J. C., Obert, L., Auber, F., et al. (2021). Applications of human amniotic membrane for tissue engineering. Membr. (Basel) 11 (6), 387. doi:10.3390/membranes11060387
Fénelon, M., Catros, S., Meyer, C., Fricain, J. C., Obert, L., Auber, F., et al. (20212021). Applications of human amniotic membrane for tissue engineering. Membr. (Basel). 1111 (6), 387. doi:10.3390/membranes11060387
Fenelon, M., Etchebarne, M., Siadous, R., Grémare, A., Durand, M., Sentilhes, L., et al. (2021). Comparison of amniotic membrane versus the induced membrane for bone regeneration in long bone segmental defects using calcium phosphate cement loaded with BMP-2. Mater. Sci. Eng. C 124, 112032. doi:10.1016/j.msec.2021.112032
Fleury, A., Martinez, M. C., and Le Lay, S. (2014). Extracellular vesicles as therapeutic tools in cardiovascular diseases. Front. Immunol. 5, 370. doi:10.3389/fimmu.2014.00370
Foglia, M. J., and Poss, K. D. (2016). Building and re-building the heart by cardiomyocyte proliferation. Development 143 (5), 729–740. doi:10.1242/dev.132910
Fu, Q., Ohnishi, S., and Sakamoto, N. (2018). Conditioned medium from human amnion-derived mesenchymal stem cells regulates activation of primary hepatic stellate cells. Stem Cells Int. 2018, 1–11. doi:10.1155/2018/4898152
Furuya, K., Zheng, Y. W., Sako, D., Iwasaki, K., Zheng, D. X., Ge, J. Y., et al. (2019). Enhanced hepatic differentiation in the subpopulation of human amniotic stem cells under 3D multicellular microenvironment. World J. Stem Cells 11 (9), 705–721. doi:10.4252/wjsc.v11.i9.705
Gilbert, T. W., Sellaro, T. L., and Badylak, S. F. (2006). Decellularization of tissues and organs. Biomaterials 27 (19), 3675–3683. doi:10.1016/j.biomaterials.2006.02.014
Gindraux, F., Loisel, F., Bourgeois, M., Oudina, K., Melin, M., de Billy, B., et al. (2020). Induced membrane maintains its osteogenic properties even when the second stage of Masquelet’s technique is performed later. Eur. J. Trauma Emerg. Surg. 46 (2), 301–312. doi:10.1007/s00068-019-01184-4
Gnecchi, M., Danieli, P., and Cervio, E. (2012). Mesenchymal stem cell therapy for heart disease. Vasc. Pharmacol. 57 (1), 48–55. doi:10.1016/j.vph.2012.04.002
Golchin, A., and Farahany, T. Z. (2019). Biological products: Cellular therapy and FDA approved products. Stem Cell Rev. Rep. 15 (2), 166–175. doi:10.1007/s12015-018-9866-1
Gorina, R., Santalucia, T., Petegnief, V., Ejarque-Ortiz, A., Saura, J., Planas, A. M., et al. (2009). Astrocytes are very sensitive to develop innate immune responses to lipid-carried short interfering RNA. Glia 57 (1), 93–107. doi:10.1002/glia.20738
Gramignoli, R., Green, M. L., Tahan, V., Dorko, K., Skvorak, K. J., Marongiu, F., et al. (2012). Development and application of purified tissue dissociation enzyme mixtures for human hepatocyte isolation. Cell Transpl. 21 (6), 1245–1260. doi:10.3727/096368911x600939
Gramignoli, R., Tahan, V., Dorko, K., Skvorak, K. J., Hansel, M. C., Zhao, W., et al. (2013). New potential cell source for hepatocyte transplantation: Discarded livers from metabolic disease liver transplants. Stem Cell Res. 11 (1), 563–573. doi:10.1016/j.scr.2013.03.002
Gramignoli, R., Tahan, V., Dorko, K., Venkataramanan, R., Fox, I. J., Ellis, E. C. S., et al. (2014). Rapid and sensitive assessment of human hepatocyte functions. Cell Transpl. 23 (12), 1545–1556. doi:10.3727/096368914x680064
Gramignoli, R., Vosough, M., Kannisto, K., Srinivasan, R. C., and Strom, S. C. (2015). Clinical hepatocyte transplantation: Practical limits and possible solutions. Eur. Surg. Res. 54 (3–4), 162–177. doi:10.1159/000369552
Gualdi, T., Laurent, R., Moutarlier, V., Fenelon, M., Nallet, A., Pouthier, F., et al. (2019). In vitro osteodifferentiation of intact human amniotic membrane is not beneficial in the context of bone repair. Cell Tissue Bank. 20 (3), 435–446. doi:10.1007/s10561-019-09778-3
Hadipour, A., Bayati, V., Rashno, M., and Orazizadeh, M. (2021). Aligned poly(ε-caprolactone) nanofibers superimposed on decellularized human amniotic membrane promoted myogenic differentiation of adipose derived stem cells. Cell J. 23 (6), 603–611. doi:10.22074/cellj.2021.7261
Hao, Y., Ma, D. H. K., Hwang, D. G., Kim, W. S., and Zhang, F. (2000). Identification of antiangiogenic and antiinflammatory proteins in human amniotic membrane. Cornea 19 (3), 348–352. doi:10.1097/00003226-200005000-00018
Hasmad, H., Yusof, M. R., Mohd Razi, Z. R., Idrus, R. B., and Chowdhury, S. R. (2018). Human amniotic membrane with aligned electrospun fiber as scaffold for aligned tissue regeneration. Tissue Eng. Part C. Methods 24 (6), 368–378. doi:10.1089/ten.tec.2017.0447
Hasselmann, J., and Blurton-Jones, M. (2020). Human iPSC-derived microglia: A growing toolset to study the brain’s innate immune cells. Glia 68 (4), 721–739. doi:10.1002/glia.23781
Herrmann, I. K., Wood, M. J. A., and Fuhrmann, G. (2021). Extracellular vesicles as a next-generation drug delivery platform. Nat. Nanotechnol. 16 (7), 748–759. doi:10.1038/s41565-021-00931-2
Heukels, P., Moor, C. C., von der Thüsen, J. H., Wijsenbeek, M. S., and Kool, M. (2019). Inflammation and immunity in IPF pathogenesis and treatment. Respir. Med. X. 147, 79–91. doi:10.1016/j.rmed.2018.12.015
Hodgkinson, C. P., Bareja, A., Gomez, J. A., and Dzau, V. J. (2016). Emerging concepts in paracrine mechanisms in regenerative cardiovascular medicine and biology. Circ. Res. 118 (1), 95–107. doi:10.1161/circresaha.115.305373
Hoffmann, K., Nagel, A. J., Tanabe, K., Fuchs, J., Dehlke, K., Ghamarnejad, O., et al. (2020). Markers of liver regeneration—the role of growth factors and cytokines: A systematic review. BMC Surg. 20 (1), 31. doi:10.1186/s12893-019-0664-8
Holloway, R. K., Ireland, G., Sullivan, G., Becher, J. C., Smith, C., Boardman, J. P., et al. (2021). Microglial inflammasome activation drives developmental white matter injury. Glia 69 (5), 1268–1280. doi:10.1002/glia.23963
Hossein-khannazer, N., Torabi, S., Hosseinzadeh, R., Shahrokh, S., Asadzadeh Aghdaei, H., Memarnejadian, A., et al. (2021). Novel cell-based therapies in inflammatory bowel diseases: The established concept, promising results. Hum. Cell 34 (5), 1289–1300. doi:10.1007/s13577-021-00560-w
Huang, L., Yang, L., Ding, Y., Jiang, X., Xia, Z., You, Z., et al. (2020). Human umbilical cord mesenchymal stem cells-derived exosomes transfers microRNA-19a to protect cardiomyocytes from acute myocardial infarction by targeting SOX6. Cell Cycle 19 (3), 339–353. doi:10.1080/15384101.2019.1711305
Huch, M., Dorrell, C., Boj, S. F., Van Es, J. H., Li, V. S. W., Van De Wetering, M., et al. (2013). In vitro expansion of single Lgr5 + liver stem cells induced by Wnt-driven regeneration. Nature 494 (7436), 247–250. doi:10.1038/nature11826
Hussein, K. H., Park, K. M., Kang, K. S., and Woo, H. M. (2016). Biocompatibility evaluation of tissue-engineered decellularized scaffolds for biomedical application. Mater. Sci. Eng. C 67, 766–778. doi:10.1016/j.msec.2016.05.068
Iansante, V., Mitry, R. R., Filippi, C., Fitzpatrick, E., and Dhawan, A. (2018). Human hepatocyte transplantation for liver disease: Current status and future perspectives. Pediatr. Res. 83 (1), 232–240. doi:10.1038/pr.2017.284
Izadi, M. R., Habibi, A., Khodabandeh, Z., and Nikbakht, M. (2021). Synergistic effect of high-intensity interval training and stem cell transplantation with amniotic membrane scaffold on repair and rehabilitation after volumetric muscle loss injury. Cell Tissue Res. 383 (2), 765–779. doi:10.1007/s00441-020-03304-8
Jadalannagari, S., Converse, G., McFall, C., Buse, E., Filla, M., Villar, M. T., et al. (2017). Decellularized Wharton’s Jelly from human umbilical cord as a novel 3D scaffolding material for tissue engineering applications. PLoS One 12 (2), e0172098. doi:10.1371/journal.pone.0172098
Jamieson, J. J., Linville, R. M., Ding, Y. Y., Gerecht, S., and Searson, P. C. (2019). Role of iPSC-derived pericytes on barrier function of iPSC-derived brain microvascular endothelial cells in 2D and 3D. Fluids Barriers CNS 16 (1), 15. doi:10.1186/s12987-019-0136-7
Jia, Y., Shu, X., Yang, X., Sun, H., Cao, H., Cao, H., et al. (2020). Enhanced therapeutic effects of umbilical cord mesenchymal stem cells after prolonged treatment for HBV-related liver failure and liver cirrhosis. Stem Cell Res. Ther. 11 (1), 277. doi:10.1186/s13287-020-01787-4
Jin, H., Yang, Q., Ji, F., Zhang, Y., Zhao, Y., Luo, M., et al. (2015). Human amniotic epithelial cell transplantation for the repair of injured brachial plexus nerve: Evaluation of nerve viscoelastic properties. Neural Regen. Res. 10 (2), 260. doi:10.4103/1673-5374.152380
Jin, Y. Z., and Lee, J. H. (2018). Mesenchymal stem cell therapy for bone regeneration. Clin. Orthop. Surg. 10 (3), 271. doi:10.4055/cios.2018.10.3.271
Joerger-Messerli, M. S., Thomi, G., Haesler, V., Keller, I., Renz, P., Surbek, D. V., et al. (2021). Human wharton’s jelly mesenchymal stromal cell-derived small extracellular vesicles drive oligodendroglial maturation by restraining MAPK/ERK and notch signaling pathways. Front. Cell Dev. Biol. 9, 622539. doi:10.3389/fcell.2021.622539
Keane, T. J., Londono, R., Turner, N. J., and Badylak, S. F. (2012). Consequences of ineffective decellularization of biologic scaffolds on the host response. Biomaterials 33 (6), 1771–1781. doi:10.1016/j.biomaterials.2011.10.054
Kim, H., Shin, T., Lee, B., Yu, K., Seo, Y., Lee, S., et al. (2013). Human umbilical cord blood mesenchymal stem cells reduce colitis in mice by activating NOD2 signaling to COX2. Gastroenterology 145 (6), 1392–1403.e8. e8. doi:10.1053/j.gastro.2013.08.033
Kim, M. J., Kim, H., Cha, I. J., Park, J. S., Shon, J. H., Liu, K. H., et al. (2005). High-throughput screening of inhibitory potential of nine cytochrome P450 enzymes in vitro using liquid chromatography/tandem mass spectrometry. Rapid Commun. Mass Spectrom. 19 (18), 2651–2658. doi:10.1002/rcm.2110
Kmiecik, G., Spoldi, V., Silini, A., and Parolini, O. (2015). Current view on osteogenic differentiation potential of mesenchymal stromal cells derived from placental tissues. Stem Cell Rev. Rep. 11 (4), 570–585. doi:10.1007/s12015-014-9569-1
Lanzoni, G., Linetsky, E., Correa, D., Messinger Cayetano, S., Alvarez, R. A., Kouroupis, D., et al. (2021). Umbilical cord mesenchymal stem cells for COVID-19 acute respiratory distress syndrome: A double-blind, phase 1/2a, randomized controlled trial. Stem Cells Transl. Med. 10 (5), 660–673. doi:10.1002/sctm.20-0472
Laschke, M. W., and Menger, M. D. (2017). Life is 3D: Boosting spheroid function for tissue engineering. Trends Biotechnol. 35 (2), 133–144. doi:10.1016/j.tibtech.2016.08.004
Laurent, R., Nallet, A., de Billy, B., Obert, L., Nicod, L., Meyer, C., et al. (2017). Fresh and in vitro osteodifferentiated human amniotic membrane, alone or associated with an additional scaffold, does not induce ectopic bone formation in Balb/c mice. Cell Tissue Bank. 18 (1), 17–25. doi:10.1007/s10561-016-9605-2
Lazzarini, E., Balbi, C., Altieri, P., Pfeffer, U., Gambini, E., Canepa, M., et al. (2016). The human amniotic fluid stem cell secretome effectively counteracts doxorubicin-induced cardiotoxicity. Sci. Rep. 21, 29994. doi:10.1038/srep29994
Lebreton, F., Lavallard, V., Bellofatto, K., Bonnet, R., Wassmer, C. H., Perez, L., et al. (2019). Insulin-producing organoids engineered from islet and amniotic epithelial cells to treat diabetes. Nat. Commun. 10 (1), 4491. doi:10.1038/s41467-019-12472-3
Lee, B. C., Shin, N., Lee, J. Y., Kang, I., Kim, J. J., Lee, S. E., et al. (2018). MIS416 enhances therapeutic functions of human umbilical cord blood-derived mesenchymal stem cells against experimental colitis by modulating systemic immune milieu. Front. Immunol. 9, 1078. doi:10.3389/fimmu.2018.01078
Lee, M. J., Jung, J., Na, K. H., Moon, J. S., Lee, H. J., Kim, J. H., et al. (2010). Anti-fibrotic effect of chorionic plate-derived mesenchymal stem cells isolated from human placenta in a rat model of CCl4-injured liver: Potential application to the treatment of hepatic diseases. J. Cell. Biochem. 111 (6), 1453–1463. doi:10.1002/jcb.22873
Legaki, E., Roubelakis, M. G., Theodoropoulos, G. E., Lazaris, A., Kollia, A., Karamanolis, G., et al. (2016). Therapeutic potential of secreted molecules derived from human amniotic fluid mesenchymal stem/stroma cells in a mice model of colitis. Stem Cell Rev. Rep. 12 (5), 604–612. doi:10.1007/s12015-016-9677-1
Lemke, A., Ferguson, J., Gross, K., Penzenstadler, C., Bradl, M., Mayer, R. L., et al. (2018). Transplantation of human amnion prevents recurring adhesions and ameliorates fibrosis in a rat model of sciatic nerve scarring. Acta Biomater. 66, 335–349. doi:10.1016/j.actbio.2017.11.042
Li, W., He, H., Chen, Y. T., Hayashida, Y., and Tseng, S. C. G. (2008). Reversal of myofibroblasts by amniotic membrane stromal extract. J. Cell. Physiol. 215 (3), 657–664. doi:10.1002/jcp.21345
Li, Z., Qin, H., Feng, Z., Liu, W., Zhou, Y., Yang, L., et al. (2013). Human umbilical cord mesenchymal stem cell-loaded amniotic membrane for the repair of radial nerve injury. Neural Regen. Res. 8 (36), 3441–3448. doi:10.3969/j.issn.1673-5374.2013.36.010
Liang, L., Dong, C., Chen, X., Fang, Z., Xu, J., Liu, M., et al. (2011). Human umbilical cord mesenchymal stem cells ameliorate mice trinitrobenzene sulfonic acid (TNBS)-induced colitis. Cell Transpl. 20 (9), 1395–1408. doi:10.3727/096368910x557245
Liddelow, S. A., Guttenplan, K. A., Clarke, L. E., Bennett, F. C., Bohlen, C. J., Schirmer, L., et al. (2017). Neurotoxic reactive astrocytes are induced by activated microglia. Nature 541 (7638), 481–487. doi:10.1038/nature21029
Lindenmair, A., Wolbank, S., Stadler, G., Meinl, A., Peterbauer-Scherb, A., Eibl, J., et al. (2010). Osteogenic differentiation of intact human amniotic membrane. Biomaterials 31 (33), 8659–8665. doi:10.1016/j.biomaterials.2010.07.090
Liu, G., Li, Y., Sun, J., Zhou, H., Zhang, W., Cui, L., et al. (2010). In vitro and in vivo evaluation of osteogenesis of human umbilical cord blood-derived mesenchymal stem cells on partially demineralized bone matrix. Tissue Eng. Part A 16 (3), 971–982. doi:10.1089/ten.tea.2009.0516
Liu, W. H., Song, F. Q., Ren, L. N., Guo, W. Q., Wang, T., Feng, Y. X., et al. (2015). The multiple functional roles of mesenchymal stem cells in participating in treating liver diseases. J. Cell. Mol. Med. 19 (3), 511–520. doi:10.1111/jcmm.12482
Lively, S., and Schlichter, L. C. (2018). Microglia responses to pro-inflammatory stimuli (LPS, IFNγ+TNFα) and reprogramming by resolving cytokines (IL-4, IL-10). Front. Cell. Neurosci. 12, 215. doi:10.3389/fncel.2018.00215
Miki, T., Lehmann, T., Cai, H., Stolz, D. B., and Strom, S. C. (2005). Stem cell characteristics of amniotic epithelial cells. Stem Cells 23 (10), 1549–1559. doi:10.1634/stemcells.2004-0357
Ma, Y., Dong, L., Zhou, D., Li, L., Zhang, W., Zhen, Y., et al. (2019). Extracellular vesicles from human umbilical cord mesenchymal stem cells improve nerve regeneration after sciatic nerve transection in rats. J. Cell. Mol. Med. 23 (4), 2822–2835. doi:10.1111/jcmm.14190
Madonna, R., Van Laake, L. W., Davidson, S. M., Engel, F. B., Hausenloy, D. J., Lecour, S., et al. (2016). Position paper of the European society of cardiology working group cellular biology of the heart: Cell-based therapies for myocardial repair and regeneration in ischemic heart disease and heart failure. Eur. Heart J. 37 (23), 1789–1798. doi:10.1093/eurheartj/ehw113
Magarotto, F., Sgrò, A., Dorigo Hochuli, A. H., Andreetta, M., Grassi, M., Saggioro, M., et al. (2021). Muscle functional recovery is driven by extracellular vesicles combined with muscle extracellular matrix in a volumetric muscle loss murine model. Biomaterials 269, 120653. doi:10.1016/j.biomaterials.2021.120653
Maghin, E., Garbati, P., Quarto, R., Piccoli, M., and Bollini, S. (2020). Young at heart: Combining strategies to rejuvenate endogenous mechanisms of cardiac repair. Front. Bioeng. Biotechnol. 13, 447. doi:10.3389/fbioe.2020.00447
Maji, S., Matsuda, A., Yan, I. K., Parasramka, M., and Patel, T. (2017). Extracellular vesicles in liver diseases. Am. J. Physiology-Gastrointestinal Liver Physiology 312 (3), G194–G200. doi:10.1152/ajpgi.00216.2016
Mao, F., Wu, Y., Tang, X., Kang, J., Zhang, B., Yan, Y., et al. (2017). Exosomes derived from human umbilical cord mesenchymal stem cells relieve inflammatory bowel disease in mice. Biomed. Res. Int. 2017, 1–12. doi:10.1155/2017/5356760
Mao, Y., Hoffman, T., Singh-Varma, A., Duan-Arnold, Y., Moorman, M., Danilkovitch, A., et al. (2017). Antimicrobial peptides secreted from human cryopreserved viable amniotic membrane contribute to its antibacterial activity. Sci. Rep. 7 (1), 13722. doi:10.1038/s41598-017-13310-6
Marchesini, A., Raimondo, S., Zingaretti, N., Riccio, V., Battiston, B., Provinciali, M., et al. (2018). The amnion muscle combined graft (AMCG) conduits in nerves repair: An anatomical and experimental study on a rat model. J. Mater. Sci. Mater. Med. 29 (8), 120. doi:10.1007/s10856-018-6126-5
Marongiu, F., Gramignoli, R., Dorko, K., Miki, T., Ranade, A. R., Paola Serra, M., et al. (2011). Hepatic differentiation of amniotic epithelial cells. Hepatology 53 (5), 1719–1729. doi:10.1002/hep.24255
Marongiu, M., Serra, M. P., Contini, A., Sini, M., Strom, S. C., Laconi, E., et al. (2015). Rat-derived amniotic epithelial cells differentiate into mature hepatocytes in vivo with no evidence of cell fusion. Stem Cells Dev. 24 (12), 1429–1435. doi:10.1089/scd.2014.0532
Masyuk, A. I., Masyuk, T. V., and Larusso, N. F. (2013). Exosomes in the pathogenesis, diagnostics and therapeutics of liver diseases. J. Hepatology 59 (3), 621–625. doi:10.1016/j.jhep.2013.03.028
Matthay, M. A., Calfee, C. S., Zhuo, H., Thompson, B. T., Wilson, J. G., Levitt, J. E., et al. (2019). Treatment with allogeneic mesenchymal stromal cells for moderate to severe acute respiratory distress syndrome (START study): A randomised phase 2a safety trial. Lancet Respir. Med. 7 (2), 154–162. doi:10.1016/s2213-2600(18)30418-1
Maymó, J. L., Riedel, R., Pérez-Pérez, A., Magatti, M., Maskin, B., Dueñas, J. L., et al. (2018). Proliferation and survival of human amniotic epithelial cells during their hepatic differentiation. PLoS One 13 (1), e0191489. doi:10.1371/journal.pone.0191489
Mechiche Alami, S., Gangloff, S. C., Laurent-Maquin, D., Wang, Y., and Kerdjoudj, H. (2016). Concise review: In vitro formation of bone-like nodules sheds light on the application of stem cells for bone regeneration. Stem Cells Transl. Med. 5 (11), 1587–1593. doi:10.5966/sctm.2015-0413
Menasché, P. (2018). Cell therapy trials for heart regeneration — Lessons learned and future directions. Nat. Rev. Cardiol. 15 (11), 659–671. doi:10.1038/s41569-018-0013-0
Meng, F., Xu, R., Wang, S., Xu, Z., Zhang, C., Li, Y., et al. (2020). Human umbilical cord-derived mesenchymal stem cell therapy in patients with COVID-19: A phase 1 clinical trial. Signal Transduct. Target. Ther. 5 (1), 172. doi:10.1038/s41392-020-00286-5
Milosavljevic, N., Gazdic, M., Simovic Markovic, B., Arsenijevic, A., Nurkovic, J., Dolicanin, Z., et al. (2017). Mesenchymal stem cells attenuate acute liver injury by altering ratio between interleukin 17 producing and regulatory natural killer T cells. Liver Transpl. 23 (8), 1040–1050. doi:10.1002/lt.24784
Mirzayan, R., Syed, S. P., and Shean, C. J. (2021). Dissection of an ulnar nerve previously transposed and wrapped with human amniotic membrane: A report of 3 cases. JBJS Case Connect. 11 (3). doi:10.2106/jbjs.cc.21.00066
Mishra, S., Sevak, J. K., Das, A., Arimbasseri, G. A., Bhatnagar, S., Gopinath, S. D., et al. (2020). Umbilical cord tissue is a robust source for mesenchymal stem cells with enhanced myogenic differentiation potential compared to cord blood. Sci. Rep. 10 (1), 18978. doi:10.1038/s41598-020-75102-9
Moodley, Y., Ilancheran, S., Samuel, C., Vaghjiani, V., Atienza, D., Williams, E. D., et al. (2010). Human amnion epithelial cell transplantation abrogates lung fibrosis and augments repair. Am. J. Respir. Crit. Care Med. 182 (5), 643–651. doi:10.1164/rccm.201001-0014oc
Moodley, Y., Vaghjiani, V., Chan, J., Baltic, S., Ryan, M., Tchongue, J., et al. (2013). Anti-inflammatory effects of adult stem cells in sustained lung injury: A comparative study. PLoS One 8 (8), e69299. doi:10.1371/journal.pone.0069299
Moris, V., Loisel, F., Cheval, D., See, L. A., Tchurukdichian, A., Pluvy, I., et al. (2016). Functional and radiographic evaluation of the treatment of traumatic bone loss of the hand using the Masquelet technique. Hand Surg. Rehabil. 35 (2), 114–121. doi:10.1016/j.hansur.2015.11.002
Moroncini, G., Paolini, C., Orlando, F., Capelli, C., Grieco, A., Tonnini, C., et al. (2018). Mesenchymal stromal cells from human umbilical cord prevent the development of lung fibrosis in immunocompetent mice. PLoS One 13 (6), e0196048. doi:10.1371/journal.pone.0196048
Murphy, S., Lim, R., Dickinson, H., Acharya, R., Rosli, S., Jenkin, G., et al. (2011). Human amnion epithelial cells prevent bleomycin-induced lung injury and preserve lung function. Cell Transpl. 20 (6), 909–924. doi:10.3727/096368910x543385
Murphy, S. V., Shiyun, S. C., Tan, J. L., Chan, S., Jenkin, G., Wallace, E. M., et al. (2012). Human amnion epithelial cells do not abrogate pulmonary fibrosis in mice with impaired macrophage function. Cell Transpl. 21 (7), 1477–1492. doi:10.3727/096368911x601028
Nagaishi, K., Ataka, K., Echizen, E., Arimura, Y., and Fujimiya, M. (2014). Mesenchymal stem cell therapy ameliorates diabetic hepatocyte damage in mice by inhibiting infiltration of bone marrow-derived cells. Hepatology 59 (5), 1816–1829. doi:10.1002/hep.26975
Nie, Y. Z., Zheng, Y. W., Miyakawa, K., Murata, S., Zhang, R. R., Sekine, K., et al. (2018). Recapitulation of Hepatitis B virus–host interactions in liver organoids from human induced pluripotent stem cells. EBioMedicine 35, 114–123. doi:10.1016/j.ebiom.2018.08.014
Oppliger, B., Joerger-Messerli, M. S., Simillion, C., Mueller, M., Surbek, D. V., Schoeberlein, A., et al. (2017). Mesenchymal stromal cells from umbilical cord Wharton’s jelly trigger oligodendroglial differentiation in neural progenitor cells through cell-to-cell contact. Cytotherapy 19 (7), 829–838. doi:10.1016/j.jcyt.2017.03.075
Panhua, X., qing, Li Q., Zhuqing, X., Li, Z., Cai, X., Qing, P R., et al. (2019). Mechanism and therapeutic effect of umbilical cord mesenchymal stem cells in inflammatory bowel disease. Sci. Rep. 9 (1), 17646. doi:10.1038/s41598-019-54194-y
Park, S. E., Jeong, J. Bin, Oh, S. J., Kim, S. J., Kim, H., Choi, A., et al. (2021). Wharton’s jelly-derived mesenchymal stem cells reduce fibrosis in a mouse model of duchenne muscular dystrophy by upregulating microRNA 499. Biomedicines 9 (9), 1089. doi:10.3390/biomedicines9091089
Passaretta, F., Bosco, D., Centurione, L., Centurione, M. A., Marongiu, F., Di Pietro, R., et al. (2020). Differential response to hepatic differentiation stimuli of amniotic epithelial cells isolated from four regions of the amniotic membrane. J. Cell. Mol. Med. 24 (7), 4350–4355. doi:10.1111/jcmm.14928
Peng, S. Y., Chou, C. J., Cheng, P. J., Ko, I. C., Kao, Y. J., Chen, Y. H., et al. (2014). Therapeutic potential of amniotic-fluid-derived stem cells on liver fibrosis model in mice. Taiwan. J. Obstet. Gynecol. 53 (2), 151–157. doi:10.1016/j.tjog.2014.04.005
Penolazzi, L., Lambertini, E., D’Agostino, S., Pozzobon, M., Notarangelo, M. P., Greco, P., et al. (2022). Decellularized extracellular matrix-based scaffold and hypoxic priming: A promising combination to improve the phenotype of degenerate intervertebral disc cells. Life Sci. 301, 120623. doi:10.1016/j.lfs.2022.120623
Penolazzi, L., Pozzobon, M., Bergamin, L. S., D’Agostino, S., Francescato, R., Bonaccorsi, G., et al. (2020). Extracellular matrix from decellularized wharton’s jelly improves the behavior of cells from degenerated intervertebral disc. Front. Bioeng. Biotechnol. 27, 262. doi:10.3389/fbioe.2020.00262
Petrenko, Y., Vackova, I., Kekulova, K., Chudickova, M., Koci, Z., Turnovcova, K., et al. (2020). A comparative analysis of multipotent mesenchymal stromal cells derived from different sources, with a focus on neuroregenerative potential. Sci. Rep. 10 (1), 4290. doi:10.1038/s41598-020-61167-z
Pettinato, G., Lehoux, S., Ramanathan, R., Salem, M. M., He, L. X., Muse, O., et al. (2019). Generation of fully functional hepatocyte-like organoids from human induced pluripotent stem cells mixed with Endothelial Cells. Sci. Rep. 9 (1), 8920. doi:10.1038/s41598-019-45514-3
Prior, N., Inacio, P., and Huch, M. (2019). Liver organoids: From basic research to therapeutic applications. Gut 68 (12), 2228–2237. doi:10.1136/gutjnl-2019-319256
Psaraki, A., Ntari, L., Karakostas, C., Korrou-Karava, D., and Roubelakis, M. G. (2021). Extracellular vesicles derived from mesenchymal stem/stromal cells: The regenerative impact in liver diseases. Hepatology 75, 1590–1603. doi:10.1002/hep.32129
Qiu, X., Liu, S., Zhang, H., Zhu, B., Su, Y., Zheng, C., et al. (2018). Mesenchymal stem cells and extracellular matrix scaffold promote muscle regeneration by synergistically regulating macrophage polarization toward the M2 phenotype. Stem Cell Res. Ther. 9 (1), 88. doi:10.1186/s13287-018-0821-5
Qiu, Y., Guo, J., Mao, R., Chao, K., Chen, B., He, Y., et al. (2017). TLR3 preconditioning enhances the therapeutic efficacy of umbilical cord mesenchymal stem cells in TNBS-induced colitis via the TLR3-Jagged-1-Notch-1 pathway. Mucosal Immunol. 10 (3), 727–742. doi:10.1038/mi.2016.78
Ramachandran, S. D., Schirmer, K., Münst, B., Heinz, S., Ghafoory, S., Wölfl, S., et al. (2015). In vitro generation of functional liver organoid-like structures using adult human cells. PLoS One 10 (10), e0139345. doi:10.1371/journal.pone.0139345
Rammal, H., Dubus, M., Aubert, L., Reffuveille, F., Laurent-Maquin, D., Terryn, C., et al. (2017). Bioinspired nanofeatured substrates: Suitable environment for bone regeneration. ACS Appl. Mater. Interfaces 9 (14), 12791–12801. doi:10.1021/acsami.7b01665
Rammal, H., Entz, L., Dubus, M., Moniot, A., Bercu, N. B., Sergheraert, J., et al. (2019). Osteoinductive material to fine-tune paracrine crosstalk of mesenchymal stem cells with endothelial cells and osteoblasts. Front. Bioeng. Biotechnol. 9, 256. doi:10.3389/fbioe.2019.00256
Rani, S., Ryan, A. E., Griffin, M. D., and Ritter, T. (2015). Mesenchymal stem cell-derived extracellular vesicles: Toward cell-free therapeutic applications. Mol. Ther. 23 (5), 812–823. doi:10.1038/mt.2015.44
Reis, M., Ogonek, J., Qesari, M., Borges, N. M., Nicholson, L., Preußner, L., et al. (2016). Recent developments in cellular immunotherapy for HSCT-associated complications. Front. Immunol. 7, 500. doi:10.3389/fimmu.2016.00500
Riccio, M., Maraldi, T., Pisciotta, A., La Sala, G. B., Ferrari, A., Bruzzesi, G., et al. (2012). Fibroin scaffold repairs critical-size bone defects in vivo supported by human amniotic fluid and dental pulp stem cells. Tissue Eng. Part A 18 (9–10), 1006–1013. doi:10.1089/ten.tea.2011.0542
Riccio, M., Pangrazi, P. P., Parodi, P. C., Vaienti, L., Marchesini, A., Neuendorf, A. D., et al. (2014). The amnion muscle combined graft (amcg) conduits: A new alternative in the repair of wide substance loss of peripheral nerves. Microsurgery 34 (8), 616–622. doi:10.1002/micr.22306
Roboon, J., Hattori, T., Ishii, H., Takarada-Iemata, M., Nguyen, D. T., Heer, C. D., et al. (2021). Inhibition of CD38 and supplementation of nicotinamide riboside ameliorate lipopolysaccharide-induced microglial and astrocytic neuroinflammation by increasing NAD+. J. Neurochem. 158 (2), 311–327. doi:10.1111/jnc.15367
Roger, V. L. (2013). Epidemiology of heart failure. Circ. Res. 113 (6), 646–659. doi:10.1161/circresaha.113.300268
Rossi, G., Manfrin, A., and Lutolf, M. P. (2018). Progress and potential in organoid research. Nat. Rev. Genet. 19 (11), 671–687. doi:10.1038/s41576-018-0051-9
Santos Nascimento, D., Mosqueira, D., Sousa, L. M., Teixeira, M., Filipe, M., Resende, T. P., et al. (2014). Human umbilical cord tissue-derived mesenchymal stromal cells attenuate remodeling after myocardial infarction by proangiogenic, antiapoptotic, and endogenous cell-activation mechanisms. Stem Cell Res. Ther. 5 (1), 5. doi:10.1186/scrt394
Scelfo, C., Caminati, A., and Harari, S. (2017). Recent advances in managing idiopathic pulmonary fibrosis. F1000Res. 276, 2052. doi:10.12688/f1000research.10720.1
Segers, V. F. M., and Lee, R. T. (2008). Stem-cell therapy for cardiac disease. Nature 451 (7181), 937–942. doi:10.1038/nature06800
Shahrbaf, M. A., Nouri, M., Zarrabi, M., Gramignoli, R., and Vosough, M. (2022). Extraembryonic mesenchymal stromal/stem cells in liver diseases: A critical revision of promising advanced therapy medicinal products. Cells 11 (7), 1074. doi:10.3390/cells11071074
Shi, M., Liu, Z., Wang, Y., Xu, R., Sun, Y., Zhang, M., et al. (2017). A pilot study of mesenchymal stem cell therapy for acute liver allograft rejection. Stem Cells Transl. Med. 6 (12), 2053–2061. doi:10.1002/sctm.17-0134
Shu, L., Niu, C., Li, R., Huang, T., Wang, Y., Huang, M., et al. (2020). Treatment of severe COVID-19 with human umbilical cord mesenchymal stem cells. Stem Cell Res. Ther. 11 (1), 361. doi:10.1186/s13287-020-01875-5
Silini, A. R., Di Pietro, R., Lang-Olip, I., Alviano, F., Banerjee, A., Basile, M., et al. (2020). Perinatal derivatives: Where do we stand? A roadmap of the human placenta and consensus for tissue and cell nomenclature. Front. Bioeng. Biotechnol. 8, 1438. doi:10.3389/fbioe.2020.610544
Skvorak, K. J., Dorko, K., Marongiu, F., Tahan, V., Hansel, M. C., Gramignoli, R., et al. (2013). Improved amino acid, bioenergetic metabolite and neurotransmitter profiles following human amnion epithelial cell transplant in intermediate maple syrup urine disease mice. Mol. Genet. Metab. 109 (2), 132–138. doi:10.1016/j.ymgme.2013.02.011
Skvorak, K. J., Dorko, K., Marongiu, F., Tahan, V., Hansel, M. C., Gramignoli, R., et al. (2013). Placental stem cell correction of murine intermediate maple syrup urine disease. Hepatology 57 (3), 1017–1023. doi:10.1002/hep.26150
Song, J. Y., Kang, H. J., Hong, J. S., Kim, C. J., Shim, J. Y., Lee, C. W., et al. (2017). Umbilical cord-derived mesenchymal stem cell extracts reduce colitis in mice by re-polarizing intestinal macrophages. Sci. Rep. 7 (1), 9412. doi:10.1038/s41598-017-09827-5
Spinelli, M., Boucard, C., Nicuolo, F. D, Haesler, V., Castellani, R., Pontecorvi, A., et al. (2020). Synthetic PreImplantation Factor (sPIF) reduces inflammation and prevents preterm birth. PLoS One 15 (6), e0232493. doi:10.1371/journal.pone.0232493
Srinivasan, B., Kolli, A. R., Esch, M. B., Abaci, H. E., Shuler, M. L., Hickman, J. J., et al. (2015). TEER measurement techniques for in vitro barrier model systems. SLAS Technol. 20 (2), 107–126. doi:10.1177/2211068214561025
Stansley, B., Post, J., and Hensley, K. (2012). A comparative review of cell culture systems for the study of microglial biology in Alzheimer’s disease. J. Neuroinflammation 9 (1), 115. doi:10.1186/1742-2094-9-115
Strom, S. C., Skvorak, K., Gramignoli, R., Marongiu, F., and Miki, T. (2013). Translation of amnion stem cells to the clinic. Stem Cells Dev. 22 (1), 96–102. doi:10.1089/scd.2013.0391
Su, C. F., Chang, L. H., Kao, C. Y., Lee, D. C., Cho, K. H., Kuo, L. W., et al. (2018). Application of amniotic fluid stem cells in repairing sciatic nerve injury in minipigs. Brain Res. 1678, 397–406. doi:10.1016/j.brainres.2017.11.010
Su, W. H., Wang, C. J., Fu, H. C., Sheng, C. M., Tsai, C. C., Cheng, J. H., et al. (2019). Human umbilical cord mesenchymal stem cells extricate bupivacaine-impaired skeletal muscle function via mitigating neutrophil-mediated acute inflammation and protecting against fibrosis. Int. J. Mol. Sci. 20 (17), 4312. doi:10.3390/ijms20174312
Sugiura, R., Ohnishi, S., Ohara, M., Ishikawa, M., Miyamoto, S., Onishi, R., et al. (2018). Effects of human amnion-derived mesenchymal stem cells and conditioned medium in rats with sclerosing cholangitis. Am. J. Transl. Res. 10 (7), 2102–2114.
Sun, E., and Karaoz, E. (2020). Can wharton jelly derived or adipose tissue derived mesenchymal stem cell can be a treatment option for duchenne muscular dystrophy? Answers as transcriptomic aspect. Am. J. Stem Cells 9 (4), 57–67.
Surolia, R., Li, F. J., Wang, Z., Li, H., Liu, G., Zhou, Y., et al. (2017). 3D pulmospheres serve as a personalized and predictive multicellular model for assessment of antifibrotic drugs. JCI Insight 2 (2), 94088. doi:10.1172/jci.insight.94088
Takashima, S., Ise, H., Zhao, P., Akaike, T., and Nikaido, T. (2004). Human amniotic epithelial cells possess hepatocyte-like characteristics and functions. Cell Struct. Funct. 29 (3), 73–84. doi:10.1247/csf.29.73
Takebe, T., Sekine, K., Enomura, M., Koike, H., Kimura, M., Ogaeri, T., et al. (2013). Vascularized and functional human liver from an iPSC-derived organ bud transplant. Nature 499 (7459), 481–484. doi:10.1038/nature12271
Takov, K., He, Z., Johnston, H. E., Timms, J. F., Guillot, P. V., Yellon, D. M., et al. (2020). Small extracellular vesicles secreted from human amniotic fluid mesenchymal stromal cells possess cardioprotective and promigratory potential. Basic Res. Cardiol. 115 (3), 26. doi:10.1007/s00395-020-0785-3
Tan, J. L., Chan, S. T., Lo, C. Y., Deane, J. A., McDonald, C. A., Bernard, C. C. A., et al. (2015). Amnion cell-mediated immune modulation following bleomycin challenge: Controlling the regulatory T cell response. Stem Cell Res. Ther. 6 (1), 8. doi:10.1186/scrt542
Tan, J. L., Chan, S. T., Wallace, E. M., and Lim, R. (2014). Human amnion epithelial cells mediate lung repair by directly modulating macrophage recruitment and polarization. Cell Transpl. 23 (3), 319–328. doi:10.3727/096368912x661409
Tan, J. L., Lau, S. N., Leaw, B., Nguyen, H. P. T., Salamonsen, L. A., Saad, M. I., et al. (2018). Amnion epithelial cell-derived exosomes restrict lung injury and enhance endogenous lung repair. Stem Cells Transl. Med. 7 (2), 180–196. doi:10.1002/sctm.17-0185
Thomi, G., Surbek, D., Haesler, V., Joerger-Messerli, M., and Schoeberlein, A. (2019). Exosomes derived from umbilical cord mesenchymal stem cells reduce microglia-mediated neuroinflammation in perinatal brain injury. Stem Cell Res. Ther. 10 (1), 105. doi:10.1186/s13287-019-1207-z
Todd, N. W., Scheraga, R. G., Galvin, J. R., Iacono, A. T., Britt, E. J., Luzina, I. G., et al. (2013). Lymphocyte aggregates persist and accumulate in the lungs of patients with idiopathic pulmonary fibrosis. J. Inflamm. Res. 6 (1), 63–70. doi:10.2147/jir.s40673
Tolomeo, A. M., Castagliuolo, I., Piccoli, M., Grassi, M., Magarotto, F., De Lazzari, G., et al. (2021). Extracellular vesicles secreted by mesenchymal stromal cells exert opposite effects to their cells of origin in murine sodium dextran sulfate-induced colitis. Front. Immunol. 13, 627605. doi:10.3389/fimmu.2021.627605
Trohatou, O., and Roubelakis, M. G. (2017). Mesenchymal stem/stromal cells in regenerative medicine: Past, present, and future. Cell. Reprogr. 19 (4), 217–224. doi:10.1089/cell.2016.0062
Ülger, M., Sezer, G., Özyazgan, İ., Özocak, H., Yay, A., Balcıoğlu, E., et al. (2021). The effect of erythropoietin and umbilical cord-derived mesenchymal stem cells on nerve regeneration in rats with sciatic nerve injury. J. Chem. Neuroanat. 114, 101958. doi:10.1016/j.jchemneu.2021.101958
Vieujean, S., Loly, J. P., Boutaffala, L., Meunier, P., Reenaers, C., Briquet, A., et al. (2022). Mesenchymal stem cell injection in Crohn’s disease strictures: A phase I-ii clinical study. J. Crohn's. Colitis 16 (3), 506–510. doi:10.1093/ecco-jcc/jjab154
Villa, F., Quarto, R., and Tasso, R. (2019). Extracellular vesicles as natural, safe and efficient drug delivery systems. Pharmaceutics 11 (11), 557. doi:10.3390/pharmaceutics11110557
Viswanathan, S., Shi, Y., Galipeau, J., Krampera, M., Leblanc, K., Martin, I., et al. (2019). Mesenchymal stem versus stromal cells: International society for cell & gene therapy (ISCT®) mesenchymal stromal cell committee position statement on nomenclature. Cytotherapy 21 (10), 1019–1024. doi:10.1016/j.jcyt.2019.08.002
Volkman, R., and Offen, D. (2017). Concise review: Mesenchymal stem cells in neurodegenerative diseases. Stem Cells 35 (8), 1867–1880. doi:10.1002/stem.2651
Vosdoganes, P., Wallace, E. M., Chan, S. T., Acharya, R., Moss, T. J. M., Lim, R., et al. (2013). Human amnion epithelial cells repair established lung injury. Cell Transpl. 22 (8), 1337–1349. doi:10.3727/096368912x657657
Wang, G., Joel, M. D. M., Yuan, J., Wang, J., Cai, X., Ocansey, D. K. W., et al. (2020). Human umbilical cord mesenchymal stem cells alleviate inflammatory bowel disease by inhibiting ERK phosphorylation in neutrophils. Inflammopharmacology 28 (2), 603–616. doi:10.1007/s10787-019-00683-5
Wang, Q. Q., Jing, X. M., Bi, Y. Z., Cao, X. F., Wang, Y. Z., Li, Y. X., et al. (2018). Human umbilical cord wharton’s jelly derived mesenchymal stromal cells may attenuate sarcopenia in aged mice induced by hindlimb suspension. Med. Sci. Monit. 24, 9272–9281. doi:10.12659/msm.913362
Wang, Y., Chen, X., Cao, W., and Shi, Y. (2014). Plasticity of mesenchymal stem cells in immunomodulation: Pathological and therapeutic implications. Nat. Immunol. 15 (11), 1009–1016. doi:10.1038/ni.3002
Wen, S., Dooner, M., Cheng, Y., Papa, E., Del Tatto, M., Pereira, M., et al. (2016). Mesenchymal stromal cell-derived extracellular vesicles rescue radiation damage to murine marrow hematopoietic cells. Leukemia 30 (11), 2221–2231. doi:10.1038/leu.2016.107
Wen, S. J., Zheng, X. M., Liu, L. F., Li, N. N., Mao, H. A., Huang, L., et al. (2021). Effects of primary microglia and astrocytes on neural stem cells in in vitro and in vivo models of ischemic stroke. Neural Regen. Res. 16 (9), 1677–1685. doi:10.4103/1673-5374.306093
Wilkinson, D. C., Alva-Ornelas, J. A., Sucre, J. M. S., Vijayaraj, P., Durra, A., Richardson, W., et al. (2017). Development of a three-dimensional bioengineering technology to generate lung tissue for personalized disease modeling. Stem Cells Transl. Med. 6 (2), 622–633. doi:10.5966/sctm.2016-0192
Willenbring, H., Bailey, A. S., Foster, M., Akkari, Y., Dorrell, C., Olson, S., et al. (2004). Myelomonocytic cells are sufficient for therapeutic cell fusion in liver. Nat. Med. 10 (7), 744–748. doi:10.1038/nm1062
Wolfe, E. M., Mathis, S. A., Ovadia, S. A., and Panthaki, Z. J. (2022). Comparison of collagen and human amniotic membrane nerve wraps and conduits for peripheral nerve repair in preclinical models: A systematic review of the literature. J. Reconstr. Microsurg. 2022. doi:10.1055/s-0041-1732432
Wu, F., Wu, D., Ren, Y., Huang, Y., Feng, B., Zhao, N., et al. (2019). Generation of hepatobiliary organoids from human induced pluripotent stem cells. J. Hepatology 70 (6), 1145–1158. doi:10.1016/j.jhep.2018.12.028
Wu, X. M., Qian, C., Jiang, F., Bao, Y. X., Qian, Z. M., Ke, Y., et al. (2021). The involvement of nuclear factor-κB in astroprotection against ischemia-reperfusion injury by ischemia-preconditioned neurons. J. Cell. Physiol. 236 (6), 4515–4527. doi:10.1002/jcp.30168
Xiong, Y., Mahmood, A., and Chopp, M. (2017). Emerging potential of exosomes for treatment of traumatic brain injury. Neural Regen. Res. 12 (1), 19. doi:10.4103/1673-5374.198966
Yang, S., Liang, X., Song, J., Li, C., Liu, A., Luo, Y., et al. (2021). A novel therapeutic approach for inflammatory bowel disease by exosomes derived from human umbilical cord mesenchymal stem cells to repair intestinal barrier via TSG-6. Stem Cell Res. Ther. 12 (1), 315. doi:10.1186/s13287-021-02404-8
Zabulica, M., Srinivasan, R. C., Vosough, M., Hammarstedt, C., Wu, T., Gramignoli, R., et al. (2019). Guide to the assessment of mature liver gene expression in stem cell-derived hepatocytes. Stem Cells Dev. 28 (14), 907–919. doi:10.1089/scd.2019.0064
Zagoura, D. S., Roubelakis, M. G., Bitsika, V., Trohatou, O., Pappa, K. I., Kapelouzou, A., et al. (2012). Therapeutic potential of a distinct population of human amniotic fluid mesenchymal stem cells and their secreted molecules in mice with acute hepatic failure. Gut 61 (6), 894–906. doi:10.1136/gutjnl-2011-300908
Zhang, C., Yin, X., Zhang, J., Ao, Q., Gu, Y., Liu, Y., et al. (2017). Clinical observation of umbilical cord mesenchymal stem cell treatment of severe idiopathic pulmonary fibrosis: A case report. Exp. Ther. Med. 13 (5), 1922–1926. doi:10.3892/etm.2017.4222
Zhang, L, Zou, D, Li, S, Wang, J, Qu, Y, Ou, S, et al. (2016). An Ultra-thin Amniotic Membrane as Carrier in corneal epithelium Tissue-Engineering. Sci. Rep. 6 (1), 21021. doi:10.1038/srep21021
Zhang, X, Zhivaki, D, and Lo-Man, R (2017). Unique aspects of the perinatal immune system. Nat. Rev. Immunol. 17 (8), 495–507. doi:10.1038/nri.2017.54
Glossary
α-SMA α- smooth muscle actin
AMP amniotic membrane protein
AMSC amniotic membrane mesenchymal stromal cells
AnnV/PI annexin V and propidium iodide
AP alkaline phosphatase
BBB blood-brain barrier
bFGF basic fibroblast growth factor
CD Crohn’s disease
CM conditioned medium
CMAP compound muscle action potential
CNS central nervous system
CPC calcium phosphate cements
CT computed tomography
DAMP danger-associated molecular patterns
dP decellularized perinatal derivative
dP-ECM dP extracellular matrix
DSS dextran sulfate sodium;
ECFC endothelial colony-forming cells
EDX energy dispersive X-ray
EGF epidermal growth factor
EMG electromyologram
EPT extensor postural thrust
ES embryonic stem cells
EV extracellular vesicles
GFAP glial fibrillary acidic protein
GT grasping test
hACM human amnio-chorionic membrane
hAEC amniotic membrane epithelial cells
hAEC-Exo exosomes from hAEC
hAFSC amniotic fluid stem cells
hAF-MSC amniotic fluid mesenchymal stromal cells
hAM human AM
hAMSC-CM human amniotic membrane mesenchymal stromal cells conditioned medium
hBMSC human bone marrow mesenchymal stromal cells
HE hematoxylin and eosin;
HUVEC human umbilical vein endothelial cells
IBD inflammatory bowel diseases
IFNγ interferon-gamma
IGF insulin-like growth factor
IL interleukin
iPSC induced pluripotent stem cells
IPF Idiopathic pulmonary fibrosis
LPS lipopolysaccharide
MgHA magnesium-enriched hydroxyapatite
MMP metalloproteinase
MSC mesenchymal stromal cells
MSCEVs MSC- derived extracellular vesicles
MSCMVs MSC-derived microvesicles
MSUD maple syrup urine disease
MVs microvesicles
NCV nerve conduction velocity
NLRP3 NLR family pyrin domain containing 3
NPC progenitor cells
NSC neural stem cells
OGD oxygen-glucose deprivation
OPC oligodendrocyte progenitor cells
PAMP pathogen-associated molecular patterns
PCL: polycaprolactone
PDGF platelet-derived growth factor;
PLA polylactate
PLGA poly(lactic-co-glycolic acid)
PnD perinatal derivatives
PNS peripheral nervous system
ROS reactive oxygen species
RGD Arginyl-glycyl-aspartic acid
SFI sciatic functional index
TEER Trans endothelial electric resistance
TGF- β1 transforming growth factor beta 1
TIMP tissue inhibitor of metalloproteinases
TNBS trinitrobenzene sulfonate;
TNFα tumor necrosis factor-alpha
UC-MSC umbilical cord mesenchymal stromal cells
UC-WJ-MSC Umbilical cord Wharton’s jelly mesenchymal stromal cells
VEGF vascular endothelial growth factor
VML volumetric muscle loss
WRL withdrawal reflex latency
XRD X-ray diffraction.
Keywords: perinatal derivatives, amniotic membrane and fluid stem cells, extracellular vesicles, tissue regeneration, regenerative medicine
Citation: Pozzobon M, D’Agostino S, Roubelakis MG, Cargnoni A, Gramignoli R, Wolbank S, Gindraux F, Bollini S, Kerdjoudj H, Fenelon M, Di Pietro R, Basile M, Borutinskaitė V, Piva R, Schoeberlein A, Eissner G, Giebel B and Ponsaerts P (2022) General consensus on multimodal functions and validation analysis of perinatal derivatives for regenerative medicine applications. Front. Bioeng. Biotechnol. 10:961987. doi: 10.3389/fbioe.2022.961987
Received: 05 June 2022; Accepted: 01 July 2022;
Published: 03 October 2022.
Edited by:
Vahid Serpooshan, Emory University, United StatesReviewed by:
Angliana Chouw, PT Prodia Stem Cell Indonesia, IndonesiaRamesh Bhonde, Dr. D.Y. Patil Vidyapeeth, India
Copyright © 2022 Pozzobon, D’Agostino, Roubelakis, Cargnoni, Gramignoli, Wolbank, Gindraux, Bollini, Kerdjoudj, Fenelon, Di Pietro, Basile, Borutinskaitė, Piva, Schoeberlein, Eissner, Giebel and Ponsaerts. This is an open-access article distributed under the terms of the Creative Commons Attribution License (CC BY). The use, distribution or reproduction in other forums is permitted, provided the original author(s) and the copyright owner(s) are credited and that the original publication in this journal is cited, in accordance with accepted academic practice. No use, distribution or reproduction is permitted which does not comply with these terms.
*Correspondence: Michela Pozzobon, bS5wb3p6b2JvbkBpcnBjZHMub3Jn, bWljaGVsYS5wb3p6b2JvbkB1bmlwZC5pdA==; Peter Ponsaerts, cGV0ZXIucG9uc2FlcnRzQHVhbnR3ZXJwZW4uYmU=