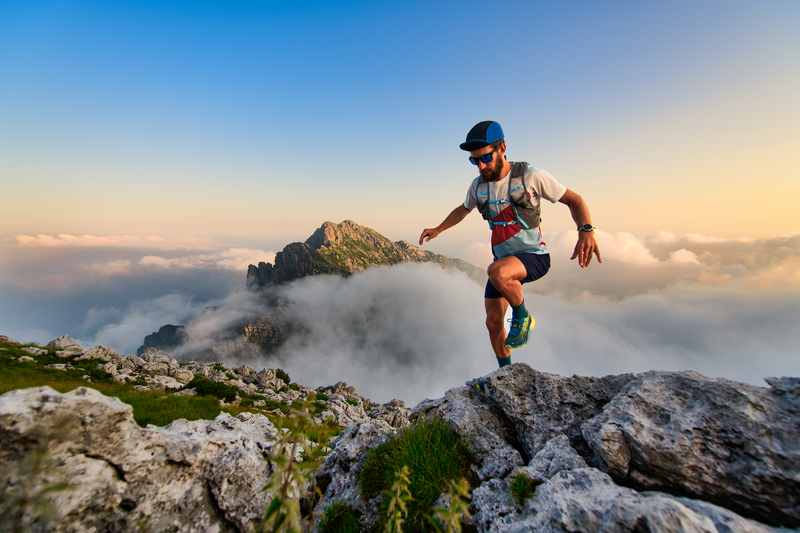
95% of researchers rate our articles as excellent or good
Learn more about the work of our research integrity team to safeguard the quality of each article we publish.
Find out more
MINI REVIEW article
Front. Allergy , 28 March 2025
Sec. Asthma
Volume 6 - 2025 | https://doi.org/10.3389/falgy.2025.1530122
Asthma is a heterogenous inflammatory bronchial disease involving complex mechanisms, several inflammatory pathways, and multiples cell-type networks. Bronchial inflammation associated to asthma is consecutive to multiple aggressions on epithelium, such as microbiologic, pollutant, and antigenic agents, which are responsible for both T2 and non-T2 inflammatory responses and further airway remodeling. Because asthma physiopathology involves multiple crosstalk between several cell types from different origins (epithelial, mesenchymal, and immune cells) and numerous cellular effectors, no single and/or representative in vitro model is suitable to study the overall of this disease. In this short review, we present and discuss the advantages and limitations of different in vitro models to decipher different aspects of virus-related asthma physiopathology and exacerbation.
Asthma affects more than 300 million individuals worldwide (1) and is characterized by airway obstruction, bronchial hyperresponsiveness, and bronchial inflammation. Asthma is schematically separated into two main immune and inflammatory-related phenotypes. The most prevalent is the T2-high phenotype, which involves allergic and/or eosinophilic inflammation, involving mainly Th2 lymphocytes, basophils, eosinophils, mast cells, and type 2 innate lymphoid cells (ILC2). By contrast, the T2-low inflammatory phenotype is driven by Th17 lymphocytes and/or neutrophils and is characterized by the absence of allergy, eosinophilic inflammation, and other T2 determinants, such as high FeNO levels, nasal polyposis, or atopic dermatitis (2). In some cases, when both neutrophil and eosinophil infiltration in the airways is low, the condition is called a mixed or pauci-granulocytic phenotype (3). A major burden of the disease is the occurrence of acute clinical events, known as asthma exacerbations, which are associated with an increase in bronchial inflammation in response to various environmental stimuli (4–6). Among these stimuli, allergens, pollutants, bacteria, and viruses are largely implicated and responsible for initiating the inflammatory cascade. They activate three main epithelial alarmins: thymic stromal lymphopoietin (TSLP), IL-33, and IL-25, which activate dendritic cells, ILC2, macrophages, T cells, and mast cells (7, 8). Respiratory viral infections are a leading cause of asthma exacerbation, with human rhinovirus (HRV) being the most commonly implicated, followed by respiratory syncytial virus (RSV) (9) and, to a lesser extent, human metapneumovirus (hMPV) and influenza viruses (9, 10). The virus-related mechanisms of asthma exacerbation are not yet fully understood and the development of relevant in vitro models to study virus/asthma functional relationships remains a significant challenge. In this short review, we summarize and discuss the advantages and limitations of the commonly used in vitro models for studying asthma and its exacerbation by viral infections.
The simplest models involve human bronchial cell lines cultured in medium or at the air–liquid interface (ALI). Some of these cell lines, such as CaLu-3 and 16HBE, express tight junction (TJ) proteins, which are key determinants of epithelium permeability and integrity (11, 12). Disrupted TJs are observed in the bronchial epithelium of asthmatic patients, significantly affecting respiratory airway barrier function (13). Studies using allergens, nanoparticles, or cigarette smoke extract have shown an increase in transepithelial permeability through the disruption of TJs on CaLu-3 (14, 15) and 16HBE (16, 17). Therefore, measuring TJ integrity and exploring functional interactions with respiratory viruses in permissive epithelial cells (Table 1) should be considered, especially as HRV, RSV, hMPV, influenza, and coronaviruses are known to target specific TJ proteins (18–21). However, although airway epithelial cell lines are more accessible than primary cells and can be cultured in ALI models to study host responses to viral infections (22), they are less physiologically relevant due to the lack of cell diversity (i.e., only ciliated cells are present, with no secretory or basal cells) (23, 24) and the absence of cell lines from asthmatic patients.
Primary bronchial epithelial cells (PBECs) are more relevant models, as they are directly recovered from patient biopsies, including those with asthma or other pulmonary diseases such as chronic obstructive pulmonary disease (COPD). PBECs can be cultured in submerged mono-cultures or in an air–liquid interface as a pseudostratified human airway epithelium (HAE) that includes several types of differentiated cells (ciliated, club, goblet, and basal) (37). These models allow for the investigation of a broader range of functional and physiological characteristics, including morphology, transepithelial electrical resistance, tight junction integrity, mucus secretion, cilia clearance, innate immune response (38, 39), sensitivity to allergens or respiratory viruses (Table 1), and transcriptomic signatures (40). As such, PBECs are widely used to study asthma physiopathology and exacerbation (41, 42). For example, the T2 associated IL-13 cytokine has been extensively studied for its ability to modulate gene expression profiles (43) and alter mucin production in primary cell-based ALI models (44), which are features of asthma physiopathology.
Moreover, HAE models are well-suited for investigating asthma exacerbation related to viral infection, as asthmatic patients exhibit physiological impairments that can be measured in HAE. These impairments include alterations in tight junctions and epithelium integrity (20, 21, 45), modifications in mucus production, mucociliary function (46–49), and aberrant epithelial tissue repair and remodeling through epithelial-mesenchymal transition (50–52).
Furthermore, PBECs can also react to viral infections through the innate immune response, which is an important factor in asthma exacerbation (8, 53, 54), as respiratory viruses are known to suppress antiviral responses and enhance inflammation in epithelial cells (55–57). For example, Wark et al. demonstrated an impairment of interferon beta (IFN-β) in ALI cultures from asthmatic patient PBECs compared to healthy controls during HRV infection (36). Consistently, the addition of IFN-β restored the expression of antiviral genes against HRV in asthmatic PBECs, thereby reducing the duration of inflammation (58). In addition, viral double-stranded RNA induces a stronger expression of the alarmin TSLP, an important enhancer of asthma exacerbation through the induction of the T2 inflammatory pathway, in asthmatic PBEC-based ALI compared to healthy controls (59). These results are similar to those obtained with HAE models infected by different respiratory viral infections, such as RSV (60), HRV (61), and SARS-CoV-2 (62).
Asthma is a complex lung disease that involves not only epithelial cells but also immune cells implicated in inflammation, viral clearance, and remodeling processes. In addition, mesenchymal cells, such as fibroblasts, endothelial cells, and smooth muscle cells, contribute to tissue inflammation and physiopathology (63). In this context, co-cultured primary cells of different origins can be very useful for investigating cellular crosstalk and their respective contributions to asthma development and exacerbation (Table 2). The ability of respiratory viruses to impair host immune responses, thereby increasing the risk of asthma exacerbation (64–67), underscores the need for further investigation into the functional relationships between epithelial, mesenchymal, and immune cells within integrated in vitro asthma models. In this regard, Transwell® cell separation system used for ALI cultures (68) allows for the co-cultivation of epithelial cells in the open air with other types of primary cells, whether adherent or not, on the lower surface of the Transwell®. Furthermore, co-culture models with direct contact between primary cells can be highly beneficial for mimicking in vivo crosstalk.
ALI co-culture models of healthy airway epithelium with fibroblast cells adhering to the lower surface of Transwell® have highlighted that chemical injury to the epithelium leads to the secretion of TGF-β2 by fibroblasts, which in turn triggers the expression of several asthma markers, such as Tenascin-C and α-smooth muscle actin (α-SMA) (69), as observed in the bronchus of asthmatic patients (81). In a similar model, Reeves et al. reported that fibroblasts secrete more hyaluronan when co-cultured with asthmatic ALI airway epithelium compared to healthy tissue, thereby promoting inflammation by increasing leukocyte binding. In this study, bronchial epithelial cells were co-cultured with human lung fibroblasts with Fibroblast Growth Media (FGM-2) and PneumaCult ALI for 96 h (82). Consistently, fibroblasts infected by RSV also secreted more hyaluronan, promoting mast cell binding and protease production, which are both known to contribute to asthma inflammation (83).
Co-culture models of epithelial cells with healthy smooth muscle cells in various culture media have been developed to study cell proliferation and pro-inflammatory responses (70), in line with their role in asthma physiopathology through bronchoconstriction and airway tissue remodeling (84). In addition, the monocyte migration chemokine CCL5 was reported to be significantly more expressed in asthmatic smooth muscle cells than in healthy ones when the ALI model was infected by HRV. This aligns with the increased risk of asthma exacerbation observed through monocyte migration in infected asthma patients (85). On the other hand, co-culturing asthmatic smooth muscle cells with bronchial epithelial cells increases their susceptibility to HRV infection compared to co-cultures with healthy smooth muscle cells, with an increased expression of CCL20 by smooth muscle cells altering the antiviral PKR/eIF2 pathway within epithelial cells (86).
Endothelial cells are also important for tissue inflammation by binding factors on the surface membrane that enhance leukocyte infiltration (87). A co-culture model involving epithelial 16HBE and endothelial HUVECs, stimulated with virus mimicking double-stranded RNA, showed an increased expression of leukocyte-recruiting adhesion molecules (E-selectin and ICAM-I) on endothelial cells. In this study, Blume et al. co-cultured human bronchial epithelial cells in minimum essential medium (MEM) with Glutamax and serum, and HUVEC cells in M199 medium supplemented with L-Glutamine seeded at the basal side, for up to 8 days (71). Similar results were obtained when healthy ALI cultures were infected with SARS-CoV-2 (88).
Finally, ALI co-culture models are not only suitable for studying crosstalk between epithelial and mesenchymal cells involved in bronchial inflammation and immune cell recruitment, but they are also essential for characterizing the epithelium’s sensibility to viral infections. This makes them highly interesting for deciphering virus-related mechanisms that lead to asthma exacerbation.
ALI airway models co-cultured with additional immune cell components are even more suitable for studying the mechanisms underlying asthma physiopathology. After initial epithelial cell injuries, immunes cells are recruited, activated, and amplify bronchial inflammatory cascades that can lead to asthma exacerbation and tissue remodeling. In this context, M1- or M2-type polarized macrophages contribute to determining the asthma phenotype (89) and exacerbation in cases where viral clearance is delayed (90).
Using Transwell® co-culture, Ronaghan et al. co-cultured human bronchial epithelial cells in ALI with macrophages in PneumaCult ALI medium or ImmunoCult-SF Macrophage Medium (IMM) for 72 h (72). They reported that M1 macrophage polarization reduced RSV infection in healthy PBECs ALI cultures, but not M2 polarization (72). These results are consistent with the predominance of M2 macrophages in asthmatic patients (91), which are associated with decreased in vivo interferon secretion and the promotion of T2 cytokines. This could help explain why asthmatics are more susceptible to RSV infection and related asthma exacerbation (92).
Other studies have developed more complex models by co-cultivating three different cell types. Paplinska-Goryca et al. used PBECs from healthy, asthmatic, or COPD patients in co-culture with macrophages on apical side and dendritic cells in the lower surface of Transwell®. This model highlighted the importance of immune cells and the origin of PBECs in the expression of alarmins when epithelium was exposed to double-stranded RNA stimulation (93). Similarly, Choe et al. showed the importance of eosinophils in airway epithelium remodeling and inflammation through a co-culture model of epithelial and fibroblast cells with eosinophils. Briefly, eosinophils, isolated from human venous blood, were seeded on top of the epithelium with RPMI 1640 medium. After 2 h of incubation and medium removal, eosinophils were stimulated with calcium ionophore for 48 h of co-culture (74). This co-culture model provides information about lung epithelium remodeling mechanisms in asthmatics (51).
Co-culturing peripheral blood mononuclear cells (PBMCs) at the lower surface of the Transwell® allows for the inclusion of a broader range of immune cells, enabling the study of crosstalk between epithelial cells and lymphocytes or natural killer (NK) cells. Several studies have focused on the T1/T2 balance and inflammatory cytokines, such as IP-10 and IFN-γ, during influenza and HRV infections of healthy nasal epithelium co-cultivated with PBMC in RPMI supplemented with serum for 48 h (75, 76). Qin et al. reported that RSV infection of healthy bronchial ALI cultures promotes an increase in Th2 cell populations from co-cultivated PBMC (77). Although NK cell polarization is known to be important for viral clearance and inflammation control through the secretion of IFN-γ or IL-4 and by killing eosinophils and neutrophils (94–96), their role in asthma physiopathology and exacerbation is not fully understood. To explore this further, co-culture models of human nasal epithelial cells with PBMC have been used to highlight NK cell activation after influenza infection (76). Future co-culture models with HAE and purified NK cells are needed to further study their role in asthma exacerbation during viral infections.
Organoids are three-dimensional structures derived from stem cells or induced pluripotent stem cells, which harbor some of the structural and functional characteristics of organs, including the bronchus, to study airway development and physiopathology (97–100). These organoids are spherical, with a lumen at the sphere’s center or epithelial cells exposed at the surface (101), allowing them to be permissive to respiratory viruses like RSV (102, 103), SARS-CoV-2 (102, 104, 105), HMPV (106), influenza (104), or human parainfluenza 3 virus (107). Studies using healthy organoids have focused on aspects of asthma pathology, including goblet cell metaplasia (108) and remodeling (109).
Organoids co-cultured with immune cells are also being developed to mimic functional cell–cell interactions. Various methods have been explored: (i) seeding immune cells and lung stem cells together, (ii) co-cultivation of organoids with isolated immune cells, (iii) injecting immune cells into the organoid lumens, and (iv) expanding cultures from tissue samples that include both epithelial and resident immunes cells (110). Seo et al. co-cultivated macrophages with organoids and stimulated them with lipopolysaccharide (LPS) to model alveolar inflammation. They showed an increase in pro-inflammatory protein expression in the presence of macrophages, supporting the relevance of such organoid models (79). Organoids with resident immune cells have also been used to study SARS-CoV-2 infection and the corresponding T lymphocyte response (80), making these models promising for studying the crosstalk between resident immune cells and lung tissue. However, the development of organoid models using cells from asthmatic patients is currently lacking whereas they would be useful to complement the ALI models to further decipher viral mediated mechanisms associated with this pathology.
Microfluidic systems, known as lung-on-chip models, are more complex systems that enable the movement of cells and media on the basal side, or the exposure of stimuli by injecting air onto the apical side of the airway (111, 112). For example, a lung-on-chip system with a co-culture of lung epithelium and circulating neutrophils in the basal medium was used to explore the mechanism behind asthma exacerbation induced by HRV infection. Briefly, the epithelium was cultivated in ALI on the surface of the chip membrane. On the other side, endothelial cells were cultivated in the vascular channel of the chip. After HRV inoculation at the apical side of the epithelium, neutrophils isolated from human peripheral blood were added to the chip’s reservoirs and perfused in RPMI 1640 (78). Studies have shown that neutrophil infiltration into the epithelium, a factor of neutrophilic asthma exacerbation, was induced by HRV infection and significantly increased with IL-13 stimulation (78). Another innovative system, known as “breathing lung-on-cheap,” mimics breathing and has been used to evaluate the effects of toxic particles potentially involved in the development and/or exacerbation of asthma (113). This model could be interesting when studying the impact of respiratory volume on asthmatic epithelium infection and asthma exacerbation. However, further development is needed to incorporate cells from patients.
In vitro 3D airway models offer significant advantages compared to monolayer cell line-based in vitro models (Figure 1). Airway epithelial functions, including relevant inflammatory and antiviral responses, are reconstituted with a large variety of primary cells from healthy individuals of different ages and/or from patients with various bronchial diseases, such as asthma or COPD. This makes ALI and organoid models valuable and complementary tools for studying the complex mechanisms involved in asthma and its exacerbations. However, further development of and characterization of co-culture models involving mesenchymal and immune cells are needed to better understand key aspects of asthma physiopathology, such as inflammation, immune cell polarization, and tissue remodeling, leading to the development of new therapeutic approaches.
In conclusion, ALI co-culture models, organoids, and lung-on-chip systems are highly effective tools for studying asthma and its exacerbation from viral infections. With a diverse range of models available, each offering unique strengths and limitations, the choice of the most suitable in vitro model will depend on its relevance and ability to complement others in revealing various aspects of virus-induced asthma pathophysiology and exacerbation.
RP: Writing – original draft, Writing – review & editing. CD: Writing – original draft, Writing – review & editing. GD: Writing – original draft, Writing – review & editing. MR-C: Writing – original draft, Writing – review & editing.
The author(s) declare that no financial support was received for the research and/or publication of this article.
The authors would like to thank Andrés Pizzorno for editing the language of this article.
The authors declare that the research was conducted in the absence of any commercial or financial relationships that could be construed as a potential conflict of interest.
The author(s) declare that no Generative AI was used in the creation of this manuscript.
All claims expressed in this article are solely those of the authors and do not necessarily represent those of their affiliated organizations, or those of the publisher, the editors and the reviewers. Any product that may be evaluated in this article, or claim that may be made by its manufacturer, is not guaranteed or endorsed by the publisher.
1. Brusselle GG, Koppelman GH. Biologic therapies for severe asthma. N Engl J Med. (2022) 386(2):157–71. doi: 10.1056/NEJMra2032506
2. Habib N, Pasha MA, Tang DD. Current understanding of asthma pathogenesis and biomarkers. Cells. (2022) 11(17):2764. doi: 10.3390/cells11172764
3. Liu T, Woodruff PG, Zhou X. Advances in non-type 2 severe asthma: from molecular insights to novel treatment strategies. Eur Respir J. (2024) 64(2):2300826. doi: 10.1183/13993003.00826-2023
4. Aghasafari P, George U, Pidaparti R. A review of inflammatory mechanism in airway diseases. Inflamm Res. (2019) 68(1):59–74. doi: 10.1007/s00011-018-1191-2
5. Calvén J, Ax E, Rådinger M. The airway epithelium—a central player in asthma pathogenesis. IJMS. (2020) 21(23):8907. doi: 10.3390/ijms21238907
6. Wang Y, Bai C, Li K, Adler KB, Wang X. Role of airway epithelial cells in development of asthma and allergic rhinitis. Respir Med. (2008) 102(7):949–55. doi: 10.1016/j.rmed.2008.01.017
7. Gauvreau GM, Sehmi R, Ambrose CS, Griffiths JM. Thymic stromal lymphopoietin: its role and potential as a therapeutic target in asthma. Expert Opin Ther Targets. (2020) 24(8):777–92. doi: 10.1080/14728222.2020.1783242
8. Jartti T, Bønnelykke K, Elenius V, Feleszko W. Role of viruses in asthma. Semin Immunopathol. (2020) 42(1):61–74. doi: 10.1007/s00281-020-00781-5
9. Papadopoulos NG, Christodoulou I, Rohde G, Agache I, Almqvist C, Bruno A, et al. Viruses and bacteria in acute asthma exacerbations – A GA 2 LEN-DARE* systematic review. Allergy. (2011 Apr) 66(4):458–68. doi: 10.1111/j.1398-9995.2010.02505.x
10. Williams JV, Crowe JE Jr, Enriquez R, Minton P, Peebles RS Jr, Hamilton RG, et al. Human metapneumovirus infection plays an etiologic role in acute asthma exacerbations requiring hospitalization in adults. J Infect Dis. (2005) 192(7):1149–53. doi: 10.1086/444392
11. Godbole NM, Chowdhury AA, Chataut N, Awasthi S. Tight junctions, the epithelial barrier, and toll-like receptor-4 during lung injury. Inflammation. (2022) 45(6):2142–62. doi: 10.1007/s10753-022-01708-y
12. Wittekindt OH. Tight junctions in pulmonary epithelia during lung inflammation. Pflugers Arch. (2017) 469(1):135–47. doi: 10.1007/s00424-016-1917-3
13. Heijink IH, Kuchibhotla VNS, Roffel MP, Maes T, Knight DA, Sayers I, et al. Epithelial cell dysfunction, a major driver of asthma development. Allergy. (2020) 75(8):1902–17. doi: 10.1111/all.14421
14. Banga A, Witzmann FA, Petrache HI, Blazer-Yost BL. Functional effects of nanoparticle exposure on calu-3 airway epithelial cells. Cell Physiol Biochem. (2012) 29(1–2):197–212. doi: 10.1159/000337601
15. Vinhas R, Cortes L, Cardoso I, Mendes VM, Manadas B, Todo-Bom A, et al. Pollen proteases compromise the airway epithelial barrier through degradation of transmembrane adhesion proteins and lung bioactive peptides: role of pollen proteases on allergic disorders. Allergy. (2011) 66(8):1088–98. doi: 10.1111/j.1398-9995.2011.02598.x
16. Gangl K, Reininger R, Bernhard D, Campana R, Pree I, Reisinger J, et al. Cigarette smoke facilitates allergen penetration across respiratory epithelium. Allergy. (2009) 64(3):398–405. doi: 10.1111/j.1398-9995.2008.01861.x
17. Heijink IH, Brandenburg SM, Postma DS, Van Oosterhout AJM. Cigarette smoke impairs airway epithelial barrier function and cell-cell contact recovery. Eur Respir J. (2012) 39(2):419–28. doi: 10.1183/09031936.00193810
18. Pharo EA, Williams SM, Boyd V, Sundaramoorthy V, Durr PA, Baker ML. Host–pathogen responses to pandemic influenza H1N1pdm09 in a human respiratory airway model. Viruses. (2020) 12(6):679. doi: 10.3390/v12060679
19. Rezaee F, Harford TJ, Linfield DT, Altawallbeh G, Midura RJ, Ivanov AI, et al. cAMP-dependent activation of protein kinase A attenuates respiratory syncytial virus-induced human airway epithelial barrier disruption. PLoS One. (2017) 12(7):e0181876. doi: 10.1371/journal.pone.0181876
20. Short KR, Kasper J, Van Der Aa S, Andeweg AC, Zaaraoui-Boutahar F, Goeijenbier M, et al. Influenza virus damages the alveolar barrier by disrupting epithelial cell tight junctions. Eur Respir J. (2016) 47(3):954–66. doi: 10.1183/13993003.01282-2015
21. Teoh KT, Siu YL, Chan WL, Schlüter MA, Liu CJ, Peiris JSM, et al. The SARS coronavirus E protein interacts with PALS1 and alters tight junction formation and epithelial morphogenesis. MBoC. (2010) 21(22):3838–52. doi: 10.1091/mbc.e10-04-0338
22. Harcourt JL, Caidi H, Anderson LJ, Haynes LM. Evaluation of the calu-3 cell line as a model of in vitro respiratory syncytial virus infection. J Virol Methods. (2011) 174(1–2):144–9. doi: 10.1016/j.jviromet.2011.03.027
23. Grainger CI, Greenwell LL, Lockley DJ, Martin GP, Forbes B. Culture of calu-3 cells at the air interface provides a representative model of the airway epithelial barrier. Pharm Res. (2006) 23(7):1482–90. doi: 10.1007/s11095-006-0255-0
24. Kreft ME, Jerman UD, Lasič E, Hevir-Kene N, Rižner TL, Peternel L, et al. The characterization of the human cell line calu-3 under different culture conditions and its use as an optimized in vitro model to investigate bronchial epithelial function. Eur J Pharm Sci. (2015) 69:1–9. doi: 10.1016/j.ejps.2014.12.017
25. Böttcher-Friebertshäuser E, Stein DA, Klenk HD, Garten W. Inhibition of influenza virus infection in human airway cell cultures by an antisense peptide-conjugated morpholino oligomer targeting the hemagglutinin-activating protease TMPRSS2. J Virol. (2011) 85(4):1554–62. doi: 10.1128/JVI.01294-10
26. Kawase M, Shirato K, Van Der Hoek L, Taguchi F, Matsuyama S. Simultaneous treatment of human bronchial epithelial cells with serine and cysteine protease inhibitors prevents severe acute respiratory syndrome coronavirus entry. J Virol. (2012) 86(12):6537–45. doi: 10.1128/JVI.00094-12
27. Rajan D, McCracken CE, Kopleman HB, Kyu SY, Lee FEH, Lu X, et al. Human rhinovirus induced cytokine/chemokine responses in human airway epithelial and immune cells. PLoS One. (2014) 9(12):e114322. doi: 10.1371/journal.pone.0114322
28. Comstock AT, Ganesan S, Chattoraj A, Faris AN, Margolis BL, Hershenson MB, et al. Rhinovirus-Induced barrier dysfunction in polarized airway epithelial cells is mediated by NADPH oxidase 1. J Virol. (2011) 85(13):6795–808. doi: 10.1128/JVI.02074-10
29. Najjar F E, Cifuentes-Muñoz N, Chen J, Zhu H, Buchholz UJ, Moncman CL, et al. Human metapneumovirus induces reorganization of the actin cytoskeleton for direct cell-to-cell spread. PLoS Pathog. (2016) 12(9):e1005922. doi: 10.1371/journal.ppat.1005922
30. Kong M, Whitley R, Peng N, Oster R, Schoeb T, Sullender W, et al. Matrix metalloproteinase-9 mediates RSV infection in vitro and in vivo. Viruses. (2015) 7(8):4230–53. doi: 10.3390/v7082817
31. Liao Y, Li X, Mou T, Zhou X, Li D, Wang L, et al. Distinct infection process of SARS-CoV-2 in human bronchial epithelial cell lines. J Med Virol. (2020) 92(11):2830–8. doi: 10.1002/jmv.26200
32. Aguiar JA, Tremblay BJM, Mansfield MJ, Woody O, Lobb B, Banerjee A, et al. Gene expression and in situ protein profiling of candidate SARS-CoV-2 receptors in human airway epithelial cells and lung tissue. Eur Respir J. (2020) 56(3):2001123. doi: 10.1183/13993003.01123-2020
33. Bao X, Liu T, Spetch L, Kolli D, Garofalo RP, Casola A. Airway epithelial cell response to human metapneumovirus infection. Virology. (2007) 368(1):91–101. doi: 10.1016/j.virol.2007.06.023
34. Chan M, Cheung C, Chui W, Tsao S, Nicholls J, Chan Y, et al. Proinflammatory cytokine responses induced by influenza A (H5N1) viruses in primary human alveolar and bronchial epithelial cells. Respir Res. (2005) 6(1):135. doi: 10.1186/1465-9921-6-135
35. Villenave R, Thavagnanam S, Sarlang S, Parker J, Douglas I, Skibinski G, et al. In vitro modeling of respiratory syncytial virus infection of pediatric bronchial epithelium, the primary target of infection in vivo. Proc Natl Acad Sci USA. (2012) 109(13):5040–5. doi: 10.1073/pnas.1110203109
36. Wark PAB, Johnston SL, Bucchieri F, Powell R, Puddicombe S, Laza-Stanca V, et al. Asthmatic bronchial epithelial cells have a deficient innate immune response to infection with rhinovirus. J Exp Med. (2005) 201(6):937–47. doi: 10.1084/jem.20041901
37. Cao X, Coyle JP, Xiong R, Wang Y, Heflich RH, Ren B, et al. Invited review: human air-liquid-interface organotypic airway tissue models derived from primary tracheobronchial epithelial cells—overview and perspectives. In Vitro Cell Dev Biol Animal. (2021) 57(2):104–32. doi: 10.1007/s11626-020-00517-7
38. Müller L, Brighton LE, Carson JL, Fischer Ii WA, Jaspers I. Culturing of human nasal epithelial cells at the air liquid interface. J Vis Exp. (2013) 80:50646. doi: 10.3791/50646
39. Rayner RE, Makena P, Prasad GL, Cormet-Boyaka E. Optimization of normal human bronchial epithelial (NHBE) cell 3D cultures for in vitro lung model studies. Sci Rep. (2019) 9(1):500. doi: 10.1038/s41598-018-36735-z
40. Pezzulo AA, Starner TD, Scheetz TE, Traver GL, Tilley AE, Harvey BG, et al. The air-liquid interface and use of primary cell cultures are important to recapitulate the transcriptional profile of in vivo airway epithelia. Am J Physiol Lung Cell Mol Physiol. (2011) 300(1):L25–31. doi: 10.1152/ajplung.00256.2010
41. Chortarea S, Barosova H, Clift MJD, Wick P, Petri-Fink A, Rothen-Rutishauser B. Human asthmatic bronchial cells are more susceptible to subchronic repeated exposures of aerosolized carbon nanotubes at occupationally relevant doses than healthy cells. ACS Nano. (2017) 11(8):7615–25. doi: 10.1021/acsnano.7b01992
42. Kooter I, Ilves M, Gröllers-Mulderij M, Duistermaat E, Tromp PC, Kuper F, et al. Molecular signature of asthma-enhanced sensitivity to CuO nanoparticle aerosols from 3D cell model. ACS Nano. (2019) 13(6):6932–46. doi: 10.1021/acsnano.9b01823
43. Schleimer RP, Kato A, Kern R, Kuperman D, Avila PC. Epithelium: at the interface of innate and adaptive immune responses. J Allergy Clin Immunol. (2007) 120(6):1279–84. doi: 10.1016/j.jaci.2007.08.046
44. Zhen G, Park SW, Nguyenvu LT, Rodriguez MW, Barbeau R, Paquet AC, et al. IL-13 and epidermal growth factor receptor have critical but distinct roles in epithelial cell mucin production. Am J Respir Cell Mol Biol. (2007) 36(2):244–53. doi: 10.1165/rcmb.2006-0180OC
45. Xiao C, Puddicombe SM, Field S, Haywood J, Broughton-Head V, Puxeddu I, et al. Defective epithelial barrier function in asthma. J Allergy Clin Immunol. (2011) 128(3):549–556.e12. doi: 10.1016/j.jaci.2011.05.038
46. Dunnill MS. The pathology of asthma, with special reference to changes in the bronchial Mucosa. J Clin Pathol. (1960) 13(1):27–33. doi: 10.1136/jcp.13.1.27
47. Gaillard D, Jouet JB, Egreteau L, Plotkowski L, Zahm JM, Benali R, et al. Airway epithelial damage and inflammation in children with recurrent bronchitis. Am J Respir Crit Care Med. (1994) 150(3):810–7. doi: 10.1164/ajrccm.150.3.8087356
48. Laitinen LA, Heino M, Laitinen A, Kava T, Haahtela T. Damage of the airway epithelium and bronchial reactivity in patients with asthma. Am Rev Respir Dis. (1985) 131(4):599–606. doi: 10.1164/arrd.1985.131.4.599
49. Look DC, Walter MJ, Williamson MR, Pang L, You Y, Sreshta JN, et al. Effects of paramyxoviral infection on airway epithelial cell Foxj1 expression, ciliogenesis, and mucociliary function. Am J Pathol. (2001) 159(6):2055–69. doi: 10.1016/S0002-9440(10)63057-X
50. Davies DE. The role of the epithelium in airway remodeling in asthma. Proc Am Thorac Soc. (2009) 6(8):678–82. doi: 10.1513/pats.200907-067DP
51. Hough KP, Curtiss ML, Blain TJ, Liu RM, Trevor J, Deshane JS, et al. Airway remodeling in asthma. Front Med. (2020) 7:191. doi: 10.3389/fmed.2020.00191
52. Pain M, Bermudez O, Lacoste P, Royer PJ, Botturi K, Tissot A, et al. Tissue remodelling in chronic bronchial diseases: from the epithelial to mesenchymal phenotype. Eur Respir Rev. (2014) 23(131):118–30. doi: 10.1183/09059180.00004413
53. Cerato JA, Da Silva EF, Porto BN. Breaking bad: inflammasome activation by respiratory viruses. Biology (Basel). (2023) 12(7):943. doi: 10.3390/biology12070943
54. Oliver BGG, Robinson P, Peters M, Black J. Viral infections and asthma: an inflammatory interface? Eur Respir J. (2014) 44(6):1666–81. doi: 10.1183/09031936.00047714
55. Gambadauro A, Galletta F, Li Pomi A, Manti S, Piedimonte G. Immune response to respiratory viral infections. IJMS. (2024) 25(11):6178. doi: 10.3390/ijms25116178
56. Kikkert M. Innate immune evasion by human respiratory RNA viruses. J Innate Immun. (2020) 12(1):4–20. doi: 10.1159/000503030
57. Newton AH, Cardani A, Braciale TJ. The host immune response in respiratory virus infection: balancing virus clearance and immunopathology. Semin Immunopathol. (2016 Jul) 38(4):471–82. doi: 10.1007/s00281-016-0558-0
58. Cakebread JA, Xu Y, Grainge C, Kehagia V, Howarth PH, Holgate ST, et al. Exogenous IFN-β has antiviral and anti-inflammatory properties in primary bronchial epithelial cells from asthmatic subjects exposed to rhinovirus. J Allergy Clin Immunol. (2011) 127(5):1148–1154.e9. doi: 10.1016/j.jaci.2011.01.023
59. Uller L, Leino M, Bedke N, Sammut D, Green B, Lau L, et al. Double-stranded RNA induces disproportionate expression of thymic stromal lymphopoietin versus interferon- in bronchial epithelial cells from donors with asthma. Thorax. (2010) 65(7):626–32. doi: 10.1136/thx.2009.125930
60. Lee HC, Headley MB, Loo YM, Berlin A, Gale M, Debley JS, et al. Thymic stromal lymphopoietin is induced by respiratory syncytial virus–infected airway epithelial cells and promotes a type 2 response to infection. J Allergy Clin Immunol. (2012) 130(5):1187–1196.e5. doi: 10.1016/j.jaci.2012.07.031
61. Williams TC, Loo SL, Nichol KS, Reid AT, Veerati PC, Esneau C, et al. IL-25 blockade augments antiviral immunity during respiratory virus infection. Commun Biol. (2022) 5(1):415. doi: 10.1038/s42003-022-03367-z
62. Gerla L, Moitra S, Pink D, Govindasamy N, Duchesne M, Reklow E, et al. SARS-CoV-2-Induced TSLP is associated with duration of hospital stay in COVID-19 patients. Viruses. (2023) 15(2):556. doi: 10.3390/v15020556
63. Osei ET, Booth S, Hackett TL. What have in vitro co-culture models taught US about the contribution of epithelial-mesenchymal interactions to airway inflammation and remodeling in asthma? Cells. (2020) 9(7):1694. doi: 10.3390/cells9071694
64. Beale J, Jayaraman A, Jackson DJ, Macintyre JDR, Edwards MR, Walton RP, et al. Rhinovirus-induced IL-25 in asthma exacerbation drives type 2 immunity and allergic pulmonary inflammation. Sci Transl Med. (2014) 6(256):256ra134. doi: 10.1126/scitranslmed.3009124
65. Durrani SR, Montville DJ, Pratt AS, Sahu S, DeVries MK, Rajamanickam V, et al. Innate immune responses to rhinovirus are reduced by the high-affinity IgE receptor in allergic asthmatic children. J Allergy Clin Immunol. (2012) 130(2):489–95. doi: 10.1016/j.jaci.2012.05.023
66. Jackson DJ, Makrinioti H, Rana BMJ, Shamji BWH, Trujillo-Torralbo MB, Footitt J, et al. IL-33–Dependent type 2 inflammation during rhinovirus-induced asthma exacerbations In Vivo. Am J Respir Crit Care Med. (2014) 190(12):1373–82. doi: 10.1164/rccm.201406-1039OC
67. Jackson DJ, Johnston SL. The role of viruses in acute exacerbations of asthma. J Allergy Clin Immunol. (2010) 125(6):1178–87. doi: 10.1016/j.jaci.2010.04.021
68. Lee DDH, Petris A, Hynds RE, O’Callaghan C. Ciliated epithelial cell differentiation at air-liquid interface using commercially available culture Media. In: Turksen K, editor. Epidermal Cells. New York, NY: Springer US (2019). p. 275–91. (Methods in Molecular Biology; vol. 2109). Available online at: http://link.springer.com/10.1007/7651_2019_269 (cited September 30, 2024).
69. Thompson HGR, Mih JD, Krasieva TB, Tromberg BJ, George SC. Epithelial-derived TGF-β2 modulates basal and wound-healing subepithelial matrix homeostasis. Am J Physiol Lung Cell Mol Physiol. (2006) 291(6):L1277–85. doi: 10.1152/ajplung.00057.2006
70. O’Sullivan MJ, Jang JH, Panariti A, Bedrat A, Ijpma G, Lemos B, et al. Airway epithelial cells drive airway smooth muscle cell phenotype switching to the proliferative and pro-inflammatory phenotype. Front Physiol. (2021) 12:687654. doi: 10.3389/fphys.2021.687654
71. Blume C, Reale R, Held M, Loxham M, Millar TM, Collins JE, et al. Cellular crosstalk between airway epithelial and endothelial cells regulates barrier functions during exposure to double-stranded RNA: epithelial-endothelial crosstalk in human airways. ImmunInflamm Dis. (2017) 5(1):45–56. doi: 10.1002/iid3.139
72. Ronaghan NJ, Soo M, Pena U, Tellis M, Duan W, Tabatabaei-Zavareh N, et al. M1-like, but not M0- or M2-like, macrophages, reduce RSV infection of primary bronchial epithelial cells in a media-dependent fashion. PLoS One. (2022) 17(10):e0276013. doi: 10.1371/journal.pone.0276013
73. Paplinska-Goryca M, Misiukiewicz-Stepien P, Proboszcz M, Nejman-Gryz P, Gorska K, Zajusz-Zubek E, et al. Interactions of nasal epithelium with macrophages and dendritic cells variously alter urban PM-induced inflammation in healthy, asthma and COPD. Sci Rep. (2021) 11(1):13259. doi: 10.1038/s41598-021-92626-w
74. Choe MM, Sporn PHS, Swartz MA. An in vitro airway wall model of remodeling. Am J Physiol Lung Cell Mol Physiol. (2003) 285(2):L427–33. doi: 10.1152/ajplung.00005.2003
75. Jornot L, Cordey S, Caruso A, Gerber C, Vukicevic M, Tapparel C, et al. T lymphocytes promote the antiviral and inflammatory responses of airway epithelial cells. PLoS One. (2011) 6(10):e26293. doi: 10.1371/journal.pone.0026293
76. Luukkainen A, Puan KJ, Yusof N, Lee B, Tan KS, Liu J, et al. A co-culture model of PBMC and stem cell derived human nasal epithelium reveals rapid activation of NK and innate T cells upon influenza a virus infection of the nasal epithelium. Front Immunol. (2018) 9:2514. doi: 10.3389/fimmu.2018.02514
77. Qin L, Hu Cp, Feng Jt, Xia Q. Activation of lymphocytes induced by bronchial epithelial cells with prolonged RSV infection. PLoS One. (2011) 6(12):e27113. doi: 10.1371/journal.pone.0027113
78. Nawroth JC, Lucchesi C, Cheng D, Shukla A, Ngyuen J, Shroff T, et al. A microengineered airway lung chip models key features of viral-induced exacerbation of asthma. Am J Respir Cell Mol Biol. (2020) 63(5):591–600. doi: 10.1165/rcmb.2020-0010MA
79. Seo HR, Han HJ, Lee Y, Noh YW, Cho SJ, Kim JH. Human pluripotent stem cell-derived alveolar organoid with macrophages. IJMS. (2022) 23(16):9211. doi: 10.3390/ijms23169211
80. Kuo C, Choi S, Unen VV, Zhang H, Rustagi A, Alwahabi S, et al. Organoid modeling of lung-resident immune responses to SARS-CoV-2 infection. (2023). Available online at: https://www.researchsquare.com/article/rs-2870695/v1 (cited July 2, 2024).
81. Boser SR, Mauad T, Araújo-Paulino BBD, Mitchell I, Shrestha G, Chiu A, et al. Myofibroblasts are increased in the lung parenchyma in asthma. PLoS One. (2017) 12(8):e0182378. doi: 10.1371/journal.pone.0182378
82. Reeves SR, Kang I, Chan CK, Barrow KA, Kolstad TK, White MP, et al. Asthmatic bronchial epithelial cells promote the establishment of a hyaluronan-enriched, leukocyte-adhesive extracellular matrix by lung fibroblasts. Respir Res. (2018) 19(1):146. doi: 10.1186/s12931-018-0849-1
83. Reeves SR, Barrow KA, Rich LM, White MP, Shubin NJ, Chan CK, et al. Respiratory syncytial virus infection of human lung fibroblasts induces a hyaluronan-enriched extracellular matrix that binds mast cells and enhances expression of mast cell proteases. Front Immunol. (2020) 10:3159. doi: 10.3389/fimmu.2019.03159
84. Doeing DC, Solway J. Airway smooth muscle in the pathophysiology and treatment of asthma. J Appl Physiol. (2013) 114(7):834–43. doi: 10.1152/japplphysiol.00950.2012
85. Allard B, Levardon H, Esteves P, Celle A, Maurat E, Thumerel M, et al. Asthmatic bronchial smooth muscle increases CCL5-dependent monocyte migration in response to rhinovirus-infected epithelium. Front Immunol. (2020) 10:2998. doi: 10.3389/fimmu.2019.02998
86. Esteves P, Allard B, Celle A, Dupin I, Maurat E, Ousova O, et al. Asthmatic bronchial smooth muscle increases rhinovirus replication within the bronchial epithelium. Cell Rep. (2022) 38(13):110571. doi: 10.1016/j.celrep.2022.110571
87. Green CE, Turner AM. The role of the endothelium in asthma and chronic obstructive pulmonary disease (COPD). Respir Res. (2017) 18(1):20. doi: 10.1186/s12931-017-0505-1
88. Bordoni V, Mariotti D, Matusali G, Colavita F, Cimini E, Ippolito G, et al. SARS-CoV-2 infection of airway epithelium triggers pulmonary endothelial cell activation and senescence associated with type I IFN production. Cells. (2022) 11(18):2912. doi: 10.3390/cells11182912
89. Van Der Veen TA, De Groot LES, Melgert BN. The different faces of the macrophage in asthma. Curr Opin Pulm Med. (2020) 26(1):62–8. doi: 10.1097/MCP.0000000000000647
90. Message SD, Johnston SL. The immunology of virus infection in asthma. Eur Respir J. (2001) 18(6):1013–25. doi: 10.1183/09031936.01.00228701
91. Draijer C, Boorsma CE, Robbe P, Timens W, Hylkema MN, Ten Hacken NH, et al. Human asthma is characterized by more IRF5+ M1 and CD206+ M2 macrophages and less IL-10+ M2-like macrophages around airways compared with healthy airways. J Allergy Clin Immunol. (2017 Jul) 140(1):280–283.e3. doi: 10.1016/j.jaci.2016.11.020
92. Zdrenghea MT, Makrinioti H, Muresan A, Johnston SL, Stanciu LA. The role of macrophage IL-10/innate IFN interplay during virus-induced asthma. Rev Med Virol. (2015) 25(1):33–49. doi: 10.1002/rmv.1817
93. Paplinska-Goryca M, Misiukiewicz-Stepien P, Nejman-Gryz P, Proboszcz M, Mlacki M, Gorska K, et al. Epithelial-macrophage-dendritic cell interactions impact alarmins expression in asthma and COPD. Clin Immunol. (2020) 215:108421. doi: 10.1016/j.clim.2020.108421
94. Gorska MM. Natural killer cells in asthma. Curr Opin Aller Clin Immunol. (2017) 17(1):50–4. doi: 10.1097/ACI.0000000000000327
95. Lepretre F, Gras D, Chanez P, Duez C. Natural killer cells in the lung: potential role in asthma and virus-induced exacerbation? Eur Respir Rev. (2023) 32(169):230036. doi: 10.1183/16000617.0036-2023
96. Wei H, Zhang J, Xiao W, Feng J, Sun R, Tian Z. Involvement of human natural killer cells in asthma pathogenesis: natural killer 2 cells in type 2 cytokine predominance. J Allergy Clin Immunol. (2005) 115(4):841–7. doi: 10.1016/j.jaci.2004.11.026
97. Chen YW, Huang SX, De Carvalho ALRT, Ho SH, Islam MN, Volpi S, et al. A three-dimensional model of human lung development and disease from pluripotent stem cells. Nat Cell Biol. (2017) 19(5):542–9. doi: 10.1038/ncb3510
98. Gkatzis K, Taghizadeh S, Huh D, Stainier DYR, Bellusci S. Use of three-dimensional organoids and lung-on-a-chip methods to study lung development, regeneration and disease. Eur Respir J. (2018) 52(5):1800876. doi: 10.1183/13993003.00876-2018
99. Kong J, Wen S, Cao W, Yue P, Xu X, Zhang Y, et al. Lung organoids, useful tools for investigating epithelial repair after lung injury. Stem Cell Res Ther. (2021) 12(1):95. doi: 10.1186/s13287-021-02172-5
100. Li Y, Wu Q, Sun X, Shen J, Chen H. Organoids as a powerful model for respiratory diseases. Stem Cells Int. (2020) 2020:1–8. doi: 10.1155/2020/5847876
101. Stroulios G, Brown T, Moreni G, Kondro D, Dei A, Eaves A, et al. Apical-out airway organoids as a platform for studying viral infections and screening for antiviral drugs. Sci Rep. (2022) 12(1):7673. doi: 10.1038/s41598-022-11700-z
102. Rajan A, Weaver AM, Aloisio GM, Jelinski J, Johnson HL, Venable SF, et al. The human nose organoid respiratory virus model: an Ex Vivo human challenge model to study respiratory syncytial virus (RSV) and severe acute respiratory syndrome coronavirus 2 (SARS-CoV-2) pathogenesis and evaluate therapeutics. mBio. (2022) 13(1):e03511–21. doi: 10.1128/mbio.03511-21
103. Rijsbergen LC, Lamers MM, Comvalius AD, Koutstaal RW, Schipper D, Duprex WP, et al. Human respiratory syncytial virus subgroup A and B infections in nasal, bronchial, small-airway, and organoid-derived respiratory cultures. mSphere. (2021) 6(3):e00237–21. doi: 10.1128/mSphere.00237-21
104. Ekanger CT, Zhou F, Bohan D, Lotsberg ML, Ramnefjell M, Hoareau L, et al. Human organotypic airway and lung organoid cells of bronchiolar and alveolar differentiation are permissive to infection by influenza and SARS-CoV-2 respiratory virus. Front Cell Infect Microbiol. (2022) 12:841447. doi: 10.3389/fcimb.2022.841447
105. Lamers MM, Van Der Vaart J, Knoops K, Riesebosch S, Breugem TI, Mykytyn AZ, et al. An organoid-derived bronchioalveolar model for SARS-CoV-2 infection of human alveolar type II-like cells. EMBO J. (2021) 40(5):e105912. doi: 10.15252/embj.2020105912
106. Ribó-Molina P, Van Nieuwkoop S, Mykytyn AZ, Van Run P, Lamers MM, Haagmans BL, et al. Human metapneumovirus infection of organoid-derived human bronchial epithelium represents cell tropism and cytopathology as observed in in vivo models. mSphere. (2024) 9(2):e00743–23. doi: 10.1128/msphere.00743-23
107. Porotto M, Ferren M, Chen YW, Siu Y, Makhsous N, Rima B, et al. Authentic modeling of human respiratory virus infection in human pluripotent stem cell-derived lung organoids. mBio. (2019) 10(3):e00723–19. doi: 10.1128/mBio.00723-19
108. Danahay H, Pessotti AD, Coote J, Montgomery BE, Xia D, Wilson A, et al. Notch2 is required for inflammatory cytokine-driven goblet cell metaplasia in the lung. Cell Rep. (2015) 10(2):239–52. doi: 10.1016/j.celrep.2014.12.017
109. Pat Y, Rückert B, Ogulur I, Yazici D, Pérez-Diego M, Küçükkase OC, et al. Differentiation of bronchial epithelial spheroids in the presence of IL-13 recapitulates characteristic features of asthmatic airway epithelia. Allergy. (2022) 77(7):2229–33. doi: 10.1111/all.15279
110. Bogoslowski A, An M, Penninger JM. Incorporating immune cells into organoid models: essential for studying human disease. Organoids. (2023) 2(3):140–55. doi: 10.3390/organoids2030011
111. Ding S, Zhang H, Wang X. Microfluidic-Chip-Integrated biosensors for lung disease models. Biosensors. (2021) 11(11):456. doi: 10.3390/bios11110456
112. Wang H, Yin F, Li Z, Su W, Li D. Advances of microfluidic lung chips for assessing atmospheric pollutants exposure. Environ Int. (2023) 172:107801. doi: 10.1016/j.envint.2023.107801
Keywords: asthma, viral infection, in vitro model, exacerbation, physiopathology
Citation: Pereira De Oliveira R, Droillard C, Devouassoux G and Rosa-Calatrava M (2025) In vitro models to study viral-induced asthma exacerbation: a short review for a key issue. Front. Allergy 6:1530122. doi: 10.3389/falgy.2025.1530122
Received: 18 November 2024; Accepted: 27 February 2025;
Published: 28 March 2025.
Edited by:
Remo Castro Russo, Federal University of Minas Gerais, BrazilReviewed by:
Ravi Lokwani, Chapman University, United StatesCopyright: © 2025 Pereira De Oliveira, Droillard, Devouassoux and Rosa-Calatrava. This is an open-access article distributed under the terms of the Creative Commons Attribution License (CC BY). The use, distribution or reproduction in other forums is permitted, provided the original author(s) and the copyright owner(s) are credited and that the original publication in this journal is cited, in accordance with accepted academic practice. No use, distribution or reproduction is permitted which does not comply with these terms.
*Correspondence: Rémi Pereira De Oliveira, cmVtaS5wZXJlaXJhLWRlLW9saXZlaXJhQHVuaXYtbHlvbjEuZnI=
Disclaimer: All claims expressed in this article are solely those of the authors and do not necessarily represent those of their affiliated organizations, or those of the publisher, the editors and the reviewers. Any product that may be evaluated in this article or claim that may be made by its manufacturer is not guaranteed or endorsed by the publisher.
Research integrity at Frontiers
Learn more about the work of our research integrity team to safeguard the quality of each article we publish.