- 1Laboratory of Veterinary Physiology, Tokyo University of Agriculture and Technology, Tokyo, Japan
- 2Central Research Institute for Feed and Livestock, ZEN-NOH (National Federation of Agricultural Cooperative Associations), Ibaraki, Japan
- 3Department of Food, Agriculture and Environment, Miyagi University, Miyagi, Japan
- 4Laboratory of Animal Science, Department of Applied Biological Sciences, Setsunan University, Osaka, Japan
- 5Institute of Zoology, Chinese Academy of Sciences, Beijing, China
The initial colonization and early development of the intestinal microbiome are important stages in the growth of calves during pre-weaning. This study investigated temporal changes in the diversity and composition of the fecal microbiota, focusing on the first 2 weeks after birth, with the aim of identifying intestinal bacteria and blood metabolites that are associated with calf diarrhea. In this study, 10 calves were fed colostrum on day 0 after birth, whole milk from days 2 to 5, and milk replacer from day 6. Six calves showed mild diarrhea in the second week (i.e., from day 8 to 14). We observed dramatic changes in the development of the fecal microbiome from day 2 to 14 and found several bacterial species, such as Lactobacillus and Collinsella, changing significantly in abundance during the milk transition (i.e., from day 4 to 10). In investigating whether there is an association between the microbiome and the milk transition, we found the level of hypotaurine and D-xylose to be significantly higher in whole milk from days 4 and 5 than in milk replacer. A comparison of four healthy calves and six diarrheal calves revealed that the diarrheal calves had a low abundance of Collinsella on day 10. Furthermore, we explored plasma metabolites statistically correlated with the change of fecal Collinsella and found a high level of dimethylglycine in healthy calves. Taken together, these findings suggest a possible link between temporary changes in the fecal microbiome and neonatal diarrhea during the milk transition in calves.
Introduction
The gastrointestinal microbiome has been proven to be a key feature in the relationship between health and disease across animal species. A balanced microbiome composition, characteristic of each host species, is necessary for maintaining the intestinal mucosal barrier, antagonizing the colonization of pathogenic bacteria, and contributing to metabolism and immune homeostasis (O'Hara & Shanahan, 2006). Disruption of the composition of microbiota, known as “dysbiosis,” can trigger several diseases, including gastrointestinal disorders (Sommer et al., 2017). Neonatal diarrhea is predominantly caused by enteric infections during the early stages of life in mammals, when the gastrointestinal microbiome starts to colonize and develop (Bauer et al., 2006).
Dairy cattle are known to have a diverse community of ruminal bacteria that provides energy for growth and milk production, whereas the rumen of neonatal calves is non-functional but starts growing on the addition of cereal to their diets at 3 or 4 weeks of age (Baldwin et al., 2004). It has been suggested that the intestinal (not ruminal) microbiome plays an instrumental role in maintaining calf health by not only providing nutrients and energy, but also stimulating the host immune system to combat pathogens (Yeoman & White, 2014; Malmuthuge & Guan, 2017; Malmuthuge et al., 2019). Neonatal diarrhea is one of the major causes of calf morbidity and mortality and has a large impact on the economics of dairy farms (Uetake, 2013; USDA, 2018). Although enteric infection is often observed, the cause of neonatal diarrhea is difficult to define because it commonly presents with coinfections and most pathogens, consistent with the occurrence of neonatal diarrhea, can also be found in healthy individuals (Cho et al., 2013; Adaska et al., 2017). Recently, studies have reported differences in fecal microbial communities between healthy and diarrheal calves during the first few weeks of life (Oikonomou et al., 2013; Gomez et al., 2017; Zeineldin et al., 2018).
The development of a healthy intestinal microbiome is profoundly influenced by the calf’s diet, which is essential in preventing calf diarrhea (Malmuthuge & Guan, 2017; Badman et al., 2019). In general, dairy calves are fed colostrum within a few hours of birth and their diet is changed from whole milk to milk replacer or a solid diet within 2–7 days. There are various management protocols to optimize the process of milk transition and weaning (Khan et al., 2011). However, even under a proper management protocol, neonatal diarrhea is often observed after the milk transition. More importantly, some calves develop diarrhea and others do not, even under the same management protocol. These observations prompted us to investigate changes in the intestinal microbiota before and after the milk transition, and to explore whether there is an association between changes in the microbiome and neonatal diarrhea.
In this study, we analyzed the change in the fecal microbiome by next-generation sequencing and determined the metabolite profiles of colostrum, whole milk, and milk replacer by untargeted metabolomics using gas chromatography–mass spectrometry (GC-MS). The abundance of bacteria significantly changed during the milk transition, and there were different levels of one species of bacterium, Collinsella, between healthy and diarrheal calves. In addition, we found that levels of dimethylglycine in plasma, which show a positive correlation with the abundance of Collinsella, decreased in the diarrheal calves. This study suggests that a temporary change in the fecal microbiome during the milk transition may be associated with diarrhea in early neonatal calves.
Materials and methods
Animals and sample collection
A total of 10 female Holstein calves born from March to June 2019 were randomly enrolled in this study for fecal and plasma sampling. In addition, the calves’ mothers (10 Holstein cows) were used for collecting corresponding milk samples. The newborn calves were moved to the calf hutch 1 h after birth. From each cow, colostrum was obtained within 1 h after birth (day 0), and whole milk was collected daily at 8:00 and 16:30 with a bucket milker from days 1 to 5 after parturition. The milk was warmed to approximately 38°C and artificially fed twice a day to the calves using a feeding bottle. The calves were fed the milk collected from their respective mothers. Milk replacer containing 28% crude protein (CP) and 4.23 Mcal/kg metabolizable energy (ME) (for composition see Supplementary Table S1) was fed to the calves at 9:30 and 16:30 from days 6 to 48 after birth. Calf starter, including 19% CP and 2.89 ME (for composition see Supplementary Table S1), and roughage (timothy hay) were fed ad libitum from day 6 after birth. A summary of the feeding procedure is shown in Supplementary Figure S1. Feces and blood samples were collected from calves at 9:00 on days 2, 4, 6, 8, 10, and 14 after birth and stored at –20°C until analysis. Fecal consistency was monitored, and feces showing a liquid appearance were recorded as diarrhea. All experiments were performed in accordance with the guidelines of the University Animal Care and Use Committee of the Tokyo University of Agriculture and Technology (Fuchu, Japan) (R03-176).
DNA extraction from fecal samples
Genomic DNA from the collected fecal samples was extracted using a QuickGene DNA tissue kit S (Kurabo Industries Ltd, Osaka, Japan) in accordance with the manufacturer’s instructions. In brief, 40 mg of feces, 15 mg of 0.2-mm glass beads (No.02, Toshin Riko Co., Ltd, Tokyo, Japan), and 200 µl of tissue lysis buffer were mixed and bead homogenized at 3,000 rpm for 120 s using a Micro Smash Cell Disrupter (MS-100, Tomy Seiko Co., Ltd, Tokyo, Japan). Proteinase K (25 µl) was added to the homogenized sample and the sample was incubated at 55°C for 1 h. The sample was then centrifuged for 10 min at 16,000 × g at 20°C, and the supernatant was transferred to another microtube containing 180 µl of lysis buffer and incubated at 70°C for 10 min. After incubation, 240 µl of > 99% ethanol was added to the microtube and vortexed at the maximum speed for 15 s. The whole lysate was transferred to a QuickGene Mini480 cartridge and pressurized. Wash buffer (750 µl) was applied three times to the cartridge. After the third wash, 200 µl of elution buffer was added and the mixture was incubated at 20°C. DNA was extracted after the third pressurization. The DNA concentration was adjusted to 20 ng/µl using elution buffer.
Library preparation and DNA sequencing
16S ribosomal RNA (rRNA) metagenomic analysis of fecal samples was performed. Briefly, a PCR targeting the variable regions 3 and 4 (V3–4) of the 16S rRNA gene was performed using the primers 341F (5′-CCTACGGGNGGCWGCAG-3′) and 805R (5′-GACTACHVGGGTATCTAATCC-3′), followed by a second PCR to attach dual indices. The amplicon of the second PCR was purified and the concentration was normalized with a SequalPrep Normalization Plate Kit (Life Technologies, Tokyo, Japan). The size and quantity of the library were assessed with a Bioanalyzer 2100 (Agilent Technologies Japan Ltd, Tokyo, Japan) and a Library Quantification Kit for Illumina (Kapa Biosystems, Inc., Wilmington, MA, USA), respectively. The library was mixed with phiX control and sequenced using a MiSeq v3 kit (Illumina Inc., San Diego, CA, USA) in accordance with the manufacturer’s instructions. Data from the sequences were processed using QIIME 2™ (version 2021.11), and the imported reads were denoised using the DADA2 plugin to generate the amplicon sequence variant (ASV) feature table. Singleton and ASVs assigned to mitochondria and chloroplasts were excluded from further analysis. Taxonomy was assigned to filtered ASVs using a pretrained QIIME 2-compatible SILVA version 138 database (99% full-length sequences). We assayed for microbial variance using the MicrobiomeAnalyst software with default settings (URL: www.microbiomeanalyst.ca). All 16S rRNA sequencing data were deposited in September 2022 and are available under the accession number PRJNA835625.
Sample preparation for metabolomic analysis
The whole-milk samples collected from individual calves’ mothers were centrifuged at 21,500 × g for 60 min at 4°C, and the middle layer was collected as skimmed milk. The milk replacers, prepared for each of the calves, were also centrifuged. Blood anticoagulated with ethylenediaminetetraacetic acid (EDTA) was centrifuged at 2,000 × g for 20 min at 4°C, and plasma samples were collected.
An untargeted metabolomics analysis was performed using GC-MS, as described previously, with some modifications (Usuda et al., 2018). For the pretreatment solution of the plasma, 50 μl of filtered plasma was mixed with 250 μl of methanol–chloroform–water (2.5 : 1 : 1) and 5 μl of 1 mg/ml 2-isopropylmalic acid as an internal standard. The samples were subsequently mixed in a shaker at 1200 rpm at 37°C for 30 min and centrifuged at 16,000 × g at 4°C for 5 min, and 225 μl of the supernatant was mixed with 200 μl of distilled water and vortexed. This was followed by centrifugation at 16,000 × g at 4°C for 5 min, and 250 μl of the supernatant was partially dried using a centrifugal evaporator CVE-2200 (Tokyo Rikakikai Co., Ltd, Tokyo, Japan) for 30 min and frozen at –80°C. The frozen samples were completely dried using vacuum freeze-drying equipment FDU-1200 (Tokyo Rikakikai Co., Ltd, Tokyo, Japan) for 16 h. The dried samples were dissolved in 40 µl of 20 mg/ml pyridine-containing methoxamine hydrochloride (Sigma-Aldrich Co. LLC, St. Louis, MO, USA) and incubated in a shaker at 1,200 rpm at 30°C for 90 min. To this, 20 μl of N-methyl-N-trimethylsilyl-trifluoroacetamide (MSTFA, Thermo Fisher Scientific, Waltham, MA, USA) was added and the samples further incubated in a shaker at 1,200 rpm at 37°C for 45 min.
For pretreatment of the skimmed milk, 50 μl of filtered skimmed milk was mixed with 50 µl of water, 800 µl of acetonitrile, and 5 μl of 1 mg/ml 2-isopropylmalic acid. The samples were subsequently mixed in a shaker at 1200 rpm at 37°C for 30 min and centrifuged at 16,000 × g at 4°C for 5 min. After centrifugation, the derivatization of skimmed milk samples was performed using a solid-phase derivatization kit for metabolome analysis (AiSTI SCIENCE Co., Ltd, Wakayama, Japan).
Metabolomic analysis using gas chromatography–mass spectrometry
One microliter (plasma) or 2 µl (milk) of the derivatized sample was injected splitless into a GC-MS QP2020 NX (Shimadzu Corporation, Kyoto, Japan). The capillary column was a 1.00-μm DB5 column (30 m × 0.25 mm i.d.; Agilent Technologies, Inc., Santa Clara, CA, USA). The temperature of the vaporizing chamber was 280°C, and the gas flow rate through the column was maintained at 5 ml/min. The temperature program was as follows: 4 min of isothermal heating at 100°C, which was then increased to 320°C at 10°C/min and held at 320°C for 11 min. Ions were generated at 70 eV using electron ionization and recorded at intervals of 0.2 s over the mass range of 45–600 m/z. The metabolites were identified using the standard Smart Metabolites Database (Shimadzu Corporation, Kyoto, Japan), with each peak being identified manually using LabSolution (Shimadzu Corporation, Kyoto, Japan), and data analysis conducted using MetaboAnalyst (URL: www.metaboanalyst.ca/) (Chong et al., 2019).
Statistical analysis
The data presented were analyzed and visualized using MicrobiomeAnalyst, MetaboAnalyst, and GraphPad Prism 9 (GraphPad Software Inc., San Diego, CA, USA). In microbiome analysis, the beta diversity of the fecal microbiome was plotted by principal coordinate analysis (PCoA) on unweighted unique fraction metric (UniFrac) distance and permutational multivariate analysis of variance. A linear discriminant analysis effect size (LEfSe) analysis was performed to discriminate microbial features among groups. In metabolomics analysis, partial least-squares discriminant analysis (PLS-DA) was used to visualize the separation among the groups [i.e., colostrum (day 0), whole milk (days 1, 2, 3, 4, and 5 after parturition), and milk replacer], and to identify important metabolites responsible for the separation. To further analyze the differences between the whole milk and milk replacer, a volcano plot selected significant metabolites with a fold change threshold of 10 and a t-test threshold of p < 0.05. Correlations between the selected microbial and serum metabolites were analyzed by Pearson’s correlation test. The identified bacteria and metabolites were statistically compared between healthy and diarrheal calves using Bonferroni correction with Dunn’s test. Differences were considered statistically significant at a p-value < 0.05.
Results
Changes in fecal microbiome composition during milk transition
To investigate the compositional changes in the fecal microbiome during the milk transition, 16S rRNA gene sequencing was conducted using fecal samples from day 2 to 14 after birth. In total, 1,376,154 (mean ± SD: 22,936 ± 5,020 reads/sample) sequence reads were obtained from 60 samples. As shown in Figure 1A, the alpha diversity index represents the dynamics of the microbiome composition. Chao1, which is defined as “richness,” and Shannon, which is defined as “evenness,” increased from day 2 to 14 after birth. Similarly, in the PCoA plot based on the unweighted UniFrac distance, beta diversity gradually changed from day 2 to 14 (Figure 1B). In this study, all calves were fed whole milk from their mothers until day 5 after birth, but were switched to a milk replacer on day 6 (Supplementary Figure S1 and Figure 2A). To detect the specific bacteria associated with the milk transition, we conducted the LEfSe using samples from days 4, 6, 8, and 10, and identified four bacterial genera, namely Clostridium sensu stricto 2, Lactobacillus, Collinsella, and Phascolarctobacterium, showing high logarithmic linear discriminant analysis scores (Figures 2B, C).
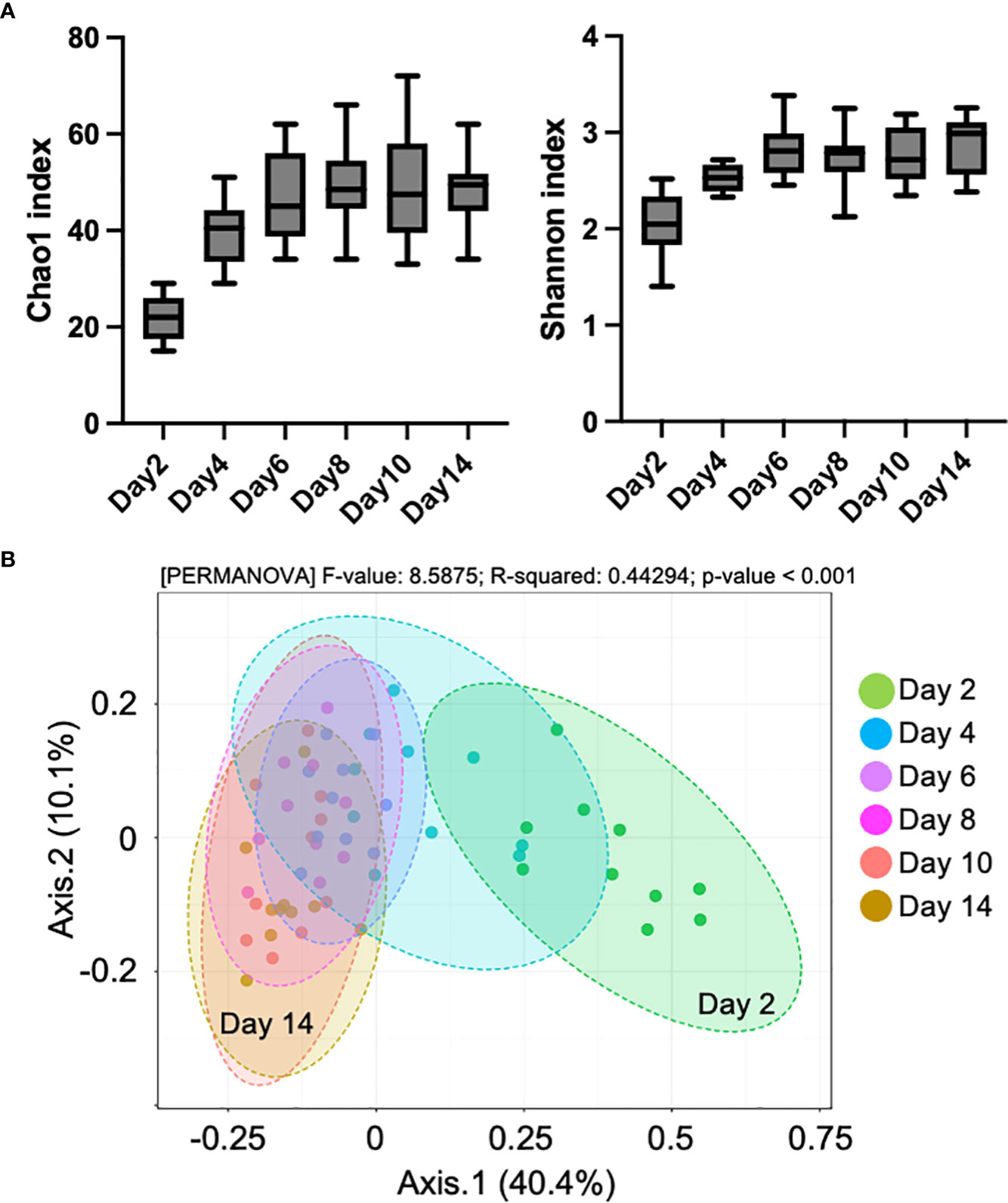
Figure 1 Changes in fecal microbiome composition in pre-weaned calves. (A) Chao1 and Shannon index of alpha diversity in fecal samples from day 2 to 14 after birth (n = 10). (B) Plots of principle coordinate analysis based on the unweighted unique fraction metric (UniFrac) distance of microbial communities.
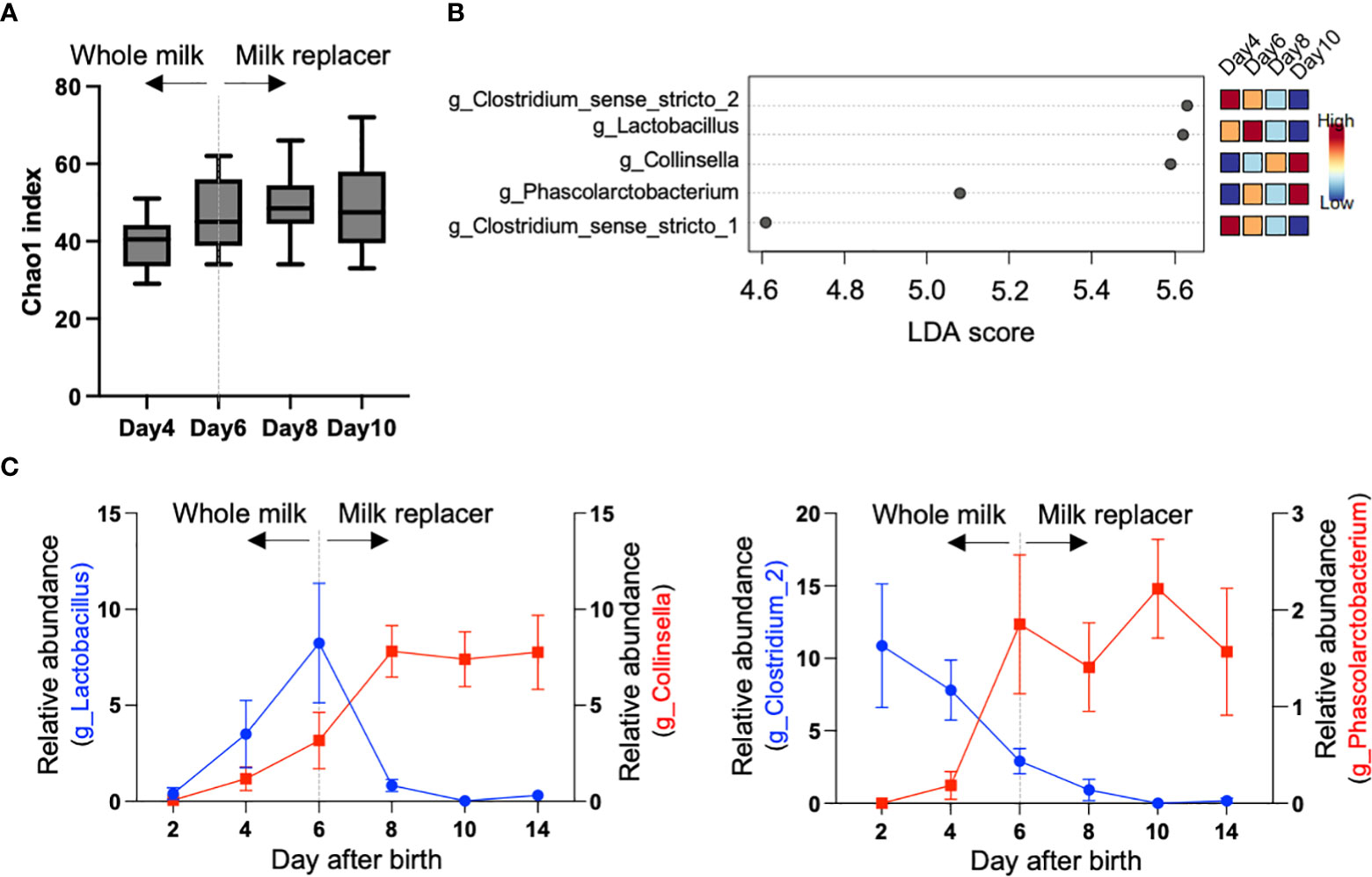
Figure 2 Changes of specific bacteria during milk transition. (A) Chao1 index of alpha diversity during milk transition (n = 10). All calves were switched from whole milk from their mother to a milk replacer on day 6. (B) Specific genera identified by linear discriminant analysis effect size (LEfSe). The colored boxes on the right indicate the relative abundance of the corresponding genera each day. (C) Changes of Clostridium_sense_stricto_2, Lactobacillus, Collinsella, and Phascolarctobacterium during milk transition.
Differences in metabolic components between whole milk and milk replacer
Untargeted metabolomic analysis of the milk samples detected 113 metabolites, and PLS-DA showed that colostrum on day 0 had a high variability among the mother cows, but the variation decreased each day and the metabolite composition in whole milk was stable on day 4 and day 5 after parturition. Milk replacer formed a separate cluster from the whole-milk sample (Figure 3A). The patterns in the abundance of several metabolites were identified by PLS-DA. For example, fructose 6-phosphate, mannose 6-phosphate, and glucose 6-phosphate decreased, whereas orotic acid and ureidosuccinic acid increased, from day 0 (in colostrum) to 5 (in whole milk and the milk replacer) (Figure 3B). To identify further differences in metabolites between the whole milk (days 4 and 5) and the milk replacer, we constructed a volcano plot, which identified seven metabolites, as shown in Figure 3C (p < 0.05, fold change threshold 10). Histograms denoting the relative abundance of the metabolites hypotaurine, D-xylose, and ureidosuccinic acid in the milk replacer and whole milk (days 4 and 5) are shown in Figure 3C (right).
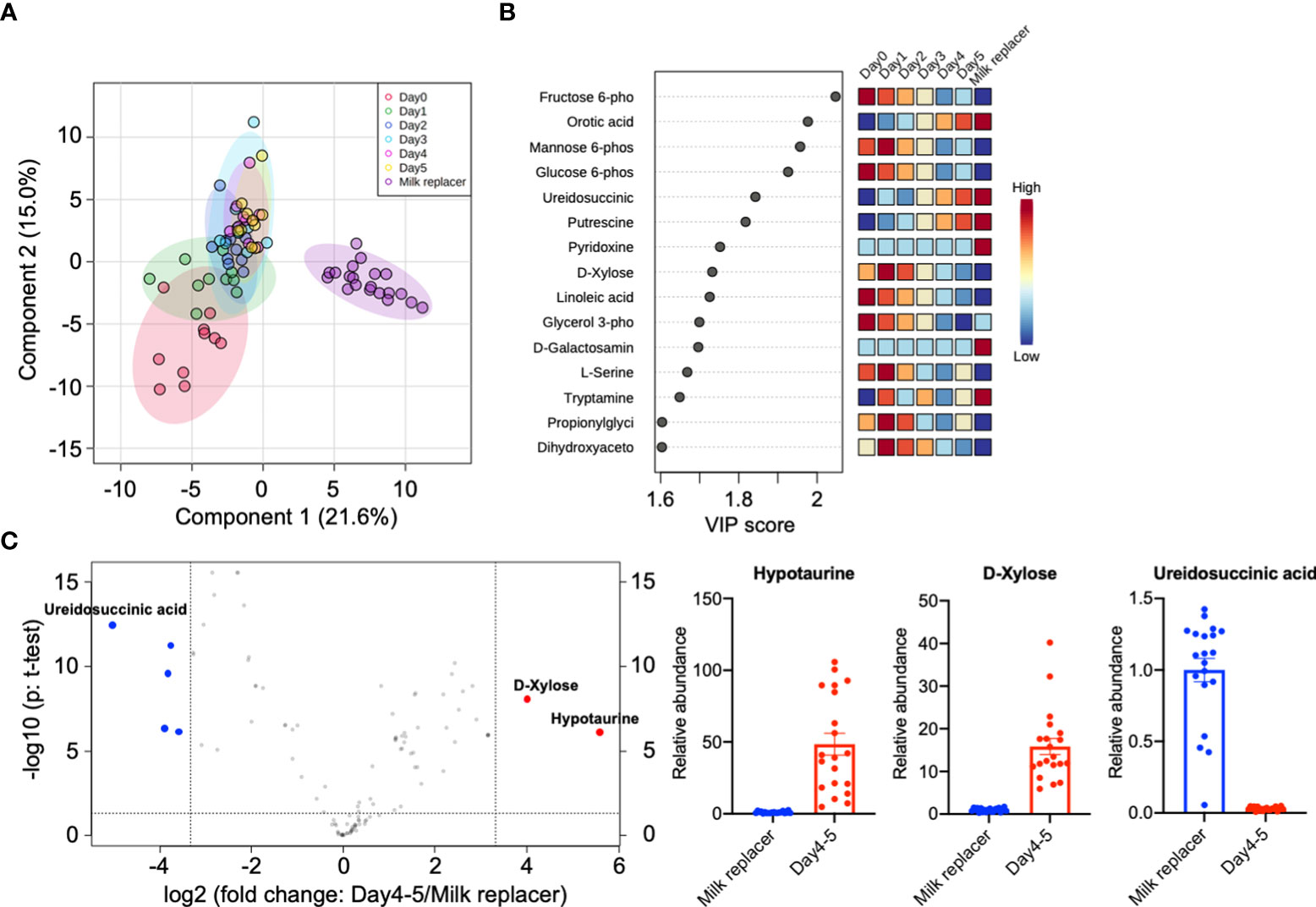
Figure 3 Differences in metabolic components between whole milk and milk replacer. (A) 2D principal component analysis score plot of colostrum (day 0, n = 10), whole milk (days 1, 2, 3, 4, and 5 after parturition, n = 10 each), and milk replacer (n = 20). (B) Important features identified by partial least-squares discriminant analysis (PLS-DA). The colored boxes on the right indicate the relative concentrations of the corresponding metabolites in each group. (C) Statistically significant features with a fold change threshold of 10 (x-axis) and a t-test threshold of p < 0.05 (y-axis) selected by a volcano plot. The red circles represent higher metabolites in whole milk on days 4 and 5 and the blue circles represent higher metabolites in milk replacer. On the right, histograms show the relative abundance of hypotaurine, D-xylose, and ureidosuccinic acid in milk replacer and whole milk on days 4 and 5.
Differences in the fecal microbiome between healthy and diarrheal calves
One calf in the first week after birth and six calves in the second week after birth showed mild diarrhea (Figure 4A and Supplementary Table S2). Diarrhea observed during this period was temporary and all animals recovered the next day, and body weight gain was normal for all calves (Supplementary Figure S2). Calves were divided into two groups (i.e., four healthy calves and six diarrheal calves) based on the observation of diarrhea in the second week after birth. There was no difference in the Chao1 index between healthy and diarrheal calves (Figure 4B). Of the four bacteria that change their abundance during the milk transition, Collinsella revealed a different trend in comparing healthy and diarrheal calves. As shown in Figure 4C, Collinsella on day 10, after milk transition, was lower in diarrheal calves than in healthy calves.
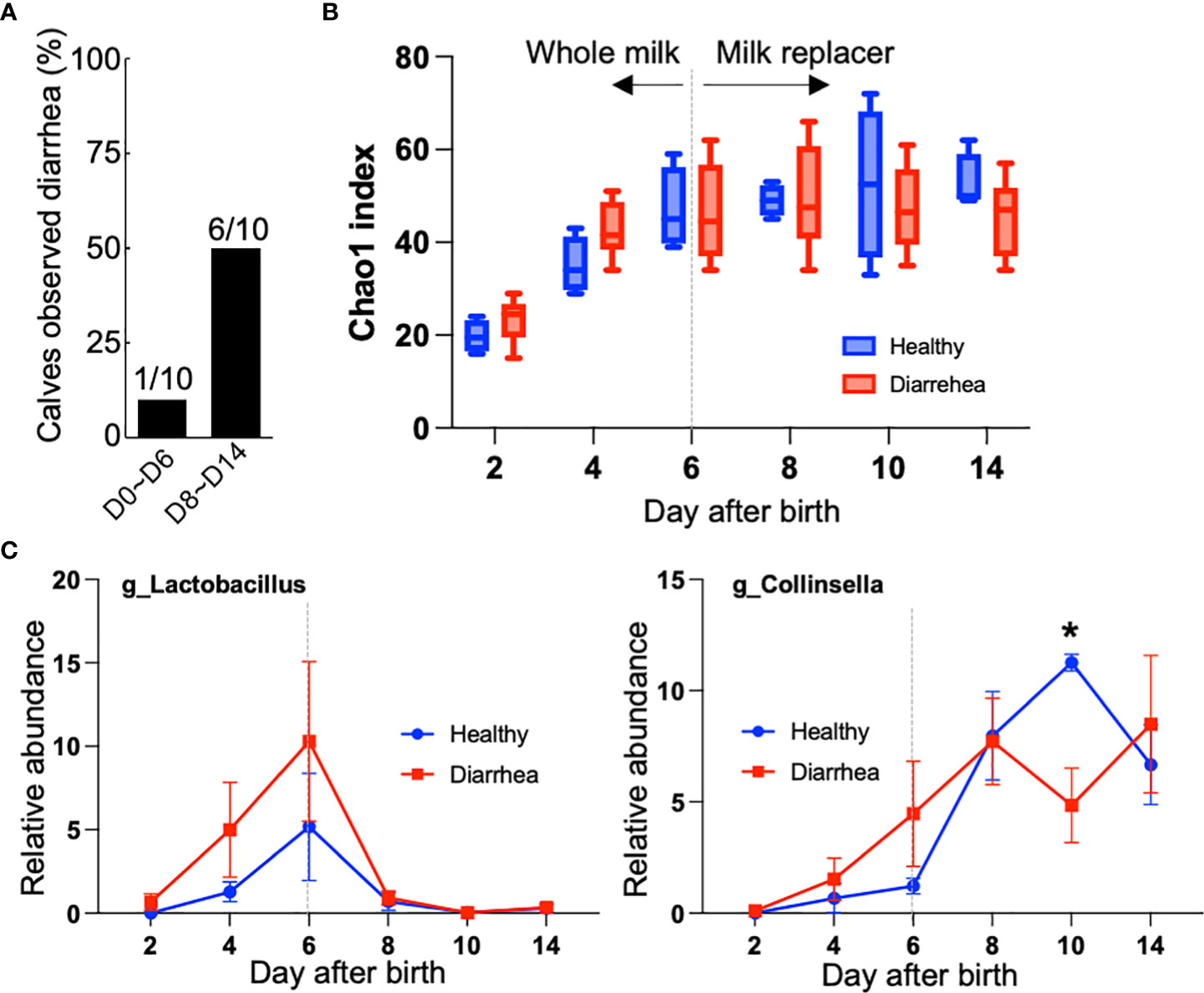
Figure 4 Differences in fecal microbiome between healthy calves and diarrheal calves. (A) Number of calves with diarrhea in the first or second week after birth. (B) Chao1 index of alpha diversity during milk transition. Calves were divided into two groups (i.e., four healthy calves and six diarrheal calves) based on the observation of diarrhea in the second week after birth. (C) Changes of Lactobacillus and Collinsella in feces from healthy and diarrheal calves during milk transition. Asterisk indicates significant difference between healthy calves and diarrheal calves using the Bonferroni Correction with Dunn Test method (p < 0.05).
Differences in plasma metabolites between healthy and diarrheal calves
We hypothesized that Collinsella is an important bacterium in the early development of calves; therefore, we explored plasma metabolites that correlate with the abundance of Collinsella in calf feces. Pearson’s correlation analysis revealed that dimethylglycine, beta-alanine, and glyoxylic acid were positively correlated with the abundance of Collinsella (Figure 5A). As shown in Figure 5B, plasma dimethylglycine showed a different trend when comparing healthy and diarrheal calves.
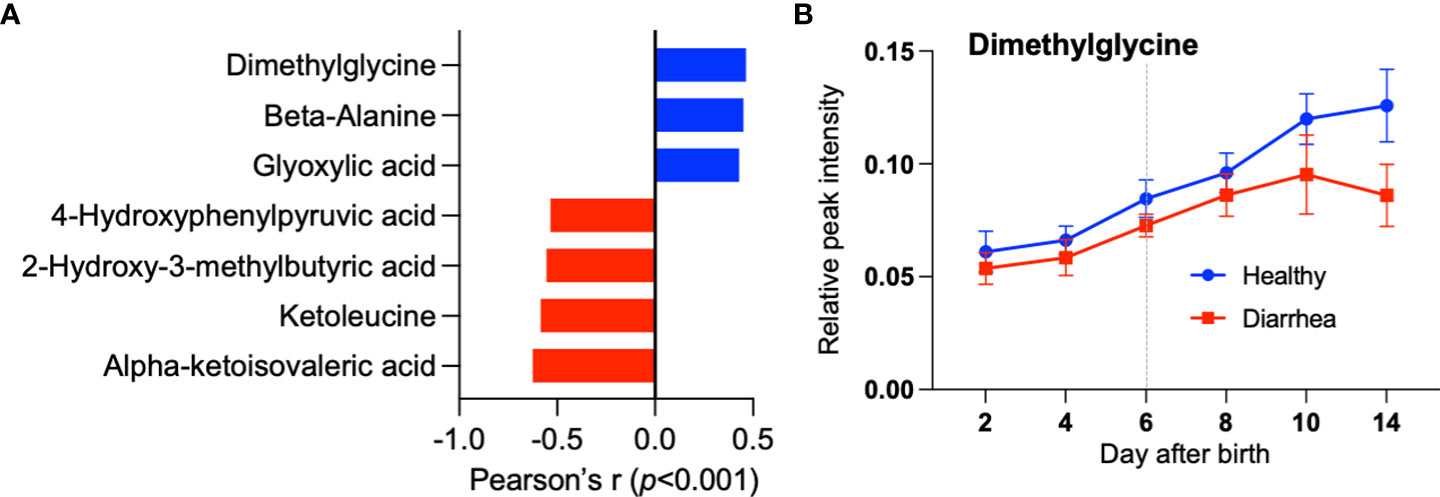
Figure 5 Differences in plasma metabolites between healthy and diarrheal calves. (A) Identified serum metabolites correlated with the abundance of Collinsella by Pearson’s correlation analysis. (B) Change of dimethylglycine in serum from healthy and diarrheal calves during milk transition.
Discussion
The gut microbiome encompasses the bacteria inhabiting the gastrointestinal tract. The gut microbiome plays a critical role in the development and functioning of the gastrointestinal tract and overall gut health across animal species. The disruption of this optimal bacterial community (i.e., dysbiosis) can contribute to gastrointestinal disorders. Neonatal diarrhea is often observed in calves with an immature rumen and has been associated with intestinal microbiome colonization and development during the pre-weaning period. In this study, we focused in particular on the first 2 weeks after birth, when the milk transition, from whole milk to milk replacer, took place, and found that the levels of several fecal bacteria, such as Lactobacillus and Collinsella, changed significantly during the milk transition. A comparison of healthy and diarrheal calves indicated that Collinsella decreased after the milk transition in diarrheal calves. Furthermore, we found that plasma dimethylglycine, which shows a positive correlation with Collinsella, also decreased in the diarrheal calves. This study suggests a link between temporary changes in the fecal microbiome and neonatal diarrhea during the milk transition in calves.
A calf’s intestinal microbiome has a simple and less diverse bacterial community at birth and it increases in complexity and diversity with growth (Mayer et al., 2012; Oikonomou et al., 2013; Dill-McFarland et al., 2017). We observed dramatic development of the fecal microbiome, with an increase in the alpha diversity (Chao1 and Shannon), from day 2 to 14 after birth. Analysis of the beta diversity also showed that the composition gradually changed as the calves grew. LEfSe identified four bacterial genera that showed significant changes during the milk transition, with a linear discriminant analysis score >5.0. It was reported that the postnatal intestinal microbiome is profoundly influenced by the type of milk (Deng et al., 2017; Badman et al., 2019) and, therefore, we also investigated the compositional differences between whole milk (from days 4 and 5 after birth) and milk replacer. Untargeted metabolomic analysis found that the milk replacer was deficient in hypotaurine and D-xylose compared with whole milk. Hypotaurine, a precursor of taurine, is known as an antioxidant and is involved in a variety of crucial biological functions, including cell proliferation, immunomodulation, and oxidative stress inhibition (Green et al., 1991; Bouckenooghe et al., 2006; Sakuragawa et al., 2010). A recent report indicated that dietary polyphenols, a major group of antioxidants, are relevant in the modulation of the human gut microbiome and that gut bacteria convert polyphenols into active and bioavailable metabolites for host health (Tomás-Barberán et al., 2016). In addition, hypotaurine and D-xylose can be utilized by bacteria as a source of carbon, nitrogen, and energy for growth; therefore, a milk replacer deficient in hypotaurine and D-xylose might alter development of the intestinal microbiome. These findings suggest that these metabolites could be used to supplement milk replacers as a means of promoting a beneficial microbiome in the early development of calves.
In this study, one calf in the first week after birth and six calves in the second week after birth showed mild diarrhea. Ma et al. (2020) reported that most of the diarrhea incidence in veal calves occurred between 7 and 21 days of age and speculated that this might be associated with the lower relative abundance of Blautia and higher relative abundance of Escherichia-Shigella at 14 days of age. Although we could not detect those two bacterial changes by LEfSe, we found that the abundance of Collinsella was significantly higher in healthy calves than in diarrheal calves on day 10. In humans, Collinsella and Bifidobacterium are known as the major lactose utilizers. Collinsella and Bifidobacterium act to modify the bile acids of the host and modulate the virulence and pathogenicity of enteric pathogens (Rajilić-Stojanović & De Vos, 2014). It was also reported that zinc supplementation to newborn calves for 14 days reduced the incidence of diarrhea, and the relative abundance of Collinsella was higher in those calves (Chang et al., 2020). Taken together, this study further suggests that low levels of Collinsella abundance during the milk transition may be associated with an increased incidence of diarrhea.
Further investigation revealed that plasma metabolites, such as dimethylglycine, beta-alanine, and glyoxylic acid, were positively correlated with the abundance of fecal Collinsella. Further experiments are required to determine the detailed relationship between these metabolites in plasma and Collinsella in the fecal microbiota; however, dimethylglycine is known as an anti-stress nutrient with antioxidant properties, and it has been reported to improve offspring growth performance in piglets (Bai et al., 2022). It is also known that dimethylglycine can be derived from gut microbiota, and microbiome- and smoking cessation-induced weight gain involves a concerted host and microbiome shunting of dietary choline to dimethylglycine, which drives an increased gut energy harvest (Fluhr et al., 2021). These observations suggest that dimethylglycine may be an essential nutrient for the early development of calves and healthy growth.
In conclusion, we investigated the temporal changes in the fecal microbiome and plasma metabolites during the milk transition in early neonatal calves. We found that the bacterium Collinsella and the metabolite dimethylglycine were key factors associated with the milk transition and/or diarrhea. However, the number of animals used in this study was small, and, because it was primarily an observational study using next-generation sequencing, further research is warranted to verify the validity of the suggested links between the bacteria, the metabolite, and diarrheal incidence in the early lives of calves.
Data availability statement
The datasets presented in this study can be found in online repositories. The names of the repository/repositories and accession number(s) can be found below: https://www.ncbi.nlm.nih.gov/genbank/, PRJNA835625.
Ethics statement
The animal study was reviewed and approved by Animal Care and Use Committee of the Tokyo University of Agriculture and Technology (R03-176).
Author contributions
MK, ST, and KN contributed to the conception and design of the study. HL and RI conducted next-generation sequencing analyses. YS performed the statistical analysis. MK wrote the first draft of the manuscript. GW and WJ wrote sections of the manuscript. All authors contributed to manuscript revision, read, and approved the submitted version.
Acknowledgments
We would like to thank Editage (Karnataka, India; URL: www.editage.com) for English language editing.
Conflict of interest
The authors declare that the research was conducted in the absence of any commercial or financial relationships that could be construed as a potential conflict of interest.
Publisher’s note
All claims expressed in this article are solely those of the authors and do not necessarily represent those of their affiliated organizations, or those of the publisher, the editors and the reviewers. Any product that may be evaluated in this article, or claim that may be made by its manufacturer, is not guaranteed or endorsed by the publisher.
Supplementary material
The Supplementary Material for this article can be found online at: https://www.frontiersin.org/articles/10.3389/fanim.2022.934204/full#supplementary-material
Supplementary Figure 1 | Diet of calves (n = 10) until day 56. Bar graph: Intake of milk feed per day (colostrum, whole milk, and milk replacer). Line graph: Intake of solid diet per day (calf starter pellet and roughage).
Supplementary Figure 2 | Weight of individual calf until day 56. The average weight gain rate showed no significant difference.
Supplementary Table 1 | Composition of milk replacer and calf starter.
Supplementary Table 2 | Diarrhea incidence and antibiotic treatment during the 2 weeks after birth.
Supplementary Table 3 | Comparison of feed intake of healthy and diarrheal calves.
References
Adaska J. M., Moeller R. B., Blanchard P. C., Aly S. S. (2017). Cecal infarction in neonatal calves. J. Veterinary Diagn. Invest. 29, 242–244. doi: 10.1177/1040638716688046
Badman J., Daly K., Kelly J., Moran A. W., Cameron J., Watson I., et al. (2019). The effect of milk replacer composition on the intestinal microbiome of pre-ruminant dairy calves. Front. Veterinary Sci. 6. doi: 10.3389/fvets.2019.00371
Bai K., Jiang L., Wang T. (2022). Dimethylglycine sodium salt alleviates intrauterine growth restriction-induced low growth performance, redox status imbalance, and hepatic mitochondrial dysfunction in suckling piglets. Front. Veterinary Sci. 9. doi: 10.3389/fvets.2022.905488
Baldwin V. I. R. L., McLeod K. R., Klotz J. L., Heitmann R. N. (2004). Rumen development, intestinal growth and hepatic metabolism in the pre- and postweaning ruminant. J. Dairy Sci. 87, E55–E65. doi: 10.3168/jds.S0022-0302(04)70061-2
Bauer E., Williams B. A., Smidt H., Verstegen M. W., Mosenthin R. (2006). Influence of the gastrointestinal microbiota on development of the immune system in young animals. Curr. Issues Intestinal Microbiol. 7, 35–51.
Bouckenooghe T., Remacle C., Reusens B. (2006). Is taurine a functional nutrient? Curr. Opin. Clin. Nutr. Metab. Care 9, 728–733. doi: 10.1097/01.mco.0000247469.26414.55
Chang M. N., Wei J. Y., Hao L. Y., Ma F. T., Li H. Y., Zhao S. G., et al. (2020). Effects of different types of zinc supplement on the growth, incidence of diarrhea, immune function, and rectal microbiota of newborn dairy calves. J. Dairy Sci. 103, 6100–6113. doi: 10.3168/jds.2019-17610
Cho Y. I., Han J. I., Wang C., Cooper V., Schwartz K., Engelken T., et al. (2013). Case-control study of microbiological etiology associated with calf diarrhea. Veterinary Microbiol. 166, 375–385. doi: 10.1016/j.vetmic.2013.07.001
Chong J., Wishart D. S., Xia J. (2019). Using MetaboAnalyst 4.0 for comprehensive and integrative metabolomics data analysis. Curr. Protoc. Bioinf. 68, e86. doi: 10.1002/cpbi.86
Deng Y. F., Wang Y. J., Zou Y., Azarfar A., Wei X. L., Ji S. K., et al. (2017). Influence of dairy by-product waste milk on the microbiomes of different gastrointestinal tract components in pre-weaned dairy calves. Sci. Rep. 7, 42689. doi: 10.1038/srep42689
Dill-McFarland K. A., Breaker J. D., Suen G. (2017). Microbial succession in the gastrointestinal tract of dairy cows from 2 weeks to first lactation. Sci. Rep. 7, 40864. doi: 10.1038/srep40864
Fluhr L., Mor U., Kolodziejczyk A. A., Dori-Bachash M., Leshem A., Itav S., et al. (2021). Gut microbiota modulates weight gain in mice after discontinued smoke exposure. Nature 600, 713–719. doi: 10.1038/s41586-021-04194-8
Gomez D. E., Arroyo L. G., Costa M. C., Viel L., Weese J. S. (2017). Characterization of the fecal bacterial microbiome of healthy and diarrheic dairy calves. J. Veterinary Internal Med. 31, 928–939. doi: 10.1111/jvim.14695
Green T. R., Fellman J. H., Eicher A. L., Pratt K. L. (1991). Antioxidant role and subcellular location of hypotaurine and taurine in human neutrophils. Biochim. Biophys. Acta 1073, 91–97. doi: 10.1016/0304-4165(91)90187-l
Khan M. A., Weary D. M., von Keyserlingk M. A. (2011). Invited review: Effects of milk ration on solid feed intake, weaning, and performance in dairy heifers. J. Dairy Sci. 94, 1071–1081. doi: 10.3168/jds.2010-3733
Malmuthuge N., Guan L. L. (2017). Understanding the gut microbiome of dairy calves: Opportunities to improve early-life gut health. J. Dairy Sci. 100, 5996–6005. doi: 10.3168/jds.2016-12239
Malmuthuge N., Liang G., Griebel P. J., Guan L. L. (2019). Taxonomic and functional compositions of the small intestinal microbiome in neonatal calves provide a framework for understanding early life gut health. Appl. Environ. Microbiol. 85, e02534-18. doi: 10.1128/AEM.02534-18
Ma T., Villot C., Renaud D., Skidmore A., Chevaux E., Steele M., et al. (2020). Linking perturbations to temporal changes in diversity, stability, and compositions of neonatal calf gut microbiota: Prediction of diarrhea. ISME J. 14, 2223–2235. doi: 10.1038/s41396-020-0678-3
Mayer M., Abenthum A., Matthes J. M., Kleeberger D., Ege M. J., Hölzel C., et al. (2012). Development and genetic influence of the rectal bacterial flora of newborn calves. Veterinary Microbiol. 161, 179–185. doi: 10.1016/j.vetmic.2012.07.023
O'Hara A. M., Shanahan F. (2006). The gut flora as a forgotten organ. EMBO Rep. 7, 688–693. doi: 10.1038/sj.embor.7400731
Oikonomou G., Teixeira A., Foditsch C., Bicalho M. L., Machado V. S., Bicalho R. C. (2013). Fecal microbial diversity in pre-weaned dairy calves as described by pyrosequencing of metagenomic 16S rDNA. associations of faecalibacterium species with health and growth. PloS One 8, e63157. doi: 10.1371/journal.pone.0063157
Rajilić-Stojanović M., De Vos W. M. (2014). The first 1000 cultured species of the human gastrointestinal microbiome. FEMS Microbiol. Rev. 38, 996–1047. doi: 10.1111/1574-6976.12075
Sakuragawa T., Hishiki T., Ueno Y., Ikeda S., Soga T., Yachie-Kinoshita A., et al. (2010). Hypotaurine is an energy-saving hepatoprotective compound against ischemia-reperfusion injury of the rat liver. J. Clin. Biochem. Nutr. 46, 126–134. doi: 10.3164/jcbn.09-91
Sommer F., Anderson J. M., Bharti R., Raes J., Rosenstiel P. (2017). The resilience of the intestinal microbiome influences health and disease. Nat. Rev. Microbiol. 15, 630–638. doi: 10.1038/nrmicro.2017.58
Tomás-Barberán F. A., Selma M. V., Espín J. C. (2016). Interactions of gut microbiome with dietary polyphenols and consequences to human health. Curr. Opin. Clin. Nutr. Metab. Care 19, 471–476. doi: 10.1097/MCO.0000000000000314
Uetake K. (2013). Newborn calf welfare: A review focusing on mortality rates. Anim. Sci. J. 84, 101–105. doi: 10.1111/asj.12019
USDA (2018). dairy 2014: Health and management proctices on U.S. dairy operation (Fort Collins, CO: USDA–APHIS–VS–CEAH–NAHMS).
Usuda K., Kawase T., Shigeno Y., Fukuzawa S., Fujii K., Zhang H., et al. (2018). Hippocampal metabolism of amino acids by l-amino acid oxidase is involved in fear learning and memory. Sci. Rep. 8, 11073. doi: 10.1038/s41598-018-28885-x
Yeoman C. J., White B. A. (2014). Gastrointestinal tract microbiome and probiotics in production animals. Annu. Rev. Anim. Biosci. 2, 469–486. doi: 10.1146/annurev-animal-022513-114149
Keywords: 16S rRNA, neonatal diarrhea, whole milk, milk replacer, dairy cows
Citation: Kojima M, Liu H, Takemoto S, Suda Y, Inoue R, Watanabe G, Jin W and Nagaoka K (2022) Temporal changes in the fecal microbiome and blood metabolites of early neonatal calves. Front. Anim. Sci. 3:934204. doi: 10.3389/fanim.2022.934204
Received: 02 May 2022; Accepted: 07 October 2022;
Published: 01 November 2022.
Edited by:
Georgios Oikonomou, University of Liverpool, United KingdomReviewed by:
Halima Sultana, University of Florida, United StatesFabio Abeni, Consiglio per la Ricerca in Agricoltura e l’Analisi dell’Economia Agraria (CREA), Italy
Copyright © 2022 Kojima, Liu, Takemoto, Suda, Inoue, Watanabe, Jin and Nagaoka. This is an open-access article distributed under the terms of the Creative Commons Attribution License (CC BY). The use, distribution or reproduction in other forums is permitted, provided the original author(s) and the copyright owner(s) are credited and that the original publication in this journal is cited, in accordance with accepted academic practice. No use, distribution or reproduction is permitted which does not comply with these terms.
*Correspondence: Kentaro Nagaoka, bmFnYW9rYWtAY2MudHVhdC5hYy5qcA==