- 1Department of Microbiology and Molecular Biology, Brigham Young University, Provo, UT, United States
- 2College of Dental Medicine, Roseman University of Health Sciences, South Jordan, UT, United States
In recent years, the interplay between oral microbiota and systemic disease has gained attention as poor oral health is associated with several pathologies. The oral microbiota plays a role in the maintenance of overall health, and its dysbiosis influences chronic inflammation and the pathogenesis of gum diseases. Periodontitis has also been associated with other diseases and health complications such as cancer, neurogenerative and autoimmune disorders, chronic kidney disease, cardiovascular diseases, rheumatic arthritis, respiratory health, and adverse pregnancy outcomes. The host microbiota can influence immune cell development and immune responses, and recent evidence suggests that changes in oral microbiota composition may also contribute to sensitization and the development of allergic reactions, including asthma and peanut allergies. Conversely, there is also evidence that allergic reactions within the gut may contribute to alterations in oral microbiota composition. Here we review the current evidence of the role of the oral microbiota in inflammatory diseases and health complications, as well as its future relevance in improving health and ameliorating allergic disease.
Introduction
The human microbiota is comprised of all the microbes (prokaryotes, archaea, and viruses) within the body, and it plays a homeostatic role in the development of a healthy immune system. Microbiota interact with host innate and adaptive immune cells in a way that facilitates immune training and tolerance towards pathogenic and commensal bacteria (Figure 1) (1). Additionally, interactions between microbiota and the host immune system are necessary for the proper development of immune cell populations and lymphoid organ development as demonstrated by early studies using germ-free (GF) mice (1–4). For example, population levels of adaptive immune cells including αβ and γδ intraepithelial lymphocytes (IEL), T regulatory (Treg) cells, and T helper 17 cells (Th17) are significantly reduced in the intestines of GF mice (2, 3, 5). The absence of microbiota within the intestines of GF mice also leads to reduced IgA antibody levels (6). Impairment of lymphoid tissue structure development and pathologies within the thymus of GF mice have also been observed (4, 7). Immune cell populations and IgA levels within the gut can be rescued by de novo microbial colonization (2, 6). The entire gastrointestinal (GI) tract is in constant contact with various microbes, most of which enter through the mouth (8). The oral cavity is home to a diverse community of microorganisms including bacteria, fungi, protozoa, and viruses (9, 10). Approximately 700 bacterial species have been identified within the oral cavity of humans using molecular analysis, and individuals typically contain approximately 100–200 species (9–11).
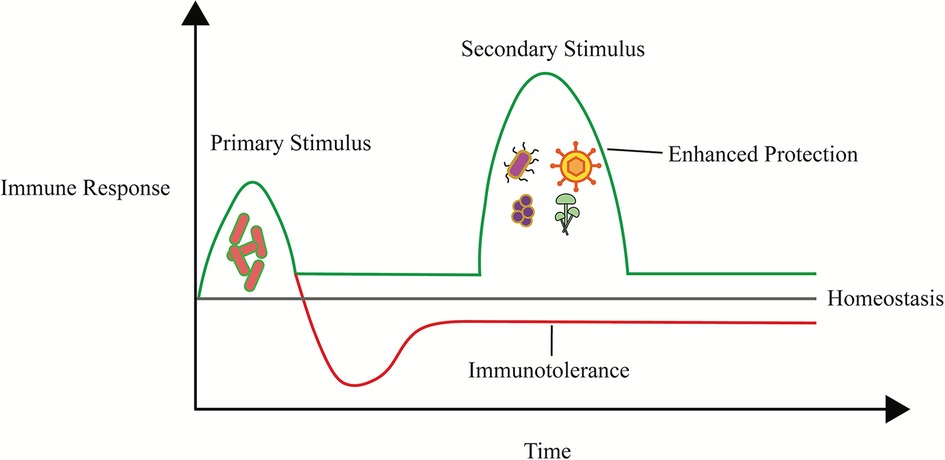
Figure 1. Host microbiota prime immune cells against pathogens or promote immunotolerance. Graph depicting immune training in which a primary stimulus either primes immune responses to protect against or tolerate microbial challenge.
Throughout the GI tract, microbes are typically compartmentalized to the mucosal layer except for the oral cavity in which bacteria may form biofilms on the surfaces of teeth (12). Microbes may break the mucosal barrier during infection when a pathogenic strain invades underlying soft tissue, such as bacteria penetrating the periodontal epithelium surrounding teeth (13). Within the oral cavity, antimicrobial peptides, IgA, and mucin serve as a chemical barrier against infection, much like the mucosal immune system within the intestines (8, 13). However, epithelia in the mouth are multilayered, vary in their permeability and function, and include keratinized and non-keratinized epithelia which differs from the characteristics of intestinal epithelia (8, 13). The multiple layers of oral epithelia provide added protection against invasive pathogens and may contribute to immune tolerance via limiting antigen recognition (8). The mucosal immune system also includes mucosa associated lymphoid tissue (includes the salivary glands and tonsils, the lymphoid follicles, and draining lymph nodes) where most antigen stimulation and clonal expansion of lymphocytes occurs (13, 14). Keratinocytes within the oral cavity recognize bacteria, fungi, protozoa, and viruses through a variety of pattern recognition receptors, including toll-like receptor 2 (TLR2), TLR4, nucleotide-binding oligomerization domain 1 (NOD1), and NOD2 (8, 15). Upon recognition of pathogens, keratinocytes release inflammatory cytokines and chemokines that attract immune cells, such as T cells and natural killer (NK) cells, to the site of infection and create an inflammatory environment (15). Being immunocompromised and the use of antibiotics and antifungals are factors that can lead to infections by opportunistic bacterial and fungal pathogens within the oral cavity (16). Additionally, poor oral hygiene can lead to alterations in microbiota composition that disrupt their metabolic and functional roles (dysbiosis) and subsequent infection.
The interactions between the host immune system and the microbiota within the oral cavity have been the focus of study in recent publications (17–19). Gum disease, particularly periodontitis, is associated with several pathologies and health complications, such as adverse pregnancy outcomes, cardiovascular disease, pulmonary disease, inflammatory bowel disease (IBD), cancer, and type 2 diabetes mellitus (20–23). A recent study linked Porphyromonas gingivalis, a gram-negative bacterium that is one of the keystone pathogens of periodontitis, with Alzheimer's disease in humans, and other researchers are investigating this association in mouse and rat models (24–28). In another study, ectopic colonization of oral microbiota, particularly Klebsiella species, was found to promote Th1 polarization and inflammation within the gut (29). Chronic inflammation due to infection, such as chronic periodontitis, or trauma can lead to several clinical consequences as inflammatory mediators drive further tissue damage and increase the risk of hypertension, hyperglycemia, type 2 diabetes, chronic kidney disease, depression, neurodegenerative diseases, and various types of cancer (30). These findings suggest that oral microbiota may influence inflammatory responses locally and systemically. The potential role of oral microbiota influencing other inflammatory conditions such as allergies is a novel field of research with an impact on health and disease (31). Most recently, evidence suggests that changes in oral microbiota composition are also associated with sensitization and the development of allergic reactions, including asthma and peanut allergies (31–34). Of note, there is evidence that allergic reactions within the gut may contribute to oral dysbiosis in mice (35). Herein, we review the current evidence of the role of the oral microbiota in inflammatory diseases and allergic conditions, as well as its future relevance in ameliorating these inflammatory diseases and allergic conditions.
Host microbiota influence immune system development
The host microbiota is comprised of the various microorganisms found and distributed in distinct parts of the body. Commensal microbiota are recognized by the innate immune system through the interactions of microbe-associated molecular patterns (MAMPs) and pattern recognition receptors (PRRs) expressed in most immune cells, contributing to the training of these immune cells to recognize and eliminate pathogens or promote immunotolerance (Figure 1) (36, 37). Upon recognition, immune cells and other cell types which express PRRs, including epithelial cells, may release cytokines that influence immune responses. Some common innate immune cell types that play roles in inflammatory and allergic diseases include mast cells, basophils, innate lymphoid cells (ILC), macrophages, and dendritic cells. Heterologous innate immune training is a phenomenon in which innate immune cells encounter a primary stimulus that enables them to better eliminate pathogens of different genera or even domains of life (37). For example, the administration of the Bacillus Calmette–Guerin vaccine, a vaccine against tuberculosis, to humans and mice has been found to provide protection against viral, bacterial, fungal, and parasitic infections via trained immunity (37, 38). Dendritic cells (DCs) are phagocytic innate immune cells that can exhibit inflammatory and anti-inflammatory profiles. They play roles in mucosal immunity as they sample antigens and activate lymphocytes after antigen recognition, processing, and peptide presentation on MHC-class II molecules to the lymphocytes. Recognition of certain MAMPs can influence the activation profiles and function of DCs (39). For instance, recognition of the β-glucan wall of Candida albicans promotes a tolerogenic state for those DCs while recognition of Mycobacterium tuberculosis promotes an inflammatory profile (37, 39). Exposure of DCs to bacterial antigens has also been reported to protect against amoeba infection (40). Another example of immune training is macrophages stimulated via TLR signaling are primed towards anti-inflammatory profiles producing lower levels of inflammatory cytokines, such as IL-6 and reactive oxygen species, and exposure to β-glucans promotes cell viability (41–44).
The adaptive immune response, comprised of T cells and B cells, complements innate immunity and is essential in the fight against infection. T cells drive cell-mediated and humoral-mediated immune responses, and their responses are influenced by their interactions with commensal microbiota. For example, microbiota-induced IL-1β production in the intestinal lamina propria induces steady-state differentiation of T helper type 17 (Th17) cells (45, 46). For both the innate and adaptive immune responses, commensal microbiota provide signals that keep the host's immune responses alert for invoking effective immunity when needed. These microorganisms also produce different metabolites and have specific genetic signatures that when interacting with the host have a homeostatic role in influencing the host's organ development, metabolism, and immune response (47–49). Studies in the gut looking at the interaction between microbiota and its host's immune cells suggest that such communication is more prominent at mucosal surfaces (50). One model supporting this communication and the role of the microbiota interactions with the host mucosal immune system is the GF mouse model. These mice have altered hepatic metabolic pathways, poor development of their gut-associated lymphoid tissue (GALT), smaller Peyer's patches, mesenteric lymph nodes, and impaired thymus development (51–54). Together these reported observations suggest that GF mice present underdeveloped immune systems and responses due to the absence of commensal microbiota. Additionally, GF mice colonized with mouse microbiota at birth no longer have altered secondary immune organs (55, 56). Bacterial metabolites such as short chain fatty acids (SCFAs), tryptophan, and retinoic acid (RA) can be detected by the host and influence hematopoiesis, immune responses, and allergic reactions (57–59). Bacterial antigens such as polysaccharide A produced by some commensal bacteria such as Bacteroides fragilis can also have an immunotolerant effect in the host by inducing IL-10 production and expanding Treg cells (60).
The oral microbiota affects inflammatory diseases
Recent evidence suggests that microbial dysbiosis and chronic inflammation within the oral cavity influences systemic inflammation and inflammatory diseases (Figure 2) (20). Of note, increased colonization of oral microbiota within the intestines has been reported in patients suffering from colorectal cancer, HIV, and treatment-naïve Crohn's disease (11, 29, 61–64). Several meta-analyses also indicate that patients with inflammatory bowel disease (IBD) are at higher risk of developing periodontitis and dental caries (65–67). These recent findings suggest that oral microbiota may impact diseases located in other organs and vice versa. In a study that investigated the interplay between oral microbiota and inflammation in the gut, Atarshi et al. reported that increased levels of Rothia, Streptococcus, Neisseria, Pervotella, and Gemella bacteria, typical oral microbiota, were found in the fecal microbiota of patients with inflammatory intestinal and liver diseases such as ulcerative colitis, primary sclerosing cholangitis, gastroesophageal reflux disease, and alcoholism (29). They also observed that colonization of Klebsiella, originally isolated from the saliva of a patient with Crohn's disease, within the intestines of mice induced inflammatory Th1 responses demonstrating that oral microbiota are potential promoters of inflammation (29). A study by Kitamoto et al. investigated how periodontitis and oral dysbiosis influences inflammation within the gut using ligature-induced periodontitis and dextran sodium sulfate (DSS)-induced experimental colitis mouse models (68). Their work demonstrated how ligature-induced periodontitis alone was able to induce the infiltration of Th17 cells, B cells, and γδ Τ cells in the lamina propria of the intestines. The increase in Th17 cells, accompanied by an increase in Th1 cells, was enhanced in oral ligature and DSS treated mice as validate with flow cytometry. Their study shows that oral pathobiont-specific effector memory T cells are able to migrate from cervical lymph nodes to the intestines and exacerbate colitis in mice (Figure 2) (68). These observations illustrate how the microbiota and immune responses in both the mouth and gut are intertwined and influence each other.
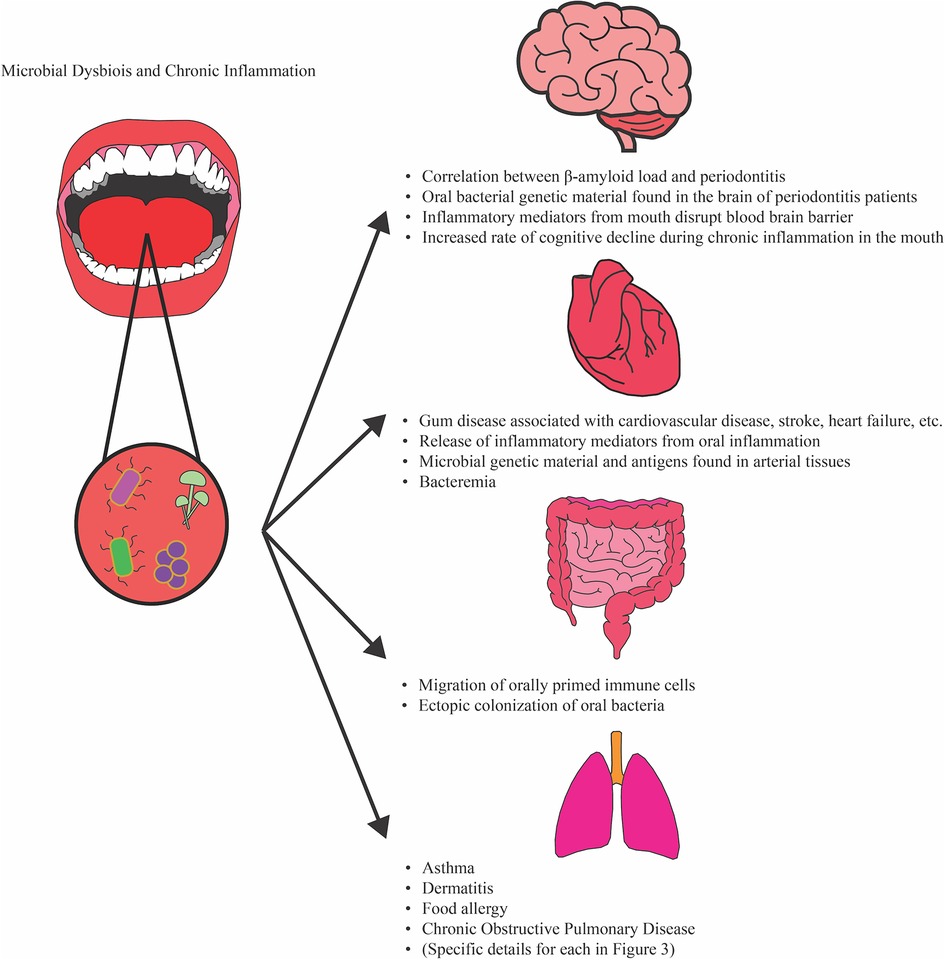
Figure 2. Associations between oral dysbiosis/chronic inflammation and pathologies in distant organs. Oral dysbiosis and chronic inflammation is associated with inflammatory conditions in distant organs, such as the brain, heart, intestines, and lungs. Associations between oral microbiota composition and allergy, dermatitis, asthma, and COPD development have also been reported and are covered in more detail in Figure 3. In the brain, the presence of inflammatory mediators and bacterial antigens may increase the risk of Alzheimer's disease progression and severity. Ectopic colonization and migration of orally primed immune cells have been found to exacerbate colitis in mice. Microbial genetic material and antigens have been found in arterial tissue, and chronic periodontitis have been associated with increased risk of cardiovascular diseases.
Oral dysbiosis and inflammation also has implications in neurodegenerative conditions, such as Alzheimer's disease. Recent studies have shown that chronic periodontitis is positively correlated with Alzheimer's disease, and investigators have determined that chronic periodontitis induces the secretion of inflammatory mediators, including C-reactive protein and IL-6 which may disrupt the blood-brain barrier and contribute to neuroinflammation (69, 70). Additionally, amyloid-β load in the brain of Alzheimer's patients correlates positively with periodontitis (71). A recent study analyzing publicly available data from the National Health and Nutrition Examination Survey performed by the CDC's National Center for Health Statistics found that Alzheimer's diagnoses and associated deaths correlated positively with levels of antibodies against P. gingivalis (72). Further research is needed to determine if P. gingivalis plays a causal role in the development and progression of Alzheimer's disease. In 2016, Ide et al. investigated whether chronic periodontitis was associated with both increased dementia severity and cognitive decline in Alzheimer's disease patients during a 6-month longitudinal study (24). They assessed the cognition and measured serum IL-10 and inflammatory markers (C-reactive protein, TNFα) of 60 patients with mild and severe Alzheimer's disease at the beginning of the study and at 6 months. They found that periodontitis was associated with a 6-fold increase in the rate of cognitive decline at the end of the 6-month period independent of baseline cognitive state. They also report a fall in serum IL-10 levels (p = 0.047) and a modest but not statistically significant increase in C-reactive protein was associated with the presence of periodontitis at baseline (24). These observations from these groups brings to question whether therapeutics to treat periodontitis reduces the risk of heightened disease progression in Alzheimer's disease.
Associations between oral dysbiosis and cardiovascular disease is another topic currently being investigated. There are certainly positive epidemiological associations between periodontitis and higher prevalence of subclinical cardiovascular disease, coronary artery disease, stroke, heart failure, and peripheral artery disease, many of these conditions probably caused by mechanical disruption of oral biofilms which lead to bacteremia (73). This hypothesis is supported by the fact that the DNA and RNA of periodontal pathogens have been found in atherothrombotic tissue (73–76). One possible mechanism for the ectopic colonization of oral bacteria in arterial tissue involves DCs which play a role in the initiation of the inflammatory response in periodontitis and direct the functions of T cells (77). Studies have observed the colocalization of a myeloid DC marker, CD209, and P. gingivalis minor fimbria protein, mfa-1, in the atherosclerotic plaques of deceased coronary artery-disease patients (77). P. gingivalis mfa-1 targets CD209 for entry into DCs and promotes survival within these cells, and the number of CD209+ DCs circulating in the periphery increase during periodontitis (77–80). Thus, inflammation and pathogen burden within the mouth may lead to further complications in cardiovascular disease. As mentioned above, inflammatory mediators, such as C-reactive protein and IL-6, are also elevated in the serum of periodontitis patients (81). C-reactive protein levels are especially higher in patients with periodontitis and cardiovascular disease than healthy patients or patients with either condition (73).
As demonstrated in the studies above, the oral cavity, along with its microbiota and inflammatory responses, is not an isolated region. Immune responses that initiate locally in the gingiva or mucosal epithelia of the mouth and develop into chronic inflammation may influence inflammatory responses in other regions of the body. Thus, further investigation on how treatment of oral inflammation impacts other inflammatory diseases can provide novel approaches to treating conditions such as Crohn's disease, cardiovascular disease, and Alzheimer's disease (Figure 2). Recently, our group performed a meta-analysis on publicly available RNAseq data to identify inflammatory mediators of periodontitis that contribute to local and systemic inflammation and potential drugs that can be repurposed to reduce inflammation (82). By performing analysis of differentially expressed genes and pathways, we determined that FDA-approved drugs that target IL-6 receptor, RANKL, and IFNα/β receptor were among our top candidates to reduce chronic inflammation during periodontitis. However, further investigation in the efficacy of these drugs in reducing inflammation during chronic periodontitis and ameliorating disease progression is needed.
Oral microbiota composition may influence allergies and respiratory disease development
Allergy and hypersensitivity are the result of over responsiveness of the immune system to an otherwise harmless antigen, and chronic allergic inflammation can lead to serious health complications including altered organ function and increased risk of infection in affected sites (83). Allergic diseases, such as respiratory and food allergies, have heterogeneous inflammatory pathologies, characterized by specific dysregulated immunological responses (84). Allergic diseases can be categorized into three temporal phases: early-phase reactions which are induced within seconds of antigen exposure, late-phase reactions which occur within a few hours, and chronic allergic inflammation which persists due to persistent antigen exposure (83). It is during chronic allergic inflammation when tissue restructuring may occur. For example, nasal polyps may appear in patients with allergic rhinitis, and impaired barrier function in the upper airways of allergic rhinitis patients may render them susceptible to chronic sinus infections (83, 85, 86). In patients with asthma, increased number of goblet cells, increased deposition of extracellular matrix molecules in the lamina reticularis, increased number and function of smooth muscle cells, and increased vascularity are some of the changes that can occur in the respiratory tract (83, 87–89). Additionally, levels of tissue-resident immune cell populations, such as ILCs, mast cells, and T cells, typically increase (83, 90). As described above, oral dysbiosis has many implications in the development of chronic inflammatory conditions that influence the mouth and possibly other organs. Thus, it is reasonable that alterations in the oral microbiota composition and, consequently, oral mucosal immunity may influence allergy development locally or in distinct parts of the body. However, research investigating associations between oral inflammation and dysbiosis is still a novel field, and much more work is needed to determine causal relationships. In this section, we highlight some of the most recent findings on the relationship between oral microbiota composition and allergic respiratory disease development. A summary of the studies mentioned in this section can be found in Table 1 which lists information such as the disease studied, the ages of subjects, and oral microbiota implicated in allergic disease development.
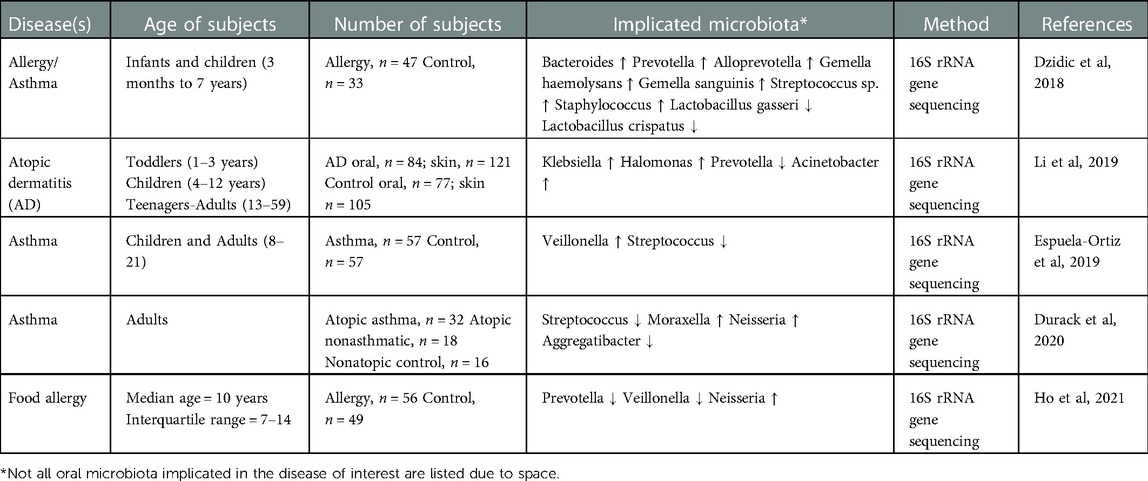
Table 1. Summary of recent studies that identify oral microbiota associated with allergic disease development.
A key finding from the human microbiome project was that there is not a standard healthy microbiota taxonomic composition, but that “healthy” microbiota compositions were best predicted by the molecular functions of the strains found in the population (91, 92). The most common oral bacterial taxa found in healthy individuals are Firmicutes, Proteobacteria, Actinobacteria, Fusobacterium, and Bacteriodetes (9, 93). Recent studies have investigated alterations in oral-pharyngeal microbiota composition and its associations with allergy development, as well as asthma development. An important distinction between allergy and asthma is worth noting here. Asthma is a chronic respiratory disorder that manifests as episodes of wheezing, coughing, and shortness of breath, and severe episodes can sometimes lead to irreversible decline in lung function (94). Atopy, or the genetic tendency to develop allergic diseases such as rhinitis, and specific allergies have been associated with increased risk of developing asthma. However, not all asthmatic patients exhibit elevated allergic responses (94). Thus, allergy and asthma development are distinct though they are both inflammatory diseases and share some common risk factors. Some of the common risk factors between allergic sensitization and asthma development include race, sex, heredity, pollution, passive smoking, obesity, and respiratory viral infection (94, 95).
Dzidic et al. found associations between oral bacteria composition and allergy and asthma development during a 7-year longitudinal study in which DNA from salivary samples was isolated from children at 3, 6, 12, 24 months, and 7 years of age who were developing allergic symptoms and sensitizations (n = 47) and from children with no clear allergic symptoms (healthy) up to 7 years of age (n = 33) (Figure 3) (32). Sensitization was determined by at least 1 positive skin prick test and/or detectable circulating allergen-specific IgE antibodies. Skin prick tests used in this study were performed at 6, 12, 24 months, and 7 years of age on the forearm of the children with potential allergens including: egg white, fresh skimmed cow milk, and standardized cat, birch, and timothy extracts. The allergic diseases considered in their study were eczema, gastrointestinal allergy, allergic rhinoconjuctivitis, and allergic urticaria. Levels of circulating IgE antibodies specific to egg white, cow's milk cod, wheat, peanut, and soybean were also analyzed at 6, 12, and 24 months of age. Asthma diagnosis was determined by either a doctor diagnosis and asthma symptoms and/or medication within the last 12 months, or the presence of wheezing or nocturnal coughing and a positive reversibility test with spirometry. Using next-generation sequencing (NGS), the authors determined that salivary bacterial diversity was significantly lower in 7-year-old children with allergies compared to healthy 7-year-olds. The same is true for 7-year-olds with asthma compared to healthy children. Specifically, they amplified the 16S rRNA gene using universal degenerate primers prior to sequencing and used bioinformatic and statistical software packages to assess bacterial alpha-diversity (diversity within the same sample). The authors identified several genera- and species-specific associations in children with allergies and asthma at different stages of development. Increased relative abundance of the genera Bacteroides at ages 3 months and 7 years were associated with the development of allergic diseases, as were increased levels of Streptococcus lactarius and Gemella haemolysans at 7 years of age. Increased levels of Alloprevotella at 1 year of age and Staphylococcus at 2 years of age were associated with asthma development. They also identified several Streptococci, including S. sanguinis and S. mitis, that were found in greater abundance in asthmatic children. Their work supports the hypothesis that the colonization of a diverse microbiota during early childhood is necessary for inducing and maintaining tolerance (32, 96). However, Dzidic et al. state that their results suggest that the abundance of oral microbiota, such as G. haemolysans, Lactobacillus gasseri, and L. crispatus, was more important than diversity in allergy development.
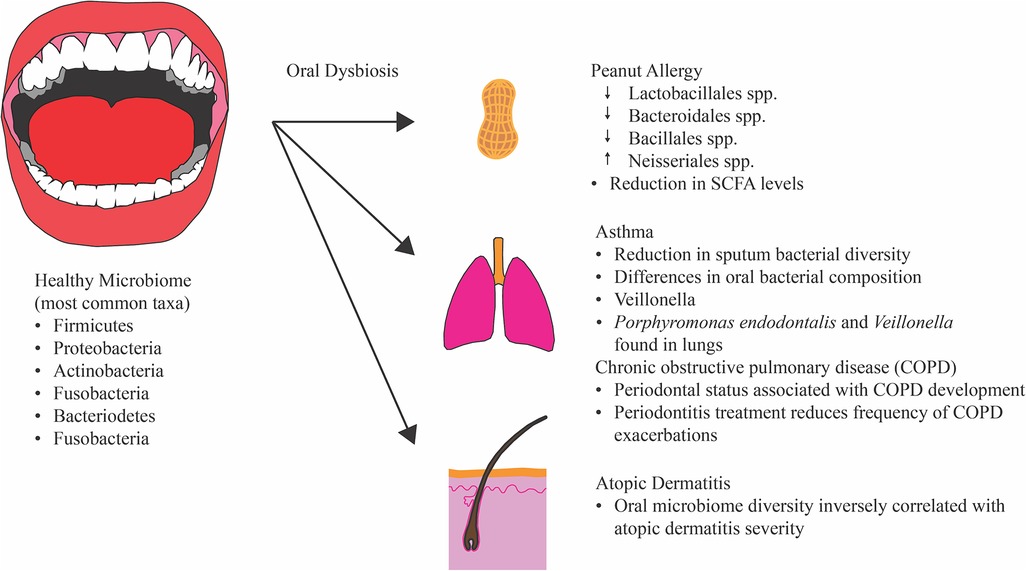
Figure 3. Alterations in oral microbiota composition is associated with allergic and respiratory diseases. Disruptions in oral microbiota composition have been associated with peanut allergy, asthma, chronic obstructive pulmonary disease, and atopic dermatitis, suggesting oral microbiota have an immunomodulatory role with allergy and respiratory development.
Another study by Durack et al. identified associations between sputum and oral microbiota composition with immunological features in atopic asthmatic (AA) and atopic non-asthmatic (ANA) subjects (97). 32 AA, 18 ANA, and 16 non-atopic healthy control subjects were included in their study, and the bacterial diversity and abundance of induced sputum and oral wash samples from the subjects were assessed using NGS of the V4 region of the 16S rRNA gene. Type 2 (T2) airway inflammation was also evaluated by measuring expression levels of 3 bronchial epithelial genes (CLCA1, SERPINB2, and POSTN) induced by IL-13 and calculating a “3 gene mean” score (TGM) score. 22 of the AA subjects were classified as T2-low (TGM < 1.117) in this study. The authors found that sputum burden was inversely associated with the bronchial expression of the T2-related genes and that a cluster of T2-low asthmatic subjects had elevated proinflammatory lung cytokine levels and lower sputum bacterial diversity (Figure 3). Differences in relative abundance of bacteria genera, such as Streptococcus, Moraxella, Neisseria, and Lactobacillus, were associated with asthma and others with atopic status (Granulicatella and Aggregatibacter among others). The authors also noted that differences in oral taxa between the groups seemed more reflective of atopic status (97). Additionally, after asthmatic subjects were treated with inhaled corticosteroids (ICS) for 6 weeks the compositional structure of sputum microbiota demonstrated significantly greater deviation from baseline in ICS non-responsive subjects than in ICS responsive subjects.
Chronic obstructive pulmonary disease (COPD) and atopic dermatitis are two inflammatory diseases that are not considered allergic diseases but share immunological pathways and risk factors to allergic diseases and are worth mentioning briefly. COPD is another chronic inflammatory disease that typically develops and progresses in response to noxious particles and gases, and its development and exacerbation have been associated with severe periodontitis in recent publications (Figure 3) (98–100). Associations between periodontal status and the risk of COPD development remained significant in these studies even after adjustments for other possible risk factors, such as years of smoking, smoking intensity, body mass index, and age (99, 100). Treatment of periodontitis was also associated with reduced frequencies of COPD exacerbations, according to a systematic review performed by Kelly et al. (98). However, a causal link between periodontal status and COPD development has yet to be established. Atopic dermatitis (AD), commonly referred to as eczema, is a skin disorder which results in itchy and inflamed skin lesions. The pathogenesis of AD includes type 1 IgE dysregulation, disruption in skin barrier function which may lead to infections, and cell-mediated immune dysregulation (101). The microbial diversity, assessed by NGS, within the mouth of AD patients (AD, n = 83) was found to be inversely correlated with AD severity in a recent study by Li et al. (Figure 3) (102). Additionally, they observed that 53 bacterial genera were significantly different in the oral cavity of AD patients (n = 83) compared to healthy patients (n = 77). However, a mechanism by which the oral microbiota composition may influence atopic dermatitis severity is still unclear. A possible explanation for the association between oral microbiota composition and AD severity is that systemic inflammation due to AD itself influences oral mucosal immunity and, consequently, oral microbial colonization rather than the reverse direction. Nevertheless, more evidence is needed to establish any sort of causality and directionality.
A study published in 2021 by Ho et al. investigated oral microbial, metabolic, and immunological associations with peanut allergy (34). The study included 56 patients with peanut allergy and 45 non-allergic subjects, and 16S rRNA gene sequencing was performed on salivary samples to assess oral microbiota composition. SCFA metabolite and oral secreted cytokine levels were measured using liquid chromatography/mass spectrometry and multiplex assays, respectively. The authors found that the oral microbiota of individuals with peanut allergy were both lower in phylogenetic diversity and different in composition. Specifically, the oral microbiota of peanut-allergic subjects was characterized by reduced levels of Lactobacillales, Bacteroidales, Streptophyta, and Bacillales. Increased levels of Neisseriales spp. were also observed. Thus, a distinct oral microbiota composition was seen in subjects with peanut allergy (Figure 3). The oral microbiota composition of peanut-allergic subjects (n = 49) was also associated with a statistically significant reduction in oral SCFA levels in comparison with non-allergic subjects (n = 39), including: acetate, butyrate, and propionate. The authors also observed an elevation in IL-4 secretion in peanut-allergic subjects. SCFAs are bacterial fermentation products that modulate immune responses (103). The authors also noted that decreased abundances of Prevotella spp. and Veillonella spp. in peanut-allergic subjects correlated significantly with reduced oral SCFA levels. Increased abundance of Neisseria spp. observed in peanut-allergic subjects was positively correlated with a Th2-skewed oral immune milieu (34).
Evidence suggests that Lactobacillus spp., such as L. crispatus and L. gasseri that can colonize oral mucosal epithelial, serve as immunomodulators of adaptive and innate immune responses and alleviate symptoms in individuals with allergic rhinitis (104, 105). It is known that microaspiration of saliva or nasopharynx secretions contribute to the translocation of oral microbiota to the respiratory tract. However, microaspiration frequency increases during lung disease, and a recent study found that increased presence of oral bacteria, such as Prevotella and Veillonella, can lead to increased Th17 inflammatory responses in the lungs (106). In fact, Espuela-Ortiz et al. evaluated the oral microbiota composition, using 16S rRNA gene sequencing, of African American children and young adults with asthma (n = 57) and non-allergic, non-asthmatic control subjects (n = 57) and observed that Veillonella was predominant in asthmatic subjects (33). Moreover, a study done to analyze the lung microbiota of asthma patients treated with inhaled corticosteroids also observed the presence of oral bacteria, Veillonella and Porphyromonas endodontalis, in the lungs (Figure 3) (107). It is known that asthma medications such as inhalers impact oral microbiota composition, and this complicates the directionality of asthma-oral microbiota conclusions. Still, the observations mentioned in this section suggest that oral microbial composition and ectopic colonization may influence immune response sensitization and contribute to the development of allergies and respiratory disease.
A goal of several of the studies mentioned in this section was to identify biomarkers of allergic disease development, and some genera of bacteria implicated in allergic disease development have been identified in multiple studies, including Prevotella, Neisesria, and Streptococcus (Table 1). The accumulation of additional correlation studies will aid in the identification of species-specific bacteria to study further for their role in allergy development. Associations are currently limited, and more work is needed to establish causal relationships. Additionally, the ambiguity of correlation studies between oral microbiota composition and allergy development is a challenge for this field of research as the directionality is sometimes not clear and some subjects were receiving ICS treatment or probiotic supplementation either during or 6 months before the beginning of the study (32, 34, 97, 107). Also, not all the mentioned studies agree in their findings. For instance, Ho et al., in their peanut allergy study, obtained oral salivary samples from 26 non-food allergic subjects with physician-diagnosed asthma, allergic rhinitis, and/or atopic dermatitis and found no significant differences in alpha-diversity or beta-diversity (variation in bacterial community between samples) between healthy controls and atopic controls without food allergy. Also, significant differences in SCFA levels observed in peanut-allergic subjects were no longer present in the atopic controls without food allergy. Though the size of this cohort is slightly smaller than those in the Dzidic, Durack, and Li studies, these discrepancies highlight the inconsistency of oral microbial associations with allergic diseases and other inflammatory diseases.
Potential therapeutics to treat oral dysbiosis
The implication of oral microbiota influencing the development of allergies and other inflammatory diseases brings to question what can be done to establish healthy oral microbiota to ameliorate disease development. Currently, guidelines to treat oral diseases such as gingivitis and periodontitis, which may influence inflammatory and allergic diseases, rely on mechanical disruption of dental plaque which is generally efficacious in preventing disease progression. The administration of antibiotics or other antimicrobials is also recommended to treat bacterial infection during periodontitis (108). Recently, researchers have investigated other therapeutics to treat oral dysbiosis and infections including the use of probiotics in randomized controlled clinical trials as reviewed by Schlagenhauf and Jockel-Schneider (108). Here, we briefly mention some of those potential approaches (Figure 4).
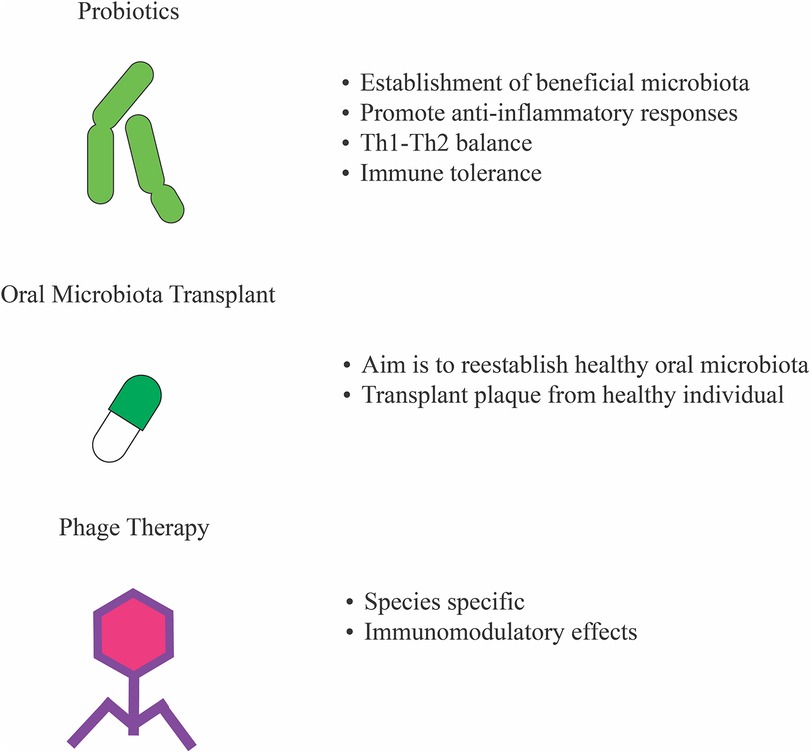
Figure 4. Potential therapeutics to treat oral dysbiosis. Suggested treatments to manage oral microbial dysbiosis to ameliorate diseases and allergies since alterations in microbiota composition is associated with immune sensitization and inflammatory disease.
Probiotics are live microorganisms known to be beneficial as they contribute to the re-establishment of healthy microbiota (109, 110). Probiotics are also associated with boosting immunity and metabolism (111). In the case of immunity, some probiotic strains can promote anti-inflammatory responses such as Lactobacillus acidophilus and Lactobacillus casei which promote Th1-Th2 balance, Lactobacillus rhamnosus which modulates wound healing, and Akkermansia muciniphila (112, 113). Probiotics also promote immune tolerance which may contribute to the prevention of allergies (114). Recent studies have demonstrated that probiotic bacteria, such as lactobacilli, can colonize the oral cavity and contribute to healthy microbiota reestablishment when used in products in contact with the mouth and not when ingested (115–117). However, results from randomized controlled clinical trials up to 2021 (36 total) evaluating the efficacy of probiotics reducing gingival inflammation in gingivitis and periodontitis patients were not consistent (108). These clinical trials varied in their dosage and application of the probiotic with some using the probiotic treatment as an adjunct to mechanical plaque removal and others using it as the only intervention. However, even studies with similar methods had contradicting results. Some studies report enhanced pocket closure in periodontitis patients receiving probiotic treatment as an adjunct to mechanical plaque removal when compared to the placebo group, while other groups failed to detect any significant additional benefit. The same was true for gingivitis patients given the probiotic as an adjunct. Nevertheless, the efficacy of probiotic use to treat oral dysbiosis can still be argued for in cases where the concept of strict mechanical plaque control fails, as supported by the positive results seen in trials where probiotic treatment was the only therapeutic measure given to individuals with chronic gingivitis or periodontitis and habitual poor oral hygiene (108).
Oral microbiota transplants (OMTs) have been suggested as a means to treat oral microbial dysbiosis (118). Inspired by the fecal microbiota transplants (FMT), OMTs involve the transfer of plaque from a healthy individual to a patient with gum disease (119, 120). FMTs have shown promising results in treating allergies such as peanut allergy by restoring healthy microbiota, and future studies can focus on the possibility to treat not only dysbiotic microbiota in the oral cavity but also aid in the prevention of allergies. However, no published study has tested the feasibility and efficacy of this treatment.
One of the more recently considered treatments to treat oral dysbiosis is bacteriophage-based therapy. Bacteriophages are viruses that target bacteria, and recent studies have suggested their potential role to reestablish beneficial microbiota and inducing immunomodulation (121). Bacteriophages have the unique ability to target specific bacteria, a characteristic that can be genetically modified (122). However, phage-resistant strains of bacteria may sometimes persist, and the targeting of one bacterial species may induce quantitative shifts in the population of another as microbiota communities are interactive (123). Bacteriophage therapy studies have shown anti-inflammatory effects by reducing levels of C-reactive protein and promoting the up-regulation of the anti-inflammatory cytokine IL-10, and the downregulation of TLR4 (124, 125). The use of bacteriophage-based therapy to alter oral microbiota composition remains an active area of research with many questions still needing to be addressed.
Conclusions and future directions
It's clear that human microbiota influence immune system development and training, and correlations between oral microbiota composition and sensitization contributing to inflammatory diseases and allergic conditions have been made recently. Indeed, alterations in oral microbiota composition has many implications in chronic inflammation and other diseases. Oral microbiota composition has been associated with systemic inflammation and diseases ranging from cardiovascular disease to colitis and neurodegenerative disorders. Regarding allergic diseases, determining associations and mechanistic links between oral microbiota and allergic disease development is a novel field that is providing insight into risk factors for allergy development and possible therapeutic targets. Impaired barrier function, altered organ function, and susceptibility to infection caused by chronic allergic reactions and other inflammatory conditions, including chronic rhinitis and asthma, highlight the importance of this research. More research is needed to establish causal relationships and mechanisms between oral microbiota composition and the development of inflammatory diseases and allergic conditions. As we better understand how microbial composition in the oral cavity plays an immunomodulatory role, it may be possible to develop improved and novel therapeutics to treat or prevent the development of these inflammatory diseases and allergic conditions.
Author contributions
Conceptualization: CM, CF, and SW. Methodology: CM, CF and SW. Writing—original draft preparation: CM, EB and CF. Writing— review and editing: CM, SW, CF and EB. Visualization: CM and SW. Supervision: CF and SW. Project administration: CF and SW. All authors have read and agreed to the published version of the manuscript.
Conflict of interest
The authors declare that the research was conducted in the absence of any commercial or financial relationships that could be construed as a potential conflict of interest.
Publisher's note
All claims expressed in this article are solely those of the authors and do not necessarily represent those of their affiliated organizations, or those of the publisher, the editors and the reviewers. Any product that may be evaluated in this article, or claim that may be made by its manufacturer, is not guaranteed or endorsed by the publisher.
References
1. Zheng D, Liwinski T, Elinav E. Interaction between microbiota and immunity in health and disease. Cell Res. (2020) 30(6):492–506. doi: 10.1038/s41422-020-0332-7
2. Umesaki Y, Setoyama H, Matsumoto S, Okada Y. Expansion of alpha beta T-cell receptor-bearing intestinal intraepithelial lymphocytes after microbial colonization in germ-free mice and its independence from thymus. Immunology. (1993) 79(1):32–7. Available from: https://www.ncbi.nlm.nih.gov/pubmed/85091408509140
3. Tan TG, Sefik E, Geva-Zatorsky N, Kua L, Naskar D, Teng F, Mathis D. Identifying species of symbiont bacteria from the human gut that, alone, can induce intestinal Th17 cells in mice. Proc Natl Acad Sci U S A. (2016) 113(50):E8141–50. doi: 10.1073/pnas.1617460113
4. Bauer H, Horowitz RE, Levenson SM, Popper H. The response of the lymphatic tissue to the microbial flora. Studies on germfree mice. Am J Pathol. (1963) 42:471–83. Available from: https://www.ncbi.nlm.nih.gov/pubmed/1396692913966929
5. Kawamoto S, Maruya M, Kato LM, Suda W, Atarashi K, Doi Y, Fagarasan S. Foxp3(+) T cells regulate immunoglobulin a selection and facilitate diversification of bacterial species responsible for immune homeostasis. Immunity. (2014) 41(1):152–65. doi: 10.1016/j.immuni.2014.05.016
6. Hapfelmeier S, Lawson MA, Slack E, Kirundi JK, Stoel M, Heikenwalder M, Macpherson AJ. Reversible microbial colonization of germ-free mice reveals the dynamics of IgA immune responses. Science. (2010) 328(5986):1705–9. doi: 10.1126/science.1188454
7. Mazmanian SK, Liu CH, Tzianabos AO, Kasper DL. An immunomodulatory molecule of symbiotic bacteria directs maturation of the host immune system. Cell. (2005) 122(1):107–18. doi: 10.1016/j.cell.2005.05.007
8. Suarez LJ, Arboleda S, Angelov N, Arce RM. Oral versus gastrointestinal mucosal immune niches in homeostasis and allostasis. Front Immunol. (2021) 12:705206. doi: 10.3389/fimmu.2021.705206
9. Deo PN, Deshmukh R. Oral microbiome: unveiling the fundamentals. J Oral Maxillofac Pathol. (2019) 23(1):122–8. doi: 10.4103/jomfp.JOMFP_304_18
10. Paster BJ, Olsen I, Aas JA, Dewhirst FE. The breadth of bacterial diversity in the human periodontal pocket and other oral sites. Periodontol 2000. (2006) 42:80–7. doi: 10.1111/j.1600-0757.2006.00174.x
11. Willis JR, Gabaldon T. The human oral microbiome in health and disease: from sequences to ecosystems. Microorganisms. (2020) 8(2):1–28. doi: 10.3390/microorganisms8020308
12. Vancamelbeke M, Vermeire S. The intestinal barrier: a fundamental role in health and disease. Expert Rev Gastroenterol Hepatol. (2017) 11(9):821–34. doi: 10.1080/17474124.2017.1343143
13. Wu RQ, Zhang DF, Tu E, Chen QM, Chen W. The mucosal immune system in the oral cavity-an orchestra of T cell diversity. Int J Oral Sci. (2014) 6(3):125–32. doi: 10.1038/ijos.2014.48
14. Kiyono H, Azegami T. The mucosal immune system: from dentistry to vaccine development. Proc Jpn Acad Ser B Phys Biol Sci. (2015) 91(8):423–39. doi: 10.2183/pjab.91.423
15. Feller L, Altini M, Khammissa RAG, Chandran R, Bouckaert M, Lemmer J. Oral mucosal immunity. Oral Surg Oral Med Oral Radiol. (2013) 116(5):576–83. doi: 10.1016/j.oooo.2013.07.013
16. Cannon RD. Oral fungal infections: past, present, and future. Front Oral Health. (2022) 3:838639. doi: 10.3389/froh.2022.838639
17. Idris A, Hasnain SZ, Huat LZ, Koh D. Human diseases, immunity and the oral microbiota-insights gained from metagenomic studies. Oral Sci Int. (2017) 14(2):27–32. doi: 10.1016/S1348-8643(16)30024-6
18. Zenobia C, Herpoldt KL, Freire M. Is the oral microbiome a source to enhance mucosal immunity against infectious diseases? NPJ Vaccines. (2021) 6(1):80. doi: 10.1038/s41541-021-00341-4
19. Ptasiewicz M, Grywalska E, Mertowska P, Korona-Glowniak I, Poniewierska-Baran A, Niedzwiedzka-Rystwej P, Chalas R. Armed to the teeth-the oral mucosa immunity system and microbiota. Int J Mol Sci. (2022) 23(2):1–19. doi: 10.3390/ijms23020882
20. Hajishengallis G, Chavakis T. Local and systemic mechanisms linking periodontal disease and inflammatory comorbidities. Nat Rev Immunol. (2021) 21(7):426–40. doi: 10.1038/s41577-020-00488-6
21. Pihlstrom BL, Michalowicz BS, Johnson NW. Periodontal diseases. Lancet. (2005) 366(9499):1809–20. doi: 10.1016/S0140-6736(05)67728-8
22. Bui FQ, Almeida-da-Silva CLC, Huynh B, Trinh A, Liu J, Woodward J, Ojcius DM. Association between periodontal pathogens and systemic disease. Biomed J. (2019) 42(1):27–35. doi: 10.1016/j.bj.2018.12.001
23. Shi B, Lux R, Klokkevold P, Chang M, Barnard E, Haake S, Li H. The subgingival microbiome associated with periodontitis in type 2 diabetes mellitus. ISME J. (2020) 14(2):519–30. doi: 10.1038/s41396-019-0544-3
24. Ide M, Harris M, Stevens A, Sussams R, Hopkins V, Culliford D, Holmes C. Periodontitis and cognitive decline in Alzheimer's disease. PloS One. (2016) 11(3):1–9. doi: 10.1371/journal.pone.0151081
25. Zhang J, Yu CB, Zhang X, Chen HW, Dong JC, Lu WL, Zhou W. Porphyromonas gingivalis lipopolysaccharide induces cognitive dysfunction, mediated by neuronal inflammation via activation of the TLR4 signaling pathway in C57BL/6 mice. J Neuroinflammation. (2018) 15:1–14. doi: 10.1186/s12974-017-1052-x
26. Hu Y, Li HX, Zhang J, Zhang X, Xia XY, Qiu C, Zhou W. Periodontitis induced by P. gingivalis-LPS is associated with neuroinflammation and learning and memory impairment in sprague-dawley rats. Front Neurosci. (2020) 14:1–15. doi: 10.3389/fnins.2020.00658
27. Olsen I. Porphyromonas gingivalis-induced neuroinflammation in Alzheimer's disease. Front Neurosci. (2021) 15:1–5: doi: 10.3389/fnins.2021.691016
28. Kanagasingam S, Chukkapalli SS, Welbury R, Singhrao SK. Porphyromonas gingivalis is a strong risk factor for Alzheimer's disease. J Alzheimer's Dis. (2020) 4(1):501–11. doi: 10.3233/ADR-200250
29. Atarashi K, Suda W, Luo C, Kawaguchi T, Motoo I, Narushima S, Honda K. Ectopic colonization of oral bacteria in the intestine drives TH1 cell induction and inflammation. Science. (2017) 358(6361):359–65. doi: 10.1126/science.aan4526
30. Furman D, Campisi J, Verdin E, Carrera-Bastos P, Targ S, Franceschi C, Slavich GM. Chronic inflammation in the etiology of disease across the life span. Nat Med. (2019) 25(12):1822–32. doi: 10.1038/s41591-019-0675-0
31. Cai Y, Zhao Y, Kang Y, Yang Y. Future prospect of oral microbiota influencing allergy/asthma development. Front Microbiol. (2022) 13:875664. doi: 10.3389/fmicb.2022.875664
32. Dzidic M, Abrahamsson TR, Artacho A, Collado MC, Mira A, Jenmalm MC. Oral microbiota maturation during the first 7 years of life in relation to allergy development. Allergy. (2018) 73(10):2000–11. doi: 10.1111/all.13449
33. Espuela-Ortiz A, Lorenzo-Diaz F, Baez-Ortega A, Eng C, Hernandez-Pacheco N, Oh SS, Pino-Yanes M. Bacterial salivary microbiome associates with asthma among African American children and young adults. Pediatr Pulmonol. (2019) 54(12):1948–56. doi: 10.1002/ppul.24504
34. Ho HE, Chun Y, Jeong S, Jumreornvong O, Sicherer SH, Bunyavanich S. Multidimensional study of the oral microbiome, metabolite, and immunologic environment in peanut allergy. J Allergy Clin Immunol. (2021) 148(2):627–32.e3. doi: 10.1016/j.jaci.2021.03.028
35. Matsui S, Kataoka H, Tanaka JI, Kikuchi M, Fukamachi H, Morisaki H, Kuwata H. Dysregulation of intestinal microbiota elicited by food allergy induces IgA-mediated oral dysbiosis. Infect Immun. (2019) 88(1):1–12. doi: 10.1128/IAI.00741-19
36. Franchi L, Kamada N, Nakamura Y, Burberry A, Kuffa P, Suzuki S, Nunez G. NLRC4-driven production of IL-1β discriminates between pathogenic and commensal bacteria and promotes host intestinal defense. Nat Immunol. (2012) 13(5):449–56. doi: 10.1038/ni.2263
37. Adams K, Weber KS, Johnson SM. Exposome and immunity training: how pathogen exposure order influences innate immune cell lineage commitment and function. Int J Mol Sci. (2020) 21(22):1–26. doi: 10.3390/ijms21228462
38. Blok BA, Arts RJW, van Crevel R, Benn CS, Netea MG. Trained innate immunity as underlying mechanism for the long-term, nonspecific effects of vaccines. J Leukocyte Biol. (2015) 98(3):347–56. doi: 10.1189/jlb.5RI0315-096R
39. Bonifazi P, Zelante T, D'Angelo C, De Luca A, Moretti S, Bozza S, Romani L. Balancing inflammation and tolerance in vivo through dendritic cells by the commensal Candida albicans. Mucosal Immunol. (2009) 2(4):362–74. doi: 10.1038/mi.2009.17
40. Burgess SL, Buonomo E, Carey M, Cowardin C, Naylor C, Noor Z, Petri WA. Bone marrow dendritic cells from mice with an altered microbiota provide interleukin 17A-dependent protection against entamoeba histolytica colitis. Mbio. (2014) 5(6):1–8. doi: 10.1128/mBio.01817-14
41. Foster SL, Hargreaves DC, Medzhitov R. Gene-specific control of inflammation by TLR-induced chromatin modifications. Nature. (2007) 447(7147):972–4. doi: 10.1038/nature05836
42. Deng H, Maitra U, Morris M, Li LW. Molecular mechanism responsible for the priming of macrophage activation. J Biol Chem. (2013) 288(6):3897–906. doi: 10.1074/jbc.M112.424390
43. Yanez A, Hassanzadeh-Kiabi N, Ng MY, Megias J, Subramanian A, Liu GY, Goodridge HS. Detection of a TLR2 agonist by hematopoietic stem and progenitor cells impacts the function of the macrophages they produce. Eur J Immunol. (2013) 43(8):2114–25. doi: 10.1002/eji.201343403
44. Garcia-Valtanen P, Guzman-Genuino RM, Williams DL, Hayball JD, Diener KR. Evaluation of trained immunity by beta-1, 3 (D)-glucan on murine monocytes in vitro and duration of response in vivo. Immunol Cell Biol. (2017) 95(7):601–10. doi: 10.1038/icb.2017.13
45. Russo E, Taddei A, Ringressi MN, Ricci F, Amedei A. The interplay between the microbiome and the adaptive immune response in cancer development. Therap Adv Gastroenterol. (2016) 9(4):594–605. doi: 10.1177/1756283X16635082
46. Shaw MH, Kamada N, Kim YG, Nunez G. Microbiota-induced IL-1beta, but not IL-6, is critical for the development of steady-state TH17 cells in the intestine. J Exp Med. (2012) 209(2):251–8. doi: 10.1084/jem.20111703
47. Spencer SP, Wilhelm C, Yang Q, Hall JA, Bouladoux N, Boyd A, Belkaid Y. Adaptation of innate lymphoid cells to a micronutrient deficiency promotes type 2 barrier immunity. Science. (2014) 343(6169):432–7. doi: 10.1126/science.1247606
48. Sommer F, Bäckhed F. The gut microbiota–masters of host development and physiology. Nat Rev Microbiol. (2013) 11(4):227–38. doi: 10.1038/nrmicro2974
49. Round JL, Mazmanian SK. Inducible Foxp3+ regulatory T-cell development by a commensal bacterium of the intestinal microbiota. Proc Natl Acad Sci U S A. (2010) 107(27):12204–9. doi: 10.1073/pnas.0909122107
50. Levy M, Thaiss CA, Elinav E. Metabolites: messengers between the microbiota and the immune system. Genes Dev. (2016) 30(14):1589–97. doi: 10.1101/gad.284091.116
51. Ennamorati M, Vasudevan C, Clerkin K, Halvorsen S, Verma S, Ibrahim S, Jain N. Intestinal microbes influence development of thymic lymphocytes in early life. Proc Natl Acad Sci U S A. (2020) 117(5):2570–8. doi: 10.1073/pnas.1915047117
52. Macpherson AJ, Harris NL. Interactions between commensal intestinal bacteria and the immune system. Nat Rev Immunol. (2004) 4(6):478–85. doi: 10.1038/nri1373
53. Bouskra D, Brézillon C, Berard M, Werts C, Varona R, Boneca IG, Eberl G. Lymphoid tissue genesis induced by commensals through NOD1 regulates intestinal homeostasis. Nature. (2008) 456(7221):507–10. doi: 10.1038/nature07450
54. Han LW, Shi Y, Paquette A, Wang L, Bammler TK, Mao Q. Key hepatic metabolic pathways are altered in germ-free mice during pregnancy. PLoS One. (2021) 16(3):e0248351. doi: 10.1371/journal.pone.0248351
55. Smith K, McCoy KD, Macpherson AJ. Use of axenic animals in studying the adaptation of mammals to their commensal intestinal microbiota. Semin Immunol. (2007) 19(2):59–69. doi: 10.1016/j.smim.2006.10.002
56. Chung H, Pamp SJ, Hill JA, Surana NK, Edelman SM, Troy EB, Kasper DL. Gut immune maturation depends on colonization with a host-specific microbiota. Cell. (2012) 149(7):1578–93. doi: 10.1016/j.cell.2012.04.037
57. Khosravi A, Yanez A, Price JG, Chow A, Merad M, Goodridge HS, Mazmanian SK. Gut microbiota promote hematopoiesis to control bacterial infection. Cell Host Microbe. (2014) 15(3):374–81. doi: 10.1016/j.chom.2014.02.006
58. Trompette A, Gollwitzer ES, Yadava K, Sichelstiel AK, Sprenger N, Ngom-Bru C, Marsland BJ. Gut microbiota metabolism of dietary fiber influences allergic airway disease and hematopoiesis. Nat Med. (2014) 20(2):159–66. doi: 10.1038/nm.3444
59. Zelante T, Iannitti RG, Cunha C, De Luca A, Giovannini G, Pieraccini G, Romani L. Tryptophan catabolites from microbiota engage aryl hydrocarbon receptor and balance mucosal reactivity via interleukin-22. Immunity. (2013) 39(2):372–85. doi: 10.1016/j.immuni.2013.08.003
60. Ochoa-Reparaz J, Mielcarz DW, Ditrio LE, Burroughs AR, Begum-Haque S, Dasgupta S, Kasper LH. Central nervous system demyelinating disease protection by the human commensal bacteroides fragilis depends on polysaccharide A expression. J Immunol. (2010) 185(7):4101–8. doi: 10.4049/jimmunol.1001443
61. Gevers D, Kugathasan S, Denson LA, Vazquez-Baeza Y, Van Treuren W, Ren B, Xavier RJ. The treatment-naive microbiome in new-onset crohn's disease. Cell Host Microbe. (2014) 15(3):382–92. doi: 10.1016/j.chom.2014.02.005
62. Lozupone CA, Li M, Campbell TB, Flores SC, Linderman D, Gebert MJ, Palmer BE. Alterations in the gut microbiota associated with HIV-1 infection. Cell Host Microbe. (2013) 14(3):329–39. doi: 10.1016/j.chom.2013.08.006
63. Vujkovic-Cvijin I, Dunham RM, Iwai S, Maher MC, Albright RG, Broadhurst MJ, McCune JM. Dysbiosis of the gut microbiota is associated with HIV disease progression and tryptophan catabolism. Sci Transl Med. (2013) 5(193):193ra91. doi: 10.1126/scitranslmed.3006438
64. Sears CL, Garrett WS. Microbes, microbiota, and colon cancer. Cell Host Microbe. (2014) 15(3):317–28. doi: 10.1016/j.chom.2014.02.007
65. Papageorgiou SN, Hagner M, Nogueira AV, Franke A, Jager A, Deschner J. Inflammatory bowel disease and oral health: systematic review and a meta-analysis. J Clin Periodontol. (2017) 44(4):382–93. doi: 10.1111/jcpe.12698
66. Zhang YH, Qiao D, Chen RX, Zhu F, Gong JF, Yan FH. The association between periodontitis and inflammatory bowel disease: a systematic review and meta-analysis. BioMed Res Int. (2021) 2021:1–8. doi: 10.1155/2021/6692420
67. Zhang L, Gao X, Zhou J, Chen S, Zhang J, Zhang Y, Yang J. Increased risks of dental caries and periodontal disease in Chinese patients with inflammatory bowel disease. Int Dent J. (2020) 70(3):227–36. doi: 10.1111/idj.12542
68. Kitamoto S, Nagao-Kitamoto H, Jiao Y, Gillilland MG 3rd, Hayashi A, Imai J, Kamada N. The intermucosal connection between the mouth and gut in commensal pathobiont-driven colitis. Cell. (2020) 182(2):447–62.e14. doi: 10.1016/j.cell.2020.05.048
69. Furutama D, Matsuda S, Yamawaki Y, Hatano S, Okanobu A, Memida T, Kurihara H. IL-6 Induced by periodontal inflammation causes neuroinflammation and disrupts the blood-brain barrier. Brain Sci. (2020) 10(10):1–13. doi: 10.3390/brainsci10100679
70. Teixeira FB, Saito MT, Matheus FC, Prediger RD, Yamada ES, Maia CSF, Lima RR. Periodontitis and Alzheimer's disease: a possible comorbidity between oral chronic inflammatory condition and neuroinflammation. Front Aging Neurosci. (2017) 9:327. doi: 10.3389/fnagi.2017.00327
71. Kamer AR, Pirraglia E, Tsui W, Rusinek H, Vallabhajosula S, Mosconi L, de Leon MJ. Periodontal disease associates with higher brain amyloid load in normal elderly. Neurobiol Aging. (2015) 36(2):627–33. doi: 10.1016/j.neurobiolaging.2014.10.038
72. Beydoun MA, Beydoun HA, Hossain S, El-Hajj ZW, Weiss J, Zonderman AB. Clinical and bacterial markers of periodontitis and their association with incident all-cause and Alzheimer's disease dementia in a large national survey. J Alzheimer's Dis. (2020) 75(1):157–72. doi: 10.3233/JAD-200064
73. Sanz M, Del Castillo AM, Jepsen S, Gonzalez-Juanatey JR, D'Aiuto F, Bouchard P, Wimmer G. Periodontitis and cardiovascular diseases: consensus report. Glob Heart. (2020) 15(1):1. doi: 10.5334/gh.400
74. Armingohar Z, Jorgensen JJ, Kristoffersen AK, Abesha-Belay E, Olsen I. Bacteria and bacterial DNA in atherosclerotic plaque and aneurysmal wall biopsies from patients with and without periodontitis. J Oral Microbiol. (2014) 6:1–13. doi: 10.3402/jom.v6.23408
75. Pinon-Esteban P, Nunez L, Moure R, Marron-Linares GM, Flores-Rios X, Aldama-Lopez G, Vazquez-Rodriguez JM. Presence of bacterial DNA in thrombotic material of patients with myocardial infarction. Sci Rep. (2020) 10(1):1–8. doi: 10.1038/s41598-020-73011-5
76. Szulc M, Kustrzycki W, Janczak D, Michalowska D, Baczynska D, Radwan-Oczko M. Presence of periodontopathic bacteria DNA in atheromatous plaques from coronary and carotid arteries. BioMed Res Int. (2015) 2015:1–6. doi: 10.1155/2015/825397
77. Meghil MM, Cutler CW. Oral microbes and mucosal dendritic cells, “spark and flame” of local and distant inflammatory diseases. Int J Mol Sci. (2020) 21(5):1–17. doi: 10.3390/ijms21051643
78. Hamada N, Sojar HT, Cho MI, Genco RJ. Isolation and characterization of a minor fimbria from Porphyromonas gingivalis. Infect Immun. (1996) 64(11):4788–94. doi: 10.1128/iai.64.11.4788-4794.1996
79. Zeituni AE, Jotwani R, Carrion J, Cutler CW. Targeting of DC-SIGN on human dendritic cells by minor fimbriated Porphyromonas gingivalis strains elicits a distinct effector T cell response. J Immunol. (2009) 183(9):5694–704. doi: 10.4049/jimmunol.0901030
80. Carrion J, Scisci E, Miles B, Sabino GJ, Zeituni AE, Gu Y, Cutler CW. Microbial carriage state of peripheral blood dendritic cells (DCs) in chronic periodontitis influences DC differentiation, atherogenic potential. J Immunol. (2012) 189(6):3178–87. doi: 10.4049/jimmunol.1201053
81. Machado V, Botelho J, Escalda C, Hussain SB, Luthra S, Mascarenhas P, D'Aiuto F. Serum C-reactive protein and periodontitis: a systematic review and meta-analysis. Front Immunol. (2021) 12:706432. doi: 10.3389/fimmu.2021.706432
82. Moreno C, Bybee E, Tellez Freitas CM, Pickett BE, Weber KS. Meta-analysis of two human RNA-seq datasets to determine periodontitis diagnostic biomarkers and drug target candidates. Int J Mol Sci. (2022) 23(10):1–17. doi: 10.3390/ijms23105580
83. Galli SJ, Tsai M, Piliponsky AM. The development of allergic inflammation. Nature. (2008) 454(7203):445–54. doi: 10.1038/nature07204
84. Pascal M, Perez-Gordo M, Caballero T, Escribese MM, Lopez Longo MN, Luengo O, Mayorga C. Microbiome and allergic diseases. Front Immunol. (2018) 9:1584. doi: 10.3389/fimmu.2018.01584
85. Pawankar R, Nonaka M, Yamagishi S, Yagi T. Pathophysiologic mechanisms of chronic rhinosinusitis. Immunol Allergy Clin North Am. (2004) 24(1):75–85. doi: 10.1016/S0889-8561(03)00109-7
86. Takano K, Kojima T, Go M, Murata M, Ichimiya S, Himi T, Sawada N. HLA-DR- and CD11c-positive dendritic cells penetrate beyond well-developed epithelial tight junctions in human nasal mucosa of allergic rhinitis. J Histochem Cytochem. (2005) 53(5):611–9. doi: 10.1369/jhc.4A6539.2005
87. Holgate ST. Epithelium dysfunction in asthma. J Allergy Clin Immunol. (2007) 120(6):1233–44; quiz 1245–6. doi: 10.1016/j.jaci.2007.10.025
88. Mauad T, Bel EH, Sterk PJ. Asthma therapy and airway remodeling. J Allergy Clin Immunol. (2007) 120(5):997–1009; quiz 1010–1. doi: 10.1016/j.jaci.2007.06.031
89. Doherty T, Broide D. Cytokines and growth factors in airway remodeling in asthma. Curr Opin Immunol. (2007) 19(6):676–80. doi: 10.1016/j.coi.2007.07.017
90. Panda SK, Colonna M. Innate lymphoid cells in mucosal immunity. Front Immunol. (2019) 10:861. doi: 10.3389/fimmu.2019.00861
91. Human Microbiome Project Consortium. Structure, function and diversity of the healthy human microbiome. Nature. (2012) 486(7402):207–14. doi: 10.1038/nature11234
92. Integrative HMP (iHMP) Research Network Consortium. The integrative human microbiome project. Nature. (2019) 569(7758):641–8. doi: 10.1038/s41586-019-1238-8
93. Zaura E, Keijser BJF, Huse SM, Crielaard W. Defining the healthy “core microbiome” of oral microbial communities. BMC Microbiol. (2009) 9(1):259. doi: 10.1186/1471-2180-9-259
94. Toskala E, Kennedy DW. Asthma risk factors. Int Forum Allergy Rhinol. (2015) 5(Suppl 1):S11–6. doi: 10.1002/alr.21557
95. Aldakheel FM. Allergic diseases: a comprehensive review on risk factors, immunological mechanisms, link with COVID-19, potential treatments, and role of allergen bioinformatics. Int J Environ Res Public Health. (2021) 18(22):1–29. doi: 10.3390/ijerph182212105
96. Ho HE, Bunyavanich S. Role of the microbiome in food allergy. Curr Allergy Asthma Rep. (2018) 18(4):27. doi: 10.1007/s11882-018-0780-z
97. Durack J, Christian LS, Nariya S, Gonzalez J, Bhakta NR, Ansel KM, AsthmaN NHLBI. Distinct associations of sputum and oral microbiota with atopic, immunologic, and clinical features in mild asthma. J Allergy Clin Immunol. (2020) 146(5):1016–26. doi: 10.1016/j.jaci.2020.03.028
98. Kelly N, Winning L, Irwin C, Lundy FT, Linden D, McGarvey L, El Karim IA. Periodontal status and chronic obstructive pulmonary disease (COPD) exacerbations: a systematic review. BMC Oral Health. (2021) 21(1):425. doi: 10.1186/s12903-021-01757-z
99. Vogelmeier CF, Criner GJ, Martinez FJ, Anzueto A, Barnes PJ, Bourbeau J, Agusti A. Global strategy for the diagnosis, management, and prevention of chronic obstructive lung disease 2017 report. Am J Respir Crit Care Med. (2017) 195(5):557–82. doi: 10.1164/rccm.201701-0218PP
100. Takeuchi K, Matsumoto K, Furuta M, Fukuyama S, Takeshita T, Ogata H, Yamashita Y. Periodontitis is associated with chronic obstructive pulmonary disease. J Dent Res. (2019) 98(5):534–40. doi: 10.1177/0022034519833630
101. David Boothe W, Tarbox JA, Tarbox MB. Atopic dermatitis: pathophysiology. Adv Exp Med Biol. (2017) 1027:21–37. doi: 10.1007/978-3-319-64804-0_3
102. Li W, Xu X, Wen H, Wang Z, Ding C, Liu X, Yao X. Inverse association between the skin and oral microbiota in atopic dermatitis. J Invest Dermatol. (2019) 139(8):1779–87.e12. doi: 10.1016/j.jid.2019.02.009
103. Correa-Oliveira R, Fachi JL, Vieira A, Sato FT, Vinolo MA. Regulation of immune cell function by short-chain fatty acids. Clin Transl Immunology. (2016) 5(4):e73. doi: 10.1038/cti.2016.17
104. Kawase M, He F, Kubota A, Hiramatsu M, Saito H, Ishii T, Akiyama K. Effect of fermented milk prepared with two probiotic strains on Japanese cedar pollinosis in a double-blind placebo-controlled clinical study. Int J Food Microbiol. (2009) 128(3):429–34. doi: 10.1016/j.ijfoodmicro.2008.09.017
105. Selle K, Klaenhammer TR. Genomic and phenotypic evidence for probiotic influences of Lactobacillus gasseri on human health. FEMS Microbiol Rev. (2013) 37(6):915–35. doi: 10.1111/1574-6976.12021
106. Segal LN, Clemente JC, Tsay JC, Koralov SB, Keller BC, Wu BG, Weiden MD. Enrichment of the lung microbiome with oral taxa is associated with lung inflammation of a Th17 phenotype. Nat Microbiol. (2016) 1:16031. doi: 10.1038/nmicrobiol.2016.31
107. Martin MJ, Zain NMM, Hearson G, Rivett DW, Koller G, Wooldridge DJ, Harrison TW. The airways microbiome of individuals with asthma treated with high and low doses of inhaled corticosteroids. PLoS One. (2021) 15(12):e0244681. doi: 10.1371/journal.pone.0244681
108. Schlagenhauf U, Jockel-Schneider Y. Probiotics in the management of gingivitis and periodontitis. A review. Front Dent Med. (2021) 2:1–11. doi: 10.3389/fdmed.2021.708666
109. Gagliardi A, Totino V, Cacciotti F, Iebba V, Neroni B, Bonfiglio G, Schippa S. Rebuilding the gut microbiota ecosystem. Int J Environ Res Public Health. (2018) 15(8):1–24. doi: 10.3390/ijerph15081679
110. Hill C, Guarner F, Reid G, Gibson GR, Merenstein DJ, Pot B, Sanders ME. Expert consensus document. The international scientific association for probiotics and prebiotics consensus statement on the scope and appropriate use of the term probiotic. Nat Rev Gastroenterol Hepatol. (2014) 11(8):506–14. doi: 10.1038/nrgastro.2014.66
111. Wang X, Zhang P, Zhang X. Probiotics regulate gut microbiota: an effective method to improve immunity. Molecules. (2021) 26(19):1–15. doi: 10.3390/molecules26196076
112. Raftar SKA, Ashrafian F, Abdollahiyan S, Yadegar A, Moradi HR, Masoumi M, Zali MR. The anti-inflammatory effects of Akkermansia muciniphila and its derivates in HFD/CCL4-induced murine model of liver injury. Sci Rep. (2022) 12(1):2453. doi: 10.1038/s41598-022-06414-1
113. Wieers G, Belkhir L, Enaud R, Leclercq S, Philippart de Foy JM, Dequenne I, Cani PD. How probiotics affect the microbiota. Front Cell Infect Microbiol. (2020) 9:1–9. doi: 10.3389/fcimb.2019.00454
114. Spacova I, Ceuppens JL, Seys SF, Petrova MI, Lebeer S. Probiotics against airway allergy: host factors to consider. Dis Model Mech. (2018) 11(7):1–13. doi: 10.1242/dmm.034314
115. Montalto M, Vastola M, Marigo L, Covino M, Graziosetto R, Curigliano V. Probiotic treatment increases salivary counts of lactobacilli: a double-blind, randomized, controlled study. Digestion. (2004) 69(1):53–6. doi: 10.1159/000076559
116. Haukioja A. Probiotics and oral health. Eur J Dent. (2010) 4(3):348–55. doi: 10.1055/s-0039-1697851
117. Allaker RP, Stephen AS. Use of probiotics and oral health. Curr Oral Health Rep. (2017) 4(4):309–18. doi: 10.1007/s40496-017-0159-6
118. Nascimento MM. Oral microbiota transplant: a potential new therapy for oral diseases. J Calif Dent Assoc. (2017) 45(10):565–8. Available from: https://www.ncbi.nlm.nih.gov/pmc/articles/PMC5828680/29497269
119. Mira A, Simon-Soro A, Curtis MA. Role of microbial communities in the pathogenesis of periodontal diseases and caries. J Clin Periodontol. (2017) 44(Suppl 18):S23–38. doi: 10.1111/jcpe.12671
120. Pozhitkov AE, Leroux BG, Randolph TW, Beikler T, Flemmig TF, Noble PA. Towards microbiome transplant as a therapy for periodontitis: an exploratory study of periodontitis microbial signature contrasted by oral health, caries and edentulism. BMC Oral Health. (2015) 15:125. doi: 10.1186/s12903-015-0109-4
121. Federici S, Nobs SP, Elinav E. Phages and their potential to modulate the microbiome and immunity. Cell Mol Immunol. (2021) 18(4):889–904. doi: 10.1038/s41423-020-00532-4
122. Paule A, Frezza D, Edeas M. Microbiota and phage therapy: future challenges in medicine. Med Sci. (2018) 6(4):1–10. doi: 10.3390/medsci6040086
123. Hsu BB, Gibson TE, Yeliseyev V, Liu Q, Lyon L, Bry L, Gerber GK. Dynamic modulation of the gut microbiota and metabolome by bacteriophages in a mouse model. Cell Host Microbe. (2019) 25(6):803–14.e5. doi: 10.1016/j.chom.2019.05.001
124. Sun Y, Feng B. Inflammation response of phage-based films on titanium surface in vitro. FASEB J. (2017) 31(S1):657–715. doi: 10.1016/j.actbio.2017.06.019
Keywords: oral microbiome, allergy, local inflammation, systemic inflammation, microbiota, oral microbiota
Citation: Moreno CM, Boeree E, Freitas CMT and Weber KS (2023) Immunomodulatory role of oral microbiota in inflammatory diseases and allergic conditions. Front. Allergy 4:1067483. doi: 10.3389/falgy.2023.1067483
Received: 11 October 2022; Accepted: 26 January 2023;
Published: 17 February 2023.
Edited by:
Gerald Tannock, University of Otago, New ZealandReviewed by:
Maria M. Escribese, CEU San Pablo University, SpainRandi Jacobsen Bertelsen, University of Bergen, Norway
© 2023 Moreno, Boeree, Freitas and Weber. This is an open-access article distributed under the terms of the Creative Commons Attribution License (CC BY). The use, distribution or reproduction in other forums is permitted, provided the original author(s) and the copyright owner(s) are credited and that the original publication in this journal is cited, in accordance with accepted academic practice. No use, distribution or reproduction is permitted which does not comply with these terms.
*Correspondence: K. Scott Weber c2NvdHRfd2ViZXJAYnl1LmVkdQ==
†ORCID K. Scott Weber orcid.org/0000-0003-3688-2191
Specialty Section: This article was submitted to Infections and Microbiome, a section of the journal Frontiers in Allergy