- 1Division of Pulmonology, Allergy-Immunology, and Sleep, Department of Pediatrics, College of Medicine, University of Tennessee Health Science Center, Memphis, TN, United States
- 2Children's Foundation Research Institute, Le Bonheur Children's Hospital, Memphis, TN, United States
Asthma is a common chronic respiratory disease that affects millions of people worldwide. Patients with allergic asthma, the most prevalent asthma endotype, are widely considered to possess a defective immune response against some respiratory infectious agents, including viruses, bacteria and fungi. Furthermore, respiratory pathogens are associated with asthma development and exacerbations. However, growing data suggest that the immune milieu in allergic asthma may be beneficial during certain respiratory infections. Immunomodulatory asthma treatments, although beneficial, should then be carefully prescribed to avoid misuse and overuse as they can also alter the host microbiome. In this review, we summarize and discuss recent evidence of the correlations between allergic asthma and the most significant respiratory infectious agents that have a role in asthma pathogenesis. We also discuss the implications of current asthma therapeutics beyond symptom prevention.
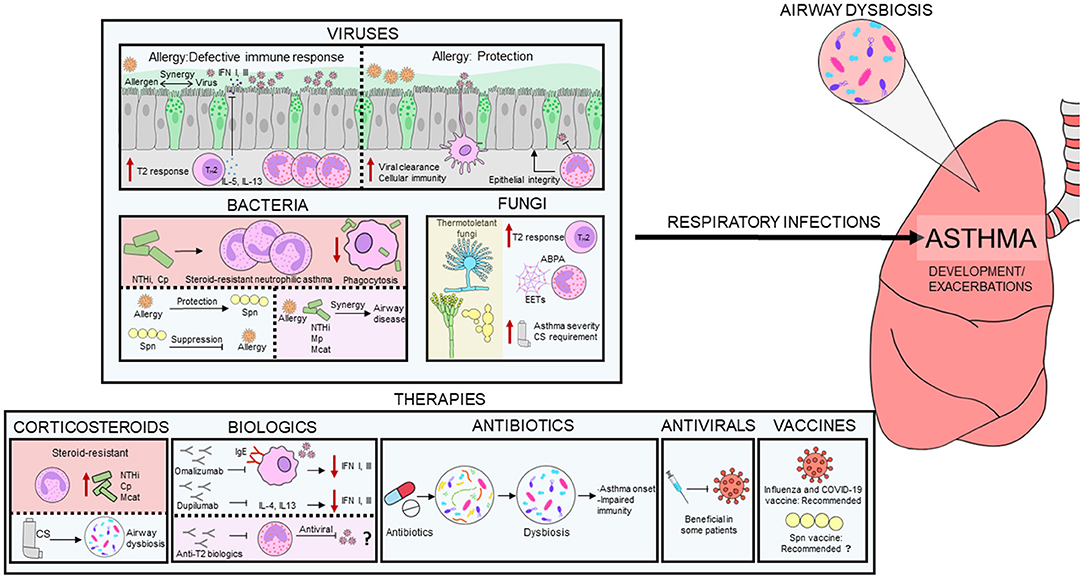
Graphical Abstract. Interplay between infectious agents and allergic milieu. Respiratory infections caused by viruses, bacteria and fungi play an important role in asthma pathogenesis. The immune milieu in allergic asthma may be both defective and protective during respiratory infections. Some bacteria are linked to steroid-resistant neutrophilic asthma and an aberrant immune response. Thermotolerant fungi generally induces a T2 immune response in asthma and are linked to asthma severity and higher corticosteroid requirement. Steroid-resistant neutrophilic asthma is associated with increased airway bacterial burden and reduced bacterial diversity. Corticosteroids and antibiotics induce dysbiosis in asthmatics, which may cause immune system alterations. Biologics and antivirals may be beneficial in some patients. However, the effect of eosinophil depletion on antiviral immunity in asthmatics remains unknown. Influenza and COVID-19 vaccination are recommended in asthmatics, but pneumococcal vaccine benefits are still under debate.
Introduction
Chronic conditions such as diabetes, obesity, cancer and illnesses affecting the heart, lungs, brain, and kidneys plague modern society. In fact, the Centers for Disease Control and Prevention (CDC) estimate that 60% of adults in the United States have at least one chronic disease while 40% have two or more (1). Afflicting over 300 million people worldwide, asthma is indeed a common chronic condition of the pulmonary system with clear nexus between genetic and environmental factors. The term “asthma” yields >2.5 million hits on Google and over 200,000 articles on PubMed (at writing) indicating that it is a major topic of interest to the lay public and scientists alike. Despite centuries of characterization and garnering knowledge on initiation and pathogenesis of this condition, a cure remains elusive. As a component of the atopic march, asthma develops in early childhood, can affect individuals throughout life (2), and may overlap with chronic obstructive pulmonary disease (COPD) with age (3). Considered a syndrome, asthma is mainly endotyped as type 2 (T2) and non-T2 based on immune bias towards TH2-type immune profile. Asthma symptoms allow for further classification based on severity ranging from mild to severe (4). Of the T2 endotype, allergen-induced eosinophilic (allergic) asthma is the most prevalent form resulting from sensitization to airborne environmental allergens, has high incidence, occurs across the ages, and correlates with other chronic conditions like obesity, and therefore will be the focus in this review.
Proposed in 1989, the hygiene hypothesis suggested that early childhood infections are protective against allergic diseases later in life (5). Among the studies of the protective influence of farming exposure in allergy, the Amish and Hutterites studies stand out, as they demonstrate that certain microbial exposures shield against asthma development (6). However, it is evident that not all microbial exposures are protective, as some viruses and bacteria are associated with asthma development instead (7–11).
Indoor and outdoor air are brimming with innocuous and pathogenic infectious agents (12, 13). Despite physical, secreted, and cellular pulmonary defenses in place, some environmental agents infiltrate these safeguards and cause disease (14). This may be particularly problematic in asthma, as the epithelial cell barrier in asthmatics is disrupted, thus facilitating the entrance of allergens and pathogens (15). Moreover, the levels of anti-inflammatory and immunoregulatory factors produced by airway epithelial cells including secretoglobin (SCGB)1A1 are decreased in patients with asthma (16), and airway epithelial cells in asthmatics contain micro-RNA (miRNA) changes implicated in regulation of epithelial cell differentiation (17). The role of the airway epithelium during T2 immune disease has been reviewed extensively (18–20) and therefore will not be the focus of this review.
With anti-inflammatory and pro-reparative functions (21), the TH2 immune profile in allergic asthma is commonly considered to be incompetent toward intra- and extra-cellular environmental pathogens like viruses, bacteria, and fungi (22). However, the growing incidence of asthma despite seasonal and pandemic respiratory infections indicate that this immune bias in asthmatics could be either host-protective or pathogen-tolerant. Herein, we explore the literature over the past 15 years focused on the correlations between common respiratory infectious agents and allergic asthma, as hosts with T2 asthma may hold hitherto unidentified anti-pathogen properties that may be of benefit to the modern-day patient.
Functional Impact of Viral Infections on Asthma Pathogenesis
Respiratory viral infections are a leading cause of morbidity and mortality worldwide in pediatric and adult populations with infection severity varying from asymptomatic or mild upper airway infections to bronchiolitis or pneumonia (23). The airway epithelium plays an important role as a first barrier to prevent unrestricted access to environmental pathogens while serving as an initiator of immune responses in the lungs (14, 24). However, allergens and viruses are able to disrupt the epithelial barrier thereby facilitating allergen sensitization and increasing infectious susceptibility (20, 25). In particular, viruses cause junctional protein dysfunction by inducing morphological alterations of epithelial cells such as cytopathic effect or formation of syncytia (18). Respiratory RNA viruses of the families Paramyxoviridae (respiratory syncytial virus, parainfluenza virus and metapneumovirus), Orthomyxoviridae (influenza virus), Picornaviridae (rhinovirus) and Coronaviridae (coronavirus) are the most common cause of asthma exacerbations (26), being associated with approximately 80% of exacerbation episodes in both children and adults (27). In the next section we summarize and discuss the current understanding of asthma and its relationship with the most important respiratory viral infections (Figure 1).
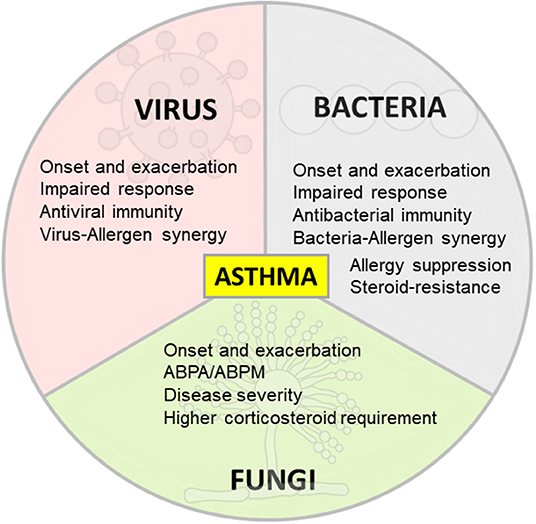
Figure 1. Differing effects of respiratory infections on asthma. Viral, bacterial and fungal respiratory infections are associated with asthma onset and exacerbation. The immune response in allergic asthma is defective against respiratory viruses and bacteria in some scenarios and promote antiviral and antibacterial immunity in other contexts. Allergy and respiratory pathogens may also synergize to increase inflammation and damage in asthma. On the contrary, some bacteria are able to suppress allergic inflammation. Specific bacteria are linked to T2-low asthma, steroid resistance and immune response impairment. Fungal sensitization/infection in allergic asthma is associated with disease severity and higher corticosteroid requirement.
Respiratory Syncytial Virus (RSV)
Paramyxoviridae family member RSV, is an enveloped negative-sense single-stranded (ss) RNA virus that invades ciliated bronchial epithelial cells using G and F proteins on its envelope (28). Being a major cause of respiratory tract (RT) infections in pediatric populations, RSV is the most common viral cause of pneumonia with peak incidences during winter (29, 30). Globally, RSV causes 33 million episodes of acute lower respiratory infection, leading to ~3.2 million hospitalizations and up to 200,000 deaths in children <5 years old per year (31). Moreover, many of the admitted children develop acute respiratory distress syndrome (ARDS), an acute life-threatening pulmonary condition (32). Additionally, there is a well-established association between severe RSV bronchiolitis in early life and asthma development in later childhood (7), where both the timing (infection during infancy) and the severity (hospitalization) are important predictors of asthma development (33). This association is more significant in sensitized children, suggesting a synergy between allergy and RSV to promote later asthma (34). However, RSV prevention in healthy preterm infants do not reduce clinically relevant asthma symptoms at 6 years old age (35).
Allergy may induce a defective antiviral immune response in asthmatics as peripheral blood mononuclear cells (PBMC) from asthmatic allergic adults secrete less interferon (IFN)-α compared to healthy controls (36). Furthermore, eosinophils in asthmatics have a reduced ability to bind and possibly inactivate RSV compared to healthy controls (37). In contrast, mouse models have shown a protective role of allergy during RSV infections. Aspergillus fumigatus-sensitized and challenged mice infected with pneumonia virus of mice [a virus related to RSV inducing similar pathology as severe RSV infection in children (38)], were protected of lethal infection (39), an effect mediated by recruited eosinophils. Interestingly, OVA-allergic animals were not protected when infected with this virus (39), suggesting that allergen-induced protection from respiratory viral disease may be strongly dependent on immunogenic properties of the allergen.
Parainfluenza Virus (PIV)
Members of the Paramyxoviridae family like PIVs are enveloped, negative sense, ssRNA viruses that utilize hemagglutinin neuraminidase glycoprotein for host cell attachment and F protein for viral fusion (40). PIV has a type-specific pattern of seasonal circulation wherein PIV1 peaks in autumn and PIV3 during the spring-summer (29). PIVs are a major cause of respiratory infections in immunocompromised patients and infants. In fact, PIV is the second most common cause of acute RT infection among children <5 years, just after RSV (41).
Similar to its protective effect during RSV infections, allergy confers host defense against PIV infection. OVA-allergic mice infected with PIV have reduced viral RNA in the lungs compared to non-sensitized mice due to antiviral effects of eosinophilic nitric oxide (42). Nonetheless, as PIVs trigger asthma exacerbations, it is possible that eosinophils, despite their antiviral activity, exaggerate TH2 immune responses in asthmatics after PIV infection (42).
Human Metapneumovirus (hMPV)
Another member of the Paramyxoviridae family, hMPV was first isolated in 2001 from children with RT infections (43, 44). The F protein binds to cell surface integrin αvβ mediating membrane fusion (45). With annual rates of hospitalization of 1 per 1000 children <5 years and 3 per 1000 infants <6 months in age, hMPV is a significant health threat to the pediatric population (46). Clinical features of hMPV infection are similar to other respiratory viruses, including upper RT symptoms like rhinorrhea and cough, and lower respiratory illnesses such as pneumonia and bronchiolitis (43).
Similar to RSV, hMPV infections are associated with asthma exacerbations (47) and children hospitalized with hMPV are more likely to have asthma (46). Moreover, hMPV has been linked to asthma development in children (8) and hMPV infection induces longterm pulmonary inflammation and airway hyperresponsiveness (AHR) in experimental models (48). In contrast to reduced IFN production by epithelia from asthmatics in response to human rhinovirus (49), nasal and tracheal epithelial cells from atopic individuals with wheeze or asthma do not display defective type I or III IFN responses after hMPV infection (50, 51). However, nasal epithelial cells from subjects with mild-to-moderate asthma show elevated hMPV replication compared with infection in cells from healthy individuals, a mechanism mediated by apoptosis inhibition via heat shock protein 70 (51).
Influenza Virus
Influenza viruses are enveloped viruses with a negative sense segmented ssRNA genome, belonging to the Orthomyxoviridae family (52). Influenza virus hemagglutinin binds to sialic acid residues on host cells for viral entry, while neuraminidase cleaves sialic acid to release virions (53). Of the four types of influenza virus, A, B, C and D, influenza A virus (IAV) is the most common and pathogenic (54). Annually, IAV causes seasonal epidemics with ~3-5 million cases of severe respiratory illness and around 650,000 deaths worldwide (55), in addition to global pandemics (56).
Allergic inflammation is traditionally associated with influenza immunity. Similar to human rhinovirus (57), plasmacytoid dendritic cells (pDCs) from allergic asthmatics secrete less IFN-α after influenza A or B exposure (58). Furthermore, FcεRI cross-linking on pDCs before virus challenge interferes with IFN-α secretion, TLR7 expression and virus-induced upregulation of pDC co-stimulatory molecules (58). In contrast to the immune protection from seasonal IAV immunized non-allergic mice, immunized allergic mice are susceptible to infection with pandemic IAV (59). However, other experimental and epidemiological studies have shown a different scenario. During the 2009 influenza pandemic, patients with chronic diseases (including asthma) were among the most commonly hospitalized (60) albeit with less severe outcomes related to viral infection compared with non-asthmatics (61). Bronchial epithelia from asthmatics were resistant to IAV-cytopathology and did not have a defective IFN response compared to cells from health donors (62). Experimental models of allergic asthma and influenza have effectively recapitulated that allergic immunity protects the host from severe influenza (62–68). This protective effect has been attributed to enhanced NK cell activation (63), CD8+ T cell support provided by eosinophils (62, 65), transforming growth factor (TGF)-β1-induced reduction of inflammation (64), CD11b+ DCs (67), and most recently, eosinophil-mediated enhancement of epithelial barrier responses (68). The timing of IAV infection in relation to asthma induction and the state of the allergic airways during infection are crucial for disease outcome. The induction of allergic airway inflammation in formerly IAV-infected mice generated enhanced lung pathology (66) while pre-existing allergic airway inflammation was protective from upcoming IAV infection (66). Infection with IAV during acute allergic inflammation with eosinophilia leads to better outcomes (maintenance of body weight and epithelial barrier and quicker viral clearance) compared to infection during the remodeling phase of allergic asthma (62). These host responses may be site specific as nose-only allergen stimulation and subsequent IAV infection results in increased influenza morbidity and mortality compared to controls (69), a finding that is contrasting to aforementioned studies performed in lower airway inflammation mouse models.
Human Rhinovirus (hRV)
Genetically classified as types A, B, and C, hRV is a non-enveloped positive sense ssRNA virus belonging to the Picornaviridae family (70). hRV-A and hRV-C are particularly relevant as they are more frequent and cause a more severe respiratory illness in infants compared to hRV-B (71). Most hRV-A and -B serotypes bind to intracellular adhesion molecule-1 receptors on host cells for infection; the minor serotypes bind to the low-density lipoprotein receptor (70). On the other hand, hRV-C attaches to cadherin-related family member 3 (72). Clinical symptoms of hRV infection range from asymptomatic and mild self-limiting in immunocompetent hosts, to more severe manifestations such as bronchiolitis and pneumonia in infants and the immunosuppressed (70). While hRVs cause respiratory illness throughout the year they are most frequent during spring and autumn, and disease severity increases in winter (71). Importantly, hRV infection is the most common trigger of viral asthma exacerbations (73), especially hRV-C, which causes the greater number of asthma attacks in children, often with greater severity than hRV-A or hRV-B (74). Furthermore, a clear relationship is established between early life hRV-induced wheezing and asthma development in later childhood (7, 9).
Allergic sensitization may be a risk factor for wheezing during hRV infection (73, 75). Compared to non-atopic asthmatic children, atopic asthmatic children are more likely to present severe viral disease and loss of asthma control after hRV infection (75). Infants and children admitted for wheezing from hRV have higher levels of serum IgE compared to non-wheezing controls and 84% of children with wheezing were sensitized to at least one aeroallergen (73). Furthermore, allergic individuals may have impaired antiviral immunity to hRV. In vitro studies have shown that the epithelial inflammatory response to hRV of asthmatics is abnormal and is associated with increased viral replication and virus-induced cytotoxicity (76). Individuals with allergic asthma have baseline differences in gene expression compared to healthy controls including a decreased expression of viral replication inhibitors and gene dysregulation following hRV infection (77). Moreover, patients with atopic asthma have impaired IFN type I and III responses during hRV infection, although not all studies are in agreement (49). Anti-T2 therapies may be beneficial for such patients, to reduce hRV-induced viral exacerbations and restore impaired antiviral responses (49).
Coronavirus (CoV)
Coronaviruses are enveloped, positive sense ssRNA viruses belonging to the Coronaviridae family. The membrane protein (M) and the envelope protein (E) participate in virus assembly, whereas the spike protein (S) mediates viral entry (78). A novel CoV infection outbreak in Wuhan China occurred in 2019 and rapidly spread across the world causing 290 million infections and 5.4 million deaths to date (79), and is now the most severe pandemic of the 21st century. Perhaps because CoVs are able to induce asthma exacerbations (80) and because some asthmatics have deficiencies in antiviral immunity (49), patients with moderate-to-severe asthma were listed at the beginning of the pandemic to be at risk for severe coronavirus disease (COVID) (81). However, the global initiative for asthma (GINA) reported that people with asthma do not seem to have an increased risk of infection from severe acute respiratory syndrome (SARS)-CoV-2, the causative agent of the ongoing COVID-19 pandemic, or present with severe COVID-19 (4). Early findings suggest that like during the 2009 influenza pandemic T2-high asthma endotype may be protective in COVID-19 infection (82). Patients with asthma with absolute eosinophil counts (AEC) ≥150 cells μL are less likely to be admitted (83). Several hypotheses have been presented to date to explain these findings. Patients with allergic asthma have lower expression of angiotensin-converting enzyme (ACE)-2, the primary receptor for SARS-CoV-2 (84), raising the possibility of a reduced risk of SARS-CoV-2 infection. The anti-inflammatory effect of inhaled corticosteroids (ICS) and T2 cytokines have also been proposed as explanations (85). Another possibility is eosinophil antiviral activity (86), which has been reported to other ssRNA respiratory viruses (87, 88). In fact, eosinopenia correlates to poor outcome in patients with COVID-19, and the restoration of eosinophil numbers is linked to disease improvement (89) and T2-low asthma endotype may correlate with severe COVID-19, as IL-17 (a cytokine that participates in T2-low asthma pathogenesis) drives the immunopathogenesis of ARDS in patients with severe COVID-19 (82).
Impact of Bacterial Infections in Asthma Pathogenesis
Bacterial infections are associated with pathogenesis, exacerbations and chronicity of asthma (90, 91). The knowledge of the relationship between bacteria and asthma continue to grow rapidly, in part due to the utilization of new technologies for bacterial identification, particularly 16S ribosomal RNA gene sequencing (92). Appreciation of the human microbiome, especially the lung microbiome [considered sterile until recently (93)], allowed researchers to recognize the impact of specific bacteria in different pulmonary disorders (94) and their interactions with viral infections in the context of asthma (95). In the following section we discuss the participation of the most significant bacteria linked to asthma (Figure 1).
Streptococcus pneumoniae (Spn)
Pneumococcus is a Gram-positive bacterium with a polysaccharide capsule that plays a critical role in its virulence (96) and is the most common cause of bacterial pneumonia in children (30). Invasive pneumococcal disease [includes bacteremic pneumonia, meningitis, and bacteremia (97)] is a more serious manifestation of Spn infection which usually causes otitis media, sinusitis and bronchitis (98, 99). Antimicrobial resistance in pneumococci is an ongoing problem and about one million children die of pneumococcal disease every year (99).
As a commensal in the upper RT, a large proportion of the population, including asthmatics, carries Spn asymptomatically (98, 100, 101) albeit carriage is more common in asthmatics (101). Infants with higher Streptococcus abundance in the nasopharynx are more likely to wheeze at 5 years of age (9, 10). Additionally, Spn infection is linked to asthma exacerbations (102) and its colonization increases in asthmatics that experienced recent exacerbations (103, 104).
Immune responses to Spn in allergic hosts may be age-dependent as neonate Spn-infected mice had elevated airway neutrophils, more severe lung inflammation, enhanced AHR, and increased IL-17A production after OVA sensitization and challenge in adulthood (105). In contrast, Spn infection in adult mice before OVA challenge induces a regulatory T cell (Treg) influx, which correlates with suppression of allergic airways inflammation (105, 106). Infection or treatment with killed Spn or its components reduce OVA-induced eosinophilic inflammation, T2 cytokine release, mucus hypersecretion and AHR (106–110). Furthermore, pneumococcal conjugate vaccine suppressed the critical features of allergic airways inflammation when administered intranasally through induction of Tregs (111) and pharyngeal Spn colonization suppresses the pathophysiology during acute asthma exacerbations in children (112).
Allergic airways inflammation can also play a protective role against Spn lung disease. OVA- or HDM-induced allergic lung inflammation confers protection against an otherwise lethal pulmonary pneumococcal infection with reduced bacterial burden and neutrophils (113). Aspergillus fumigatus-induced allergic mice infected with Spn survived the infection as opposed to over 50% mortality in controls potentially through IL-6 regulation of airway barrier integrity (114). In contrast, HDM-induced allergic mice are unable to mount an effective antibacterial response, as allergy impairs neutrophil recruitment resulting in bacterial invasion and dissemination (115). These differences in antibacterial immunity may be due to methodological variations such as the use of different allergen models, different Spn serotypes, and infectious doses and regimens used (116).
Haemophilus influenzae (Hi)
Haemophilus species are Gram-negative coccobacilli broadly classified into typeable (encapsulated) and non-typeable (non-encapsulated) strains. Encapsulated bacteria are further subtyped (a through f) by capsule antigenicity (117). While H. influenzae type b (Hib) was one of the most frequent causes of lower respiratory infection, its incidence has decreased largely due to vaccination, while that of non-typeable H. influenzae (NTHi) has increased (118). A variety of clinical manifestations such as otitis media, sinusitis, conjunctivitis and pneumonia can be caused by NTHi especially in children. Newborns or immunocompromised individuals can also present with invasive infections, including bacteremia and meningitis (119).
As commensals of the lower RT, Haemophilus spp. can be found in healthy individuals (120). However, Hi is more frequently associated in patients with asthma (93) and Hi colonization in neonates is a risk factor of asthma development early in life (10). Experimental studies have confirmed this observation wherein NTHi infection in 3-day-old mice increases granulocyte infiltration, elevated mucus production, T2 cytokines and AHR following OVA-challenge (121).
There are multiple associations between NTHi infection and neutrophilic asthma. Infection of mice with NTHi during OVA-induced inflammation suppresses T2-mediated eosinophilic inflammation and while enhancing neutrophilic inflammation through IL-17 (122). Furthermore, the combination of NTHi infection and allergic airways inflammation resulted in a steroid-resistant disease, a feature that resembles neutrophilic asthma in humans (123). In accord, Hi (124, 125) or members of the Haemophilus genera (126, 127) have been found in sputum of patients with neutrophilic asthma. Interestingly, the combination of Hi infection and allergic inflammation in mice impairs airway macrophage and neutrophil activation resulting in chronic bacterial infection (123). Similarly, impaired alveolar or monocyte-derived macrophage phagocytosis of Hi has also been reported in patients with severe asthma (128).
Moraxella catarrhalis (Mcat)
As a Gram-negative diplococcus human-restricted commensal of the upper RT, Mcat is pathogenic to both upper and the lower RTs (129, 130). Also functioning as a causative agent of acute otitis media in children, Mcat is a frequent cause of COPD exacerbations in adults (130). Besides its association with asthma development (10), Mcat colonization is linked to loss of asthma control (131) and wheezing episodes (112, 132). Moreover, Moraxella is the reported dominant species in nasal passages of children who develop asthma exacerbations, is stably maintained in their airways (133), and found more commonly in acute respiratory infections (9).
Both neutrophilic and eosinophilic inflammation can result after Mcat infection (95). In patients with neutrophilic asthma, high abundance of Moraxella and Haemophilus taxa have been identified in sputum samples (126, 127). Moreover, sputum neutrophils positively correlate with the relative abundance of Moraxella (127), and Mcat colonization have been associated with prolonged and more severe airway obstruction in treatment resistant severe asthma (126). The relative abundance of upper RT Moraxella species correlates positively with systemic and airway eosinophilia (134) and elevated levels of eosinophil cationic protein in nasal samples (133). PBMCs from asymptomatic infants that developed asthma by age 7 secrete higher levels of T2 cytokines (IL-5 and IL-13) and IL-17 when exposed to Mcat or NTHi (135), and Mcat promotes IL-8 and IL-33 gene upregulation in A549 human alveolar epithelial cells (133). Infection during HDM sensitization in mice induced airway neutrophilia, eosinophilia, and IFN-γ+, IL-17+, and IL5+/IL13+ T CD4+ cells, in addition to goblet cell hyperplasia and mucus production compared to allergic mice without Mcat infection (136).
Mycoplasma pneumoniae (Mp)
Mycoplasma species possess unique characteristics. They are the smallest prokaryotes, which allow them to pass through cell filters and because they lack cell walls they are insensitive to cellular antimicrobial agents and Gram-staining (137, 138). A member of the Mycoplasmataceae family, Mp predominates among disease causing Mycoplasma species (138). While Mp infections can occur worldwide at any time of the year, incidence is higher in summer or early autumn (139). Like many other respiratory pathogens, Mp can asymptomatically colonize the RT (140) but have the ability to cause upper and lower respiratory complications including pneumonia (139, 141) and may be responsible for 4–8% of community-acquired bacterial pneumonias, with increases up to 70% during epidemics, affecting groups of all ages, especially children and young adults (141).
The association between Mp and asthma is longstanding where Mp infections have been linked to asthma inception (11) and exacerbations (139). Community-acquired respiratory distress syndrome (CARDS) toxin produced by Mp has been reported to induce allergic airways inflammation in mice, characterized by eosinophilia, mucus production and T2 cytokine secretion (142). Moreover, history of asthma and atopic sensitization are risk factors for refractory Mp pneumonia requiring steroid therapy in children (143) and Mp detection is involved with worsening chronic asthma (140).
Multiple experimental studies have evaluated the role of Mp infection in allergic asthma. Low dose Mp infection enhances IL-4 and eotaxin-2 expression in allergic mice (144) and CARDS toxin exacerbates asthma in OVA-induced allergic mice (145). In contrast, Mp infection before OVA challenge reduces airway mucin secretion through toll-like receptor (TLR)-2/IFN-γ signaling pathway (146). Surfactant protein A (SP-A), the most abundant of the pulmonary surfactant proteins, binds and opsonizes pathogens, including Mp (147). Interestingly, SPA−/− allergic mice infected with Mp have significantly decreased Mp burden compared to controls, a mechanism attributed to eosinophil-mediated killing of Mp, and limited by SPA (148). On the other hand, it has been reported that allergy impairs the immune response against Mp. OVA-allergic mice infected with Mp have higher bacterial burden than non-allergic mice due to inhibition of TLR2 expression and IL-6 production in lung cells (149), and reduced expression of bactericidal/permeability-increasing protein fold-containing family member A1 (150), a protein with antimicrobial properties against bacteria (151) including Mp (152).
Chlamydia pneumoniae (Cp)
The bacterial family Chlamydiaceae includes the human pathogen Chlamydophila pneumoniae, a Gram-negative obligate intracellular bacteria that is a common cause of acute respiratory infections (153). Pneumonia and bronchitis are the most common clinical manifestations, with approximately 10% of community-acquired pneumonia (CAP) cases and 5% of bronchitis cases (153).
Also associated with asthma exacerbations (154), early-life chlamydial infection enhances allergic characteristics in OVA-sensitized mice (155). Moreover, Cp is found more frequently in asthmatics (156) and the age at which Cp infection occurs seems to be crucial for asthma development as chlamydial infection during early-life (neonatal and infant), but not adult, increases IL-13 expression, mucus-secreting cell numbers and AHR (155). Moreover, infant infection, but not neonatal, increases airway eosinophilia, T2 cytokines and changes in hematopoietic cells, leading to more severe allergic airways disease in later life (155, 157).
Similar to NTHi, Cp-induced airway inflammation is predominantly T2-low. Current chlamydial infection during OVA-induced allergic disease induces neutrophil influx associated with TH1/TH17 immune responses while attenuating eosinophil recruitment and T2 response (158). Chlamydia-induced severe steroid-insensitive allergic airways disease in mice induces lung mRNA expression of TH1 and TH17 associated molecules (Tlr2, Stat1, Ifng, Cxcl9, Cxcl10, Tnf , Il17, Il6, Tgfb, and Il1b) and reduction of TH2 associated genes (Il5 and Il13) (159). Elevated IL-8 levels and airway lavage fluid neutrophils are reported in Cp positive asthmatic children (160), and Cp infection may drive increased steroid resistance (161).
Effects of Fungal Sensitization/Infection on Asthma Pathogenesis
As with viruses and bacteria, fungi participate in asthma development and exacerbations (162) despite considerably less information available regarding causation. The fungal microbiome, or mycobiome, has been increasingly appreciated as an important player in health and disease (162, 163), which is evidenced in asthma by the airway fungal alterations in asthmatics compared with healthy subjects (164–166). Fungi that participate in asthma are divided into thermotolerant (allergenic and potentially infectious), and not thermotolerant (mesophilic), which are allergenic but typically not infectious (167). In this segment, we summarize the current understanding of the relationship between the most important thermotolerant fungi and asthma pathology (Figure 1).
Aspergillus
The genus Aspergillus consists of a variety of ubiquitous opportunistic filamentous mold species although only some are human pathogens (168). Inhaled Aspergillus conidia are removed by the mucociliary escalator and resident alveolar macrophages in healthy individuals (169). However, Aspergillus conidia can germinate and cause invasive infection in immunocompromised individuals (169, 170).
Sensitization to Aspergillus species is associated with severe asthma (171, 172), and greater corticosteroid requirement (172). Sensitization to A. fumigatus is related to reduced lung function (173), bronchiectasis (174), and asthma exacerbations in children and adults (175). Aspergillus is implicated in epithelial barrier impairment as the alkaline protease 1 of A. fumigatus causes disruptions between airway smooth muscle cells and extracellular matrix and promotes AHR (176). Host defense against Aspergillus in asthmatics is predominantly associated with T2 responses. Mice sensitized and challenged with A. fumigatus conidia develop allergic pulmonary inflammation and AHR with elevated serum IgE and pulmonary IL-4 (177), inhalation of Aspergillus-associated proteases by naïve mice promotes airway eosinophilia through protease-activated receptor-2 engagement (178), and chitin promotes eosinophil recruitment (179, 180). Consistently, enrichment of Aspergillus in the airways is associated with T2-high asthma in humans (166).
Pulmonary aspergillosis can be divided in chronic pulmonary aspergillosis, invasive pulmonary aspergillosis, and allergic bronchopulmonary aspergillosis (ABPA) (181) that principally affects patients with cystic fibrosis and asthma (182). It is estimated that 9% of cystic fibrosis patients (183) and 2.5% of adult asthmatics (184) suffer from ABPA. Furthermore, nearly 35–50% of patients with cystic fibrosis (183) and 24% of patients with severe asthma (185) have sensitization to A. fumigatus. Although many fungi are associated with the disease, A. fumigatus is by far the most common cause of ABPA (186) due to its marked thermotolerance and small size and surface properties of its conidia that can reach terminal airways (187, 188). The inflammatory response in patients with ABPA is characterized to be T2-biased caused by hypersensitivity to A. fumigatus, which includes high levels of total serum IgE and peripheral eosinophilia (189). Eosinophil extracellular traps (EETs) have been identified in bronchial mucus samples of A. fumigatus positive asthmatics with ABPA (190, 191). However, since EETs do not affect fungal viability, it is possible that EETs contribute to ABPA pathology by the formation of sticky mucus and granule-mediated epithelial damage (190, 192). In addition, eosinophils contribute to elevated morbidity and decreased A. fumigatus clearance to invasive fungal infection in mice (179). In contrast, eosinophil antifungal activity has been demonstrated against Aspergillus species in experimental studies such that mouse eosinophils are important contributors of A. fumigatus clearance in vivo and are able to kill the fungus in vitro (193). Similarly, T2 allergic inflammation has a protective role against A. niger infection in mice, while in vitro experiments showed that eosinophils possess anti-fungal activity (194).
Penicillium
Penicillium species, members of the Trichocomaceae family, are common indoor fungi that can be found in a diverse range of habitats (187, 195). Exposure to Penicillium has been linked to asthma (187). Increased levels of Penicillium is associated with increased exacerbation of current asthma symptoms in children and adults (175). Individuals sensitized to P. chrysogenum and A. fumigatus have lower lung function compared to those sensitized to Candida albicans (a thermotolerant yeast) or non-thermotolerant fungi (196). Additionally, children with asthma sensitized to thermotolerant fungi, including Penicillium, have worse lung function, greater systemic corticosteroid requirement, higher total serum IgE and FeNO, and greater sputum eosinophils compared to asthmatic children not sensitized to thermotolerant fungi (197). As such, Penicillium species are significantly enriched in patients with asthma, particularly with atopic asthma (166).
Candida
Candida genus is composed of approximately 200 species, only few of them being implicated in human diseases (198, 199). Included in the mycobiome Candida is able to colonize the skin, oropharynx, genitourinary and gastrointestinal tracts (187, 198) and when pathogenic (as with C. albicans) can quickly progress from superficial mucosal manifestations to life-threatening systemic infections (198, 199). Candida species participate in allergic diseases such as atopic dermatitis and asthma (187), are common etiologic agents of allergic bronchopulmonary mycosis (ABPM) (200), and are linked to severe asthma (201).
A high relative abundance of Candida in neonatal stool samples is linked to an increased risk of atopy at 2 years and physician-diagnosed asthma at 4 years (202). The use of antibiotics, which has been associated with asthma development (discussed below), triggers bacterial and fungal imbalances resulting in immune dysregulation (162). In an experimental study, antibiotic treatment in allergen-induced airway inflammation resulted in C. parapsilosis overgrowth in the gut, which correlated with airway inflammatory cell influx (203). Candida overgrowth-induced plasma prostaglandin E2 promotes lung macrophage polarization to M2, resulting in enhanced allergic airway inflammation. Moreover, oral treatment with human-isolated C. albicans, C. glabrata or C. tropicalis after antibiotic treatment exacerbates airway inflammation (203). In addition to fungal proteases that are known to drive T2 responses and elicit airways disease (204), it was recently demonstrated that C. albicans peptide toxin candidalysin is able to induce allergic disease (205). Candidalysin activates platelets stimulating the release of Dickkopf-1 peptide, which in turn coordinates TH2 and TH17 development during C. albicans airway mycosis (205).
Cryptococcus
C. neoformans and C. gattii are the etiologic agents of cryptococcosis (206). Cryptococcus species possess a polysaccharide capsule that participates in their virulence and differentiate it from other pathogenic yeasts (207, 208). C. neoformans has a worldwide distribution and it is disseminated by bird droppings (206, 209). While healthy individuals are able to clear the fungi or establish an asymptomatic infection after inhalation of spores or fungal cells, Cryptococcus can either cause pneumonia (210) or disseminate causing conditions such as meningoencephalitis (206) in immunosuppressed patients.
C. neoformans can induce a TH2 polarization associated with a non-protective antifungal immunity (211, 212). C. neoformans infection exacerbates OVA-induced allergic inflammation in rats with increased eosinophils, IgE titers, goblet cells and AHR compared to controls (213). Following infection with C. neoformans, IL-13−/− mice show higher survival, lower fungal burden, lower mucus production and reduced AHR compared to IL-13Tg+ and wild-type mice (214). On the other hand, IL-4Rα−/− mice are protected to C. neoformans infection, depicted by 100% survival compared with 100% mortality of wild-type mice (215). IL-4Rα−/− mice show reduced lung fungal burden compared with controls, in addition to absence dissemination to the brain, and decreased allergic inflammation and AHR (215). Interestingly, it has recently been reported that C. pseudolongus is more abundant in patients with asthma than in healthy individuals, and may play a role in asthma pathogenesis, which requires further investigation (216).
Current Treatment Strategies for Asthma Symptom Prevention and Their Impact on Immunity to Respiratory Infections
Asthma is a multifaceted disease with a varied response to therapy. Although patients with asthma usually respond well to standard therapies, some patients continue to have persistent symptoms (217). Comorbid conditions (e.g. obesity), environmental triggers, and asthma phenotypes are important factors to consider when selecting the optimal therapy for personalized patient care (218, 219). Moreover, increased knowledge about functions of airway and gut microbiota in respiratory diseases (94) obtained in the last decade adds a new level of complexity to effective asthma treatment (Figure 2). In this section we summarize the current known information about asthma therapy and discuss unknowns and areas that could benefit from further research.
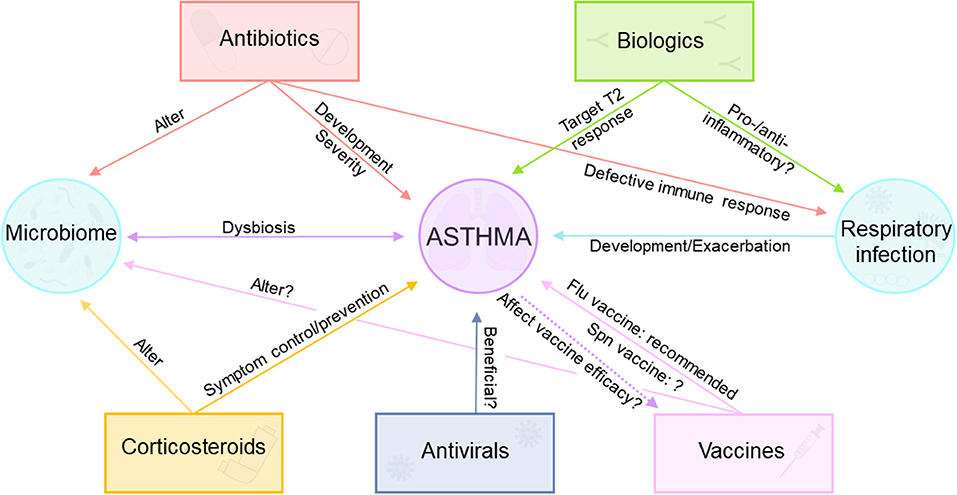
Figure 2. Complex interactions between asthma, asthma therapeutics, and vaccination. Corticosteroids are commonly used for asthma symptom control/prevention, but they alter the airway microbiome. Biologic therapy approved for asthma targets T2 immune response, but their effect during respiratory infections remains unclear. Antivirals may be useful for some asthma patients. Antibiotics cause alterations in the microbiome, are implicated in asthma development and severity, and cause impaired immune responses during viral and bacterial respiratory infections. Influenza vaccine is recommended for patients with asthma, although the usefulness of pneumococcal vaccination is controversial.
Corticosteroids
The use of inhaled (ICS) or systemic corticosteroids are recommended by the international asthma management guidelines for the control of asthma symptoms and exacerbations (4). In spite of being an effective treatment for most asthmatics, not all patients are responsive. In fact, patients with asthma can be clinically classified depending on the administered CS efficacy into steroid-sensitive and steroid-resistant patients (207). Importantly, CS can cause important adverse effects that can be life-term such as osteoporosis, diabetes, and respiratory infections (220). Furthermore, CS seem to decrease SCGB1A1 expression (221), and they only modestly correct the alterations of airway epithelial cell miRNA levels found in asthmatics (17). The transglutaminase 2, wingless/integrase 5a and secretory phospholipase A2 cascades have been associated with steroid resistance in normal human bronchial epithelial cells and nasal polyp tissues (222), therefore drugs targeting these pathways may be a personalized therapeutic choice for patients with steroid-resistance (222).
Neutrophils, unlike eosinophils, are resistant to CS-induced apoptosis (223), and therefore neutrophilic asthma is steroid-resistant (224). Significant differences in the microbiome of steroid-resistant and -sensitive asthmatics have been reported (225, 226). The baseline composition of bronchial bacterial microbiota from ICS responsive (enriched in Streptococcaceae, Fusobacteriaceae and Sphingomonodaceae) is different from ICS resistant patients (enriched in Microbacteriaceae and Pasteurellaceae) and more similar to healthy controls (226). In fact, a member of the Pasteurellaceae family, Haemophilus, is linked to steroid-resistant neutrophilic asthma (124, 225). In addition, Hi and Cp, bacteria related to neutrophilic phenotype, drive a steroid-resistant TH1/17-associated neutrophilic allergic airways disease in allergen sensitized mice (123, 159, 227). Furthermore, differences in the microbiome between asthma endotypes are reported (226, 228). Patients with neutrophilic asthma have increased airway bacterial burden and reduced bacterial diversity compared to non-neutrophilic asthmatics (228). Similarly, patients with COPD, a chronic disease that in general possess similarities with T2-low asthma phenotype such as airway neutrophilia (229), display reduced bacterial diversity, (230) and an increase in Haemophilus and Moraxella (231). Conversely, patients with T2-high asthma have lower bronchial bacterial burden than patients with T2-low asthma (226), and lower eosinophil counts are associated with increased airway bacterial load in COPD patients (232).
Augmenting differences in the airway microbiome among asthmatics, CS use is associated with significant changes on the composition (and probably diversity) of the airway microbiome in patients with asthma, COPD and chronic rhinosinusitis (233). Treatment with CS is associated with decreased relative abundance of Prevotella species and increased Pseudomonas species in asthmatics (234). Children with persistent asthma on regular ICS therapy are nearly four times more likely to have Spn oropharyngeal colonization compared to children not taking ICS (235). Steroid-sensitive asthmatics have an increased relative abundance of Neisseria and Moraxella species after ICS treatment (226). These studies suggest that CS use can affect the microbiome and neutrophilic inflammation and that the airway dysbiosis and steroid resistance are interrelated. However, as there is considerable heterogeneity between studies with respect to study cohorts, treatment duration, doses and type of evaluated CS (233), further studies are needed to clarify the impact CS have in airway dysbiosis during asthma and other respiratory diseases.
Biologics
Currently there are five Food and Drug Administration (FDA)-approved drugs – omalizumab (anti-IgE), mepolizumab and reslizumab (anti-IL-5), benralizumab (anti-IL-5Rα), and dupilumab (anti-IL-4Rα) – for moderate to severe asthma that can reduce asthma exacerbations in patients with T2-high asthma (236). These may also be efficacious during viral infections by improving the antiviral response. For example, children with allergic asthma treated with omalizumab have decreased duration and peak level of viral shedding during hRV infections, and ameliorated hRV illness (237). IgE receptor activation increases host susceptibility to viral infection and the use of omalizumab could be beneficial to improve the antiviral response in asthmatics (49). Intriguingly, IgE receptor activation on asthma donor pDCs reduces type I IFN secretion in response to IAV (58), and type I and III IFN release in response to hRV compared to pDCs from non-asthmatics (57). Furthermore, IgE cross-linking on PBMCs exposed to hRV from patients with asthma treated with omalizumab presented an IFN-α increased secretion compared with a placebo group (238). Dupilumab may also improve the antiviral immune response in patients with T2-high asthma, as IL-4 and IL-13 impair the viral-induced interferon production and TLR3 expression (239). In contrast, patients with mild asthma receiving mepolizumab and challenged with hRV have higher viral loads in nasal swabs compared to those that receive placebo, suggesting a protective role of T2 immune response against viral infection (240). This observation, along with the eosinophil antiviral activity against ssRNA viruses demonstrated in experimental studies mentioned above (39, 42, 62, 65, 68), raise the question whether eosinophil-targeted therapies may have a negative impact on viral disease and antiviral host defense. However, currently there are no specific clinical studies that demonstrate that anti-T2 biologics could be detrimental in patients with asthma. Moreover, GINA recommends continuing biologic therapy in patients with severe asthma during the COVID-19 pandemic (4).
Antivirals
Patients that possess defective IFN responses may benefit from type I and III IFN therapies (241) as these IFNs are able to suppress the T2 responses implicated in asthma (49). In fact, IFN therapies have demonstrated protective roles in experimental models of asthma (241, 242), and a randomized controlled trial suggests that inhaled IFN-β could be beneficial in virus-induced asthma exacerbations in severe asthmatics (243). TLR agonists have also demonstrated positive roles in asthma (244). Resiquimod, a TLR7 agonist, attenuates experimentally-induced allergic inflammation (245) and TLR-9 agonists have demonstrated improvement of asthma symptoms (246). Although palivizumab, a monoclonal antibody against the RSV fusion protein, may reduce subsequent recurrent wheezing in premature infants (247), GINA does not support its use since due to lack of evidence that its effect is sustained (4).
Antibiotics
Antibiotics are one of the most commonly prescribed medications for children (248), including those with asthma, of which in the United States about 17% of them are prescribed unjustifiably (249). Although antibiotic treatment is crucial against bacterial infections, and may seem attractive for asthma treatment given the implication of bacteria in asthma, they alter the healthy microbiome (250) including the mycobiome (203). This antibiotic-induced dysbiosis, besides the intrinsic gut and lung dysbiosis from asthmatics (94, 251), may cause immune system alterations that are linked to asthma and other pathologies (91, 250). Animal models have supported this concept by showcasing the positive correlation between antibiotic and antifungal treatment and enhanced asthma severity (203, 252–254). Moreover, several studies report an association between prenatal and early life antibiotic exposure in humans and increased risk of asthma development (251, 255–260). Additionally, antibiotic treatment causes longer hospitalization stays and higher costs (261), and have not proven to be beneficial in alleviating asthma exacerbations in adults (262). In fact, the current GINA guidelines do not support the routine prescription of antibiotics to treat asthma exacerbations (unless there is a strong evidence of lung bacterial infection) and recommend avoiding the prescription of broad-spectrum antibiotics during the first years of life (4). Despite this, antibiotics are still commonly used as a general treatment for asthma (263, 264).
Intestinal antibiotic-induced dysbiosis alters immune responses during respiratory bacterial and viral infections. Mice orally treated with broad-spectrum antibiotics and then intranasally infected with Spn have increased lung bacterial burden and accelerated mortality compared with non-treated mice (265). In another study, antibiotic-treated mice displayed impaired innate and adaptive antiviral immunity against IAV infection leading to severe influenza compared to untreated controls (266). Microbe-associated metabolites, like desaminotyrosine and acetate, enhance antiviral responses against IAV and RSV in mice through an upregulation of IFN signaling (267, 268). TLR stimulus provided by bacteria has also been demonstrated to be important to regulate immune responses against respiratory infections like IAV (269). Host protection noted in A. fumigatus-sensitized and challenged mice that were co-infected with IAV and Spn is lost after antibiotic-induced airway dysbiosis (270) highlighting the vast impact antibiotics have on pulmonary host defense. Consistent with these studies, children under antibiotic therapy in infancy may have impaired antiviral immunity later in life (271). Furthermore, a reduction of the bacterial genera Faecalibacterium, Lachonospira, Veillonella, and Rothia has a causal role in asthma development (251). Cumulatively, these studies demonstrate the importance of the gut-lung axis including the microbiome during respiratory infections and highlight the necessity to cease antibiotic misuse and overuse.
Macrolides, one of the most extensively used antibiotics, have been proposed as an attractive therapy for asthma due to its antimicrobial, immunomodulatory and possibly antiviral activities (272). In fact, clinical trials report that azithromycin therapy can reduce asthma exacerbations in adults (273–275), albeit with conflicting data (262) as airway dysbiosis in asthmatics may contribute to asthma pathogenesis (94). Bronchial brushings from asthmatics show a dominance of Proteobacteria, including families of potential pathogens such as Haemophilus and Moraxella (276), and this phyla was associated with epithelial expression of TH17-related genes and worse asthma control (277). A question that still remains is if certain antibiotics are able to equilibrate airway dysbiosis from asthmatics (278), therefore restoring the healthy microbiome. Unfortunately, antibiotics cannot differentiate between commensal and pathogenic bacteria, so pathogen-selective treatments are needed. However, due to antibiotic resistance (279), gut and airway microbiome alterations (280–282), and the aforementioned immunoregulation against respiratory pathogens, antibiotics are not ideal therapies for asthma in the longterm.
Allergen-Specific Immunotherapy (AIT)
There are a few options for the management of allergic diseases. Excluding allergen avoidance, which is not always practical or possible, conventional pharmacotherapy (e.g. anti-histamines, anti-leukotrienes, CS, etc.) and AIT are the other available options (283). Although pharmacotherapy can control allergic symptoms, they may reappear when medication is interrupted (284, 285). As a treatment based on the administration of increasing doses of clinically relevant allergens over a period of time, AIT represents a promising option through gradual desensitization and/or tolerance (4, 284), therefore providing a longterm solution. The induction of regulatory B cells and Treg and their products (IL-10 and TGF-β) are crucial to obtain tolerance during AIT (284). More recently AIT efficacy was shown to reduce allergic inflammation through SCGB1A1 induction (286). The first record of AIT is over a century old for the treatment of grass pollen-induced hay fever (287). Since then, the mechanisms behind AIT have been uncovered in some extent, and currently AIT is used for some allergic disorders including rhinitis, venom allergies, and allergic asthma (283). At present, there are two AIT approaches for allergic asthma, which includes subcutaneous immunotherapy (SCIT) and sublingual immunotherapy (SLIT) (4, 288), and SCIT has been shown to successfully alleviate asthma symptoms including bronchial hyperreactivity consequently decreasing the use of asthma medications (288). Although SLIT has demonstrated efficacy as an asthma treatment (289, 290), drawing conclusions is incumbered by the lack of data on outcomes such as exacerbation frequencies, pulmonary functions, quality of life, etc. (291). There are some important disadvantages of AIT such as discomfort from repeated injections, the prolonged time of therapy, lack of commitment in patients, absence of biomarkers that are able to predict the clinical outcome, and importantly, the possibility of life-threatening anaphylactic reactions (283). Additionally, it is important to consider that patients may not feel confident enough to discontinue CS or β-agonist treatments after AIT. Currently, GINA is reviewing evidence regarding AIT as a therapy for asthma, and the next update will cover those findings (4). Therefore, despite its promise as an immunologic solution to asthma, there may be substantial challenges in broad use implementation of AIT as a standard therapy for asthma (283).
Impact of Vaccinations on Asthma Development/Exacerbation
Influenza Vaccine
Seasonal influenza vaccines are necessary as circulating influenza strains regularly undergo antigenic drifts. There are two available influenza vaccine formulations in the United States, the inactivated influenza vaccines (IIVs) and live attenuated influenza vaccines (LAIVs) (292). Influenza vaccines are especially relevant during the SARS-CoV-2 pandemic to decrease the burden of respiratory illnesses and avoid hospital saturation (292, 293). Despite this, vaccination coverage in the United States in 2020-21 is nearly 20 percentage points lower than the target of 70% (293). Annual vaccination against influenza virus infection is recommended for all individuals aged ≥6 months who do not have contraindications, especially in populations at higher risk of infection including patients with asthma (4, 294). In fact, asthmatic children are 4-fold more likely to have seasonal influenza-associated hospitalizations than healthy children (295). Influenza vaccination has proven safety and efficacy in asthmatics, as it can reduce the risk of asthma exacerbations, healthcare use, respiratory illnesses, and medications for asthma (296). However, LAIV is contraindicated in wheezing children aged 2–4 years, and precautions should be taken in patients with asthma aged ≥5 years (294) since it was reported to increase the risk of wheezing episodes in infants vaccinated with LAIV compared to IIV (297). However, contraindication of LAIV in asthmatics is not clear since other studies have not reproduced these findings (298–301) and several studies showed that LAIV is safe in children and adults with asthma (301–303).
Pneumococcal Vaccination
Presently, the 13-valent pneumococcal conjugate vaccine (PCV13) and the 23-valent pneumococcal polysaccharide vaccine (PPSV23) are available in the United States (304). Although the CDC recommend pneumococcal vaccination in asthmatics (305), GINA argues against this due to data insufficiency (4). It has been documented that asthmatics have an increased risk for IPD susceptibility (306) although the influence of the different asthma endotypes is not clear. Interestingly, despite being vaccinated, children with asthma continue to have a higher risk for IPD compared to controls (307). Alternatively, no correlation between IPD-mediated mortality in asthmatics vs. non-asthmatics is reported (308) and asthmatics with CAP have a similar clinical outcome and shorter length of stay compared to the general population (264). Furthermore, asthmatics are not at increased risk of pneumococcal pneumonia hospitalizations compared to COPD patients (309). Recent experimental studies have demonstrated that allergic inflammation confers protection against Spn (113, 114, 270). Notably, the Spn-protected allergic mice displayed reduced levels of pro-inflammatory cytokines and lower levels of airway neutrophils compared to non-protected non-allergic mice (113). Given the heterogeneity of asthma, it is possible that patients with different endotypes show contrasting clinical outcomes to Spn infection. Varying other mechanisms may contribute to these findings. For example, COPD patients [also at high risk for IPD (308)], display reduced bacteria (310) and apoptotic cell (311, 312) phagocytosis. Similarly, macrophages from steroid-resistant severe asthmatics have defects in their phagocytic activity (128), and macrophage efferocytosis is impaired in patients with non-eosinophilic asthma (312). Thus, it is possible that patients with neutrophilic asthma or those on the asthma-COPD spectrum may be at higher risk of bacterial infections.
A subset of asthmatic children with high eosinophil count had poor antibody titers to Spn, even with complete PCV-13 immunization (313). Specific clinical and preclinical studies are necessary to determine the efficacy of pneumococcal vaccines in asthmatics as it is under-investigated, and studies are confounded by differences in age and other susceptibility factors like comorbidity. The need for boosters and their impact on asthma pathogenesis must also be addressed in greater depth. Therefore, the decision to administer pneumococcal vaccines to asthmatics, may need to be done at a more personalized level taking into consideration the type of asthma, other underlying conditions, smoking history, and immunomodulatory therapeutics. It is also important to consider that pneumococcal vaccination has an impact in the airway microbiome as Spn is found as a commensal in healthy subjects, and the eradication of Spn vaccine types may induce airway dysbiosis (314). For example, pneumococcal vaccination increases Hi carriage in healthy children (315, 316), and NTHi-mediated acute otitis media (317). Overall, microbial dysbiosis caused by pneumococcal vaccines is worthy of further investigation.
COVID-19 Vaccination
At the moment, there are 137 and 194 COVID-19 vaccines in clinical and preclinical development, respectively, and 10 vaccines approved for use by World Health Organization (318) with considerable protection against SARS-CoV-2 infection and disease (319). Currently, COVID-19 vaccination is internationally recommended for asthmatics (4) as vaccination rarely drives allergic reactions (4, 320). However, possible long-term implications of COVID-19 vaccines in asthmatics are currently unknown and require further investigation.
Conclusion
Immune response “flavor” at steady state is expected to differ between patients living with underlying chronic diseases and healthy hosts. Additional deviations of immune responses are to be expected when considering patients across the age spectrum. Therefore, invariant treatment strategies may not be optimally suited for the modern-day patient. Tests that permit the identification of asthma endotype (blood leukocyte panel, IgE levels, standard cytokine panel to include TH1, TH2, and TH17 cytokines, combined with the allergen identification) should be performed as standard care and results captured in medical records. Considering altered immune responses due to underlying chronic conditions, genetic profiles, and microbial signatures in addition to current parameters of age, sex, and race, hold promise to improve individualized patient care. Incorporating information regarding immune response attributes into standard treatment protocols may help reduce the overuse of immune-altering medications such as antibiotics and corticosteroids. Host-pathogen interactions that occur in patients with underlying allergic asthma when infected with common airborne pathogens are complex, multifaceted, and context dependent. Therefore, targeted studies are necessary to profile these patients for treatment regimens to influence and improve personalized, efficient healthcare with reduced drug burden during respiratory infections.
Author Contributions
The article was conceived by AES who also wrote the Introduction and conclusion. ASF-T wrote the first draft and drew the figures. Both authors participated in editing the paper and approved the final submission.
Funding
The Samarasinghe group supported in part by the National Institute of Allergy and Infectious Diseases of the National Institutes of Health under the Award number R01 AI125481 to AES, the Plough Foundation to AES, and the American Lung Association to AES.
Author Disclaimer
The content is solely the responsibility of the authors and does not necessarily represent the official views of the National Institutes of Health.
Conflict of Interest
The authors declare that the research was conducted in the absence of any commercial or financial relationships that could be construed as a potential conflict of interest.
Publisher's Note
All claims expressed in this article are solely those of the authors and do not necessarily represent those of their affiliated organizations, or those of the publisher, the editors and the reviewers. Any product that may be evaluated in this article, or claim that may be made by its manufacturer, is not guaranteed or endorsed by the publisher.
Acknowledgments
The authors wish to thank Darius J. Amos for literature search and helpful discussions on the section on fungi.
References
1. CDC. National Center for Chronic Disease Prevention and Health Promotion, Division of Population Health. BRFSS Prevalence & Trends Data. Available online at: https://www.cdc.gov/brfss/brfssprevalence/. (accessed January 06, 2022).
2. Hill DA, Spergel JM. The atopic march: critical evidence and clinical relevance. Ann Allergy Asthma Immunol. (2018) 120:131–7. doi: 10.1016/j.anai.2017.10.037
3. Gibson PG, Simpson JL. The overlap syndrome of asthma and COPD: what are its features and how important is it? Thorax. (2009) 64:728–35. doi: 10.1136/thx.2008.108027
4. Global nitiative for Asthma. Global Strategy for Asthma Management and Prevention, (2021). Available online at: http://www.ginasthma.org.
5. Pfefferle PI, Keber CU, Cohen RM, Garn H. The hygiene hypothesis - learning from but not living in the past. Front Immunol. (2021) 12:635935. doi: 10.3389/fimmu.2021.635935
6. Ege MJ. The hygiene hypothesis in the age of the microbiome. Ann Am Thorac Soc. (2017) 14:S348–53. doi: 10.1513/AnnalsATS.201702-139AW
7. Jackson DJ, Gangnon RE, Evans MD, Roberg KA, Anderson EL, Pappas TE, et al. Wheezing rhinovirus illnesses in early life predict asthma development in high-risk children. Am J Respir Crit Care Med. (2008) 178:667–72. doi: 10.1164/rccm.200802-309OC
8. García-García ML, Calvo C, Casas I, Bracamonte T, Rellán A, Gozalo F, et al. Human metapneumovirus bronchiolitis in infancy is an important risk factor for asthma at age 5. Pediatr Pulmonol. (2007) 42:458–64. doi: 10.1002/ppul.20597
9. Teo SM, Mok D, Pham K, Kusel M, Serralha M, Troy N, et al. The infant nasopharyngeal microbiome impacts severity of lower respiratory infection and risk of asthma development. Cell Host Microbe. (2015) 17:704–15. doi: 10.1016/j.chom.2015.03.008
10. Bisgaard H, Hermansen MN, Buchvald F, Loland L, Halkjaer LB, Bønnelykke K, et al. Childhood asthma after bacterial colonization of the airway in neonates. N Engl J Med. (2007) 357:1487–95. doi: 10.1056/NEJMoa052632
11. Yeh JJ, Wang YC, Hsu WH, Kao CH. Incident asthma and Mycoplasma pneumoniae: a nationwide cohort study. J Allergy Clin Immunol. (2016) 137:1017–23.e6. doi: 10.1016/j.jaci.2015.09.032
12. Cutler TD, Wang C, Hoff SJ, Zimmerman JJ. A method to quantify infectious airborne pathogens at concentrations below the threshold of quantification by culture. Can J Vet Res. (2013) 77:95–9.
13. CDC. Environmental Infection Control Guidelines. Guidelines for Environmental Infection Control in Health-Care Facilities (2003). Available online at: https://www.cdc.gov/infectioncontrol/guidelines/environmental/background/air.html. (accessed January 06, 2022).
14. LeMessurier KS, Tiwary M, Morin NP, Samarasinghe AE. Respiratory barrier as a safeguard and regulator of defense against influenza a virus and streptococcus pneumoniae. Front Immunol. (2020) 11:3. doi: 10.3389/fimmu.2020.00003
15. Heijink IH, Kuchibhotla VNS, Roffel MP, Maes T, Knight DA, Sayers I, et al. Epithelial cell dysfunction, a major driver of asthma development. Allergy. (2020) 75:1902–17. doi: 10.1111/all.14421
16. Mootz M, Jakwerth CA, Schmidt-Weber CB, Zissler UM. Secretoglobins in the big picture of immunoregulation in airway diseases. Allergy. (2022) 77:767–77. doi: 10.1111/all.15033
17. Solberg OD, Ostrin EJ, Love MI, Peng JC, Bhakta NR, Hou L, et al. Airway epithelial miRNA expression is altered in asthma. Am J Respir Crit Care Med. (2012) 186:965–74. doi: 10.1164/rccm.201201-0027OC
18. Frey A, Lunding LP, Ehlers JC, Weckmann M, Zissler UM, Wegmann M. More than just a barrier: the immune functions of the airway epithelium in asthma pathogenesis. Front Immunol. (2020) 11:761. doi: 10.3389/fimmu.2020.00761
19. Lambrecht BN, Hammad H. The airway epithelium in asthma. Nat Med. (2012) 18:684–92. doi: 10.1038/nm.2737
20. Hellings PW, Steelant B. Epithelial barriers in allergy and asthma. J Allergy Clin Immunol. (2020) 145:1499–509. doi: 10.1016/j.jaci.2020.04.010
21. de Kouchkovsky DA, Ghosh S, Rothlin C V. Negative regulation of type 2 immunity. Trends Immunol. (2017) 38:154–67. doi: 10.1016/j.it.2016.12.002
22. James KM, Peebles RS, Hartert T V. Response to infections in patients with asthma and atopic disease: An epiphenomenon or reflection of host susceptibility? J Allergy Clin Immunol. (2012) 130:343–51. doi: 10.1016/j.jaci.2012.05.056
23. Hodinka RL. Respiratory RNA viruses. Microbiol Spectr. (2016) 4. doi: 10.1128/microbiolspec.DMIH2-0028-2016
24. Ganesan S, Comstock AT, Sajjan US. Barrier function of airway tract epithelium. Tissue Barriers. (2013) 1:e24997. doi: 10.4161/tisb.24997
25. Gon Y, Hashimoto S. Role of airway epithelial barrier dysfunction in pathogenesis of asthma. Allergol Int. (2018) 67:12–7. doi: 10.1016/j.alit.2017.08.011
26. Edwards MR, Bartlett NW, Hussell T, Openshaw P, Johnston SL. The microbiology of asthma. Nat Rev Microbiol. (2012) 10:459–71. doi: 10.1038/nrmicro2801
27. Kumar K, Singanayagam A, Johnston SL. Respiratory virus infections in asthma: research developments and therapeutic advances. Acta Med Acad. (2020) 49:130–43. doi: 10.5644/ama2006-124.292
28. Borchers AT, Chang C, Gershwin ME, Gershwin LJ. Respiratory syncytial virus - a comprehensive review. Clin Rev Allergy Immunol. (2013) 45:331–79. doi: 10.1007/s12016-013-8368-9
29. Moriyama M, Hugentobler W, Iwasaki A. Seasonality of respiratory viral infections. Annu Rev Virol. (2020) 7:83–101. doi: 10.1146/annurev-virology-012420-022445
30. Pneumonia Fact Sheet. World Health Organization, 2019. Available online at: https://www.who.int/news-room/fact-sheets/detail/pneumonia. (accessed October 24, 2021).
31. Shi T, McAllister DA, O'Brien KL, Simoes EAF, Madhi SA, Gessner BD, et al. Global, regional, and national disease burden estimates of acute lower respiratory infections due to respiratory syncytial virus in young children in 2015: a systematic review and modelling study. Lancet. (2017) 390:946–58. doi: 10.1016/S0140-6736(17)30938-8
32. Schene KM, van den Berg E, Wösten-van Asperen RM, van Rijn RR, Bos AP, van Woensel JB. FiO2 predicts outcome in infants with respiratory syncytial virus-induced acute respiratory distress syndrome. Pediatr Pulmonol. (2014) 49:1138–44. doi: 10.1002/ppul.22974
33. Wu P, Hartert TV. Evidence for a causal relationship between respiratory syncytial virus infection and asthma. Expert Rev Anti Infect Ther. (2011) 9:731–45. doi: 10.1586/eri.11.92
34. Kusel MM, de Klerk NH, Kebadze T, Vohma V, Holt PG, Johnston SL, et al. Early-life respiratory viral infections, atopic sensitization, and risk of subsequent development of persistent asthma. J Allergy Clin Immunol. (2007) 119:1105–10. doi: 10.1016/j.jaci.2006.12.669
35. Scheltema NM, Nibbelke EE, Pouw J, Blanken MO, Rovers MM, Naaktgeboren CA, et al. Respiratory syncytial virus prevention and asthma in healthy preterm infants: a randomised controlled trial. Lancet Respir Med. (2018) 6:257–64. doi: 10.1016/S2213-2600(18)30055-9
36. Gehlhar K, Bilitewski C, Reinitz-Rademacher K, Rohde G, Bufe A. Impaired virus-induced interferon-α2 release in adult asthmatic patients. Clin Exp Allergy. (2006) 36:331–7. doi: 10.1111/j.1365-2222.2006.02450.x
37. Sabogal Piñeros YS, Bal SM, Dijkhuis A, Majoor CJ, Dierdorp BS, Dekker T, et al. Eosinophils capture viruses, a capacity that is defective in asthma. Allergy. (2019) 74:1898–909. doi: 10.1111/all.13802
38. Rosenberg HF, Bonville CA, Easton AJ, Domachowske JB. The pneumonia virus of mice infection model for severe respiratory syncytial virus infection: identifying novel targets for therapeutic intervention. Pharmacol Ther. (2005) 105:1–6. doi: 10.1016/j.pharmthera.2004.09.001
39. Percopo CM, Dyer KD, Ochkur SI, Luo JL, Fischer ER, Lee JJ, et al. Activated mouse eosinophils protect against lethal respiratory virus infection. Blood. (2014) 123:743–52. doi: 10.1182/blood-2013-05-502443
40. Branche AR, Falsey AR. Parainfluenza virus infection. Semin Respir Crit Care Med. (2016) 37:538–54. doi: 10.1055/s-0036-1584798
41. Pawełczyk M, Kowalski ML. The role of human parainfluenza virus infections in the immunopathology of the respiratory tract. Curr Allergy Asthma Rep. (2017) 17:16. doi: 10.1007/s11882-017-0685-2
42. Drake MG, Bivins-smith ER, Proskocil BJ, Nie Z, Scott GD, Lee JJ, et al. Human and mouse eosinophils have antiviral activity against parainfluenza virus. Am J Respir Cell Mol Biol. (2016) 55:387–94. doi: 10.1165/rcmb.2015-0405OC
43. Schildgen V, van den Hoogen B, Fouchier R, Tripp RA, Alvarez R, Manoha C, et al. Human metapneumovirus: lessons learned over the first decade. Clin Microbiol Rev. (2011) 24:734–54. doi: 10.1128/CMR.00015-11
44. van den Hoogen BG, de Jong JC, Groen J, Kuiken T, de Groot R, Fouchier RA, et al. A newly discovered human pneumovirus isolated from young children with respiratory tract disease. Nat Med. (2001) 7:719–24. doi: 10.1038/89098
45. Cseke G, Maginnis MS, Cox RG, Tollefson SJ, Podsiad AB, Wright DW, et al. Integrin αvβ1 promotes infection by human metapneumovirus. Proc Natl Acad Sci U S A. (2009) 106:1566–71. doi: 10.1073/pnas.0801433106
46. Edwards KM, Zhu Y, Griffin MR, Weinberg GA, Hall CB, Szilagyi PG, et al. Burden of human metapneumovirus infection in young children. N Engl J Med. (2013) 368:633–43. doi: 10.1056/NEJMoa1204630
47. Coverstone AM, Wang L, Sumino K. Beyond respiratory syncytial virus and rhinovirus in the pathogenesis and exacerbation of asthma the role of metapneumovirus, bocavirus and influenza virus. Immunol Allergy Clin N Am. (2019) 39:391–401. doi: 10.1016/j.iac.2019.03.007
48. Hamelin ME, Prince GA, Gomez AM, Kinkead R, Boivin G. Human metapneumovirus infection induces long-term pulmonary inflammation associated with airway obstruction and hyperresponsiveness in mice. J Infect Dis. (2006) 193:1634–42. doi: 10.1086/504262
49. Edwards MR, Strong K, Cameron A, Walton RP, Jackson DJ, Johnston SL. Viral infections in allergy and immunology: How allergic inflammation influences viral infections and illness. J Allergy Clin Immunol. (2017) 140:909–20. doi: 10.1016/j.jaci.2017.07.025
50. Spann KM, Baturcam E, Schagen J, Jones C, Straub CP, Preston FM, et al. Viral and host factors determine innate immune responses in airway epithelial cells from children with wheeze and atopy. Thorax. (2014) 69:918–25. doi: 10.1136/thoraxjnl-2013-204908
51. Baturcam E, Snape N, Yeo TH, Schagen J, Thomas E, Logan J, et al. Human metapneumovirus impairs apoptosis of nasal epithelial cells in asthma via HSP70. J Innate Immun. (2017) 9:52–64. doi: 10.1159/000449101
52. Bouvier N, Palese P. The biology of influenza viruses. Vaccine. (2008) 12:D49–53. doi: 10.1016/j.vaccine.2008.07.039
53. Taubenberger JK, Kash JC. Influenza virus evolution, host adaptation, and pandemic formation. Cell Host Microbe. (2010) 7:440–51. doi: 10.1016/j.chom.2010.05.009
54. Flerlage T, Boyd DF, Meliopoulos V, Thomas PG. Influenza virus and SARS-CoV-2: pathogenesis and host responses in the respiratory tract. Nat Rev Microbiol. (2021) 19:425–41. doi: 10.1038/s41579-021-00542-7
55. Influenza Seasonal Fact Sheet. World Health Organization (2018). Available online at: https://www.who.int/news-room/fact-sheets/detail/influenza-(seasonal). (accessed October 25, 2021).
56. Influenza (Avian and other zoonotic) Fact Sheet. World Health Organization (2018). Available online at: https://www.who.int/news-room/fact-sheets/detail/influenza-(avian-and-other-zoonotic). (accessed October 25, 2021).
57. Durrani SR, Montville DJ, Pratt AS, Sahu S, Devries MK, Rajamanickam V, et al. Innate immune responses to rhinovirus are reduced by the high-affinity IgE receptor in allergic asthmatic children. J Allergy Clin Immunol. (2012) 130:489–95. doi: 10.1016/j.jaci.2012.05.023
58. Gill MA, Bajwa G, George TA, Dong CC, Dougherty II, Jiang N, et al. Counterregulation between the FcεRI pathway and antiviral responses in human plasmacytoid dendritic cells. J Immunol. (2010) 184:5999–6006. doi: 10.4049/jimmunol.0901194
59. Furuya Y, Roberts S, Hurteau GJ, Sanfilippo AM, Racine R, Metzger DW. Asthma increases susceptibility to heterologous but not homologous secondary influenza. J Virol. (2014) 88:9166–81. doi: 10.1128/JVI.00265-14
60. Jain S, Kamimoto L, Bramley AM, Schmitz AM, Benoit SR, Louie J, et al. Hospitalized patients with 2009 H1N1 influenza in the United States, April-June 2009. N Engl J Med. (2009) 361:1935–44. doi: 10.1056/NEJMoa0906695
61. Veerapandian R, Snyder JD, Samarasinghe AE. Influenza in asthmatics: for better or for worse? Front Immunol. (2018) 9:1843. doi: 10.3389/fimmu.2018.01843
62. Samarasinghe AE, Woolard SN, Boyd KL, Hoselton SA, Schuh JM, McCullers JA. The immune profile associated with acute allergic asthma accelerates clearance of influenza virus. Immunol Cell Biol. (2014) 92:449–59. doi: 10.1038/icb.2013.113
63. Ishikawa H, Sasaki H, Fukui T, Fujita K, Kutsukake E, Matsumoto T. Mice with asthma are more resistant to influenza virus infection and NK cells activated by the induction of asthma have potentially protective effects. J Clin Immunol. (2012) 32:256–67. doi: 10.1007/s10875-011-9619-2
64. Furuya Y, Furuya AK, Roberts S, Sanfilippo AM, Salmon SL, Metzger DW. Prevention of influenza virus-induced immunopathology by TGF-β produced during allergic asthma. PLoS Pathog. (2015) 11:e1005180. doi: 10.1371/journal.ppat.1005180
65. Samarasinghe AE, Melo RC, Duan S, LeMessurier KS, Liedmann S, Surman SL, et al. Eosinophils promote antiviral immunity in mice infected with influenza a virus. J Immunol. (2017) 198:3214–26. doi: 10.4049/jimmunol.1600787
66. Kawaguchi A, Suzuki T, Ohara Y, Takahashi K, Sato Y, Ainai A, et al. Impacts of allergic airway inflammation on lung pathology in a mouse model of influenza A virus infection. PLoS ONE. (2017) 12:e0173008. doi: 10.1371/journal.pone.0173008
67. Lee DCP, Tay NQ, Thian M, Prabhu N, Furuhashi K, Kemeny DM. Prior exposure to inhaled allergen enhances anti-viral immunity and T cell priming by dendritic cells. PLoS ONE. (2018) 13:e0190063. doi: 10.1371/journal.pone.0190063
68. Tiwary M, Rooney RJ, Liedmann S, LeMessurier KS, Samarasinghe AE. Eosinophil responses at the airway epithelial barrier during the early phase of influenza a virus infection in C57BL/6 mice. Cells. (2021) 10:509. doi: 10.3390/cells10030509
69. Ma M, Redes JL, Percopo CM, Druey KM, Rosenberg HF. Alternaria alternata challenge at the nasal mucosa results in eosinophilic inflammation and increased susceptibility to influenza virus infection. Clin Exp Allergy. (2018) 48:691–702. doi: 10.1111/cea.13123
70. Jacobs SE, Lamson DM, Kirsten S, Walsh TJ. Human rhinoviruses. Clin Microbiol Rev. (2013) 26:135–62. doi: 10.1128/CMR.00077-12
71. Lee WM, Lemanske RF Jr, Evans MD, Vang F, Pappas T, Gangnon R, et al. Human rhinovirus species and season of infection determine illness severity. Am J Respir Crit Care Med. (2012) 186:886–91. doi: 10.1164/rccm.201202-0330OC
72. Bochkov YA, Watters K, Ashraf S, Griggs TF, Devries MK, Jackson DJ, et al. Cadherin-related family member 3, a childhood asthma susceptibility gene product, mediates rhinovirus C binding and replication. Proc Natl Acad Sci USA. (2015) 112:5485–90. doi: 10.1073/pnas.1421178112
73. Heymann PW, Carper HT, Murphy DD, Platts-Mills TA, Patrie J, McLaughlin AP, et al. Viral infections in relation to age, atopy, and season of admission among children hospitalized for wheezing. J Allergy Clin Immunol. (2004) 114:239–47. doi: 10.1016/j.jaci.2004.04.006
74. Bizzintino J, Lee WM, Laing IA, Vang F, Pappas T, Zhang G, et al. Association between human rhinovirus C and severity of acute asthma in children. Eur Respir J. (2011) 37:1037–42. doi: 10.1183/09031936.00092410
75. Olenec JP, Kim WK, Lee WM, Vang F, Pappas TE, Salazar LE, et al. Weekly monitoring of children with asthma for infections and illness during common cold seasons. J Allergy Clin Immunol. (2010) 125:1001–6.e1. doi: 10.1016/j.jaci.2010.01.059
76. Xatzipsalti M, Psarros F, Konstantinou G, Gaga M, Gourgiotis D, Saxoni-Papageorgiou P, et al. Modulation of the epithelial inflammatory response to rhinovirus in an atopic environment. Clin Exp Allergy. (2008) 38:466–72. doi: 10.1111/j.1365-2222.2007.02906.x
77. Heymann PW, Nguyen HT, Steinke JW, Turner RB, Woodfolk JA, Platts-Mills TAE, et al. Rhinovirus infection results in stronger and more persistent genomic dysregulation: evidence for altered innate immune response in asthmatics at baseline, early in infection, and during convalescence. PLoS ONE. (2017) 12:e0178096. doi: 10.1371/journal.pone.0178096
78. Belouzard S, Millet JK, Licitra BN, Whittaker GR. Mechanisms of coronavirus cell entry mediated by the viral spike protein. Viruses. (2012) 4:1011–33. doi: 10.3390/v4061011
79. World Health Organization. WHO. Coronavirus Disease (COVID-19) Dashboard. Available online at: https://covid19.who.int/. (accessed October 18, 2021).
80. Papadopoulos NG, Christodoulou I, Rohde G, Agache I, Almqvist C, Bruno A, et al. Viruses and bacteria in acute asthma exacerbations - A GA2 LEN-DARE systematic review. Allergy. (2011) 66:458–68. doi: 10.1111/j.1398-9995.2010.02505.x
81. CDC. Coronavirus Disease 2019 (COVID-19): people with certain medical conditions. Available online at: https://www.cdc.gov/coronavirus/2019-ncov/need-extra-precautions/people-with-medical-conditions.html. (accessed October 30, 2021).
82. Ramakrishnan RK, Al Heialy S, Hamid Q. Implications of preexisting asthma on COVID-19 pathogenesis. Am J Physiol - Lung Cell Mol Physiol. (2021) 320:L880–91. doi: 10.1152/ajplung.00547.2020
83. Ferastraoaru D, Hudes G, Jerschow E, Jariwala S, Karagic M, de Vos G, et al. Eosinophilia in asthma patients is protective against severe COVID-19 illness. J Allergy Clin Immunol Pr. (2021) 9:1152–62.e3. doi: 10.1016/j.jaip.2020.12.045
84. Song J, Zeng M, Wang H, Qin C, Hou HY, Sun ZY, et al. Distinct effects of asthma and COPD comorbidity on disease expression and outcome in patients with COVID-19. Allergy. (2021) 76:483–96. doi: 10.1111/all.14517
85. Gaspar-Marques J, van Zeller M, Carreiro-Martins P, Chaves Loureiro C. Severe asthma in the era of COVID-19: a narrative review. Pulmonology. (2021) 30. doi: 10.1016/j.pulmoe.2021.04.001
86. Drake MG, Fryer AD, Jacoby DB. Protective effects of eosinophils against COVID-19: more than an ACE(2) in the hole? J Allergy Clin Immunol Pract. (2021) 9:2539–40. doi: 10.1016/j.jaip.2021.02.062
87. Flores-Torres AS, Salinas-Carmona MC, Salinas E, Rosas-Taraco AG. Eosinophils and respiratory viruses. Viral Immunol. (2019) 32:198–207. doi: 10.1089/vim.2018.0150
88. LeMessurier KS, Samarasinghe AE. Eosinophils: nemeses of pulmonary pathogens? Curr Allergy Asthma Rep. (2019) 19:36. doi: 10.1007/s11882-019-0867-1
89. Tan Y, Zhou J, Zhou Q, Hu L, Long Y. Role of eosinophils in the diagnosis and prognostic evaluation of COVID-19. J Med Virol. (2021) 93:1105–10. doi: 10.1002/jmv.26506
90. Resiliac J, Grayson MH. Epidemiology of infections and development of asthma. Immunol Allergy Clin North Am. (2019) 39:297–307. doi: 10.1016/j.iac.2019.03.001
91. Tramper-Stranders G, Ambrozej D, Arcolaci A, Atanaskovic-Markovic M, Boccabella C, Bonini M, et al. Dangerous liaisons: Bacteria, antimicrobial therapies, and allergic diseases. Allergy Eur J Allergy Clin Immunol. (2021) 76:3276–91. doi: 10.1111/all.15046
92. Moffatt MF, Cookson WO. The lung microbiome in health and respiratory diseases. Clin Med. (2017) 17:525–9. doi: 10.7861/clinmedicine.17-6-525
93. Hilty M, Burke C, Pedro H, Cardenas P, Bush A, Bossley C, et al. Disordered microbial communities in asthmatic airways. PLoS One. (2010) 5:e8578. doi: 10.1371/journal.pone.0008578
94. Hufnagl K, Pali-Schöll I, Roth-Walter F, Jensen-Jarolim E. Dysbiosis of the gut and lung microbiome has a role in asthma. Semin Immunopathol. (2020) 42:75–93. doi: 10.1007/s00281-019-00775-y
95. Ackland J, Watson A, Wilkinson TMA, Staples KJ. Interrupting the conversation: implications for crosstalk between viral and bacterial infections in the asthmatic airway. Front Allergy. (2021) 2:1–21. doi: 10.3389/falgy.2021.738987
96. Mitchell AM, Mitchell TJ. Streptococcus pneumoniae: virulence factors and variation. Clin Microbiol Infect. (2010) 16:411–8. doi: 10.1111/j.1469-0691.2010.03183.x
97. Fitzgerald D, Waterer GW. Invasive pneumococcal and meningococcal disease. Infect Dis Clin North Am. (2019) 33:1125–41. doi: 10.1016/j.idc.2019.08.007
98. Mitchell T. Streptococcus pneumoniae: infection, inflammation and disease. Adv Exp Med Biol. (2006) 582:111–24. doi: 10.1007/0-387-33026-7_10
99. Pneumococcal Disease. World Health Organization. Available online at: https://www.who.int/teams/health-product-policy-and-standards/standards-and-specifications/vaccine-standardization/pneumococcal-disease. (accessed October 24, 2021).
100. Esposito S, Terranova L, Patria MF, Marseglia GL, Miraglia del Giudice M, Bodini A, et al. Streptococcus pneumoniae colonisation in children and adolescents with asthma: impact of the heptavalent pneumococcal conjugate vaccine and evaluation of potential effect of thirteen-valent pneumococcal conjugate vaccine. BMC Infect Dis. (2016) 16:12. doi: 10.1186/s12879-016-1335-3
101. Jounio U, Juvonen R, Bloigu A, Silvennoinen-Kassinen S, Kaijalainen T, Kauma H, et al. Pneumococcal carriage is more common in asthmatic than in non-asthmatic young men. Clin Respir J. (2010) 4:222–9. doi: 10.1111/j.1752-699X.2009.00179.x
102. Iikura M, Hojo M, Koketsu R, Watanabe S, Sato A, Chino H, et al. The importance of bacterial and viral infections associated with adult asthma exacerbations in clinical practice. PLoS ONE. (2015) 10:e0123584. doi: 10.1371/journal.pone.0123584
103. Cardozo DM, Nascimento-Carvalho CM, Andrade ASS, Silvany-Neto AM, Daltro CHC, Brandão MS, et al. Prevalence and risk factors for nasopharyngeal carriage of Streptococcus pneumoniae among adolescents. J Med Microbiol. (2008) 57:185–9. doi: 10.1099/jmm.0.47470-0
104. Zaidi SR, Blakey JD. Why are people with asthma susceptible to pneumonia? A review of factors related to upper airway bacteria. Respirology. (2019) 24:423–30. doi: 10.1111/resp.13528
105. Yang B, Liu R, Yang T, Jiang X, Zhang L, Wang L, et al. Neonatal Streptococcus pneumoniae infection may aggravate adulthood allergic airways disease in association with IL-17A. PLoS ONE. (2015) 10:e0123010. doi: 10.1371/journal.pone.0123010
106. Preston JA, Thorburn AN, Starkey MR, Beckett EL, Horvat JC, Wade MA, et al. Streptococcus pneumoniae infection suppresses allergic airways disease by inducing regulatory T-cells. Eur Respir J. (2011) 37:53–64. doi: 10.1183/09031936.00049510
107. Thorburn AN, Foster PS, Gibson PG, Hansbro PM. Components of streptococcus pneumoniae suppress allergic airways disease and NKT cells by inducing regulatory T cells. J Immunol. (2012) 188:4611–20. doi: 10.4049/jimmunol.1101299
108. Preston JA, Essilfie AT, Horvat JC, Wade MA, Beagley KW, Gibson PG, et al. Inhibition of allergic airways disease by immunomodulatory therapy with whole killed Streptococcus pneumoniae. Vaccine. (2007) 25:8154–62. doi: 10.1016/j.vaccine.2007.09.034
109. Wu G, Zhang X, Chen X, Wang J, Yang J, Wang L, et al. Streptococcus pneumoniae aminopeptidase N regulates dendritic cells that attenuates type-2 airway inflammation in murine allergic asthma. Br J Pharmacol. (2020) 177:5063–77. doi: 10.1111/bph.15216
110. Thorburn AN, Tseng HY, Donovan C, Hansbro NG, Jarnicki AG, Foster PS, et al. TLR2, TLR4 AND MyD88 mediate allergic airway disease (AAD) and streptococcus pneumoniae-induced suppression of AAD. PLoS ONE. (2016) 11:e0156402. doi: 10.1371/journal.pone.0156402
111. Thorburn AN, O'Sullivan BJ, Thomas R, Kumar RK, Foster PS, Gibson PG, et al. Pneumococcal conjugate vaccine-induced regulatory T cells suppress the development of allergic airways disease. Thorax. (2010) 65:1053–60. doi: 10.1136/thx.2009.131508
112. Kama Y, Kato M, Yamada Y, Koike T, Suzuki K, Enseki M, et al. The suppressive role of streptococcus pneumoniae colonization in acute exacerbations of childhood bronchial asthma. Int Arch Allergy Immunol. (2020) 181:191–9. doi: 10.1159/000504541
113. Sanfilippo AM, Furuya Y, Roberts S, Salmon SL, Metzger DW. Allergic lung inflammation reduces tissue invasion and enhances survival from pulmonary pneumococcal infection in mice, which correlates with increased expression of transforming growth factor β1 and Siglec F (low) alveolar macrophages. Infect Immun. (2015) 83:2976–83. doi: 10.1128/IAI.00142-15
114. Schmit T, Ghosh S, Mathur RK, Barnhardt T, Ambigapathy G, Wu M, et al. IL-6 deficiency exacerbates allergic asthma and abrogates the protective effect of allergic inflammation against streptococcus pneumoniae pathogenesis. J Immunol. (2020) 205:469–79. doi: 10.4049/jimmunol.1900755
115. Habibzay M, Saldana JI, Goulding J, Lloyd CM, Hussell T. Altered regulation of Toll-like receptor responses impairs antibacterial immunity in the allergic lung. Mucosal Immunol. (2012) 5:524–34. doi: 10.1038/mi.2012.28
116. Papanicolaou A, Wang H, Satzke C, Vlahos R, Wilson N, Bozinovski S. Novel therapies for pneumonia-associated severe asthma phenotypes. Trends Mol Med. (2020) 26:1047–58. doi: 10.1016/j.molmed.2020.07.006
117. Wen S, Feng D, Chen D, Yang L, Xu Z. Molecular epidemiology and evolution of Haemophilus influenzae. Infect Genet Evol. (2020) 80:104205. doi: 10.1016/j.meegid.2020.104205
118. Adam HJ, Richardson SE, Jamieson FB, Rawte P, Low DE, Fisman DN. Changing epidemiology of invasive Haemophilus influenzae in Ontario, Canada: evidence for herd effects and strain replacement due to Hib vaccination. Vaccine. (2010) 28:4073–8. doi: 10.1016/j.vaccine.2010.03.075
119. Murphy TF, Faden H, Bakaletz LO, Kyd JM, Forsgren A, Campos J, et al. Nontypeable haemophilus influenzae as a pathogen in children. Pediatr Infect Dis J. (2009) 28:43–8. doi: 10.1097/INF.0b013e318184dba2
120. Duell BL, Su YC, Riesbeck K. Host–pathogen interactions of nontypeable Haemophilus influenzae: from commensal to pathogen. FEBS Lett. (2016) 590:3840–53. doi: 10.1002/1873-3468.12351
121. Mccann JR, Mason SN, Auten RL, St Geme J, Seed P. Early-life intranasal colonization with nontypeable haemophilus influenzae exacerbates juvenile airway disease in mice. Infect immun. (2016) 84:2022–30. doi: 10.1128/IAI.01539-15
122. Essilfie AT, Simpson JL, Horvat JC, Preston JA, Dunkley ML, Foster PS, et al. Haemophilus influenzae infection drives IL-17-mediated neutrophilic allergic airways disease. PLoS Pathog. (2011) 7:e1002244. doi: 10.1371/journal.ppat.1002244
123. Essilfie AT, Simpson JL, Dunkley ML, Morgan LC, Oliver BG, Gibson PG, et al. Combined Haemophilus influenzae respiratory infection and allergic airways disease drives chronic infection and features of neutrophilic asthma. Thorax. (2012) 67:588–99. doi: 10.1136/thoraxjnl-2011-200160
124. Simpson JL, Daly J, Baines KJ, Yang IA, Upham JW, Reynolds PN, et al. Airway dysbiosis: Haemophilus influenza and Tropheryma in poorly controlled asthma. Eur Respir J. (2016) 47:792–800. doi: 10.1183/13993003.00405-2015
125. Wood LG, Simpson JL, Hansbro PM, Gibson PG. Potentially pathogenic bacteria cultured from the sputum of stable asthmatics are associated with increased 8-isoprostane and airway neutrophilia. Free Radic Res. (2010) 44:146–54. doi: 10.3109/10715760903362576
126. Green BJ, Wiriyachaiporn S, Grainge C, Rogers GB, Kehagia V, Lau L, et al. Potentially pathogenic airway bacteria and neutrophilic inflammation in treatment resistant severe asthma. PLoS ONE. (2014) 9:e100645. doi: 10.1371/journal.pone.0100645
127. Taylor SL, Leong LEX, Choo JM, Wesselingh S, Yang IA, Upham JW, et al. Inflammatory phenotypes in patients with severe asthma are associated with distinct airway microbiology. J Allergy Clin Immunol. (2018) 141:94–103.e15. doi: 10.1016/j.jaci.2017.03.044
128. Liang Z, Zhang Q, Thomas CM, Chana KK, Gibeon D, Barnes PJ, et al. Impaired macrophage phagocytosis of bacteria in severe asthma. Respir Res. (2014) 15:72. doi: 10.1186/1465-9921-15-72
129. Murphy TF, Parameswaran GI. Moraxella catarrhalis, a human respiratory tract pathogen. Clin Infect Dis. (2009) 49:124–31. doi: 10.1086/599375
130. de Vries SP, Bootsma HJ, Hays JP, Hermans PW. Molecular aspects of moraxella catarrhalis pathogenesis. Microbiol Mol Biol Rev. (2009) 73:389–406. doi: 10.1128/MMBR.00007-09
131. Zhou Y, Jackson D, Bacharier LB, Mauger D, Boushey H, Castro M, et al. The upper-airway microbiota and loss of asthma control among asthmatic children. Nat Commun. (2019) 10:5714. doi: 10.1038/s41467-019-13698-x
132. Nagayama Y, Tsubaki T, Nakayama S, Sawada K, Taguchi K, Toba T, et al. Bacterial colonization in respiratory secretions from acute and recurrent wheezing infants and children. Pediatr Allergy Immunol. (2007) 18:110–7. doi: 10.1111/j.1399-3038.2006.00492.x
133. McCauley K, Durack J, Valladares R, Fadrosh DW, Lin DL, Calatroni A, et al. Distinct nasal airway bacterial microbiotas differentially relate to exacerbation in pediatric patients with asthma. J Allergy Clin Immunol. (2019) 144:1187–97. doi: 10.1016/j.jaci.2019.05.035
134. Durack J, Huang YJ, Nariya S, Christian LS, Ansel KM, Beigelman A, et al. Bacterial biogeography of adult airways in atopic asthma. Microbiome. (2018) 6:104. doi: 10.1186/s40168-018-0487-3
135. Larsen JM, Brix S, Thysen AH, Birch S, Rasmussen MA, Bisgaard H. Children with asthma by school age display aberrant immune responses to pathogenic airway bacteria as infants. J Allergy Clin Immunol. (2014) 133:1008–13. doi: 10.1016/j.jaci.2014.01.010
136. Alnahas S, Hagner S, Raifer H, Kilic A, Gasteiger G, Mutters R, et al. IL-17 and TNF-α are key mediators of Moraxella catarrhalis triggered exacerbation of allergic airway inflammation. Front Immunol. (2017) 8:1562. doi: 10.3389/fimmu.2017.01562
137. He J, Liu M, Ye Z, Tan T, Liu X, You X, et al. Insights into the pathogenesis of Mycoplasma pneumoniae (Review). Mol Med Rep. (2016) 14:4030–6. doi: 10.3892/mmr.2016.5765
138. Kumar S. Mycoplasma pneumoniae: a significant but underrated pathogen in paediatric community-acquired lower respiratory tract infections. Indian J Med Res. (2018) 147:23–31. doi: 10.4103/ijmr.IJMR_1582_16
139. Waites K, Xiao L, Liu Y, Balish M, Atkinson T. Mycoplasma pneumoniae from the respiratory tract and beyond. Clin Microbiol Rev. (2017) 30:747–809. doi: 10.1128/CMR.00114-16
140. Wood PR, Hill VL, Burks ML, Peters JI, Singh H, Kannan TR, et al. Mycoplasma pneumoniae in children with acute and refractory asthma. Ann Allergy, Asthma Immunol. (2013) 110:328–34.e1. doi: 10.1016/j.anai.2013.01.022
141. Bajantri B, Venkatram S, Diaz-Fuentes G. Mycoplasma pneumoniae: a potentially severe infection. J Clin Med Res. (2018) 10:535–44. doi: 10.14740/jocmr3421w
142. Medina JL, Coalson JJ, Brooks EG, Winter VT, Chaparro A, Principe MF, et al. Mycoplasma pneumoniae CARDS toxin induces pulmonary eosinophilic and lymphocytic inflammation. Am J Respir Cell Mol Biol. (2012) 46:815–22. doi: 10.1165/rcmb.2011-0135OC
143. Shin JE, Cheon BR, Shim JW, Kim DS, Jung HL, Park MS, et al. Increased risk of refractory Mycoplasma pneumoniae pneumonia in children with atopic sensitization and asthma. Korean J Pediatr. (2014) 57:271–7. doi: 10.3345/kjp.2014.57.6.271
144. Wu Q, Martin RJ, LaFasto S, Chu H. Low dose of Mycoplasma pneumoniae (Mp) infection enhances an established allergic inflammation in mice: Role of prostaglandin E2 pathway. Clin Exp Allergy. (2009) 39:1754–63. doi: 10.1111/j.1365-2222.2009.03309.x
145. Medina JL, Coalson JJ, Brooks EG, Le Saux CJ, Winter VT, Chaparro A, et al. Mycoplasma pneumoniae CARDS toxin exacerbates ovalbumin-induced asthma-like inflammation in BALB/c mice. PLoS ONE. (2014) 9:e102613. doi: 10.1371/journal.pone.0102613
146. Wu Q, Martin RJ, Rino JG, Jeyaseelan S, Breed R, Chu HW. A deficient TLR2 signaling promotes airway mucin production in Mycoplasma pneumoniae-infected allergic mice. Am J Physiol Lung Cell Mol Physiol. (2007) 292:L1064–72. doi: 10.1152/ajplung.00301.2006
147. Dy ABC, Tanyaratsrisakul S, Voelker DR, Ledford JG. The emerging roles of surfactant protein-a in asthma. J Clin Cell Immunol. (2018) 9:553. doi: 10.4172/2155-9899.1000553
148. Ledford JG, Mukherjee S, Kislan MM, Nugent JL, Hollingsworth JW, Wright JR. Surfactant protein-a suppresses eosinophil-mediated killing of mycoplasma pneumoniae in allergic lungs. PLoS ONE. (2012) 7:e32436. doi: 10.1371/journal.pone.0032436
149. Wu Q, Martin RJ, LaFasto S, Efaw BJ, Rino JG, Harbeck RJ, et al. Toll-like receptor 2 down-regulation in established mouse allergic lungs contributes to decreased mycoplasma clearance. Am J Respir Crit Care Med. (2008) 177:720–9. doi: 10.1164/rccm.200709-1387OC
150. Chu HW, Thaikoottathil J, Rino JG, Zhang G, Wu Q, Moss T, et al. Function and regulation of SPLUNC1 protein in mycoplasma infection and allergic inflammation. J Immunol. (2007) 179:3995–4002. doi: 10.4049/jimmunol.179.6.3995
151. Britto CJ, Cohn L. Bactericidal/permeability-increasing protein fold-containing family member A1 in airway host protection and respiratory disease. Am J Respir Cell Mol Biol. (2015) 52:525–34. doi: 10.1165/rcmb.2014-0297RT
152. Gally F, Di YP, Smith SK, Minor MN, Liu Y, Bratton DL, et al. SPLUNC1 promotes lung innate defense against mycoplasma pneumoniae infection in mice. Am J Pathol. (2011) 178:2159–67. doi: 10.1016/j.ajpath.2011.01.026
153. Burillo A, Bouza E. Chlamydophila pneumoniae. Infect Dis Clin North Am. (2010) 24:61–71. doi: 10.1016/j.idc.2009.10.002
154. Cosentini R, Tarsia P, Canetta C, Graziadei G, Brambilla AM, Aliberti S, et al. Severe asthma exacerbation: role of acute Chlamydophila pneumoniae and Mycoplasma pneumoniae infection. Respir Res. (2008) 9:48. doi: 10.1186/1465-9921-9-48
155. Horvat JC, Starkey MR, Kim RY, Phipps S, Gibson PG, Beagley KW, et al. Early-life chlamydial lung infection enhances allergic airways disease through age-dependent differences in immunopathology. J Allergy Clin Immunol. (2010) 125:617–25. doi: 10.1016/j.jaci.2009.10.018
156. Webley WC, Tilahun Y, Lay K, Patel K, Stuart ES, Andrzejewski C, et al. Occurrence of Chlamydia trachomatis and Chlamydia pneumoniae in paediatric respiratory infections. Eur Respir J. (2009) 33:360–7. doi: 10.1183/09031936.00019508
157. Starkey MR, Kim RY, Beckett EL, Schilter HC, Shim D, Essilfie AT, et al. Chlamydia muridarum lung infection in infants alters hematopoietic cells to promote allergic airway disease in mice. PLoS ONE. (2012) 7:e42588. doi: 10.1371/journal.pone.0042588
158. Horvat JC, Starkey MR, Kim RY, Beagley KW, Preston JA, Gibson PG, et al. Chlamydial respiratory infection during allergen sensitization drives neutrophilic allergic airways disease. J Immunol. (2010) 184:4159–69. doi: 10.4049/jimmunol.0902287
159. Kim RY, Horvat JC, Pinkerton JW, Starkey MR, Essilfie AT, Mayall JR, et al. MicroRNA-21 drives severe, steroid-insensitive experimental asthma by amplifying phosphoinositide 3-kinase–mediated suppression of histone deacetylase 2. J Allergy Clin Immunol. (2017) 139:519–32. doi: 10.1016/j.jaci.2016.04.038
160. Patel KK, Vicencio AG, Du Z, Tsirilakis K, Salva PS, Webley WC. Infectious chlamydia pneumoniae is associated with elevated interleukin-8 and airway neutrophilia in children with refractory asthma. Pediatr Infect Dis J. (2010) 29:1093–8. doi: 10.1097/INF.0b013e3181eaebdc
161. Park CS, Lee YS, Kwon HS, Lee T, Kim TB, Moon KA, et al. Chlamydophila pneumoniae inhibits corticosteroid-induced suppression of metalloproteinase-9 and tissue inhibitor metalloproteinase-1 secretion by human peripheral blood mononuclear cells. J Med Microbiol. (2012) 61:705–11. doi: 10.1099/jmm.0.036624-0
162. van Tilburg Bernardes E, Gutierrez MW, Arrieta MC. The fungal microbiome and asthma. Front Cell Infect Microbiol. (2020) 10:584318. doi: 10.3389/fcimb.2020.583418
163. Huseyin CE, O'Toole PW, Cotter PD, Scanlan PD. Forgotten fungi-the gut mycobiome in human health and disease. FEMS Microbiol Rev. (2017) 41:479–511. doi: 10.1093/femsre/fuw047
164. van Woerden HC, Gregory C, Brown R, Marchesi JR, Hoogendoorn B, Matthews IP. Differences in fungi present in induced sputum samples from asthma patients and non-atopic controls: a community based case control study. BMC Infect Dis. (2013) 13:69. doi: 10.1186/1471-2334-13-69
165. Goldman DL, Chen Z, Shankar V, Tyberg M, Vicencio A, Burk R. Lower airway microbiota and mycobiota in children with severe asthma. J Allergy Clin Immunol. (2018) 141:808–11.e7. doi: 10.1016/j.jaci.2017.09.018
166. Sharma A, Laxman B, Naureckas ET, Hogarth DK, Sperling AI, Solway J, et al. Associations between fungal and bacterial microbiota of airways and asthma endotypes. J Allergy Clin Immunol. (2019) 144:1214–27.e7. doi: 10.1016/j.jaci.2019.06.025
167. Pashley CH, Wardlaw AJ. Allergic fungal airways disease (AFAD): an under-recognised asthma endotype. Mycopathologia. (2021) 186:609–22. doi: 10.1007/s11046-021-00562-0
168. Mousavi B, Hedayati MT, Hedayati N, Ilkit M, Syedmousavi S. Aspergillus species in indoor environments and their possible occupational and public health hazards. Curr Med Mycol. (2016) 2:36–42. doi: 10.18869/acadpub.cmm.2.1.36
169. van de Veerdonk FL, Gresnigt MS, Romani L, Netea MG, Latgé JP. Aspergillus fumigatus morphology and dynamic host interactions. Nat Rev Microbiol. (2017) 15:661–74. doi: 10.1038/nrmicro.2017.90
170. Latgé J, Chamilos G. Aspergillus fumigatus and Aspergillosis in 2019. Clin Microbiol Rev. (2019) 33:e00140–18. doi: 10.1128/CMR.00140-18
171. Backman H, Jansson SA, Stridsman C, Eriksson B, Hedman L, Eklund BM, et al. Severe asthma—a population study perspective. Clin Exp Allergy. (2019) 49:819–28. doi: 10.1111/cea.13378
172. Goh KJ, Yii ACA, Lapperre TS, Chan AK, Chew FT, Chotirmall SH, et al. Sensitization to Aspergillus species is associated with frequent exacerbations in severe asthma. J Asthma Allergy. (2017) 10:131–40. doi: 10.2147/JAA.S130459
173. Fairs A, Agbetile J, Hargadon B, Bourne M, Monteiro WR, Brightling CE, et al. IgE sensitization to Aspergillus fumigatus is associated with reduced lung function in asthma. Am J Respir Crit Care Med. (2010) 182:1362–8. doi: 10.1164/rccm.201001-0087OC
174. Menzies D, Holmes L, McCumesky G, Prys-Picard C, Niven R. Aspergillus sensitization is associated with airflow limitation and bronchiectasis in severe asthma. Allergy. (2011) 66:679–85. doi: 10.1111/j.1398-9995.2010.02542.x
175. Sharpe RA, Bearman N, Thornton CR, Husk K, Osborne NJ. Indoor fungal diversity and asthma: a meta-analysis and systematic review of risk factors. J Allergy Clin Immunol. (2015) 135:110–22. doi: 10.1016/j.jaci.2014.07.002
176. Balenga NA, Klichinsky M, Xie Z, Chan EC, Zhao M, Jude J, et al. A fungal protease allergen provokes airway hyper-responsiveness in asthma. Nat Commun. (2015) 6:6763. doi: 10.1038/ncomms7763
177. Hoselton SA, Samarasinghe AE, Seydel JM, Schuh JM. An inhalation model of airway allergic response to inhalation of environmental Aspergillus fumigatus conidia in sensitized BALB/c mice. Med Mycol. (2010) 48:1056–65. doi: 10.3109/13693786.2010.485582
178. Hiraishi Y, Yamaguchi S, Yoshizaki T, Nambu A, Shimura E, Takamori A, et al. IL-33, IL-25 and TSLP contribute to development of fungal-associated protease-induced innate-type airway inflammation. Sci Rep. (2018) 8:1–12. doi: 10.1038/s41598-018-36440-x
179. O'Dea EM, Amarsaikhan N, Li H, Downey J, Steele E, Van Dyken SJ, et al. Eosinophils are recruited in response to chitin exposure and enhance Th2-mediated immune pathology in aspergillus Fumigatus infection. Infect Immun. (2014) 82:3199–205. doi: 10.1128/IAI.01990-14
180. Amarsaikhan N, O'Dea EM, Tsoggerel A, Templeton SP. Lung eosinophil recruitment in response to Aspergillus fumigatus is correlated with fungal cell wall composition and requires γδ T cells. Microbes Infect. (2017) 19:422–31. doi: 10.1016/j.micinf.2017.05.001
181. Kanj A, Abdallah N, Soubani AO. The spectrum of pulmonary aspergillosis. Respir Med. (2018) 141:121–31. doi: 10.1016/j.rmed.2018.06.029
182. Knutsen AP, Slavin RG. Allergic bronchopulmonary aspergillosis in asthma and cystic fibrosis. Clin Dev Immunol. (2011) 2011:843763. doi: 10.1155/2011/843763
183. Sunman B, Ademhan Tural D, Ozsezen B, Emiralioglu N, Yalcin E, Özçelik U. Current approach in the diagnosis and management of allergic bronchopulmonary aspergillosis in children with cystic fibrosis. Front Pediatr. (2020) 8:582964. doi: 10.3389/fped.2020.582964
184. Denning DW, Pleuvry A, Cole DC. Global burden of allergic bronchopulmonary aspergillosis with asthma and its complication chronic pulmonary aspergillosis in adults. Med Mycol. (2013) 51:361–70. doi: 10.3109/13693786.2012.738312
185. Mistry H, Ajsivinac Soberanis HM, Kyyaly MA, Azim A, Barber C, Knight D, et al. The clinical implications of aspergillus fumigatus sensitization in difficult-to-treat asthma patients. J Allergy Clin Immunol Pract. (2021) 9:4254–67.e10. doi: 10.1016/j.jaip.2021.08.038
186. Greenberger PA, Bush RK, Demain JG, Luong A, Slavin RG, Knutsen AP. Allergic bronchopulmonary aspergillosis. J Allergy Clin Immunol Pr. (2014) 2:703–8. doi: 10.1016/j.jaip.2014.08.007
187. Fukutomi Y, Taniguchi M. Sensitization to fungal allergens: resolved and unresolved issues. Allergol Int. (2015) 64:321–31. doi: 10.1016/j.alit.2015.05.007
188. Tiwary M, Samarasinghe AE. Initiation and pathogenesis of severe asthma with fungal sensitization. Cells. (2021) 10:913. doi: 10.3390/cells10040913
189. Agarwal R, Sehgal IS, Dhooria S, Muthu V, Prasad KT, Bal A, et al. Allergic bronchopulmonary aspergillosis. Indian J Med Res. (2020) 151:529–49. doi: 10.4103/ijmr.IJMR_1187_19
190. Muniz VS, Silva JC, Braga YAV, Melo RCN, Ueki S, Takeda M, et al. Eosinophils release extracellular DNA traps in response to Aspergillus fumigatus. J Allergy Clin Immunol. (2018) 141:571–85.e7. doi: 10.1016/j.jaci.2017.07.048
191. Omokawa A, Ueki S, Kikuchi Y, Takeda M, Asano M, Sato K et al. Mucus plugging in allergic bronchopulmonary aspergillosis: implication of the eosinophil DNA traps. Allergol Int. (2018) 67:280–2. doi: 10.1016/j.alit.2017.08.002
192. Ueki S, Hebisawa A, Kitani M, Asano K, Neves JS. Allergic bronchopulmonary aspergillosis-A luminal hypereosinophilic disease with extracellular trap cell death. Front Immunol. (2018) 9:2346. doi: 10.3389/fimmu.2018.02346
193. Lilly LM, Scopel M, Nelson MP, Burg AR, Dunaway CW, Steele C. Eosinophil deficiency compromises lung defense against Aspergillus fumigatus. Infect Immun. (2014) 82:1315–25. doi: 10.1128/IAI.01172-13
194. Porter P, Susarla SC, Polikepahad S, Qian Y, Hampton J, Kiss A, et al. Link between allergic asthma and airway mucosal infection suggested by proteinase-secreting household fungi. Mucosal Immunol. (2009) 2:504–17. doi: 10.1038/mi.2009.102
195. Visagie CM, Houbraken J, Frisvad JC, Hong SB, Klaassen CH, Perrone G, et al. Identification and nomenclature of the genus Penicillium. Stud Mycol. (2014) 78:343–71. doi: 10.1016/j.simyco.2014.09.001
196. Woolnough KF, Richardson M, Newby C, Craner M, Bourne M, Monteiro W, et al. The relationship between biomarkers of fungal allergy and lung damage in asthma. Clin Exp Allergy. (2017) 47:48–56. doi: 10.1111/cea.12848
197. Welsh KG, Holden KA, Wardlaw AJ, Satchwell J, Monteiro W, Pashley CH, et al. Fungal sensitization and positive fungal culture from sputum in children with asthma are associated with reduced lung function and acute asthma attacks respectively. Clin Exp Allergy. (2021) 51:790–800. doi: 10.1111/cea.13799
198. Polke M, Hube B, Jacobsen ID. Candida survival strategies. Adv Appl Microbiol. (2015) 91:139–235. doi: 10.1016/bs.aambs.2014.12.002
199. Pendleton KM, Huffnagle GB, Dickson RP. The significance of Candida in the human respiratory tract: our evolving understanding. Pathog Dis. (2017) 75:ftx029. doi: 10.1093/femspd/ftx029
200. Chowdhary A, Agarwal K, Kathuria S, Gaur SN, Randhawa HS, Meis JF. Allergic bronchopulmonary mycosis due to fungi other than Aspergillus: a global overview. Crit Rev Microbiol. (2014) 40:30–48. doi: 10.3109/1040841X.2012.754401
201. Masaki K, Fukunaga K, Matsusaka M, Kabata H, Tanosaki T, Mochimaru T, et al. Characteristics of severe asthma with fungal sensitization. Ann Allergy, Asthma Immunol. (2017) 119:253–7. doi: 10.1016/j.anai.2017.07.008
202. Fujimura KE, Sitarik AR, Havstad S, Lin DL, Levan S, Fadrosh D, et al. Neonatal gut microbiota associates with childhood multisensitized atopy and T cell differentiation. Nat Med. (2016) 22:1187–91. doi: 10.1038/nm.4176
203. Kim YG, Udayanga KG, Totsuka N, Weinberg JB, Núñez G, Shibuya A. Gut dysbiosis promotes M2 macrophage polarization and allergic airway inflammation via fungi-induced PGE2. Cell Host Microbe. (2014) 15:95–102. doi: 10.1016/j.chom.2013.12.010
204. Yike I. Fungal Proteases and Their Pathophysiological Effects. Mycopathologia. (2011) 171:299–323. doi: 10.1007/s11046-010-9386-2
205. Wu Y, Zeng Z, Guo Y, Song L, Weatherhead JE, Huang X, et al. Candida albicans elicits protective allergic responses via platelet mediated T helper 2 and T helper 17 cell polarization. Immunity. (2021) 54:2595–610.e7. doi: 10.1016/j.immuni.2021.08.009
206. Zaragoza O. Basic principles of the virulence of Cryptococcus. Virulence. (2019) 10:490–501. doi: 10.1080/21505594.2019.1614383
207. Casadevall A, Coelho C, Cordero RJB, Dragotakes Q, Jung E, Vij R, et al. The capsule of Cryptococcus neoformans. Virulence. (2019) 10:822–31. doi: 10.1080/21505594.2018.1431087
208. Chen SCA, Meyer W, Sorrell TC. Cryptococcus gattii infections. Clin Microbiol Rev. (2014) 27:980–1024. doi: 10.1128/CMR.00126-13
209. Kwon-Chung KJ, Fraser JA, Doering TÁL, Wang ZA, Janbon G, Idnurm A, et al. Cryptococcus neoformans and Cryptococcus gattii, the etiologic agents of cryptococcosis. Cold Spring Harb Perspect Med. (2015) 4:1–27. doi: 10.1101/cshperspect.a019760
210. May RC, Stone NR, Wiesner DL, Bicanic T, Nielsen K. Cryptococcus: from environmental saprophyte to global pathogen. Nat Rev Microbiol. (2016) 14:106–17. doi: 10.1038/nrmicro.2015.6
211. Wiesner DL, Specht CA, Lee CK, Smith KD, Mukaremera L, Lee ST, et al. Chitin recognition via chitotriosidase promotes pathologic Type-2 Helper T cell responses to cryptococcal infection. PLoS Pathog. (2015) 11:1–28. doi: 10.1371/journal.ppat.1004701
212. Davis MJ, Tsang TM, Qiu Y, Dayrit JK, Freij JB, Huffnagle GB, et al. Macrophage M1/M2 polarization dynamically adapts to changes in cytokine microenvironments in Cryptococcus neoformans infection. MBio. (2013) 4. doi: 10.1128/mBio.00264-13
213. Goldman DL, Davis J, Bommarito F, Shao X, Casadevall A. Enhanced allergic inflammation and airway responsiveness in rats with chronic Cryptococcus neoformans infection: potential role for fungal pulmonary infection in the pathogenesis of asthma. J Infect Dis. (2006) 193:1178–86. doi: 10.1086/501363
214. Müller U, Stenzel W, Köhler G, Werner C, Polte T, Hansen G, et al. IL-13 induces disease-promoting type 2 cytokines, alternatively activated macrophages and allergic inflammation during pulmonary infection of mice with cryptococcus neoformans. J Immunol. (2007) 179:5367–77. doi: 10.4049/jimmunol.179.8.5367
215. Müller U, Stenzel W, Köhler G, Polte T, Blessing M, Mann A, et al. A gene-dosage effect for interleukin-4 receptor α-chain expression has an impact on Th2-mediated allergic inflammation during bronchopulmonary mycosis. J Infect Dis. (2008) 198:1714–21. doi: 10.1086/593068
216. Rick EM, Woolnough KF, Seear PJ, Fairs A, Satchwell J, Richardson M, et al. The airway fungal microbiome in asthma. Clin Exp Allergy. (2020) 50:1325–41. doi: 10.1111/cea.13722
217. Schoettler N, Strek ME. Recent advances in severe asthma: from phenotypes to personalized medicine. Chest. (2020) 157:516–28. doi: 10.1016/j.chest.2019.10.009
218. Huang J, Pansare M. New treatments for asthma. Pediatr Clin North Am. (2019) 66:925–39. doi: 10.1016/j.pcl.2019.06.001
219. Agache I, Eguiluz-Gracia I, Cojanu C, Laculiceanu A, del Giacco S, Zemelka-Wiacek M, et al. Advances and highlights in asthma in 2021. Allergy. (2021) 76:3390–407. doi: 10.1111/all.15054
220. Heffler E, Madeira LNG, Ferrando M, Puggioni F, Racca F, Malvezzi L, et al. Inhaled corticosteroids safety and adverse effects in patients with asthma. J Allergy Clin Immunol Pract. (2018) 6:776–81. doi: 10.1016/j.jaip.2018.01.025
221. An JY, Ahn C, Kang HY, Jeung EB. Inhibition of mucin secretion via glucocorticoid-induced regulation of calcium-related proteins in mouse lung. Am J Physiol Lung Cell Mol Physiol. (2018) 314:L956–66. doi: 10.1152/ajplung.00417.2017
222. Dietz K, de Los Reyes Jiménez M, Gollwitzer ES, Chaker AM, Zissler UM, Rådmark OP, et al. Age dictates a steroid-resistant cascade of Wnt5a, transglutaminase 2, and leukotrienes in inflamed airways. J Allergy Clin Immunol. (2017) 139:1343–54.e6. doi: 10.1016/j.jaci.2016.07.014
223. Saffar AS, Ashdown H, Gounni AS. The molecular mechanisms of glucocorticoids-mediated neutrophil survival. Curr Drug Targets. (2011) 12:556–62. doi: 10.2174/138945011794751555
224. Nabe T. Steroid-resistant asthma and neutrophils. Biol Pharmac Bull. (2020) 43:31–5. doi: 10.1248/bpb.b19-00095
225. Goleva E, Jackson LP, Harris JK, Robertson CE, Sutherland ER, Hall CF, et al. The effects of airway microbiome on corticosteroid responsiveness in asthma. Am J Respir Crit Care Med. (2013) 188:1193–201. doi: 10.1164/rccm.201304-0775OC
226. Durack J, Lynch SV, Nariya S, Bhakta NR, Beigelman A, Castro M, et al. Features of the bronchial bacterial microbiome associated with atopy, asthma, and responsiveness to inhaled corticosteroid treatment. J Allergy Clin Immunol. (2017) 140:63–75. doi: 10.1016/j.jaci.2016.08.055
227. Yang X, Wang Y, Zhao S, Wang R, Wang C. Long-term exposure to low-dose Haemophilus influenzae during allergic airway disease drives a steroid-resistant neutrophilic inflammation and promotes airway remodeling. Oncotarget. (2018) 9:24898–913. doi: 10.18632/oncotarget.24653
228. Yang X, Li H, Ma Q, Zhang Q, Wang C. Neutrophilic asthma is associated with increased airway bacterial burden and disordered community composition. Biomed Res Int. (2018) 2018:9230234. doi: 10.1155/2018/9230234
229. Cukic V, Lovre V, Dragisic D, Ustamujic A. Asthma and chronic obstructive pulmonary disease (copd)- differences and similarities. Mater Socio Medica. (2012) 24:100. doi: 10.5455/msm.2012.24.100-105
230. Dicker AJ, Huang JTJ, Lonergan M, Keir HR, Fong CJ, Tan B, et al. The sputum microbiome, airway inflammation, and mortality in chronic obstructive pulmonary disease. J Allergy Clin Immunol. (2021) 147:158–67. doi: 10.1016/j.jaci.2020.02.040
231. Wang Z, Bafadhel M, Haldar K, Spivak A, Mayhew D, Miller BE, et al. Lung microbiome dynamics in COPD exacerbations. Eur Respir J. (2016) 47:1082–92. doi: 10.1183/13993003.01406-2015
232. Contoli M, Pauletti A, Rossi MR, Spanevello A, Casolari P, Marcellini A, et al. Long-term effects of inhaled corticosteroids on sputum bacterial and viral loads in COPD. Eur Respir J. (2017) 50:1700451. doi: 10.1183/13993003.00451-2017
233. Hartmann JE, Albrich WC, Dmitrijeva M, Kahlert CR. The effects of corticosteroids on the respiratory microbiome: a systematic review. Front Med. (2021) 8:588584. doi: 10.3389/fmed.2021.588584
234. Denner DR, Sangwan N, Becker JB, Hogarth DK, Oldham J, Castillo J, et al. Corticosteroid therapy and airflow obstruction influence the bronchial microbiome, which is distinct from that of bronchoalveolar lavage in asthmatic airways. J Allergy Clin Immunol. (2016) 137:1398–405.e3. doi: 10.1016/j.jaci.2015.10.017
235. Zhang L, Prietsch SO, Mendes AP, Von Groll A, Rocha GP, Carrion L, et al. Inhaled corticosteroids increase the risk of oropharyngeal colonization by Streptococcus pneumoniae in children with asthma. Respirology. (2013) 18:272–7. doi: 10.1111/j.1440-1843.2012.02280.x
236. Doroudchi A, Pathria M, Modena BD. Asthma biologics: comparing trial designs, patient cohorts and study results. Ann Allergy, Asthma Immunol. (2020) 124:44–56. doi: 10.1016/j.anai.2019.10.016
237. Esquivel A, Busse WW, Calatroni A, Togias AG, Grindle KG, Bochkov YA et al. Effects of omalizumab on rhinovirus infections, illnesses, and exacerbations of asthma. Am J Respir Crit Care Med. (2017) 196:985–92. doi: 10.1164/rccm.201701-0120OC
238. Teach SJ, Gill MA, Togias A, Sorkness CA, Arbes SJJ, Calatroni A, et al. Preseasonal treatment with either omalizumab or an inhaled corticosteroid boost to prevent fall asthma exacerbations. J Allergy Clin Immunol. (2015) 136:1476–85. doi: 10.1016/j.jaci.2015.09.008
239. Contoli M, Ito K, Padovani A, Poletti D, Marku B, Edwards MR, et al. Th2 cytokines impair innate immune responses to rhinovirus in respiratory epithelial cells. Allergy. (2015) 70:910–20. doi: 10.1111/all.12627
240. Sabogal Piñeros YS, Bal SM, van de Pol MA, Dierdorp BS, Dekker T, Dijkhuis A, et al. Anti–IL-5 in Mild Asthma Alters Rhinovirus-induced Macrophage, B-Cell, and Neutrophil Responses (MATERIAL) A Placebo-controlled, Double-Blind Study. Am J Respir Crit Care Med. (2019) 199:508–17. doi: 10.1164/rccm.201803-0461OC
241. Rich HE, Antos D, Melton NR, Alcorn JF, Manni ML. Insights into type I and III interferons in asthma and exacerbations. Front Immunol. (2020) 11:1–13. doi: 10.3389/fimmu.2020.574027
242. Koltsida O, Hausding M, Stavropoulos A, Koch S, Tzelepis G, Übel C, et al. IL-28A (IFN-λ2) modulates lung DC function to promote Th1 immune skewing and suppress allergic airway disease. EMBO Mol Med. (2011) 3:348–61. doi: 10.1002/emmm.201100142
243. Djukanović R, Harrison T, Johnston SL, Gabbay F, Wark P, Thomson NC, et al. The effect of inhaled IFN-b on worsening of asthma symptoms caused by viral infections a randomized trial. Am J Respir Crit Care Med. (2014) 190:145–54. doi: 10.1164/rccm.201312-2235OC
244. Dhariwal J, Edwards MR, Johnston SL. Anti-viral agents: potential utility in exacerbations of asthma. Curr Opin Pharmacol. (2013) 13:331–6. doi: 10.1016/j.coph.2013.04.010
245. Pham Van L, Bardel E, Gregoire S, Vanoirbeek J, Schneider E, Dy M, et al. Treatment with the TLR7 agonist R848 induces regulatory T-cell-mediated suppression of established asthma symptoms. Eur J Immunol. (2011) 41:1992–9. doi: 10.1002/eji.201040914
246. Beeh KM, Kanniess F, Wagner F, Schilder C, Naudts I, Hammann-Haenni A, et al. The novel TLR-9 agonist QbG10 shows clinical efficacy in persistent allergic asthma. J Allergy Clin Immunol. (2013) 131:866–74. doi: 10.1016/j.jaci.2012.12.1561
247. Simoes EAF, Groothuis JR, Carbonell-Estrany X, Rieger CHL, Mitchell I, Fredrick LM, et al. Palivizumab prophylaxis, respiratory syncytial virus, and subsequent recurrent wheezing. J Pediatr. (2007) 151:34–42.e1. doi: 10.1016/j.jpeds.2007.02.032
248. Hales CM, Kit BK, Gu Q, Ogden CL. Trends in prescription medication use among children and adolescents-United States, 1999-2014. JAMA. (2018) 319:2009–20. doi: 10.1001/jama.2018.5690
249. Paul IM, Maselli JH, Hersh AL, Boushey HA, Nielson DW, Cabana MD. Antibiotic prescribing during pediatric ambulatory care visits for asthma. Pediatrics. (2011) 127:1014–21. doi: 10.1542/peds.2011-0218
250. Konstantinidis T, Tsigalou C, Karvelas A, Stavropoulou E, Voidarou C, Bezirtzoglou E. Effects of antibiotics upon the gut microbiome: a review of the literature. Biomedicines. (2020) 8:502. doi: 10.3390/biomedicines8110502
251. Arrieta MC, Stiemsma LT, Dimitriu PA, Thorson L, Russell S, Yurist-Doutsch S, et al. Early infancy microbial and metabolic alterations affect risk of childhood asthma. Sci Transl Med. (2015) 7:307ra152. doi: 10.1126/scitranslmed.aab2271
252. Russell SL, Gold MJ, Hartmann M, Willing BP, Thorson L, Wlodarska M, et al. Early life antibiotic-driven changes in microbiota enhance susceptibility to allergic asthma. EMBO Rep. (2012) 13:440–7. doi: 10.1038/embor.2012.32
253. Yang X, Feng H, Zhan X, Zhang C, Cui R, Zhong L, et al. Early-life vancomycin treatment promotes airway inflammation and impairs microbiome homeostasis. Aging. (2019) 11:2071–81. doi: 10.18632/aging.101901
254. Wheeler ML, Limon JJ, Bar AS, Leal CA, Gargus M, Tang J, et al. Immunological consequences of intestinal fungal dysbiosis. Cell Host Microbe. (2016) 19:865–73. doi: 10.1016/j.chom.2016.05.003
255. Martel MJ, Rey E, Malo JL, Perreault S, Beauchesne MF, Forget A, et al. Determinants of the incidence of childhood asthma: a two-stage case-control study. Am J Epidemiol. (2009) 169:195–205. doi: 10.1093/aje/kwn309
256. Marra F, Marra CA, Richardson K, Lynd LD, Kozyrskyj A, Patrick DM, et al. Antibiotic use in children is associated with increased risk of asthma. Pediatrics. (2009) 123:1003–10. doi: 10.1542/peds.2008-1146
257. Kozyrskyj AL, Ernst P, Becker AB. Increased risk of childhood asthma from antibiotic use in early life. Chest. (2007) 131:1753–9. doi: 10.1378/chest.06-3008
258. Ni J, Friedman H, Boyd BC, McGurn A, Babinski P, Markossian T, et al. Early antibiotic exposure and development of asthma and allergic rhinitis in childhood. BMC Pediatr. (2019) 19:225. doi: 10.1186/s12887-019-1594-4
259. Donovan BM, Abreo A, Ding T, Gebretsadik T, Turi KN Yu C, et al. Dose, timing, and type of infant antibiotic use and the risk of childhood asthma. Clin Infect Dis. (2020) 70:1658–65. doi: 10.1093/cid/ciz448
260. Zhong Y, Zhang Y, Wang Y, Huang R. Maternal antibiotic exposure during pregnancy and the risk of allergic diseases in childhood: A meta-analysis. Pediatr Allergy Immunol. (2021) 32:445–56. doi: 10.1111/pai.13411
261. Stefan MS, Shieh MS, Spitzer KA, Pekow PS, Krishnan JA, Au DH, et al. Association of antibiotic treatment with outcomes in patients hospitalized for an asthma exacerbation treated with systemic corticosteroids. JAMA Intern Med. (2019) 179:333–9. doi: 10.1001/jamainternmed.2018.5394
262. Johnston SL, Szigeti M, Cross M, Brightling C, Chaudhuri R, Harrison T, et al. Azithromycin for acute exacerbations of asthma: the AZALEA randomized clinical trial. JAMA Intern Med. (2016) 176:1630–7. doi: 10.1001/jamainternmed.2016.5664
263. Edwards MR, Walton RP, Jackson DJ, Feleszko W, Skevaki C, Jartti T, et al. The potential of anti-infectives and immunomodulators as therapies for asthma and asthma exacerbations. Allergy. (2018) 73:50–63. doi: 10.1111/all.13257
264. Terraneo S, Polverino E, Cilloniz C, Amaro R, Vennera MC, Gabarrus A, et al. Severity and outcomes of community acquired pneumonia in asthmatic patients. Respir Med. (2014) 108:1713–22. doi: 10.1016/j.rmed.2014.09.001
265. Schuijt TJ, Lankelma JM, Scicluna BP. de Sousa e Melo F, Roelofs JJ, de Boer JD, et al. The gut microbiota plays a protective role in the host defence against pneumococcal pneumonia. Gut. (2016) 65:575–83. doi: 10.1136/gutjnl-2015-309728
266. Abt MC, Osborne LC, Monticelli LA, Doering TA, Alenghat T, Sonnenberg GF, et al. Commensal bacteria calibrate the activation threshold of innate antiviral immunity. Immunity. (2012) 37:158–70. doi: 10.1016/j.immuni.2012.04.011
267. Steed AL, Christophi GP, Kaiko GE, Sun L, Goodwin VM, Jain U, et al. The microbial metabolite desaminotyrosine protects from influenza through type I interferon. Science. (2017) 357:498–502. doi: 10.1126/science.aam5336
268. Antunes KH, Fachi JL, de Paula R, da Silva EF, Pral LP, dos Santos AÁ, et al. Microbiota-derived acetate protects against respiratory syncytial virus infection through a GPR43-type 1 interferon response. Nat Commun. (2019) 10:3273. doi: 10.1038/s41467-019-11152-6
269. Ichinohe T, Pang IK, Kumamoto Y, Peaper DR, Ho JH, Murray TS, et al. Microbiota regulates immune defense against respiratory tract influenza a virus infection. Proc Natl Acad Sci U S A. (2011) 108:5354–9. doi: 10.1073/pnas.1019378108
270. LeMessurier KS, Iverson AR, Chang TC, Palipane M, Vogel P, Rosch JW, et al. Allergic inflammation alters the lung microbiome and hinders synergistic co-infection with H1N1 influenza virus and Streptococcus pneumoniae in C57BL/6 mice. Sci Rep. (2019) 9:1–16. doi: 10.1038/s41598-019-55712-8
271. Semic-Jusufagic A, Belgrave D, Pickles A, Telcian AG, Bakhsoliani E, Sykes A, et al. Assessing the association of early life antibiotic prescription with asthma exacerbations, impaired antiviral immunity, and genetic variants in 17q21: a population-based birth cohort study. Lancet Respir Med. (2014) 2:621–30. doi: 10.1016/S2213-2600(14)70096-7
272. Wong EH, Porter JD, Edwards MR, Johnston SL. The role of macrolides in asthma: current evidence and future directions. Lancet Respir Med. (2014) 2:657–70. doi: 10.1016/S2213-2600(14)70107-9
273. Gibson PG, Yang IA, Upham JW, Reynolds PN, Hodge S, James AL, et al. Effect of azithromycin on asthma exacerbations and quality of life in adults with persistent uncontrolled asthma (AMAZES): a randomised, double-blind, placebo-controlled trial. Lancet. (2017) 390:659–68. doi: 10.1016/S0140-6736(17)31281-3
274. Hiles SA, McDonald VM, Guilhermino M, Brusselle GG, Gibson PG. Does maintenance azithromycin reduce asthma exacerbations? An individual participant data meta-analysis. Eur Respir J. (2019) 54:1901381. doi: 10.1183/13993003.01381-2019
275. Brusselle GG, Vanderstichele C, Jordens P, Deman R, Slabbynck H, Ringoet V, et al. Azithromycin for prevention of exacerbations in severe asthma (AZISAST): a multicentre randomised double-blind placebo-controlled trial. Thorax. (2013) 68:322–9. doi: 10.1136/thoraxjnl-2012-202698
276. Marri PR, Stern DA, Wright AL, Billheimer D, Martinez FD. Asthma-associated differences in microbial composition of induced sputum. J Allergy Clin Immunol. (2013) 131:346s52.e3. doi: 10.1016/j.jaci.2012.11.013
277. Huang YJ, Nariya S, Harris JM, Lynch SV, Choy DF, Arron JR, et al. The airway microbiome in patients with severe asthma: associations with disease features and severity. J Allergy Clin Immunol. (2015) 136:874–84. doi: 10.1016/j.jaci.2015.05.044
278. Chung KF. Airway microbial dysbiosis in asthmatic patients: a target for prevention and treatment? J Allergy Clin Immunol. (2017) 139:1071–81. doi: 10.1016/j.jaci.2017.02.004
279. Dinos GP. The macrolide antibiotic renaissance. Br J Pharmacol. (2017) 174:2967–83. doi: 10.1111/bph.13936
280. McDonnell L, Gilkes A, Ashworth M, Rowland V, Harries TH, Armstrong D, et al. Association between antibiotics and gut microbiome dysbiosis in children: systematic review and meta-analysis. Gut Microbes. (2021) 13:1–18. doi: 10.1080/19490976.2020.1870402
281. Slater M, Rivett DW, Williams L, Martin M, Harrison T, Sayers I, et al. The impact of azithromycin therapy on the airway microbiota in asthma. Thorax. (2014) 69:673–4. doi: 10.1136/thoraxjnl-2013-204517
282. Lopes Dos Santos Santiago G, Brusselle G, Dauwe K, Deschaght P, Verhofstede C, Vaneechoutte D, et al. Influence of chronic azithromycin treatment on the composition of the oropharyngeal microbial community in patients with severe asthma. BMC Microbiol. (2017) 17:109. doi: 10.1186/s12866-017-1022-6
283. Kucuksezer UC, Ozdemir C, Cevhertas L, Ogulur I, Akdis M, Akdis CA. Mechanisms of allergen-specific immunotherapy and allergen tolerance. Allergol Int. (2020) 69:549–60. doi: 10.1016/j.alit.2020.08.002
284. Tsabouri S, Mavroudi A, Feketea G, Guibas GV. Subcutaneous and sublingual immunotherapy in allergic asthma in children. Front Pediatr. (2017) 5:82. doi: 10.3389/fped.2017.00082
285. Takaku Y, Nakagome K, Kobayashi T, Yamaguchi T, Nishihara F, Soma T, et al. Changes in airway inflammation and hyperresponsiveness after inhaled corticosteroid cessation in allergic asthma. Int Arch Allergy Immunol. (2010) 152:41–6. doi: 10.1159/000312124
286. Zissler UM, Jakwerth CA, Guerth F, Lewitan L, Rothkirch S, Davidovic M, et al. Allergen-specific immunotherapy induces the suppressive secretoglobin 1A1 in cells of the lower airways. Allergy. (2021) 76:2461–74. doi: 10.1111/all.14756
287. NOON L. Prophylactic inoculation against hay fever. Historical document. Ann Allergy. (1960) 18:287–91.
288. Nakagome K, Nagata M. Allergen immunotherapy in asthma. Pathogens. (2021) 10:1406. doi: 10.3390/pathogens10111406
289. Xu K, Deng Z, Li D, Yuan H, Liu C, Chen Z, et al. Efficacy of add-on sublingual immunotherapy for adults with asthma: a meta-analysis and systematic review. Ann Allergy Asthma Immunol. (2018) 121:186–94. doi: 10.1016/j.anai.2018.05.019
290. Lin SY, Erekosima N, Kim JM, Ramanathan M, Suarez-Cuervo C, Chelladurai Y, et al. Sublingual immunotherapy for the treatment of allergic rhinoconjunctivitis and asthma: a systematic review. JAMA. (2013) 309:1278–88. doi: 10.1001/jama.2013.2049
291. Normansell R, Kew KM, Bridgman AL. Sublingual immunotherapy for asthma. Cochrane Database Syst Rev. (2015) 2015:CD011293. doi: 10.1002/14651858.CD011293.pub2
292. Maldonado YA, O'Leary ST, Ardura MI, Banerjee R, Bryant KA, Campbell JD, et al. Recommendations for prevention and control of influenza in children, 2021-2022. Pediatrics. (2021) 148:e2021053744. doi: 10.1542/peds.2021-053745
293. Flu Vaccination Coverage United States 2020-21 Influenza Season. Available online at: https://www.cdc.gov/flu/fluvaxview/coverage-2021estimates.htm. (accessed November 14, 2021).
294. Grohskopf LA, Alyanak E, Broder KR, Blanton LH, Fry AM, Jernigan DB, et al. Prevention and control of seasonal influenza with vaccines: recommendations of the advisory committee on immunization practices-United States, 2020-21 influenza season. MMWR Recomm Reports. (2020) 69:1–24. doi: 10.15585/mmwr.rr6908a1
295. Miller EK, Griffin MR, Edwards KM, Weinberg GA, Szilagyi PG, Staat MA, et al. Influenza burden for children with asthma. Pediatrics. (2008) 121:1–8. doi: 10.1542/peds.2007-1053
296. Vasileiou E, Sheikh A, Butler C, El Ferkh K, von Wissmann B, McMenamin J, et al. Efectiveness of influenza vaccines in Asthma: a systematic review and meta-analysis. Clin Infect Dis. (2017) 65:1388–95. doi: 10.1093/cid/cix524
297. Belshe RB, Edwards KM, Vesikari T, Black SV, Walker RE, Hultquist M, et al. Live attenuated versus inactivated influenza vaccine in infants and young children. N Engl J Med. (2007) 356:685–96. doi: 10.1056/NEJMoa065368
298. Fleming DM, Crovari P, Wahn U, Klemola T, Schlesinger Y, Langussis A, et al. Comparison of the efficacy and safety of live attenuated cold-adapted influenza vaccine, trivalent, with trivalent inactivated influenza virus vaccine in children and adolescents with asthma. Pediatr Infect Dis J. (2006) 25:860–9. doi: 10.1097/01.inf.0000237797.14283.cf
299. Ashkenazi S, Vertruyen A, Arístegui J, Esposito S, McKeith DD, Klemola T, et al. Superior relative efficacy of live attenuated influenza vaccine compared with inactivated influenza vaccine in young children with recurrent respiratory tract infections. Pediatr Infect Dis J. (2006) 25:870–9. doi: 10.1097/01.inf.0000237829.66310.85
300. Gaglani MJ, Piedra PA, Riggs M, Herschler G, Fewlass C, Glezen WP. Safety of the intranasal, trivalent, live attenuated influenza vaccine (LAIV) in children with intermittent wheezing in an open-label field trial. Pediatr Infect Dis J. (2008) 27:444–52. doi: 10.1097/INF.0b013e3181660c2e
301. Ray GT, Lewis N, Goddard K, Ross P, Duffy J, DeStefano F, et al. Asthma exacerbations among asthmatic children receiving live attenuated versus inactivated influenza vaccines. Vaccine. (2017) 35:2668–75. doi: 10.1016/j.vaccine.2017.03.082
302. Nordin JD, Vazquez-Benitez G, Olsen A, Kuckler LC, Gao AY, Kharbanda EO. Safety of guidelines recommending live attenuated influenza vaccine for routine use in children and adolescents with asthma. Vaccine. (2019) 37:4055–60. doi: 10.1016/j.vaccine.2019.05.081
303. Bandell A, Ambrose CS, Maniaci J, Wojtczak H. Safety of live attenuated influenza vaccine (LAIV) in children and adults with asthma: a systematic literature review and narrative synthesis. Expert Rev Vaccines. (2021) 20:717–28. doi: 10.1080/14760584.2021.1925113
304. van Werkhoven CH, Huijts SM. Vaccines to prevent pneumococcal community-acquired pneumonia. Clin Chest Med. (2018) 39:733–52. doi: 10.1016/j.ccm.2018.07.007
305. CDC. Lung Disease including Asthma and Adult Vaccination. Available online at: https://www.cdc.gov/vaccines/adults/rec-vac/health-conditions/lung-disease.html. (accessed November 25, 2021).
306. Li L, Cheng Y, Tu X, Yang J, Wang C, Zhang M, et al. Association between asthma and invasive pneumococcal disease risk: a systematic review and meta-analysis. Allergy, Asthma Clin Immunol. (2020) 16:94. doi: 10.1186/s13223-020-00492-4
307. Castro-Rodríguez JA, Abarca K, Forno E. Asthma and the risk of invasive pneumococcal disease: a meta-analysis. Pediatrics. (2020) 145:e20191200. doi: 10.1542/peds.2019-1200
308. Inghammar M, Engström G, Kahlmeter G, Ljungberg B, Löfdahl CG, Egesten A. Invasive pneumococcal disease in patients with an underlying pulmonary disorder. Clin Microbiol Infect. (2013) 19:1148–54. doi: 10.1111/1469-0691.12182
309. Lee TA, Weaver FM, Weiss KB. Impact of pneumococcal vaccination on pneumonia rates in patients with COPD and asthma. J Gen Intern Med. (2007) 22:62–7. doi: 10.1007/s11606-007-0118-3
310. Taylor AE, Finney-Hayward TK, Quint JK, Thomas CM, Tudhope SJ, Wedzicha JA, et al. Defective macrophage phagocytosis of bacteria in COPD. Eur Respir J. (2010) 35:1039–47. doi: 10.1183/09031936.00036709
311. Hodge S, Hodge G, Jersmann H, Matthews G, Ahern J, Holmes M, et al. Azithromycin improves macrophage phagocytic function and expression of mannose receptor in chronic obstructive pulmonary disease. Am J Respir Crit Care Med. (2008) 178:139–48. doi: 10.1164/rccm.200711-1666OC
312. Simpson JL, Gibson PG, Yang IA, Upham J, James A, Reynolds PN, et al. Impaired macrophage phagocytosis in non-eosinophilic asthma. Clin Exp Allergy. (2013) 43:29–35. doi: 10.1111/j.1365-2222.2012.04075.x
313. Eisenlohr CP, Chartrand EM, Barzaga MR, Lanz MJ. Impact of pneumococcal vaccine response on asthma exacerbation frequency in young children. Immun Inflamm Dis. (2020) 8:493–6. doi: 10.1002/iid3.331
314. Devine VT, Jefferies JM, Clarke SC, Faust SN. Nasopharyngeal bacterial carriage in the conjugate vaccine era with a focus on pneumococci. J Immunol Res. (2015) 2015:394368. doi: 10.1155/2015/394368
315. Spijkerman J, Prevaes SM, van Gils EJ, Veenhoven RH, Bruin JP, Bogaert D, et al. Long-term effects of pneumococcal conjugate vaccine on nasopharyngeal carriage of S. pneumoniae, S aureus, H influenzae and M catarrhalis. PLoS ONE. (2012) 7:e39730. doi: 10.1371/journal.pone.0039730
316. Camilli R, Vescio MF, Giufrè M, Daprai L, Garlaschi ML, Cerquetti M, et al. Carriage of Haemophilus influenzae is associated with pneumococcal vaccination in Italian children. Vaccine. (2015) 33:4559–64. doi: 10.1016/j.vaccine.2015.07.009
317. Wiertsema SP, Kirkham LA, Corscadden KJ, Mowe EN, Bowman JM, Jacoby P, et al. Predominance of nontypeable haemophilus influenzae in children with otitis media following introduction of a 3 + 0 pneumococcal conjugate vaccine schedule. Vaccine. (2011) 29:5163–70. doi: 10.1016/j.vaccine.2011.05.035
318. WHO: COVID-19 Vaccine Tracker and Landscape. 2021 Available online at: https://www.who.int/publications/m/item/draft-landscape-of-covid-19-candidate-vaccines. (accessed January 06, 2022).
319. Sadarangani M, Marchant A, Kollmann TR. Immunological mechanisms of vaccine-induced protection against COVID-19 in humans. Nat Rev Immunol. (2021) 21:475–84. doi: 10.1038/s41577-021-00578-z
Keywords: allergic asthma, respiratory infection, asthma therapy, microbiome, respiratory virus, eosinophils
Citation: Flores-Torres AS and Samarasinghe AE (2022) Impact of Therapeutics on Unified Immunity During Allergic Asthma and Respiratory Infections. Front. Allergy 3:852067. doi: 10.3389/falgy.2022.852067
Received: 10 January 2022; Accepted: 21 February 2022;
Published: 25 March 2022.
Edited by:
Lisa A. Miller, University of California, Davis, United StatesReviewed by:
Ulrich Matthias Zissler, Technical University of Munich, GermanyDawn C. Newcomb, Vanderbilt University, United States
Copyright © 2022 Flores-Torres and Samarasinghe. This is an open-access article distributed under the terms of the Creative Commons Attribution License (CC BY). The use, distribution or reproduction in other forums is permitted, provided the original author(s) and the copyright owner(s) are credited and that the original publication in this journal is cited, in accordance with accepted academic practice. No use, distribution or reproduction is permitted which does not comply with these terms.
*Correspondence: Amali E. Samarasinghe, YW1hbGkuc2FtYXJhc2luZ2hlQHV0aHNjLmVkdQ==