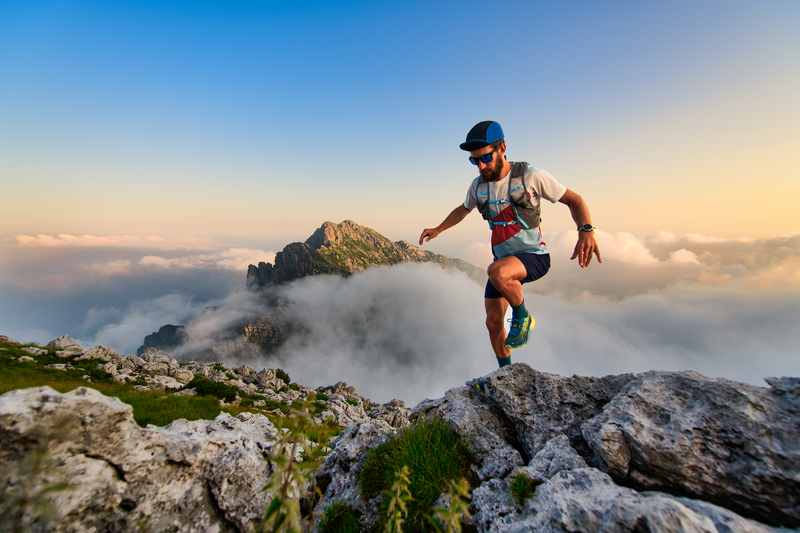
95% of researchers rate our articles as excellent or good
Learn more about the work of our research integrity team to safeguard the quality of each article we publish.
Find out more
ORIGINAL RESEARCH article
Front. Aging Neurosci. , 13 March 2025
Sec. Neurocognitive Aging and Behavior
Volume 17 - 2025 | https://doi.org/10.3389/fnagi.2025.1514594
This article is part of the Research Topic Enhancing Gait Therapy with Artificial Intelligence: Current Trends and Future Prospects View all articles
Purpose: Motor-cognitive exergames may be beneficial for addressing both motor and cognitive residual impairments in chronic stroke, however, effective training schedules are yet to be determined. Therefore, this study investigates the effects of a concept-guided, personalized, motor-cognitive exergame training on cognitive functions and gait in chronic stroke survivors.
Methods: In this single-blind, randomized, controlled trial, stroke survivors (at least six-months post-stroke and able to perform step-based exergaming) were allocated either to the intervention (usual care + concept-guided, personalized, motor-cognitive exergame training) or the control group (usual care only). Global cognitive functioning was primarily targeted, while health-related quality of life (HRQoL), cognitive functions, mobility, and gait were evaluated secondarily. Analyses were performed with linear-mixed effect models.
Results: Effects on global cognitive functioning were non-significant, with no differences between responders (participants exhibiting a clinically relevant change) and non-responders (participants exhibiting no clinically relevant change). Among secondary outcomes, the mobility domain of the HRQoL questionnaire, intrinsic visual alertness, cognitive flexibility, working memory, and outdoor walking speed as well as swing width (unaffected side) showed significant interaction effects in favour of the exergame group.
Discussion: Additional exergaming helped maintaining global cognitive functioning and showed encouraging effects in mobility and cognitive outcomes. Responders and non-responders did not differ in adherence, baseline values or age. Enhancing the frequency and intensity of sessions could unlock more substantial benefits. Adopting a blended therapy approach may be key to maximizing positive effects.
Clinical trial registration: clinicaltrials.gov, identifier NCT05524727.
Stroke is a major cause of long-term disability in adults (Katan and Luft, 2018; Johnson et al., 2019), with common sequelae being cognitive and motor impairments (Sun et al., 2014; Ursin et al., 2019). In many stroke survivors, these impairments reside in the long-term, which hampers their daily-life functioning, independence, psychological and health-related quality of life (Nakling et al., 2017; Hotter et al., 2018; Katan and Luft, 2018; Sennfalt et al., 2019). Cognitive impairments are highly prevalent in chronic stroke [up to 84% (Mahon et al., 2017)], with processing speed, executive functions, memory, and visuospatial functions being the domains most often affected (Cumming et al., 2013; Middleton et al., 2014). Nevertheless, rehabilitation of cognitive functions has been neglected for a long time (Jokinen et al., 2015; McDonald et al., 2019), and residual cognitive impairments are an important unmet need in chronic stroke survivors (Pollock et al., 2012; Hotter et al., 2018; Virani et al., 2020; Rudberg et al., 2021). Additionally, up to 80% of stroke survivors experience hemiparesis (Barker and Mullooly, 1997; Patten et al., 2004). Hemiparesis alters spatiotemporal gait parameters including shorter stride length, lower cadence, and higher asymmetry, which can reduce gait speed, walking efficiency, and mobility (Olney and Richards, 1996; Katan and Luft, 2018).
Post-stroke cognitive and motor impairments are linked, as they share structural and functional roots in the nervous system (Verstraeten et al., 2016; Ursin et al., 2019). Motoric-Cognitive Risk (MCR) syndrome, which has been associated with stroke (Verghese et al., 2013; Beauchet et al., 2018), describes the collective deterioration and mutual influence of cognitive functions and specifically gait. This raises the idea that cognitive and gait impairments could also be addressed together to improve collectively (Verstraeten et al., 2016; Li et al., 2018; Ursin et al., 2019; Montero-Odasso et al., 2020). The guided plasticity facilitation model (Kraft, 2012; Fissler et al., 2013) suggests that the combination of physical and cognitive training specifically triggers neuroplasticity, which is key in stroke rehabilitation (Maier et al., 2019). Combined motor-cognitive interventions may thus be a promising approach to address cognitive deficits and gait impairments in stroke (Herold et al., 2018; Yang and Wang, 2021). Confirming the theory, motor-cognitive interventions have been found superior in improving motor and cognitive functions in healthy older adults (Bamidis et al., 2015; Lauenroth et al., 2016; Levin et al., 2017; Wollesen et al., 2020; Rieker et al., 2022; Teraz et al., 2022) and neurological populations (Gavelin et al., 2020; Sun et al., 2021; Zhou et al., 2021).
Exergames are a promising type of combined motor-cognitive training (Huber et al., 2022a), as the gamified training fosters motivation and enjoyment, which was attributed with higher adherence (Sailer et al., 2017; Valenzuela et al., 2018; Yoong et al., 2024). Exergames have been suggested as adjunct to standard stroke rehabilitation, especially for long-term and repetitious therapy (Chan et al., 2022; Zhang et al., 2022; Wicaksono et al., 2023). Potentially beneficial effects of exergames on cognitive and physical functioning have been reported in several systematic reviews in healthy older adults (Stojan and Voelcker-Rehage, 2019; Fang et al., 2020; Pacheco et al., 2020; Janhunen et al., 2021; Suleiman-Martos et al., 2021; Yen and Chiu, 2021), as well as in neurological and general populations (Stanmore et al., 2017; Mura et al., 2018; Calafiore et al., 2021; Prosperini et al., 2021). In chronic stroke, exergames have been reported to beneficially affect motor functions including balance, mobility, and gait (Chan et al., 2022; Huber et al., 2022a; Ghazavi Dozin et al., 2024). However, effective training schedules remain to be determined, as exergame interventions in previous stroke studies were mainly implemented in an unstructured manner, while description of and rationales for exercise variables and personalized schedules were insufficient (Cano Porras et al., 2018; Stojan and Voelcker-Rehage, 2019; Huber et al., 2022a; Rüth et al., 2023). Regarding cognitive functions, a recent systematic review revealed that exergame trainings had a beneficial effect in the acute phase of stroke, while no effect was found for chronic stroke (Duta et al., 2023). Notably, the subgroup analysis in chronic stroke included only two studies with small sample sizes and short intervention durations (Duta et al., 2023). Both reported on the same exergame intervention, which was performed seated. However, step-based exergaming in a standing position may be most beneficial for improving cognitive functions and gait (Tahmosybayat et al., 2018; Manser and de Bruin, 2021; Hou and Li, 2022; Manser and de Bruin, 2024). Hence, the effects of (step-based) exergames on cognitive functions in chronic stroke should be further investigated. Besides cognitive functions, detailed spatiotemporal gait parameters, real-world walking, and dual-task functions are underrepresented outcomes in studies investigating exergames (Gallou-Guyot et al., 2020; Huber et al., 2022a; Stretton et al., 2022). To address these research gaps, we developed an evidence-based concept for PErsonalized MOtor-Cognitive exergame training in chronic Stroke [PEMOCS, (Huber et al., 2024b)]. The aim of this study was to evaluate the effects of exergame training guided by the PEMOCS concept on cognitive functions, health-related quality of life, single- and dual-task walking mobility, as well as indoor and outdoor gait in community-dwelling chronic stroke survivors.
The PEMOCS study was a single-blind, parallel, randomized, controlled trial in three hospitals / rehabilitation centres in Canton Zurich (Switzerland). Participants signed written informed consent before any study procedures started. After the baseline assessments (T0), participants were 1:1 randomly allocated to the intervention group (usual care + concept-guided, personalized, motor-cognitive exergame training) or the control group (usual care only). After the 12-week intervention period, post-intervention assessments (T1) were performed, followed by a 12-week follow-up period (usual care only in both groups), and the follow-up assessments (T2). The initial protocol was adhered to during the trial. The study protocol was approved by the ethics committee of Canton Zurich (KEK Zürich, Switzerland), registered on clinicaltrials.gov (NCT05524727), and published (Huber et al., 2024a). CONSORT 2010 guidelines were followed for reporting (Schulz et al., 2010).
Randomization was stratified by sex (Sohrabji et al., 2017; Dong et al., 2020) and cognitive impairment absent or present [MoCA score ≥ 24 or < 24, respectively (Chiti and Pantoni, 2014; Shi et al., 2018; Potocnik et al., 2020)]. It was performed using the randomization module within REDCap (Harris et al., 2009), which was used for the electronic case report forms (eCRF). A senior researcher, who was not involved in the study otherwise, created the randomization list following the instructions provided by REDCap (2018). Outcome assessors were blinded to group allocation, while care providers and participants were not. Care providers enrolled participants and assigned them to the groups.
Eligible were adult, chronic stroke survivors (≥ 18 years old, ≥ 6 months post-stroke), who were able to stand for 3 min, walk 10 m without personal assistance [Functional Ambulation Category ((Mehrholz et al., 2007), FAC ≥ 3)], follow a two-stage command, and give informed consent as documented by own signature. Excluded were people who were unable or unwilling to give informed consent, suffered from other neurological diseases except for cognitive deficits or dementia, presented clinical contra-indications against the study intervention, were unable to perform the study intervention or the primary outcome test [Montreal Cognitive Assessment (Nasreddine et al., 2005), MoCA], or were overlappingly enrolled in another clinical trial. All study visits including assessments and training sessions took place in the study centres.
The intervention group received concept-guided, personalized, motor-cognitive exergame training additionally to usual care. The exergame training was performed twice per week for 30–40 min over 12 weeks, resulting in 840 min of total training time (Huber et al., 2024b). The control group received no additional intervention to usual care, but weekly phone calls, to balance for contact to the study team. These phone calls consisted of 5- to 10-minute conversations covering the questions to gather further activity outcomes (see below). Among these further activity outcomes, amount, intensity and content of each participant’s usual care was inquired as this can vary considerably from patient to patient. Depending on type and severity of remaining impairments (among other determinants), usual care for chronic stroke survivors in Switzerland can range from no therapies to several physical, occupational and / or speed therapy sessions (usually lasting 45 min) a week.
A concept for PErsonalized MOtor-Cognitive exergame training in chronic Stroke (PEMOCS) was specifically developed for this study and detailed elsewhere (Huber et al., 2024b). It has the aim to provide an optimal exergame training load for inducing neuroplasticity, cognitive and motor learning in each individual participant (Huber et al., 2024b). In short, the PEMOCS concept is based on Gentile’s Taxonomy for Motor Learning (Gentile, 1987), which was extended by a cognitive dimension for the use in exergame interventions. The taxonomy is a table of skill-categories and difficulty levels, to which the different games and game versions were assigned. By focusing on the most impaired cognitive domain of each individual, and applying individualized progression, the PEMOCS concept enables the personally tailored application of an exergame intervention (Huber et al., 2024b). Participants received one-to-one training sessions, which were planned individually and supervised by trained movement scientists of the study team. The motor-cognitive exergame training was delivered using the exergame system “Dividat Senso” (Dividat AG, Schindellegi, Switzerland, Figure 1). It comprises of a pressure-sensitive plate with handrails on three sides, which is placed in front of a screen on eye level. Cognitively challenging video games are presented on the screen, and stepping tasks are performed on the plate to interact with the games. Different games and game settings were available to train various cognitive and motor functions (Huber et al., 2024b).
Figure 1. Study device in action: the Dividat Senso including pressure-sensitive plate, handrails in use, and the screen showing targets, one of the motor-cognitive exergames.
All primary and secondary outcomes were collected at the three measurement time points (T0, T1, T2) by blinded assessors. Study data were collected and managed using REDCap electronic data capture tools hosted at ETH Zurich (Harris et al., 2009; Harris et al., 2019). REDCap (Research Electronic Data Capture) is a secure, web-based software platform designed to support data capture for research studies, providing (1) an intuitive interface for validated data capture; (2) audit trials for tracking data manipulation and export procedures; (3) automated export procedures for seamless data downloads to common statistical packages; and (4) procedures for data integration and interoperability with external sources. For a more detailed description of all outcomes and their conduct, see the protocol paper, which also presents definitions of all outcome variables (Tables 3, 4 in Huber et al., 2024a). The primary outcome was global cognitive functioning assessed by the total score of the Montreal Cognitive Assessment [MoCA (Nasreddine et al., 2005)]. The MoCA is a structured interview comprising of sub-tests assessing attention, executive functions, working memory, short-term memory recall, visuospatial skills, and orientation (Chiti and Pantoni, 2014). To prevent learning effects, three different versions of the test exist, which were used at the three measurement time points in this study.1 Blinded assessors were certified for conduction of the MoCA test (see text footnote 1).
Secondary outcomes were health-related quality of life, cognitive functions including alertness, processing speed, executive and visuospatial functions, single- and dual-task mobility, as well as indoor and outdoor walking. Participants filled the German Stroke Impact Scale (SIS) 3.0, which covers eight domains (strength, memory/thinking, emotion, communication, ADL/IADL, mobility, hand function, and participation) and the perceived state of recovery [0 to 100% (Duncan et al., 1999)]. Computer-based cognitive tests were performed in the Vienna Test System (VTS, Schuhfried GmbH, Mödling, Austria), including a simple reaction test [SRT (Zimmermann and Fimm, 1992; Sturm, 2006; Spikman and van Zomeren, 2010), “WAFA” within the VTS], the Trail-making test [TMT (Reitan, 1958; Tombaugh, 2004; Bowie and Harvey, 2006), “TMT – Langensteinbacher Version” within the VTS], the Stroop Interference test [(Faria et al., 2015; Scarpina and Tagini, 2017), “STROOP” within the VTS], the 2-back test [NBT (Owen et al., 2005; Schellig and Schuri, 2009; León-Domínguez et al., 2015; Gajewski et al., 2018), “NBV” within the VTS], and a mental rotation test [MRT (Shepard and Metzler, 1971), “3D” within the VTS]. The Timed-up-and-go test was performed in single-task (TUG) and dual-task (TUG-Cogn) mode (Ng and Hui-Chan, 2005; Yang et al., 2016; Ng et al., 2017). As cognitive task, participants subtracted three from a random number between 50 and 100 and not part of the row of three (Yang et al., 2016; Pumpho et al., 2020). To strive real dual-task (simultaneous) performance, participants were instructed to try and not prioritize one task over the other (“Please neither stop walking nor stop calculating throughout the whole trial.”). The cognitive single-task was performed until they reached 0 or until 60 s were complete (Huber et al., 2024a). To assess gait, a 10-Meter Walk Test [10MWT (Cheng et al., 2020)] and an Outdoor Walking Assessment [OWA (Huber et al., 2022b)] were performed. The 10MWT was performed indoors at preferred and fast walking speed. The OWA was performed on a flat 400-meter outdoor route without stairs walking at a preferred walking speed. All gait assessments were timed using a stopwatch, while inertial gait sensors (Physilog® sensors, Gait Up SA, Lausanne, Switzerland) measured spatiotemporal gait parameters during the 10MWT preferred and the OWA. From the measured parameters, the gait variability index (Gouelle et al., 2013) for both legs, the asymmetry index (Anker, 2019), and the walk ratio (Bogen et al., 2018) were calculated. The gait variability index was determined following published instructions (Gouelle et al., 2013) (Supplementary material S1 and R scripts in https://doi.org/10.5281/zenodo.14849242). The walk ratio was calculated using the formulas in Bogen et al. (2018).
Intervention outcomes included compliance (completed sessions/offered sessions) and adherence rates (completed training time/offered training time), reasons for not attending or aborting training sessions, as well as the ratings of perceived motor-cognitive task difficulty and perceived performance provided in the training sessions. Further activity outcomes were inquired weekly (intervention group: at one of the study appointments, control group: during the phone calls) to gather information on physical and cognitive therapies (usual care), as well as physical and cognitive leisure activities. Participants reported on how many days per week, for how long, and at what perceived intensity they had performed the therapies and activities (Huber et al., 2024a).
Baseline factors included demographics, further participant characteristics, stroke diagnosis details, and several clinical characteristics, namely the Functional Ambulation Category [FAC (Mehrholz et al., 2007)], the initial and current National Institute of Health Stroke Scale [NIHSS (Kwah and Diong, 2014)], the Lower Extremity component of the Fugl-Meyer Assessment [FMA-LE (Fugl-Meyer et al., 1975)], and the Berg Balance Scale [BBS (Blum and Korner-Bitensky, 2008)]. The Charlson Comorbidity Index [CCI (Charlson et al., 2022)] was determined from self-reported health data. The initial NIHSS was collected from patient files, or if unavailable, retrospectively determined using the algorithm presented in Williams et al. (2000).
The target sample size was 38 participants, determined by an a-priori sample size estimation based on existing systematic reviews on the effects of exergame and motor-cognitive training interventions on global cognitive functions in stroke and comparable populations (Zhu et al., 2016; Stanmore et al., 2017; Gavelin et al., 2020; Soares et al., 2021; Yen and Chiu, 2021; Yu and Chan, 2021). A small to medium effect size (f = 0.21) for global cognitive functions was anticipated and the following parameters entered in G*Power; α-level = 0.05, power = 0.80, number of groups = 2, number of measurements = 3, correlation among rep measures = 0.5, non-sphericity correction = 1. Withdrawals were replaced with further recruitment.
Statistical analyses were performed using RStudio open-source software [Boston, United States, (RStudio_Team, 2020), Version 4.3.1] and Microsoft Excel (Microsoft Corporation, 2016). Data were explored, checking on distributions with the Shapiro–Wilk test (Field et al., 2012). Appropriate descriptives were obtained for all variables. Differences between groups at baseline were tested using independent t-tests (for interval data allowing parametric testing), Wilcoxon rank-sum tests (for interval data requiring non-parametric testing), Chi-square tests, or Fisher exact tests [for ordinal / categorical data with frequencies >5 or ≤ 5, respectively (Kim, 2017)]. For intervention outcomes, means overall and per week were calculated. For further activity outcomes, within participant means over the intervention and follow-up periods were calculated and compared between the two groups using independent t-tests or Wilcoxon rank-sum tests.
Interaction effects of interval data were analysed using linear mixed-effects models (LMEM, lmer-function of the lme4 package in R). Models were built by starting with a basic model including main fixed effects (group, time) and the interaction effect (group*time). Potential covariates (for choice, see below) were added first individually and then combined (Meteyard and Davies, 2020). The basic and covariate models were compared using the anova-function in R to choose the final model for each outcome [model with lowest AIC and BIC (Akaike and Bayesian Information Criteria, respectively) values (Meteyard and Davies, 2020)]. LMEMs have been recommended for data analysis of intervention studies despite frequent violation of assumptions in such data sets and have been reported to be sufficiently robust to smaller sample sizes (N < 50) and violated assumptions (Wiley and Rapp, 2019; Schielzeth et al., 2020). Nevertheless, the following assumptions were tested; (1) homogeneity of variance using Levene’s test, (2) linearity using ZRESID/ZPRED plots, (3) normality of residuals using the DHARMa package in R (Harting, 2022) and visually inspecting histograms of the residuals, (4) normality of random-effects by visually inspecting histograms of the random-effects, and (5) multicollinearity by calculating the variance inflation factor (VIF) (Field et al., 2012). In case severe violation of one or several assumptions was detected, these violations were reported alongside with the model results. Log-transformation of the data in violated models was considered, however, it was remarked that back-transformed model coefficients (b, standard errors) and effect sizes were not comparable to the original data and other models, as described in Feng et al. (2014). Therefore, no further action was taken in case of assumption violations. Final models, information on the process of model choice, as well as results of the assumption tests were documented in supplements (Meteyard and Davies, 2020). Effect sizes for the models were calculated as r (Bravais-Pearson correlation coefficient) = sqrt(t2 / (t2 + df)) (Field et al., 2012).
Ordinal outcomes were analysed with LMEMs of the family “poisson” (glmer-function of the lme4 package in R). Before analysis, clear outliers that could be associated to problems with handling the computer-based tests were replaced with the next higher / lower value ± 1 (Field et al., 2012). R scripts for all LMEMs are available online at https://doi.org/10.5281/zenodo.14849242.
All primary and secondary outcomes were primarily analysed following the intention-to-treat (ITT) principle. In these ITT analyses, missing data due to withdrawals were imputed using the last-observation-carried-forward method. Single missing data points (e.g., one assessment was not performed because of technical issues), were not imputed, as LMEMs can also be fitted with some missing data (Field et al., 2012; Gabrio et al., 2022). In case, a participant lacked data for an outcome at two or three time points, they were excluded from these analyses. Secondarily, per-protocol analyses were performed excluding individuals who withdrew from the study during the intervention period (T0–T1) or attended less than 85% of the total training sessions / time [for rationale, see Huber et al. (2024a)].
Potential covariates in the LMEMs were pre-defined as follows; (1) any baseline characteristic exhibiting a difference between groups, (2) parameters, which are established moderators of treatment effects and predictors of outcomes after stroke in literature. Therefore, age was considered as covariate in all analyses (Falck et al., 2019; Saa et al., 2021). Years of education was chosen for the secondary cognitive outcomes (Ojala-Oksala et al., 2012; Basak et al., 2020; Umarova et al., 2021; Hua et al., 2022). It was not a covariate in the analysis of the primary outcome, as the MoCA considers years of education for the total score (Nasreddine et al., 2005). Secondly, the FMA-LE score was chosen for the gait and mobility outcomes (Kollen et al., 2005; Burke et al., 2014; Rech et al., 2020; Selves et al., 2020). Sex and cognitive impairment were not considered as these variables were accounted for by the randomization stratification. Moreover, time post-stroke, which is often reported to be a moderator / predictor of post-stroke outcomes, was not considered for two reasons. Firstly, most studies that report time since stroke to be a moderator / predictor discriminate between (sub-)acute and chronic stroke (Lam et al., 2010; Burke et al., 2014; Prat-Luri et al., 2020), however, in our study, all participants were chronic. Secondly, there is an ongoing debate whether time post-stroke in the chronic stage is really a moderator of treatment effects (Rogers et al., 2018), and two recent studies found no moderating effect on treatment effects, and no correlations between time since stroke and spatiotemporal gait parameters, respectively (Gama et al., 2017; Zeng et al., 2023).
Within-group changes were tested using Wilcoxon signed rank tests (wilcox.test function in R, setting ‘paired = TRUE’) and effect sizes were calculated as r (Bravais-Pearson correlation coefficient) = z / sqrt(N) (Field et al., 2012). Additionally, responder analyses were performed for the MoCA, and those outcomes that would exhibit a significant interaction effect (Snapinn and Jiang, 2007). For a responder analysis, participants are divided into responders (exhibiting changes greater than a minimal clinically important difference, MCID) and non-responders (showing no clinically important change), and ratios thereof are compared between groups. To potentially identify characteristics of responders, we additionally compared baseline and covariate (see above) values of responders and non-responders in the intervention group with a Wilcoxon rank sum test.
Significance for all analyses was set to p < 0.05. No p-value adjustments for multiple testing were performed as this study had one primary outcome, while secondary analyses were rather exploratory (Feise, 2002). Bravais-Pearson correlation coefficients (r) were interpreted as small (r < 0.3), medium (0.3 ≤ r < 0.5), and large (r ≥ 0.5) (Field et al., 2012).
No changes to the initial protocol were made in terms of study procedures (Huber et al., 2024a), however, the following changes were made in the analysis. (1) A responder analysis was also performed for the MoCA, even though no significant interaction effect was found. (2) Gait variability was reported as the Gait Variability Index [GVI, (Gouelle et al., 2013), Supplementary material S1] instead of individual stride time / length variability. The GVI is a composite score of gait variability, which considers the nine most relevant parameters for gait variability (Gouelle et al., 2013). This has the advantage that one composite score replaced nine parameters, which may even add small changes in individual variability parameters, which would not be detected individually (Gouelle et al., 2013). (3) In the NBT, the reaction time of the mistakes (RTmistakes) was no longer an outcome. The N in the analysis became very small, as several participants made no mistakes in this test. As RTmistakes is less relevant than other outcomes of the test (main outcomes: number of omissions and mistakes, reaction times of the correct answers (Schellig and Schuri, 2009), it was removed. (4) For the TUG-Cogn, it was not possible to report the cognitive dual-task effect due to a protocol limitation we only noticed when analysing the data. Particularly, the time given for the cognitive single-task should have been matched to participants’ time to complete the dual-task (Yang et al., 2016). However, participants were given 60 s to complete the single task. This time mismatch led to unrealistic values when calculating the cognitive dual-task effect. Therefore, it was decided to report single- and dual-task response rates separately instead of the cognitive dual-task effect. The motor task and dual-task effect were not affected by this protocol limitation. (5) Participants who performed the OWA twice were not excluded from the OWA analyses, as fewer missing data was present than expected. This way, valuable collected outcome data was considered for the analysis instead of being excluded.
Recruitment ran between September 2022 and October 2023, and data collection was completed by April 2024. The study was completed as planned. Of 926 screened stroke survivors, 46 were included. The most frequent reason for non-inclusion was ineligibility (N = 766). Reasons for not participating among those who would have been eligible were “not being interested in study participation” (N = 58), “too high time consumption of the study” (N = 41), and “would be interested but cannot reach the study centre” (N = 4, all women). Women were underrepresented in all phases of recruitment and inclusion, namely screening on eligibility (ratio women / men: 0.73), contact for participation (ratio women / men: 0.43), and inclusion (ratio women / men: 0.24). Twenty-four and twenty-two participants were allocated to the intervention group and the control group, respectively. Forty-one participants completed the intervention period and thirty-seven the whole study duration, resulting in an attrition rate of 19.6%. In the intervention group, four participants withdrew during the intervention period (T0–T1), and none in the follow-up period (T1–T2). Reasons for withdrawal were (1) a temporally but not causally related adverse event (compare ‘Adverse events’, N = 1), (2) an unrelated serious adverse event (N = 1), (3) remarking that scheduling the training sessions in daily life was not possible (N = 1), and (4) not reported (withdrew without giving a reason, N = 1). In the control group, one participant withdrew during the intervention period (T0–T1), and four in the follow-up period (T1–T2). Reasons for withdrawal were (1) not reported (withdrew without giving a reason, N = 1), (2) boredom and / or discomfort with the phone calls (N = 3), and (3) an unrelated serious adverse event (compare ‘Adverse events’, N = 1). Intention-to-treat analyses included 46, per-protocol analyses 36 participants. Three participants were excluded from the SIS analyses, as they withdrew before initially answering the questionnaire. One participant was excluded from the TUG-Cogn, MRT, Stroop, and NBT analyses as he was not able to perform these assessments. Three participants were excluded from 10MWT-fast analyses, and three from the OWA analyses, because they did not want to perform the assessment. The participant flow is shown in Figure 2.
Participants were on average 66 years old. Men were over-represented in the study (women: 9 / men: 37), however, both sexes were equally stratified over both groups. Approximately, a fifth of participants (19.6%) had suffered a haemorrhagic stroke, and there was a wide range of stroke chronicity in the sample (6–222 months post-stroke). Participants were highly educated (15 years of education, range: 8–24). Most participants showed neither cognitive nor physical impairments at baseline. However, 13 were cognitively impaired [MoCA <24, (Chiti and Pantoni, 2014)], 3 showed reduced motor function [FMA-LE < 21, (Kwong and Ng, 2019)] and 6 had restrictions in community ambulation [gait speed ≤0.78 m/s, (Bijleveld-Uitman et al., 2013)]. Baseline characteristics are summarized in Table 1. Median values per group of all outcomes at the three time points are shown in Supplementary Tables S1–S3.
The MoCA total score remained stable in both groups, and there were no interaction effects (Figure 3, Supplementary Tables S4, S5, S10). The responder analysis was performed with 1.22 points as MCID (Wu et al., 2019). The rate of responders in the intervention group (responders / non-responders: 0.40 / 0.60) was higher compared to the control group (responders / non-responders: 0.20 / 0.80, Table 2). It did not considerably change when only adhering participants were considered (responders / non-responders: 0.44 / 0.56, Table 2). There was no significant difference between responders and non-responders in baseline MoCA score (p = 0.35) or age (p = 0.94, Table 2).
Figure 3. Median MoCA scores with IQRs from the ITT analyses of both groups over time. IQR, Interquartile ranges; MoCA, Montreal Cognitive Assessment; T1, Post-intervention measurements; T2, Follow-up measurements.
The SIS domain Mobility showed a significant interaction effect (T2, ITT: p = 0.03, r = 0.24; PP: p = 0.06, r = 0.23; Figure 4A, Supplementary Tables S6, S7). The exergame group reported a significant improvement in perceived mobility with a large effect size, while the control group showed no changes in this domain (Table 3, Supplementary Table S10). The responder analysis was performed using 4.5 points as MCID (Lin et al., 2010). It showed that the rate of responders in the exergame group (responders / non-responders: 0.45 / 0.55) was higher compared to the control group (responders / non-responders: 0.25 / 0.75, Table 2). Responders showed significantly lower (p < 0.001) SIS Mobility values at baseline, while there was no difference in age (p = 0.32, Table 2).
Figure 4. Median SIS-mobility scores (A), reaction times in intrinsic visual alertness (B), outdoor gait speed (C), and outdoor swing width values (D) with IQRs from the ITT analyses of both groups over time. IQR, Interquartile ranges; OWA, Outdoor Walking Assessment; SIS, Stroke Impact Scale; SRT, Simple Reaction Test; T1, Post-intervention measurements; T2, Follow-up measurements.
Among the secondary cognitive assessments, a significant interaction effect in favour of the exergame group was found (Supplementary Tables S4, S5) in reaction time in the SRT—intrinsic visual alertness (T2, ITT: p = 0.02, r = 0.26; PP: p = 0.04, r = 0.25, Figure 4B). Additionally, the per-protocol analyses showed significant interaction effects in favour of the exergame group for mistakes in TMT-B (T1, p = 0.01; T2, p = 0.02, Figure 5A, Supplementary Table S5) and in mistakes in the NBT (T2, p = 0.02, Figure 5B, Supplementary Table S5). No responder analyses were performed for these outcomes, as no clinically relevant changes could be identified. In the MRT, both groups significantly improved in accuracy with large (exergame group) and medium (control group) effect sizes, respectively (Table 3, Supplementary Table S10).
Figure 5. Median mistakes in the TMT-B (A) and NBT (B) with IQRs from the PP analyses of both groups over time. IQR, interquartile ranges; NBT, N-back test; TMT, trail-making test; T1, post-intervention measurements; T2, follow-up measurements.
The TUG(-Cogn) revealed no significant interaction effects, however, several significant within-group changes with medium to large effect sizes, which demonstrate performance improvements in single- and dual-task mobility in both groups (Table 3, Supplementary Table S10). In the 10MWT, there were no significant interaction effects for any parameter (Supplementary Tables S8, S9). In the 10MWT-fast, both groups significantly improved with medium to large effect sizes (Table 3, Supplementary Table S10). In outdoor gait speed, there was a significant, small interaction effect in favour of the exergame group (T1, ITT: p = 0.02, r = 0.25; PP: p = 0.11, r = 0.20, Figure 4C, Supplementary Tables S8, S9). The responder analysis was performed with 0.175 m/s as MCID (Fulk et al., 2011). It showed that the rate of responders in the exergame group (responders / non-responders: 0.2 / 0.8) was higher compared to the control group (responders / non-responders: 0.15 / 0.85, Table 2). OWA gait speeds at baseline, age and baseline FMA-scores in responders and non-responders were not significantly different (Table 2). Additionally, a significant interaction effect with a medium size favouring the exergame group was found for swing width unaffected measured outdoors (T1, ITT: p = 0.004, r = 0.31; PP: p = 0.02, r = 0.29 / T2, ITT: p = 0.003, r = 0.33; PP: p = 0.007, r = 0.33, Figure 4D, Supplementary Tables S8, S9). Furthermore, the exergame group showed significant improvements in gait speed, stride length affected and stride time unaffected indoors as well as in cadence, stride time affected, and stride time unaffected outdoors, while the control group showed a significant improvement only in cadence indoors (Table 3, Supplementary Table S10).
Intervention and further activity outcomes: Mean compliance (89.8%) and adherence (89.1%) rates were high (Supplementary Table S11, considering participants who completed the study). Reasons for not attending or aborting training sessions were all intervention-unrelated, including sickness, holidays, working appointments, or having forgotten the appointment. The mean perceived task difficulty of the participants started low, moved below the targeted range in weeks 3 to 6, and at the lower edge of the targeted range in weeks 7 to 12 (Figure 6A). The mean perceived performance started within the targeted range, moved at its upper edge throughout weeks 4 to 10, and raised higher in the last 2 weeks of the intervention (Figure 6B). Further activity outcomes showed that 18 participants received no usual care throughout the study, while the remaining participants received on average (median) 38 min of therapy per week (Supplementary Table S11). Ten participants received both physical and cognitive therapy, 12 received physical therapy only and 2 received cognitive therapy only. Overall, the average amount of physical therapy was higher compared to the average amount of cognitive therapy (Supplementary Table S11). In leisure time, participants were highly physically and cognitively active (median: 180–300 min of activity on 3–5 day per week, Supplementary Table S11). None of the further activity outcomes showed differences between the groups (Supplementary Table S11).
Figure 6. Median ratings of perceived task difficulty (A) and perceived performance (B) of all participants over time. Training number: week.session, e.g., 2.2 → week 2, second training in this week.
A mild and possibly (temporally but not causally) related AE was recorded in a participant with pre-existing heart condition. This participant experienced an uncomfortable but tolerable feeling in the chest / heart region during the more exhaustive parts of the measurement and training sessions, which decomposed after stopping the activities. The participant reported experiencing the same feelings when performing moderately to highly intense activities in daily life, but nevertheless withdrew. Another mild and unlikely related AE occurred in a participant, who experienced mild to moderate (tolerable) back pain during a few training sessions. The participant reported that the pain had been present already before the study and fluctuated, which was not attributable to the training sessions (pain was not stronger after the sessions). The training was adapted to the participant’s condition from the day of reporting; jumps were no longer integrated, and the cognitive load was reduced to enable focus on core stability during exercises. Furthermore, four unrelated SAEs (hospitalisations, for which a causal relationship to the study could be ruled out) and several unrelated AEs (e.g., flu, study-unrelated pain) occurred.
This single-blind, randomized, controlled trial investigated the effects of a concept-guided, personalized, motor-cognitive exergame training (PEMOCS) added to usual care compared to usual care alone on global cognitive function, health-related quality of life (HRQoL), cognitive functions, single- and dual-task walking mobility, as well as indoor and outdoor gait in community-dwelling chronic stroke survivors. Global cognitive functioning (MoCA, primary outcome) remained stable in both groups, albeit the rate of responders showing a MCID in this outcome was higher in the exergame compared to the control group. The Mobility domain of the HRQoL questionnaire, reaction time in intrinsic visual alertness, mistakes in the TMT-B and the 2-back test, and gait speed as well as swing width on the unaffected side in outdoor walking showed significant interaction effects favouring the exergame group. However, as the study was not powered to these secondary outcomes, these results should be interpreted prudently. Neither the MoCA nor any of the outcomes showing significant interaction effects exhibited clinically meaningful changes on group level. Participants showed high compliance and adherence to the exergame training, and no definitely related adverse events occurred. The task load in the exergame training was below the targeted range.
We found no interaction effects in the MoCA (Figure 3), however, a higher responder rate in the exergame compared to the control group (Table 2). Visual simple reaction time showed an interaction effect favouring the exergame group (Figure 4B). Additionally, the exergame group exhibited more and larger within-group improvements in cognitive assessments compared to the control group (Table 3). It seems that cognitive improvements were measurable with specific and sensitive tests but did not appear in a less sensitive summary score of global cognition as the MoCA. These findings are partly in line with three RCTs implementing exergame trainings in chronic stroke survivors with comparable baseline characteristics (Rozental-Iluz et al., 2016; Hung et al., 2017; Maier et al., 2020). Two of these studies compared exergame trainings in a standing position to conventional balance / physical training and found significant within-group improvements in the exergame groups but no significant interaction effects (Rozental-Iluz et al., 2016; Hung et al., 2017). The third study implemented seated video-game training including gross arm movements compared to conventional cognitive training, and found neither within-group changes nor interaction effects (Maier et al., 2020). These results align with findings in older adults that exergames should be performed in a standing position to improve cognitive functions (Tahmosybayat et al., 2018; Manser and de Bruin, 2021; Hou and Li, 2022; Manser et al., 2024). Moreover, they also align with evidence that supports exergames as adjunct to usual care or substitute to physical trainings but does not suggest them to outperform conventional cognitive trainings for improving specific cognitive functions (Stanmore et al., 2017; Mura et al., 2018).
Recent systematic reviews present mixed evidence regarding the effect of exergames on global and specific cognitive functions. Some found no beneficial effects on global cognitive functions of VR interventions including exergames in chronic stroke (Gao et al., 2021), and of exergames in neurological populations and healthy older adults (Mura et al., 2018; Chen et al., 2023; Li et al., 2023). Others, investigating the effects of exergames compared to active or passive control groups in acute stroke and older adults with or without cognitive impairments, did find significant beneficial effects on MoCA scores (Soares et al., 2021; Buyle et al., 2022; Cai et al., 2023). Attentional functions have been reported to significantly improve with exergames in stroke (Gao et al., 2021) or to not change in neurological populations and healthy older adults (Yen and Chiu, 2021; Li et al., 2023). In stroke, neurological populations, and healthy older adults, executive functions often show beneficial effects after exergaming (Mura et al., 2018; Gao et al., 2021; Yen and Chiu, 2021; Jiang et al., 2022; Cai et al., 2023), however, systematic reviews showing no beneficial effects exist as well (Soares et al., 2021; Huber et al., 2022a; Li et al., 2023). Moreover, working memory and visuospatial functions have rarely been investigated in exergame studies, and effects were mostly non-significant (Unibaso-Markaida et al., 2019; Cai et al., 2023; Li et al., 2023). To conclude, evidence on the effects of exergames on cognitive functions in chronic stroke and related populations is inconsistent to date, therefore, more research is needed. A possible reason may be varying training protocols, on which we elaborate below.
Another explanatory factor may be different populations, for instance in terms of baseline cognitive status. Beneficial effects of exergames in cognitively impaired populations (Swinnen et al., 2021; Manser and de Bruin, 2024) compared to no beneficial effects in healthy older adults (Sturnieks et al., 2024) may support the hypothesis that cognitively impaired people benefit more from exergame training compared to cognitively healthy people. However, in our sample, baseline cognitive impairment did not affect responding to the intervention (Table 2). This is in line with several meta-analyses, wherein the positive effects of exergames on cognitive functions were mediated by studies including cognitively healthy older adults, while the subgroups of participants with mild cognitive impairment showed no beneficial effects (Soares et al., 2021; Jiang et al., 2022). We also analysed whether number of responders differed based on adherence to the exergame training, or relevant covariates. However, neither of the evaluated parameters showed a significant difference between responders and non-responders (Table 2). Therefore, future research should determine which populations benefit from exergame training, and under which circumstances.
We found a significant interaction effect in the health-related quality of life domain Mobility at follow-up (Figure 4A). Mean changes did not exceed the MCID of 4.5 points for this SIS domain [Supplementary Tables S6, S7 (Lin et al., 2010)], nevertheless the rate of responders was higher in the exergame group compared to the control group (Table 2). This indicates that participants attending the exergame training felt more mobile afterwards, while control participants remarked no difference. Responders in the exergame group showed significantly lower perceived mobility at baseline than non-responders (Table 2), which could indicate that less mobile participants benefitted more from the exergame training. Besides the training, a possible explanation for this finding could be that not only the exergame training but also the study participation itself, including leaving the house and coming into the study centres, may have helped less mobile participants to improve their perceived mobility. However, one must keep in mind that a ceiling effect was probably present in this outcome. The non-responders showed a mean Mobility score of 100 (the maximum) already at baseline. Therefore, the reason for becoming a non-responder could also be that there was no room for improvement over the intervention period. Consequently, it remains open to clarify whether participants with impaired perceived mobility profit more from the exergame training compared to participants with high perceived mobility.
We found no interaction effects, but significant within-group improvements in single-task mobility in both groups (Table 3). These results are partly in line with existing literature. Significant within-group improvements in TUG performance after exergaming have been reported in stroke (Unibaso-Markaida and Iraurgi, 2022), and non-significant interaction effects in older adults, MCI and PD patients (Taylor et al., 2018; Elena et al., 2021; Buyle et al., 2022; Cai et al., 2023). However, a larger body of evidence in related populations supports a superior effect of exergames compared to passive and active controls on TUG performance (Fang et al., 2020; Pacheco et al., 2020; Chen et al., 2021; Prosperini et al., 2021; Suleiman-Martos et al., 2021; Zhang et al., 2022; Elhusein et al., 2024; Yoong et al., 2024). Indoor walking did not change in neither group, which is not in line with literature in (chronic) stroke (Corbetta et al., 2015; De Rooij et al., 2016; Gibbons et al., 2016; Ghai et al., 2020; Zhang et al., 2021, 2023; Huber et al., 2022a). Both groups were highly physically active before and throughout the study duration, which may explain their high performance in the gait analyses. For example, our participants showed higher gait speeds (1.24–1.41 m/s) compared to samples of other stroke studies (Yang et al., 2008; Fritz, 2013; Choi et al., 2018; Cikajlo et al., 2020; Lee and Bae, 2022). The high baseline status of the participants may explain the lack of significant interaction effects on single-task motor functions. Another explanation may be a limitation of how the PEMOCS concept was implemented. Namely, many of the more complex and intense motor tasks of the intervention (including squats, double steps, jumps) were added to the games via verbal instruction only. The missing in-game feedback and / or reward on the execution of these complex and intense motor tasks may have induced participants to prioritize the cognitive task (presented and rewarded by the game) over the additional motor task (similarly as suggested in Gallou-Guyot et al., 2023). This led to (partially) incomplete execution of these tasks, which may have diminished possible benefits on motor functions. We present a possible solution for this problem in the Future Directions.
In dual-task mobility, we found no interaction effects, but significant within-group improvements in both groups. The exergame group showed larger improvements in the cognitive dual-task measures (correct response rates), while the control group exhibited larger improvements in the motor dual-task effect (Table 3). In outdoor gait, gait speed and swing width on the unaffected side showed significant interaction effects favouring the exergame group (Figures 4C,D). The responder analysis in gait speed showed an only slightly higher responder rate in the exergame group compared to the control group (Table 2), and changes did not exceed MCIDs for preferred gait speed [Supplementary Tables S8, S9, (Fulk et al., 2011; Bohannon and Glenney, 2014)]. It should be considered that this responder analysis was performed with a MCID for indoor gait speed, as no value for outdoor gait speed is, to our knowledge, available in literature. Moreover, only the exergame group showed significant improvements in several spatiotemporal gait parameters of the OWA (Table 3). Even though significant, the median changes in these parameters were below the smallest detectable changes (SDCs) determined in a study using the same Physilog sensors in stroke patients (Lefeber et al., 2019), which is why these results warrant cautious interpretation. Nevertheless, the summarized findings imply an overall picture. Namely, these partially beneficial effects on dual-task mobility and outdoor walking may be linked as both tasks can be considered complex walking tasks (Grosboillot et al., 2024). Outdoor walking needs, due to distractions of the environment, more cognitive resources compared to indoor walking (Hillel et al., 2019). The cognitive Timed-up-and-go is even more complex with the additional cognitive task (Grosboillot et al., 2024). Considering that we found significant interaction effects favouring the exergame over the control group in some cognitive measures but not in single motor-tasks, one could hypothesize that the improvements in dual-task mobility and outdoor gait are linked to increased cognitive resources triggered by the exergame intervention. This makes sense when observing that the improvements in dual-task performance in the exergame group were seen in the cognitive dual-task measures, while in the control group they were found in the motor dual-task measures (Table 3). Therefore, exergames may be beneficial for improving activities that require cognitive contribution in chronic stroke survivors.
The general training volume of 14 h of this study’s intervention may have been too low to trigger more interaction effects (Saeedi et al., 2021). At least 15 h of training has been recommended for improving cognitive and motor functions in stroke and older adults (Kwakkel et al., 2004; Lauenroth et al., 2016; Laver et al., 2017; Zhang et al., 2021). As duration and session duration of the presented intervention were in line with current recommendations for exergames (Fang et al., 2020; Jiang et al., 2022; Duta et al., 2023; Peng et al., 2024), the frequency may be the most suitable parameter to adjust. This aligns with three systematic reviews showing that frequency was a moderator of exergame-interventions effects (Prosperini et al., 2021; Hai et al., 2022; Jiang et al., 2022). While motor functions profit from high-frequency interventions (Prosperini et al., 2021), a lower frequency (≤ 3x/week) was reported to be more beneficial for improving cognitive functions (Zhu et al., 2016; Gallou-Guyot et al., 2020; Jiang et al., 2022). Three sessions per week may thus be most beneficial for improving both, cognitive and motor functions (Fang et al., 2020; Gallou-Guyot et al., 2020; Prosperini et al., 2021; Hai et al., 2022). Community-dwelling stroke survivors reported in our feasibility study that more than two centre-based sessions were not feasible for them (Huber et al., 2021) (which was the reason for not implementing a higher frequency in this study). Therefore, a blended-therapy approach may be a possible solution to increase the number of sessions per week (Mehra et al., 2018) (see Section 4.8).
A second influential training principle may have been insufficient exercise intensity. It has been postulated that cognitive and motor benefits of exergames (and other motor-cognitive and physical trainings) may be induced by neurotrophin-mediated neuroplasticity (Monteiro-Junior et al., 2016; Levin et al., 2017; Stojan and Voelcker-Rehage, 2019). Special attention has been given to brain-derived neurotrophic factor (BDNF), insulin growth factor-1 (IGF1), and vascular endothelial growth factor (VEGF), which were suggested to collectively mediate exergame-induced neuroplasticity (Yang et al., 2023). Supportive of this hypothesis are several studies, which found that exergaming increased BDNF levels in chronic stroke, older adults and Parkinson’s disease patients (Monteblanco Cavalcante et al., 2021; Huang et al., 2022; Schaeffer et al., 2022), as well as IGF-1 and other neuroplasticity-indicative biomarkers in people with mild cognitive impairment (Nath et al., 2023). Wide results from animal and human studies report that at least moderate exercise intensity is necessary for BDNF increase (Ploughman et al., 2015; Morais et al., 2018; Li et al., 2024). In accordance, low intensity exercise did not induce neuroplasticity in chronic stroke survivors (Murdoch et al., 2016), while exercise at moderate intensity increased neuroplasticity-associated factors and triggered neuroplasticity in stroke (Boyne et al., 2020; Hill et al., 2023). Moreover, moderate-intensity exercise has also been recommended for improving cognitive and physical functioning in stroke and older adults (Dhir et al., 2021; Yang and Wang, 2021; Li et al., 2022; Maeneja et al., 2024). Exergames have been shown to equal physical activity of low to moderate intensity, depending on the content of the games (Peng et al., 2011; Mat Rosly et al., 2017). Considering this, we hypothesize that the intensity of the exergame intervention in this study was too low to trigger neuroplasticity, and consequently effects on cognitive and motor functions were little. We did not specifically measure intensity during our intervention; however, participants reported their motor-cognitive task load via perceived task difficulty and performance. These parameters may align with intensity and showed that participants were under-challenged (Figures 6A,B). This was already the case in the feasibility study preceding this RCT (Huber et al., 2021), and we took actions in the further development of the PEMOCS concept to address this limitation (Huber et al., 2024b). It seems that the adaptions undertaken [namely, coupling motor and cognitive progression, and inclusion of more difficult motor tasks (Huber et al., 2024b)] did not increase intensity and task load sufficiently. One reason for this may be that these adaptions rather increased complexity of the tasks, instead of raising intensity (compare Section 4.3). Recent findings suggest that physical activity decreases with increased cognitive complexity in step-based exergames (Muller et al., 2023). We present a possible solution in Section 4.8. Two further reasons for insufficient intensity may be related to limitations of the used exergaming device (compare Section 4.7, limitations 1 + 2).
Compliance and adherence to the exergame intervention was high, which is in line with previous studies (Pacheco et al., 2020; Rüth et al., 2023; Tsurayya et al., 2023). The attrition rate was at the upper edge or higher compared to reported ranges (Cheok et al., 2015; Taylor et al., 2018; Yoong et al., 2024), which may be attributed to different reasons in the two treatments groups. In the exergame group, several causally unrelated adverse events occurred, which hindered participants from further study participation. In the control group, some participants reported boredom or even discontent with the study, as they received no intervention and still had to be available for the phone calls and answer the same questions every week. Hence, withdrawals were unrelated to the exergame intervention, however, control groups should be planned differently in future studies. Finally, no causally related adverse events and few technical problems occurred, which is in line with previous exergame studies (Cheok et al., 2015; Pacheco et al., 2020; Prosperini et al., 2021; Rüth et al., 2023; Yoong et al., 2024).
Strengths of the presented study are the following. (1) We implemented a concept-guided exergame intervention with a personalized progression and evidence-based rationales for all exercise variables (Huber et al., 2024b). (2) We used a customized and step-based exergame device with cognitively challenging games to be played in a standing position (Deutsch et al., 2019; Gallou-Guyot et al., 2020; Manser et al., 2024). (3) We assessed cognitive functions, dual-task ability, indoor and outdoor spatiotemporal gait parameters, which are outcomes that are yet under-investigated in exergame studies (Isha, 2019; Gallou-Guyot et al., 2020; Huber et al., 2022a). (4) The assessors in this study completed MoCA training certification before conducting assessments, which ensures high quality and validity of the assessment performance (mocacognition.com). However, there are also several limitations, which should be considered. (1) Several motor-cognitive skill categories of the PEMOCS concept’s underlying taxonomy could not be filled sufficiently due to limitations of the exergame device used. Especially the skill-categories in the higher difficulty levels [see Section 2.4 and (Huber et al., 2024b)] contained only few or even no games, which led to limitations for participants moving in these higher levels. Most importantly, not all cognitive domains could be targeted, and insufficient variability was provided in training sessions for these participants. This may be especially relevant for the presented study population as many participants presented mild residual impairments and may probably have profited from more difficult games and higher variability in the higher levels. (2) Due to a lack of resources for additional developmental work, many of the more complex and intense motor tasks of the intervention were only instructed verbally instead of being integrated into the game, so in-game feedback on their execution was missing. This is unfortunate in the light of literature describing that one advantage of exergames is multisensory feedback and higher enjoyment, which motivates the trainee for more exercise repetitions at a higher intensity (Sailer et al., 2017; Valenzuela et al., 2018). We had started the integration, e.g., a battery visually rewarded participants for dribbling in the according games [(Huber et al., 2024b), Supplementary material S2], however, for future implementations of the PEMOCS concept, in-game feedback for all tasks should be provided. (3) Women were clearly underrepresented in our study, which limits the generalizability of our results to the general chronic stroke population. This is a known phenomenon in studies implementing exercise interventions in stroke (Li et al., 2022), also represented in comparable examples (Hung et al., 2017; Cikajlo et al., 2020; Lee and Bae, 2022; Sultan et al., 2023). A possible reason may be that women are on average older than men are, when they experience a stroke (Eriksson et al., 2021). Consequently, they are more severely impaired and less able to participate in exercise (Eriksson et al., 2021). (4) To calculate the gait variability index (GVI), values for step length and step time had to be estimated from other parameters, as they were not provided by the Gait Up system. For exact procedures, see Supplementary material S1.
Appropriate schedules and settings for exergame training to improve cognitive functions, health-related quality of life, indoor and outdoor gait in chronic stroke should be further investigated in future high-quality trials. For such trials as well as an implementation in clinical practice, cost and time efforts of the intervention should be considered. A possible solution to increase the number of sessions per week would be to implement a blended therapy approach, meaning that participants attend one or two supervised sessions per week in a study centre and perform further sessions at home with a telerehabilitation system (Baschung-Pfister et al., 2021; Seinsche et al., 2022). This would combine close contact and supervision by the study / health professionals with reduced time expense and travel-time to the study centres while enabling a higher training frequency (Mehra et al., 2018). Such an intervention design may be more attractive for potential participants with limited time resources or ability to reach the study centres. It may thus help increase the recruitment rate of more severely impaired study participants. To increase the intensity of the exergame training and reduce the prioritization problem (compare Section 4.3), we suggest increasing game speed before progressing game complexity (Muller et al., 2023). Participants would then execute simpler tasks at a higher speed, which may increase the cardiovascular load and cognitive difficulty of the training. Additionally, in-game feedback / reward on all tasks should be included to increase the motivation for proper task execution (compare Section 4.7, limitation 2). This would warrant adjusting the progression rules in the application of the PEMOCS concept (Huber et al., 2024b). Additionally, an objective performance parameter should be included (Huber et al., 2024b), which would enable automated progression and variability independent of a training supervisor. This would contribute to the self-reliant home-based sessions as well as help reducing the time efforts of the personal providing the intervention and therefore enable more patients to receive the intervention. Finally, to contribute to the on-going debate on the role of disease duration in chronic stroke survivors, a future study could investigate the effects of exergame training on cognitive functions in patients 6–24 months post-stroke (Saa et al., 2021). In such a sample earlier post-stroke, the cognitive benefits of exergame training may be greater (Saa et al., 2021).
Concept-guided, personalized, motor-cognitive exergame training preserved global cognitive functioning in chronic stroke, while it showed no additional benefits compared to usual care only. The rate of responders was higher in the exergame group compared to the control group. Secondarily, significant beneficial effects were found on alertness, working memory, perceived mobility, as well as outdoor gait speed and swing width on the unaffected side. No potential characteristic of responders could be identified as responders and non-responders did not differ in terms of adherence to intervention, baseline value of the according outcome, or age. Therefore, the identification of responsive sub-populations remains subject to future research. Participants showed high adherence to the training and no related adverse events occurred. Reasons for the lack of significant findings may be a high-functioning study population in combination with an insufficient training load in terms of session frequency and intensity. Future studies may increase number of sessions per week by implementing a blended therapy approach, and intensity by adjusting training progression in the PEMOCS concept.
The raw data supporting the conclusions of this article will be made available by the authors, without undue reservation.
The studies involving humans were approved by Kantonale Ethikkommission Zürich. The studies were conducted in accordance with the local legislation and institutional requirements. The participants provided their written informed consent to participate in this study.
SH: Conceptualization, Data curation, Formal analysis, Funding acquisition, Investigation, Methodology, Project administration, Validation, Visualization, Writing – original draft. RK: Conceptualization, Funding acquisition, Project administration, Writing – review & editing. JH: Conceptualization, Methodology, Project administration, Writing – review & editing. MB: Conceptualization, Methodology, Project administration, Writing – review & editing. SG: Investigation, Methodology, Project administration, Visualization, Writing – review & editing. NN: Investigation, Methodology, Project administration, Writing – review & editing. EB: Conceptualization, Funding acquisition, Methodology, Supervision, Writing – review & editing.
The author(s) declare that financial support was received for the research and/or publication of this article. This study was supported by grants of the USZ Innovation Pool (University Hospital Zurich, Switzerland, https://usz-foundation.com/en/usz-innovation-pool/) and the Swiss Association of Physiotherapy (physioswiss). Open access funding by ETH Zurich.
We would like to thank all participants for their time and patience in the study procedures. Special thanks go to Prof. Susanne Wegener MD, Prof. Michael Weller MD, and Anina Enderli MD for their support in recruitment. We want thank Vivianne Blöchlinger MSc. ETH, Jeannine Breindl MSc. ETH, Martina Hofmann MSc. ETH, Markus Kuhn MSc. ETH, Sumana Leuenberger MSc. ETH, Asmy Srisathiyakumaran MSc. ETH, and Tamara Sutter MSc. ETH for their work as investigators. More thanks go to Emanuel Brunner PhD, Fabiana Renna BSc. PT, and the therapy team of Ambulate Reha Triemli Zürich for their support at the study sites. We also want to thank Dividat AG for their support regarding content, soft- and hardware of the exergame training; special thanks go to Manuela Adcock Dr.Sc. ETH, Johannes Emerich, and Marco Höfliger. Further thanks go to Jakob Heimer MD for statistical consulting. Finally, we would like to thank Christoph Bauer PhD being the study monitor and Nanda Telgenkamp (CTC-Zurich) for the educational study visit.
The authors declare that the research was conducted in the absence of any commercial or financial relationships that could be construed as a potential conflict of interest. Dividat AG was not involved in any study-related decisions including but not limited to study design, collection, management, analysis, and interpretation of the data, as well as writing this study report.
The author(s) declared that they were an editorial board member of Frontiers, at the time of submission. This had no impact on the peer review process and the final decision.
The authors declare that no Gen AI was used in the creation of this manuscript.
All claims expressed in this article are solely those of the authors and do not necessarily represent those of their affiliated organizations, or those of the publisher, the editors and the reviewers. Any product that may be evaluated in this article, or claim that may be made by its manufacturer, is not guaranteed or endorsed by the publisher.
The Supplementary material for this article can be found online at: https://www.frontiersin.org/articles/10.3389/fnagi.2025.1514594/full#supplementary-material
Anker, R. (2019). PhysiGait lab: user manual and outcome parameters. Available online at: https://clinical.gaitup.com/wp-content/uploads/2019/10/PhysiGaitLab_User_Manual_v131.pdf (Accessed April 09, 2022).
Bamidis, P. D., Fissler, P., Papageorgiou, S. G., Zilidou, V., Konstantinidis, E. I., Billis, A. S., et al. (2015). Gains in cognition through combined cognitive and physical training: the role of training dosage and severity of neurocognitive disorder. Front. Aging Neurosci. 7:152. doi: 10.3389/fnagi.2015.00152
Barker, W. H., and Mullooly, J. P. (1997). Stroke in a defined elderly population, 1967-1985. A less lethal and disabling but no less common disease. Stroke 28, 284–290. doi: 10.1161/01.str.28.2.284
Basak, C., Qin, S., and O'Connell, M. A. (2020). Differential effects of cognitive training modules in healthy aging and mild cognitive impairment: a comprehensive meta-analysis of randomized controlled trials. Psychol. Aging 35, 220–249. doi: 10.1037/pag0000442
Baschung-Pfister, P., Knols, R. H., de Bie, R. A., and de Bruin, E. D. (2021). Feasibility of a blended therapy approach in the treatment of patients with inflammatory myopathies. Arch. Physiother. 11:14. doi: 10.1186/s40945-021-00108-z
Beauchet, O., Sekhon, H., Barden, J., Liu-Ambrose, T., Chester, V. L., Szturm, T., et al. (2018). Association of Motoric Cognitive Risk Syndrome with cardiovascular disease and risk factors: results from an original study and Meta-analysis. J. Alzheimers Dis. 64, 875–887. doi: 10.3233/jad-180203
Bijleveld-Uitman, M., van de Port, I., and Kwakkel, G. (2013). Is gait speed or walking distance a better predictor for community walking after stroke? J. Rehabil. Med. 45, 535–540. doi: 10.2340/16501977-1147
Blum, L., and Korner-Bitensky, N. (2008). Usefulness of the Berg balance scale in stroke rehabilitation: a systematic review. Phys. Ther. 88, 559–566. doi: 10.2522/ptj.20070205
Bogen, B., Moe-Nilssen, R., Ranhoff, A. H., and Aaslund, M. K. (2018). The walk ratio: investigation of invariance across walking conditions and gender in community-dwelling older people. Gait Posture 61, 479–482. doi: 10.1016/j.gaitpost.2018.02.019
Bohannon, R. W., and Glenney, S. S. (2014). Minimal clinically important difference for change in comfortable gait speed of adults with pathology: a systematic review. J. Eval. Clin. Pract. 20, 295–300. doi: 10.1111/jep.12158
Bowie, C. R., and Harvey, P. D. (2006). Administration and interpretation of the trail making test. Nat. Protoc. 1, 2277–2281. doi: 10.1038/nprot.2006.390
Boyne, P., Meyrose, C., Westover, J., Whitesel, D., Hatter, K., Reisman, D. S., et al. (2020). Effects of exercise intensity on acute circulating molecular responses poststroke. Neurorehabil. Neural Repair 34, 222–234. doi: 10.1177/1545968319899915
Burke, E., Dobkin, B. H., Noser, E. A., Enney, L. A., and Cramer, S. C. (2014). Predictors and biomarkers of treatment gains in a clinical stroke trial targeting the lower extremity. Stroke 45, 2379–2384. doi: 10.1161/STROKEAHA.114.005436
Buyle, M., Jung, Y., Pavlou, M., Gonzalez, S. C., and Bamiou, D. E. (2022). The role of motivation factors in exergame interventions for fall prevention in older adults: a systematic review and meta-analysis. Front. Neurol. 13:903673. doi: 10.3389/fneur.2022.903673
Cai, Z., Ma, Y., Li, L., and Lu, G. Z. (2023). Effects of exergaming in older individuals with mild cognitive impairment and dementia: a systematic review and meta-analysis. Geriatr. Nurs. 51, 351–359. doi: 10.1016/j.gerinurse.2023.03.028
Calafiore, D., Invernizzi, M., Ammendolia, A., Marotta, N., Fortunato, F., Paolucci, T., et al. (2021). Efficacy of virtual reality and exergaming in improving balance in patients with multiple sclerosis: a systematic review and meta-analysis. Front. Neurol. 12:773459. doi: 10.3389/fneur.2021.773459
Cano Porras, D., Siemonsma, P., Inzelberg, R., Zeilig, G., and Plotnik, M. (2018). Advantages of virtual reality in the rehabilitation of balance and gait: systematic review. Neurology 90, 1017–1025. doi: 10.1212/wnl.0000000000005603
Chan, K. G. F., Jiang, Y., Choo, W. T., Ramachandran, H. J., Lin, Y., and Wang, W. (2022). Effects of exergaming on functional outcomes in people with chronic stroke: a systematic review and meta-analysis. J. Adv. Nurs. 78, 929–946. doi: 10.1111/jan.15125
Charlson, M. E., Carrozzino, D., Guidi, J., and Patierno, C. (2022). Charlson comorbidity index: a critical review of clinimetric properties. Psychother. Psychosom. 91, 8–35. doi: 10.1159/000521288
Chen, P. J., Hsu, H. F., Chen, K. M., and Belcastro, F. (2023). VR exergame interventions among older adults living in long-term care facilities: a systematic review with Meta-analysis. Ann. Phys. Rehabil. Med. 66:101702. doi: 10.1016/j.rehab.2022.101702
Chen, Y., Zhang, Y., Guo, Z., Bao, D., and Zhou, J. (2021). Comparison between the effects of exergame intervention and traditional physical training on improving balance and fall prevention in healthy older adults: a systematic review and meta-analysis. J. Neuroeng. Rehabil. 18:164. doi: 10.1186/s12984-021-00917-0
Cheng, D. K., Nelson, M., Brooks, D., and Salbach, N. M. (2020). Validation of stroke-specific protocols for the 10-meter walk test and 6-minute walk test conducted using 15-meter and 30-meter walkways. Top. Stroke Rehabil. 27, 251–261. doi: 10.1080/10749357.2019.1691815
Cheok, G., Tan, D., Low, A., and Hewitt, J. (2015). Is Nintendo Wii an effective intervention for individuals with stroke? A systematic review and Meta-analysis. J. Am. Med. Dir. Assoc. 16, 923–932. doi: 10.1016/j.jamda.2015.06.010
Chiti, G., and Pantoni, L. (2014). Use of Montreal cognitive assessment in patients with stroke. Stroke 45, 3135–3140. doi: 10.1161/STROKEAHA.114.004590
Choi, D., Choi, W., and Lee, S. (2018). Influence of Nintendo Wii fit balance game on visual perception, postural balance, and walking in stroke survivors: a pilot randomized clinical trial. Games Health J. 7, 377–384. doi: 10.1089/g4h.2017.0126
Cikajlo, I., Rudolf, M., Mainetti, R., and Borghese, N. A. (2020). Multi-exergames to set targets and supplement the intensified conventional balance training in patients with stroke: a randomized pilot trial. Front. Psychol. 11:572. doi: 10.3389/fpsyg.2020.00572
Corbetta, D., Imeri, F., and Gatti, R. (2015). Rehabilitation that incorporates virtual reality is more effective than standard rehabilitation for improving walking speed, balance and mobility after stroke: a systematic review. J. Physiother. 61, 117–124. doi: 10.1016/j.jphys.2015.05.017
Cumming, T. B., Marshall, R. S., and Lazar, R. M. (2013). Stroke, cognitive deficits, and rehabilitation: still an incomplete picture. Int. J. Stroke 8, 38–45. doi: 10.1111/j.1747-4949.2012.00972.x
De Rooij, I. J. M., Van de Port, I. G. L., and Meijer, J.-W. G. (2016). Effect of virtual reality training on balance and gait ability in patients with stroke: systematic review and meta-analysis. Phys. Ther. 96, 1905–1918. doi: 10.2522/ptj.20160054
Deutsch, J. E., Hoehlein, B., Priolo, M., Pacifico, J., Damodaran, H., and Puh, U. (2019). "Custom game paced video games played by persons post-stroke have comparable exercise intensity but higher accuracy, greater enjoyment and less effort than off-the-shelf game", in: International conference on virtual rehabilitation, ICVR).
Dhir, S., Teo, W. P., Chamberlain, S. R., Tyler, K., Yucel, M., and Segrave, R. A. (2021). The effects of combined physical and cognitive training on inhibitory control: a systematic review and Meta-analysis. Neurosci. Biobehav. Rev. 128, 735–748. doi: 10.1016/j.neubiorev.2021.07.008
Dong, L., Briceno, E., Morgenstern, L. B., and Lisabeth, L. D. (2020). Poststroke cognitive outcomes: sex differences and contributing factors. J. Am. Heart Assoc. 9:e016683. doi: 10.1161/jaha.120.016683
Duncan, P. W., Wallace, D., Lai, S. M., Johnson, D., Embretson, S., and Laster, L. J. (1999). The stroke impact scale version 2.0: evaluation of reliability, validity, and sensitivity to change. Stroke 30, 2131–2140. doi: 10.1161/01.STR.30.10.2131
Duta, T. F., Tsurayya, G., and Naufal, M. A. (2023). Exergame for post-stroke rehabilitation among elderly patients: a systematic review and meta-analysis. Narra X 1. doi: 10.52225/narrax.v1i1.73
Elena, P., Demetris, S., Christina, M., and Marios, P. (2021). Differences between exergaming rehabilitation and conventional physiotherapy on quality of life in Parkinson's disease: a systematic review and meta-analysis. Front. Neurol. 12:683385. doi: 10.3389/fneur.2021.683385
Elhusein, A. M., Fadlalmola, H. A., Awadalkareem, E. M., Alhusain, E. Y. M., Alnassry, S. M., Alshammari, M., et al. (2024). Exercise-based gaming in patients with multiple sclerosis: a systematic review and meta-analysis. Belitung Nurs J 10, 1–14. doi: 10.33546/bnj.3006
Eriksson, M., Åsberg, S., Sunnerhagen, K. S., and von Euler, M. (2021). Sex differences in stroke care and outcome 2005-2018: observations from the Swedish stroke register. Stroke 52, 3233–3242. doi: 10.1161/strokeaha.120.033893
Falck, R. S., Davis, J. C., Best, J. R., Crockett, R. A., and Liu-Ambrose, T. (2019). Impact of exercise training on physical and cognitive function among older adults: a systematic review and meta-analysis. Neurobiol. Aging 79, 119–130. doi: 10.1016/j.neurobiolaging.2019.03.007
Fang, Q., Ghanouni, P., Anderson, S. E., Touchett, H., Shirley, R., Fang, F., et al. (2020). Effects of exergaming on balance of healthy older adults: a systematic review and Meta-analysis of randomized controlled trials. Games Health J. 9, 11–23. doi: 10.1089/g4h.2019.0016
Faria, C. A., Alves, H. V. D., and Charchat-Fichman, H. (2015). The most frequently used tests for assessing executive functions in aging. Dement. Neuropsychol. 9, 149–155. doi: 10.1590/1980-57642015DN92000009
Feise, R. J. (2002). Do multiple outcome measures require p-value adjustment? BMC Med. Res. Methodol., 2, 1–4. doi: 10.1186/1471-2288-2-8
Feng, C., Wang, H., Lu, N., Chen, T., He, H., Lu, Y., et al. (2014). Log-transformation and its implications for data analysis. Shanghai Arch. Psychiatry 26, 105–109. doi: 10.3969/j.issn.1002-0829.2014.02.009
Field, A., Miles, J., and Field, Z. (2012). Discovering statistics using R. London: SAGE Publications Ltd.
Fissler, P., Küster, O., Schlee, W., and Kolassa, I. (2013). Novelty interventions to enhance broad cognitive abilities and prevent dementia: synergistic approaches for the facilitation of plastic change. Prog. Brain Res 207, 403–434. doi: 10.1016/B978-0-444-63327-9.00017-5
Fritz, N. E. (2013). Contribution of motor and cognitive factors to gait variability and fall risk: From clinical assessment to neural connectivity. PhD Dissertation, The Ohio State University.
Fugl-Meyer, A. R., Jaasko, L., Leyman, I., Olsson, S., and Steglind, S. (1975). The post-stroke hemiplegic patient. 1. A method for evaluation of physical performance. Scand. J. Rehabil. Med. 7, 13–31. doi: 10.2340/1650197771331
Fulk, G. D., Ludwig, M., Dunning, K., Golden, S., Boyne, P., and West, T. (2011). Estimating clinically important change in gait speed in people with stroke undergoing outpatient rehabilitation. J. Neurol. Phys. Ther. 35, 82–89. doi: 10.1097/NPT.0b013e318218e2f2
Gabrio, A., Plumpton, C., Banerjee, S., and Leurent, B. (2022). Linear mixed models to handle missing at random data in trial-based economic evaluations. Health Econ. 31, 1276–1287. doi: 10.1002/hec.4510
Gajewski, P. D., Hanisch, E., Falkenstein, M., Thönes, S., and Wascher, E. (2018). What does the n-Back task measure as we get older? Relations between working-memory measures and other cognitive functions across the lifespan. Front. Psychol. 9. doi: 10.3389/fpsyg.2018.02208
Gallou-Guyot, M., Mandigout, S., Bherer, L., and Perrochon, A. (2020). Effects of exergames and cognitive-motor dual-task training on cognitive, physical and dual-task functions in cognitively healthy older adults: an overview. Ageing Res. Rev. 63:101135. doi: 10.1016/j.arr.2020.101135
Gallou-Guyot, M., Mandigout, S., Marie, R., Robin, L., Daviet, J. C., and Perrochon, A. (2023). Feasibility and potential cognitive impact of a cognitive-motor dual-task training program using a custom exergame in older adults: a pilot study. Front. Aging Neurosci. 15:1046676. doi: 10.3389/fnagi.2023.1046676
Gama, G. L., de Lucena, L. C., Brasileiro, A., Silva, E., Galvao, E., Maciel, A. C., et al. (2017). Post-stroke hemiparesis: does chronicity, etiology, and lesion side are associated with gait pattern? Top. Stroke Rehabil. 24, 388–393. doi: 10.1080/10749357.2017.1304865
Gao, Y., Ma, L., Lin, C., Zhu, S., Yao, L., Fan, H., et al. (2021). Effects of virtual reality-based intervention on cognition, motor function, mood, and activities of daily living in patients with chronic stroke: a systematic review and meta-analysis of randomized controlled trials. Front. Aging Neurosci. 13. doi: 10.3389/fnagi.2021.766525
Gavelin, H. M., Dong, C., Minkov, R., Bahar-Fuchs, A., Ellis, K. A., Lautenschlager, N. T., et al. (2020). Combined physical and cognitive training for older adults with and without cognitive impairment: a systematic review and network meta-analysis of randomized controlled trials. Ageing Res. Rev. 66:101232. doi: 10.1016/j.arr.2020.101232
Gentile, A. M. (1987). "Skill acquisition: action, movement, and neuromotor processes.," in Movement science: Foundations for Physical therapy in rehabilitation, ed. J. H. S. Carr, Gordon, J., Gentile, A. M., and Hind, J. M. (Michigan: Aspen Publishers, Inc), 93–154.
Ghai, S., Ghai, I., and Lamontagne, A. (2020). Virtual reality training enhances gait poststroke: a systematic review and meta-analysis. Ann. N. Y. Acad. Sci. 1478, 18–42. doi: 10.1111/nyas.14420
Ghazavi Dozin, S. M., Mohammad Rahimi, N., and Aminzadeh, R. (2024). WII fit-based biofeedback rehabilitation among post-stroke patients: a systematic review and meta-analysis of randomized controlled trial. Biol. Res. Nurs. 26, 5–20. doi: 10.1177/10998004231180316
Gibbons, E. M., Thomson, A. N., de Noronha, M., and Joseph, S. (2016). Are virtual reality technologies effective in improving lower limb outcomes for patients following stroke - a systematic review with meta-analysis. Top. Stroke Rehabil. 23, 440–457. doi: 10.1080/10749357.2016.1183349
Gouelle, A., Megrot, F., Presedo, A., Husson, I., Yelnik, A., and Pennecot, G. F. (2013). The gait variability index: a new way to quantify fluctuation magnitude of spatiotemporal parameters during gait. Gait Posture 38, 461–465. doi: 10.1016/j.gaitpost.2013.01.013
Grosboillot, N., Gallou-Guyot, M., Lamontagne, A., Bonnyaud, C., Perrot, A., Allali, G., et al. (2024). Towards a comprehensive framework for complex walking tasks: characterization, behavioral adaptations, and clinical implications in ageing and neurological populations. Ageing Res. Rev. 101:102458. doi: 10.1016/j.arr.2024.102458
Hai, L., Hou, H. Y., Zhou, C., and Li, H. J. (2022). The effect of exergame training on physical functioning of healthy older adults: a meta-analysis. Games Health J. 11, 207–224. doi: 10.1089/g4h.2021.0173
Harris, P. A., Taylor, R., Minor, B. L., Elliott, V., Fernandez, M., O'Neal, L., et al. (2019). The REDCap consortium: building an international community of software platform partners. J. Biomed. Inform. 95:103208. doi: 10.1016/j.jbi.2019.103208
Harris, P. A., Taylor, R., Thielke, R., Payne, J., Gonzalez, N., and Conde, J. G. (2009). Research electronic data capture (REDCap)—a metadata-driven methodology and workflow process for providing translational research informatics support. J. Biomed. Inform. 42, 377–381. doi: 10.1016/j.jbi.2008.08.010
Harting, F. (2022). DHARMa: residual diagnostics for hierarchical (multi-level / mixed) regression models. R package version 0.4.6. Available online at: http://florianhartig.github.io/DHARMa/ (Accessed November 05, 2024).
Herold, F., Hamacher, D., Schega, L., and Muller, N. G. (2018). Thinking while moving or moving while thinking - concepts of motor-cognitive training for cognitive performance enhancement. Front. Aging Neurosci. 10:228. doi: 10.3389/fnagi.2018.00228
Hill, G., Johnson, F., Uy, J., Serrada, I., Benyamin, B., Van Den Berg, M., et al. (2023). Moderate intensity aerobic exercise may enhance neuroplasticity of the contralesional hemisphere after stroke: a randomised controlled study. Sci. Rep. 13:14440. doi: 10.1038/s41598-023-40902-2
Hillel, I., Gazit, E., Nieuwboer, A., Avanzino, L., Rochester, L., Cereatti, A., et al. (2019). Is every-day walking in older adults more analogous to dual-task walking or to usual walking? Elucidating the gaps between gait performance in the lab and during 24/7 monitoring. Eur. Rev. Aging Phys. Act. 16:6. doi: 10.1186/s11556-019-0214-5
Hotter, B., Padberg, I., Liebenau, A., Knispel, P., Heel, S., Steube, D., et al. (2018). Identifying unmet needs in long-term stroke care using in-depth assessment and the post-stroke checklist – the managing aftercare for stroke (MAS-I) study. Eur. Stroke J. 3, 237–245. doi: 10.1177/2396987318771174
Hou, H. Y., and Li, H. J. (2022). Effects of exergame and video game training on cognitive and physical function in older adults: a randomized controlled trial. Appl. Ergon. 101:103690. doi: 10.1016/j.apergo.2022.103690
Hua, J., Zhou, Y., Chen, L., Tang, X., Diao, S., and Fang, Q. (2022). How do cardiovascular risk factors correlate with post-stroke cognitive function: directly or indirectly through stroke severity? Front. Neurol. 13:917295. doi: 10.3389/fneur.2022.917295
Huang, C. Y., Chiang, W. C., Yeh, Y. C., Fan, S. C., Yang, W. H., Kuo, H. C., et al. (2022). Effects of virtual reality-based motor control training on inflammation, oxidative stress, neuroplasticity and upper limb motor function in patients with chronic stroke: a randomized controlled trial. BMC Neurol. 22:21. doi: 10.1186/s12883-021-02547-4
Huber, S. K., Held, J. P. O., de Bruin, E. D., and Knols, R. H. (2021). Personalized motor-cognitive exergame training in chronic stroke patients-a feasibility study. Front. Aging Neurosci. 13:730801. doi: 10.3389/fnagi.2021.730801
Huber, S. K., Knols, R. H., Arnet, P., and de Bruin, E. D. (2022a). Motor-cognitive intervention concepts can improve gait in chronic stroke, but their effect on cognitive functions is unclear: a systematic review with meta-analyses. Neurosci. Biobehav. Rev. 132, 818–837. doi: 10.1016/j.neubiorev.2021.11.013
Huber, S. K., Knols, R. H., Held, J. P. O., Betschart, M., and de Bruin, E. D. (2024a). PEMOCS: evaluating the effects of a concept-guided, PErsonalised, MOtor-cognitive exergame training on cognitive functions and gait in chronic stroke—study protocol for a randomised controlled trial. Trials 25:451. doi: 10.1186/s13063-024-08283-7
Huber, S. K., Knols, R. H., Held, J. P. O., Christen, T., and de Bruin, E. D. (2022b). Agreement, reliability, and concurrent validity of an outdoor, wearable-based walk ratio assessment in healthy adults and chronic stroke survivors. Front. Physiol. 13:857963. doi: 10.3389/fphys.2022.857963
Huber, S. K., Manser, P., and de Bruin, E. D. (2024b). PEMOCS: theory derivation of a concept for PErsonalized MOtor-cognitive exergame training in chronic stroke—a methodological paper with an application example. Front. Sports Active Living 6. doi: 10.3389/fspor.2024.1397949
Hung, J. W., Chou, C. X., Chang, H. F., Wu, W. C., Hsieh, Y. W., Chen, P. C., et al. (2017). Cognitive effects of weight-shifting controlled exergames in patients with chronic stroke: a pilot randomized comparison trial. Eur. J. Phys. Rehabil. Med. 53, 694–702. doi: 10.23736/S1973-9087.17.04516-6
Isha, S. (2019). Impact of cognitive impairments on functional ambulation in stroke patients. Int. J. Phys. Med. Rehabil 7:528. Available online at: https://www.researchgate.net/profile/Isha-Akulwar-Tajane/publication/349214124_Impact_of_Cognitive_Impairments_on_Functional_Ambulation_in_Stroke_Patients/links/6025380f92851c4ed566569b/Impact-of-Cognitive-Impairments-on-Functional-Ambulation-in-Stroke-Patients.pdf (Accessed June 06, 2024).
Janhunen, M., Karner, V., Katajapuu, N., Niiranen, O., Immonen, J., Karvanen, J., et al. (2021). Effectiveness of exergame intervention on walking in older adults: a systematic review and meta-analysis of randomized controlled trials. Phys. Ther. 101. doi: 10.1093/ptj/pzab152
Jiang, J., Guo, W., and Wang, B. (2022). Effects of exergaming on executive function of older adults: a systematic review and meta-analysis. PeerJ 10:e13194. doi: 10.7717/peerj.13194
Johnson, C. O., Nguyen, M., Roth, G. A., Nichols, E., Alam, T., Abate, D., et al. (2019). Global, regional, and national burden of stroke, 1990-2016: a systematic analysis for the global burden of disease study 2016. Lancet Neurol. 18, 439–458. doi: 10.1016/S1474-4422(19)30034-1
Jokinen, H., Melkas, S., Ylikoski, R., Pohjasvaara, T., Kaste, M., Erkinjuntti, T., et al. (2015). Post-stroke cognitive impairment is common even after successful clinical recovery. Eur. J. Neurol. 22, 1288–1294. doi: 10.1111/ene.12743
Katan, M., and Luft, A. (2018). Global burden of stroke. Semin. Neurol. 38, 208–211. doi: 10.1055/s-0038-1649503
Kim, H. Y. (2017). Statistical notes for clinical researchers: chi-squared test and Fisher's exact test. Restor. Dent. Endod. 42, 152–155. doi: 10.5395/rde.2017.42.2.152
Kollen, B., van de Port, I., Lindeman, E., Twisk, J., and Kwakkel, G. (2005). Predicting improvement in gait after stroke: a longitudinal prospective study. Stroke 36, 2676–2680. doi: 10.1161/01.STR.0000190839.29234.50
Kraft, E. (2012). Cognitive function, physical activity, and aging: possible biological links and implications for multimodal interventions. Neuropsychol. Dev. Cogn. B Aging Neuropsychol. Cogn. 19, 248–263. doi: 10.1080/13825585.2011.645010
Kwah, L. K., and Diong, J. (2014). National Institutes of Health stroke scale (NIHSS). J. Physiother. 60:61. doi: 10.1016/j.jphys.2013.12.012
Kwakkel, G., van Peppen, R., Wagenaar, R. C., Wood Dauphinee, S., Richards, C., Ashburn, A., et al. (2004). Effects of augmented exercise therapy time after stroke: a meta-analysis. Stroke 35, 2529–2539. doi: 10.1161/01.STR.0000143153.76460.7d
Kwong, P. W. H., and Ng, S. S. M. (2019). Cutoff score of the lower-extremity motor subscale of Fugl-Meyer assessment in chronic stroke survivors: a cross-sectional study. Arch. Phys. Med. Rehabil. 100, 1782–1787. doi: 10.1016/j.apmr.2019.01.027
Lam, J. M., Globas, C., Cerny, J., Hertler, B., Uludag, K., Forrester, L. W., et al. (2010). Predictors of response to treadmill exercise in stroke survivors. Neurorehabil. Neural Repair 24, 567–574. doi: 10.1177/1545968310364059
Lauenroth, A., Ioannidis, A. E., and Teichmann, B. (2016). Influence of combined physical and cognitive training on cognition: a systematic review. BMC Geriatr. 16:141. doi: 10.1186/s12877-016-0315-1
Laver, K. E., Lange, B., George, S., Deutsch, J. E., Saposnik, G., and Crotty, M. (2017). Virtual reality for stroke rehabilitation. Cochrane Database Syst. Rev. 2018:CD008349. doi: 10.1002/14651858.CD008349.pub4
Lee, D., and Bae, Y. (2022). Interactive videogame improved rehabilitation motivation and walking speed in chronic stroke patients: a dual-center controlled trial. Games Health J 11, 268–274. doi: 10.1089/g4h.2021.0123
Lefeber, N., Degelaen, M., Truyers, C., Safin, I., and Beckwee, D. (2019). Validity and reproducibility of inertial Physilog sensors for spatiotemporal gait analysis in patients with stroke. IEEE Trans. Neural Syst. Rehabil. Eng. 27, 1865–1874. doi: 10.1109/TNSRE.2019.2930751
León-Domínguez, U., Martín-Rodríguez, J. F., and León-Carrión, J. (2015). Executive n-back tasks for the neuropsychological assessment of working memory. Behav. Brain Res. 292, 167–173. doi: 10.1016/j.bbr.2015.06.002
Levin, O., Netz, Y., and Ziv, G. (2017). The beneficial effects of different types of exercise interventions on motor and cognitive functions in older age: a systematic review. Eur. Rev. Aging Phys. Act. 14:20. doi: 10.1186/s11556-017-0189-z
Li, K., Wang, Y., Wu, Z., Yao, X., and Fan, Y. (2023). Effectiveness of active exergames for improving cognitive function in patients with neurological disabilities: a systematic review and meta-analysis. Games Health J 12, 198–210. doi: 10.1089/g4h.2022.0134
Li, K. Z. H., Bherer, L., Mirelman, A., Maidan, I., and Hausdorff, J. M. (2018). Cognitive involvement in balance, gait and dual-tasking in aging: a focused review from a neuroscience of aging perspective. Front. Neurol. 9:913. doi: 10.3389/fneur.2018.00913
Li, X., Geng, D., Wang, S., and Sun, G. (2022). Aerobic exercises and cognitive function in post-stroke patients: a systematic review with meta-analysis. Medicine (Baltimore) 101:e31121. doi: 10.1097/md.0000000000031121
Li, Z., Guo, H., Yuan, Y., and Liu, X. (2024). The effect of moderate and vigorous aerobic exercise training on the cognitive and walking ability among stroke patients during different periods: a systematic review and meta-analysis. PLoS One 19:e0298339. doi: 10.1371/journal.pone.0298339
Lin, K.-C., Fu, T., Wu, C.-Y., Wang, Y.-H., Liu, J.-S., Hsieh, C.-J., et al. (2010). Minimal detectable change and clinically important difference of the stroke impact scale in stroke patients 24, 486–492. doi: 10.1177/1545968309356295
Maeneja, R., Ferreira, I. S., and Abreu, A. M. (2024). Duration and efficiency of combined versus isolated aerobic training interventions in post-stroke cognition: a systematic review. Port. J. Public Health 42, 43–62. doi: 10.1159/000535272
Mahon, S., Parmar, P., Barker-Collo, S., Krishnamurthi, R., Jones, K., Theadom, A., et al. (2017). Determinants, prevalence, and trajectory of long-term post-stroke cognitive impairment: results from a 4-year follow-up of the ARCOS-IV study. Neuroepidemiology 49, 129–134. doi: 10.1159/000484606
Maier, M., Ballester, B. R., Leiva Bañuelos, N., Duarte Oller, E., and Verschure, P. F. M. J. (2020). Adaptive conjunctive cognitive training (ACCT) in virtual reality for chronic stroke patients: a randomized controlled pilot trial. J. Neuroeng. Rehabil. 17:42. doi: 10.1186/s12984-020-0652-3
Maier, M., Ballester, B. R., and Verschure, P. (2019). Principles of neurorehabilitation after stroke based on motor learning and brain plasticity mechanisms. Front. Syst. Neurosci. 13:74. doi: 10.3389/fnsys.2019.00074
Manser, P., and de Bruin, E. D. (2021). Making the Best out of IT: design and development of exergames for older adults with mild neurocognitive disorder – a methodological paper. Front. Aging Neurosci. 13:734012. doi: 10.3389/fnagi.2021.734012
Manser, P., and de Bruin, E. D. (2024). "Brain-IT": Exergame training with biofeedback breathing in neurocognitive disorders. Alzheimers Dement. 20, 4747–4764. doi: 10.1002/alz.13913
Manser, P., Herold, F., and de Bruin, E. D. (2024). Components of effective exergame-based training to improve cognitive functioning in middle-aged to older adults – a systematic review and meta-analysis. Ageing Res. Rev. 99:102385. doi: 10.1016/j.arr.2024.102385
Mat Rosly, M., Mat Rosly, H., Davis Oam, G. M., Husain, R., and Hasnan, N. (2017). Exergaming for individuals with neurological disability: a systematic review. Disabil. Rehabil. 39, 727–735. doi: 10.3109/09638288.2016.1161086
McDonald, M. W., Black, S. E., Copland, D. A., Corbett, D., Dijkhuizen, R. M., Farr, T. D., et al. (2019). Cognition in stroke rehabilitation and recovery research: consensus-based Core recommendations from the second stroke recovery and rehabilitation roundtable. Neurorehabil. Neural Repair 33, 943–950. doi: 10.1177/1545968319886444
Mehra, S., Visser, B., Dadema, T., van den Helder, J., Engelbert, R. H., Weijs, P. J., et al. (2018). Translating behavior change principles into a blended exercise intervention for older adults: design study. JMIR Res. Protoc. 7:e117. doi: 10.2196/resprot.9244
Mehrholz, J., Wagner, K., Rutte, K., Meissner, D., and Pohl, M. (2007). Predictive validity and responsiveness of the functional ambulation category in hemiparetic patients after stroke. Arch. Phys. Med. Rehabil. 88, 1314–1319. doi: 10.1016/j.apmr.2007.06.764
Meteyard, L., and Davies, R. A. (2020). Best practice guidance for linear mixed-effects models in psychological science. J. Mem. Lang. 112:104092. doi: 10.1016/j.jml.2020.104092
Middleton, L. E., Lam, B., Fahmi, H., Black, S. E., McIlroy, W. E., Stuss, D. T., et al. (2014). Frequency of domain-specific cognitive impairment in sub-acute and chronic stroke. NeuroRehabilitation 34, 305–312. doi: 10.3233/NRE-131030
Monteblanco Cavalcante, M., Fraga, I., Dalbosco, B., De Marchi, P., Iraci, L., Baechtold da Silva, M. E., et al. (2021). Exergame training-induced neuroplasticity and cognitive improvement in institutionalized older adults: a preliminary investigation. Physiol. Behav. 241:113589. doi: 10.1016/j.physbeh.2021.113589
Monteiro-Junior, R. S., Vaghetti, C. A., Nascimento, O. J., Laks, J., and Deslandes, A. C. (2016). Exergames: neuroplastic hypothesis about cognitive improvement and biological effects on physical function of institutionalized older persons. Neural Regen. Res. 11, 201–204. doi: 10.4103/1673-5374.177709
Montero-Odasso, M., Speechley, M., Muir-Hunter, S. W., Pieruccini-Faria, F., Sarquis-Adamson, Y., Hachinski, V., et al. (2020). Dual decline in gait speed and cognition is associated with future dementia: evidence for a phenotype. Age Ageing 49, 995–1002. doi: 10.1093/ageing/afaa106
Morais, V. A. C. D., Tourino, M. F. D. S., Almeida, A. C. D. S., Albuquerque, T. B. D., Linhares, R. C., Christo, P. P., et al. (2018). A single session of moderate intensity walking increases brain-derived neurotrophic factor (BDNF) in the chronic post-stroke patients. Top. Stroke Rehabil. 25, 1–5. doi: 10.1080/10749357.2017.1373500
Muller, H., Baumeister, J., Bardal, E. M., Vereijken, B., and Skjaeret-Maroni, N. (2023). Exergaming in older adults: the effects of game characteristics on brain activity and physical activity. Front. Aging Neurosci. 15:1143859. doi: 10.3389/fnagi.2023.1143859
Mura, G., Carta, M. G., Sancassiani, F., Machado, S., and Prosperini, L. (2018). Active exergames to improve cognitive functioning in neurological disabilities: a systematic review and meta-analysis. Eur. J. Phys. Rehabil. Med. 54, 450–462. doi: 10.23736/S1973-9087.17.04680-9
Murdoch, K., Buckley, J. D., and McDonnell, M. N. (2016). The effect of aerobic exercise on neuroplasticity within the motor cortex following stroke. PLoS One 11:e0152377. doi: 10.1371/journal.pone.0152377
Nakling, A. E., Aarsland, D., Naess, H., Wollschlaeger, D., Fladby, T., Hofstad, H., et al. (2017). Cognitive deficits in chronic stroke patients: neuropsychological assessment, depression, and self-reports. Dement. Geriatr. Cogn. Dis. Extra 7, 283–296. doi: 10.1159/000478851
Nasreddine, Z. S., Phillips, N. A., Bedirian, V., Charbonneau, S., Whitehead, V., Collin, I., et al. (2005). The Montreal cognitive assessment, MoCA: a brief screening tool for mild cognitive impairment. J. Am. Geriatr. Soc. 53, 695–699. doi: 10.1111/j.1532-5415.2005.53221.x
Nath, K., Ferguson, I., Puleio, A., Wall, K., Stark, J., Clark, S., et al. (2023). Brain health indicators following acute neuro-exergaming: biomarker and cognition in mild cognitive impairment (MCI) after pedal-n-play (iPACES). Brain Sci. 13:844. doi: 10.3390/brainsci13060844
Ng, M. M., Hill, K. D., Batchelor, F., and Burton, E. (2017). Factors predicting falls and mobility outcomes in patients with stroke returning home after rehabilitation who are at risk of falling. Arch. Phys. Med. Rehabil. 98, 2433–2441. doi: 10.1016/j.apmr.2017.05.018
Ng, S. S., and Hui-Chan, C. W. (2005). The timed up & go test: its reliability and association with lower-limb impairments and locomotor capacities in people with chronic stroke. Arch. Phys. Med. Rehabil. 86, 1641–1647. doi: 10.1016/j.apmr.2005.01.011
Ojala-Oksala, J., Jokinen, H., Kopsi, V., Lehtonen, K., Luukkonen, L., Paukkunen, A., et al. (2012). Educational history is an independent predictor of cognitive deficits and long-term survival in postacute patients with mild to moderate ischemic stroke. Stroke 43, 2931–2935. doi: 10.1161/STROKEAHA.112.667618
Olney, S. J., and Richards, C. (1996). Hemiparetic gait following stroke. Part I: Characteristics. Gait Posture 4, 136–148. doi: 10.1016/0966-6362(96)01063-6
Owen, A. M., McMillan, K. M., Laird, A. R., and Bullmore, E. (2005). N-back working memory paradigm: a meta-analysis of normative functional neuroimaging studies. Hum. Brain Mapp. 25, 46–59. doi: 10.1002/hbm.20131
Pacheco, T. B. F., de Medeiros, C. S. P., de Oliveira, V. H. B., Vieira, E. R., and de Cavalcanti, F. A. C. (2020). Effectiveness of exergames for improving mobility and balance in older adults: a systematic review and meta-analysis. Syst. Rev. 9:163. doi: 10.1186/s13643-020-01421-7
Patten, C., Lexell, J., and Brown, H. E. (2004). Weakness and strength training in persons with poststroke hemiplegia: rationale, method, and efficacy. J. Rehabil. Res. Dev. 41, 293–312. doi: 10.1682/jrrd.2004.03.0293
Peng, W., Lin, J. H., and Crouse, J. (2011). Is playing exergames really exercising? A meta-analysis of energy expenditure in active video games. Cyberpsychol. Behav. Soc. Netw. 14, 681–688. doi: 10.1089/cyber.2010.0578
Peng, Y., Wang, Y., Zhang, L., Zhang, Y., Sha, L., Dong, J., et al. (2024). Virtual reality exergames for improving physical function, cognition and depression among older nursing home residents: a systematic review and meta-analysis. Geriatr. Nurs. 57, 31–44. doi: 10.1016/j.gerinurse.2024.02.032
Ploughman, M., Austin, M. W., Glynn, L., and Corbett, D. (2015). The effects of poststroke aerobic exercise on neuroplasticity: a systematic review of animal and clinical studies. Transl. Stroke Res. 6, 13–28. doi: 10.1007/s12975-014-0357-7
Pollock, A., St George, B., Fenton, M., and Firkins, L. (2012). Top ten research priorities relating to life after stroke. Lancet Neurol. 11:209. doi: 10.1016/S1474-4422(12)70029-7
Potocnik, J., Ovcar Stante, K., and Rakusa, M. (2020). The validity of the montreal cognitive assessment (MoCA) for the screening of vascular cognitive impairment after ischemic stroke. Acta Neurol. Belg. 120, 681–685. doi: 10.1007/s13760-020-01330-5
Prat-Luri, A., Moreno-Navarro, P., García, J. A., Barbado, D., Vera-Garcia, F. J., and Elvira, J. L. L. (2020). Do initial trunk impairment, age, intervention onset, and training volume modulate the effectiveness of additional trunk exercise programs after stroke? A systematic review with Meta-analyses. Int. J. Environ. Res. Public Health 17. doi: 10.3390/ijerph17238714
Prosperini, L., Tomassini, V., Castelli, L., Tacchino, A., Brichetto, G., Cattaneo, D., et al. (2021). Exergames for balance dysfunction in neurological disability: a meta-analysis with meta-regression. J. Neurol. 268, 3223–3237. doi: 10.1007/s00415-020-09918-w
Pumpho, A., Chaikeeree, N., Saengsirisuwan, V., and Boonsinsukh, R. (2020). Selection of the better dual-timed up and go cognitive task to be used in patients with stroke characterized by subtraction operation difficulties. Front. Neurol. 11:262. doi: 10.3389/fneur.2020.00262
Rech, K. D., Salazar, A. P., Marchese, R. R., Schifino, G., Cimolin, V., and Pagnussat, A. S. (2020). Fugl-Meyer assessment scores are related with kinematic measures in people with chronic hemiparesis after stroke. J. Stroke Cerebrovasc. Dis. 29:104463. doi: 10.1016/j.jstrokecerebrovasdis.2019.104463
REDCap. (2018). Setting up the randomization module in REDCap – how-to guide. Available online at: https://www.ctsi.ufl.edu/files/2018/12/Setting-Up-the-Randomization-Module-in-REDCap.pdf (Accessed March 26, 2024).
Reitan, R. M. (1958). Validity of the trail making test as an indicator of organic brain damage. Percept. Mot. Skills 8, 271–276. doi: 10.2466/pms.1958.8.3.271
Rieker, J. A., Reales, J. M., Muinos, M., and Ballesteros, S. (2022). The effects of combined cognitive-physical interventions on cognitive functioning in healthy older adults: a systematic review and multilevel meta-analysis. Front. Hum. Neurosci. 16:838968. doi: 10.3389/fnhum.2022.838968
Rogers, J. M., Foord, R., Stolwyk, R. J., Wong, D., and Wilson, P. H. (2018). General and domain-specific effectiveness of cognitive remediation after stroke: systematic literature review and meta-analysis. Neuropsychol. Rev. 28, 285–309. doi: 10.1007/s11065-018-9378-4
Rozental-Iluz, C., Zeilig, G., Weingarden, H., and Rand, D. (2016). Improving executive function deficits by playing interactive video-games: secondary analysis of a randomized controlled trial for individuals with chronic stroke. Eur. J. Phys. Rehabil. Med. 52, 508–515. Available online at: https://www.ncbi.nlm.nih.gov/pubmed/26761562
RStudio_Team. (2020). RStudio: integrated development for F. RStudio, PBC, Boston. Available online at: http://www.rstudio.com/ (Accessed April 29, 2024)
Rudberg, A. S., Berge, E., Laska, A. C., Jutterstrom, S., Nasman, P., Sunnerhagen, K. S., et al. (2021). Stroke survivors' priorities for research related to life after stroke. Top. Stroke Rehabil. 28, 153–158. doi: 10.1080/10749357.2020.1789829
Rüth, M., Schmelzer, M., Burtniak, K., and Kaspar, K. (2023). Commercial exergames for rehabilitation of physical health and quality of life: a systematic review of randomized controlled trials with adults in unsupervised home environments. Front. Psychol. 14:1155569. doi: 10.3389/fpsyg.2023.1155569
Saa, J. P., Tse, T., Baum, C. M., Cumming, T., Josman, N., Rose, M., et al. (2021). Cognitive recovery after stroke: a meta-analysis and metaregression of intervention and cohort studies. Neurorehabil. Neural Repair 35, 585–600. doi: 10.1177/15459683211017501
Saeedi, S., Ghazisaeedi, M., and Rezayi, S. (2021). Applying game-based approaches for physical rehabilitation of poststroke patients: a systematic review. J. Healthc. Eng. 2021, 9928509–9928527. doi: 10.1155/2021/9928509
Sailer, M., Hense, J. U., Mayr, S. K., and Mandl, H. (2017). How gamification motivates: an experimental study of the effects of specific game design elements on psychological need satisfaction. Comput. Hum. Behav. 69, 371–380. doi: 10.1016/j.chb.2016.12.033
Scarpina, F., and Tagini, S. (2017). The Stroop color and word test. Front. Psychol. 8:557. doi: 10.3389/fpsyg.2017.00557
Schaeffer, E., Roeben, B., Granert, O., Hanert, A., Liepelt-Scarfone, I., Leks, E., et al. (2022). Effects of exergaming on hippocampal volume and brain-derived neurotrophic factor levels in Parkinson’s disease. Eur. J. Neurol. 29, 441–449. doi: 10.1111/ene.15165
Schellig, D., and Schuri, U. (2009). Vienna test system – N-Back verbal (NBV) – manual. Available online at: https://marketplace.schuhfried.com/en/NBV (Accessed March 15, 2022).
Schielzeth, H., Dingemanse, N. J., Nakagawa, S., Westneat, D. F., Allegue, H., Teplitsky, C., et al. (2020). Robustness of linear mixed-effects models to violations of distributional assumptions. Methods Ecol. Evol. 11, 1141–1152. doi: 10.1111/2041-210x.13434
Schulz, K. F., Altman, D. G., and Moher, D.CONSORT Group (2010). CONSORT 2010 statement: updated guidelines for reporting parallel group randomised trials. Trials 11:32. doi: 10.1186/1745-6215-11-32
Seinsche, J., de Bruin, E. D., Carpinella, I., Ferrarin, M., Moza, S., Rizzo, F., et al. (2022). Older adults' needs and requirements for a comprehensive exergame-based telerehabilitation system: a focus group study. Front. Public Health 10:1076149. doi: 10.3389/fpubh.2022.1076149
Selves, C., Stoquart, G., and Lejeune, T. (2020). Gait rehabilitation after stroke: review of the evidence of predictors, clinical outcomes and timing for interventions. Acta Neurol. Belg. 120, 783–790. doi: 10.1007/s13760-020-01320-7
Sennfalt, S., Norrving, B., Petersson, J., and Ullberg, T. (2019). Long-term survival and function after stroke: a longitudinal observational study from the Swedish stroke register. Stroke 50, 53–61. doi: 10.1161/STROKEAHA.118.022913
Shepard, R. N., and Metzler, J. (1971). Mental rotation of three-dimensional objects. Science 171, 701–703. doi: 10.1126/science.171.3972.701
Shi, D., Chen, X., and Li, Z. (2018). Diagnostic test accuracy of the Montreal cognitive assessment in the detection of post-stroke cognitive impairment under different stages and cutoffs: a systematic review and meta-analysis. Neurol. Sci. 39, 705–716. doi: 10.1007/s10072-018-3254-0
Snapinn, S. M., and Jiang, Q. (2007). Responder analyses and the assessment of a clinically relevant treatment effect. Trials 8:31. doi: 10.1186/1745-6215-8-31
Soares, V. N., Yoshida, H. M., Magna, T. S., Sampaio, R. A. C., and Fernandes, P. T. (2021). Comparison of exergames versus conventional exercises on the cognitive skills of older adults: a systematic review with meta-analysis. Arch. Gerontol. Geriatr. 97:104485. doi: 10.1016/j.archger.2021.104485
Sohrabji, F., Park, M. J., and Mahnke, A. H. (2017). Sex differences in stroke therapies. J. Neurosci. Res. 95, 681–691. doi: 10.1002/jnr.23855
Spikman, J., and van Zomeren, E. (2010). “Assessment of attention” in The handbook of clinical neuropsychology. eds. J. Gurd, U. Kischka, and J. Marshall. 2nd ed (Oxford: Oxford University Press), 81–96.
Stanmore, E., Stubbs, B., Vancampfort, D., de Bruin, E. D., and Firth, J. (2017). The effect of active video games on cognitive functioning in clinical and non-clinical populations: a meta-analysis of randomized controlled trials. Neurosci. Biobehav. Rev. 78, 34–43. doi: 10.1016/j.neubiorev.2017.04.011
Stojan, R., and Voelcker-Rehage, C. (2019). A systematic review on the cognitive benefits and neurophysiological correlates of exergaming in healthy older adults. J. Clin. Med. 8. doi: 10.3390/jcm8050734
Stretton, C. M., Mudge, S., Kayes, N. M., and McPherson, K. M. (2022). What does real-world walking mean to people with stroke? An interpretive descriptive study. Disabil Rehabil 44, 315–322. doi: 10.1080/09638288.2020.1767704
Sturm, W. (2006). Vienna test system – perceptional and attentional functions: alertness (WAFA) – manual. Available online at: https://marketplace.schuhfried.com/en/WAFA (Accessed March 15, 2022)
Sturnieks, D. L., Hicks, C., Smith, N., Ratanapongleka, M., Menant, J., Turner, J., et al. (2024). Exergame and cognitive training for preventing falls in community-dwelling older people: a randomized controlled trial. Nat. Med. 30, 98–105. doi: 10.1038/s41591-023-02739-0
Suleiman-Martos, N., García-Lara, R., Albendín-García, L., Romero-Béjar, J. L., Cañadas-De La Fuente, G. A., Monsalve-Reyes, C., et al. (2021). Effects of active video games on physical function in independent community-dwelling older adults: a systematic review and meta-analysis. J. Adv. Nurs. 78, 1228–1244. doi: 10.1111/jan.15138
Sultan, N., Khushnood, K., Qureshi, S., Altaf, S., Khan, M. K., Malik, A. N., et al. (2023). Effects of virtual reality training using Xbox Kinect on balance, postural control, and functional independence in subjects with stroke. Games Health J 12, 440–444. doi: 10.1089/g4h.2022.0193
Sun, J. H., Tan, L., and Yu, J. T. (2014). Post-stroke cognitive impairment: epidemiology, mechanisms and management. Ann Transl Med 2:80. doi: 10.3978/j.issn.2305-5839.2014.08.05
Sun, Q. Q., Xu, S. R., Guo, S., You, Y., Xia, R., and Liu, J. (2021). Effects of combined physical activity and cognitive training on cognitive function in older adults with subjective cognitive decline: a systematic review and meta-analysis of randomized controlled trials. Evid. Based Complement. Alternat. Med. 2021, 1–14. doi: 10.1155/2021/8882961
Swinnen, N., Vandenbulcke, M., de Bruin, E. D., Akkerman, R., Stubbs, B., Firth, J., et al. (2021). The efficacy of exergaming in people with major neurocognitive disorder residing in long-term care facilities: a pilot randomized controlled trial. Alzheimers Res. Ther. 13:70. doi: 10.1186/s13195-021-00806-7
Tahmosybayat, R., Baker, K., Godfrey, A., Caplan, N., and Barry, G. (2018). Movements of older adults during exergaming interventions that are associated with the systems framework for postural control: a systematic review. Maturitas 111, 90–99. doi: 10.1016/j.maturitas.2018.03.005
Taylor, L. M., Kerse, N., Frakking, T., and Maddison, R. (2018). Active video games for improving physical performance measures in older people: a meta-analysis. J. Geriatr. Phys. Ther. 41, 108–123. doi: 10.1519/JPT.0000000000000078
Teraz, K., Slosar, L., Paravlic, A. H., de Bruin, E. D., and Marusic, U. (2022). Impact of motor-cognitive interventions on selected gait and balance outcomes in older adults: a systematic review and meta-analysis of randomized controlled trials. Front. Psychol. 13:837710. doi: 10.3389/fpsyg.2022.837710
Tombaugh, T. N. (2004). Trail making test A and B: normative data stratified by age and education. Arch. Clin. Neuropsychol. 19, 203–214. doi: 10.1016/S0887-6177(03)00039-8
Tsurayya, G., Duta, T. F., Naufal, M. A., Alina, M., Isitua, C. C., and Ohanu, E. C. (2023). Acceptance, safety, and impact on quality of life of exergame for elderly patients with neurodegenerative diseases: a systematic review and meta-analysis. Narra X 1. doi: 10.52225/narrax.v1i3.94
Umarova, R. M., Schumacher, L. V., Schmidt, C. S. M., Martin, M., Egger, K., Urbach, H., et al. (2021). Interaction between cognitive reserve and age moderates effect of lesion load on stroke outcome. Sci. Rep. 11:4478. doi: 10.1038/s41598-021-83927-1
Unibaso-Markaida, I., and Iraurgi, I. (2022). Commercial videogames in stroke rehabilitation: systematic review and meta-analysis. Top. Stroke Rehabil. 29, 551–567. doi: 10.1080/10749357.2021.1943798
Unibaso-Markaida, I., Iraurgi, I., Ortiz-Marqués, N., Amayra, I., and Martínez-Rodríguez, S. (2019). Effect of the Wii sports resort on the improvement in attention, processing speed and working memory in moderate stroke. J. Neuroeng. Rehabil. 16:32. doi: 10.1186/s12984-019-0500-5
Ursin, M. H., Bergland, A., Fure, B., Thommessen, B., Hagberg, G., Oksengard, A. R., et al. (2019). Gait and balance one year after stroke; relationships with lesion side, subtypes of cognitive impairment and neuroimaging findings-a longitudinal, cohort study. Physiotherapy 105, 254–261. doi: 10.1016/j.physio.2018.07.007
Valenzuela, T., Okubo, Y., Woodbury, A., Lord, S. R., and Delbaere, K. (2018). Adherence to technology-based exercise programs in older adults: a systematic review. J. Geriatr. Phys. Ther. 41, 49–61. doi: 10.1519/JPT.0000000000000095
Verghese, J., Wang, C., Lipton, R. B., and Holtzer, R. (2013). Motoric cognitive risk syndrome and the risk of dementia. J. Gerontol. A Biol. Sci. Med. Sci. 68, 412–418. doi: 10.1093/gerona/gls191
Verstraeten, S., Mark, R., and Sitskoorn, M. (2016). Motor and cognitive impairment after stroke: a common bond or a simultaneous deficit. Stroke Res. Ther 1. Available online at: https://stroke.imedpub.com/motor-and-cognitive-impairment-after-strokea-common-bond-or-a-simultaneous-deficit.php?aid=9074 (Accessed February 01, 2022).
Virani, S. S., Alonso, A., Benjamin, E. J., Bittencourt, M. S., Callaway, C. W., Carson, A. P., et al. (2020). Heart Disease and Stroke Statistics-2020 update: a report from the American Heart Association. Circulation 141, e139–e596. doi: 10.1161/CIR.0000000000000757
Wicaksono, P. P., Rahayu, U. B., and Maghfiroh, R. A. (2023). "Check for updates effect of Exergame on post-stroke management: a literature review", in: Proceedings of the International Conference on Health and Well-Being (ICHWB 2022): (Springer Nature), 490.
Wiley, R. W., and Rapp, B. (2019). Statistical analysis in small-N designs: using linear mixed-effects modeling for evaluating intervention effectiveness. Aphasiology 33, 1–30. doi: 10.1080/02687038.2018.1454884
Williams, L. S., Yilmaz, E. Y., and Lopez-Yunez, A. M. (2000). Retrospective assessment of initial stroke severity with the NIH stroke scale. Stroke 31, 858–862. doi: 10.1161/01.str.31.4.858
Wollesen, B., Wildbredt, A., van Schooten, K. S., Lim, M. L., and Delbaere, K. (2020). The effects of cognitive-motor training interventions on executive functions in older people: a systematic review and meta-analysis. Eur. Rev. Aging Phys. Act. 17:9. doi: 10.1186/s11556-020-00240-y
Wu, C. Y., Hung, S. J., Lin, K. C., Chen, K. H., Chen, P., and Tsay, P. K. (2019). Responsiveness, minimal clinically important difference, and validity of the MoCA in stroke rehabilitation. Occup. Ther. Int. 2019, 2517658–2517657. doi: 10.1155/2019/2517658
Yang, B., and Wang, S. M. (2021). Meta-analysis on cognitive benefit of exercise after stroke. Complexity 2021. doi: 10.1155/2021/5569346
Yang, L., He, C., and Pang, M. Y. (2016). Reliability and validity of dual-task mobility assessments in people with chronic stroke. PLoS One 11:e0147833. doi: 10.1371/journal.pone.0147833
Yang, Y., Wang, K., Liu, S., Liu, H., Zhang, T., and Luo, J. (2023). Exergames improve cognitive function in older adults and their possible mechanisms: a systematic review. J. Glob. Health 13:04177. doi: 10.7189/jogh.13.04177
Yang, Y. R., Tsai, M. P., Chuang, T. Y., Sung, W. H., and Wang, R. Y. (2008). Virtual reality-based training improves community ambulation in individuals with stroke: a randomized controlled trial. Gait Posture 28, 201–206. doi: 10.1016/j.gaitpost.2007.11.007
Yen, H.-Y., and Chiu, H.-L. (2021). Virtual reality exergames for improving older adults’ cognition and depression: a systematic review and meta-analysis of randomized control trials. J. Am. Med. Dir. Assoc. 22, 995–1002. doi: 10.1016/j.jamda.2021.03.009
Yoong, S. Q., Wu, V. X., Chen, C., Lee, P. Y., Wee, K. S. L., Teo, J., et al. (2024). Dance Exergames for older adults: a systematic review and Meta-analysis with narrative synthesis. J. Gerontol. A Biol. Sci. Med. Sci. 79. doi: 10.1093/gerona/glae035
Yu, R. W. L., and Chan, A. H. S. (2021). Meta-analysis of the effects of game types and devices on older adults-video game interaction: implications for video game training on cognition. Appl. Ergon. 96:103477. doi: 10.1016/j.apergo.2021.103477
Zeng, X., Balikuddembe, J. K., and Liang, P. (2023). Impact of community-based rehabilitation on the physical functioning and activity of daily living of stroke patients: a systematic review and meta-analysis. Disabil. Rehabil. 45, 403–414. doi: 10.1080/09638288.2022.2037755
Zhang, B., Li, D., Liu, Y., Wang, J., and Xiao, Q. (2021). Virtual reality for limb motor function, balance, gait, cognition and daily function of stroke patients: a systematic review and meta-analysis. J. Adv. Nurs. 77, 3255–3273. doi: 10.1111/jan.14800
Zhang, B., Wong, K. P., and Qin, J. (2023). Effects of virtual reality on the limb motor function, balance, gait, and daily function of patients with stroke: systematic review. Medicina (Kaunas) 59:813. doi: 10.3390/medicina59040813
Zhang, J., Luximon, Y., Pang, M. Y. C., and Wang, H. (2022). Effectiveness of exergaming-based interventions for mobility and balance performance in older adults with Parkinson's disease: systematic review and meta-analysis of randomised controlled trials. Age Ageing 51. doi: 10.1093/ageing/afac175
Zhou, Q., Yang, H., Zhou, Q., and Pan, H. (2021). Effects of cognitive motor dual-task training on stroke patients: a RCT-based meta-analysis. J. Clin. Neurosci. 92, 175–182. doi: 10.1016/j.jocn.2021.08.009
Zhu, X., Yin, S., Lang, M., He, R., and Li, J. (2016). The more the better? A meta-analysis on effects of combined cognitive and physical intervention on cognition in healthy older adults. Ageing Res. Rev. 31, 67–79. doi: 10.1016/j.arr.2016.07.003
Keywords: stroke, exergaming, rehabilitation, cognition, gait, dual-task
Citation: Huber SK, Knols RH, Held JPO, Betschart M, Gartmann S, Nauer N and de Bruin ED (2025) PEMOCS: effects of a concept-guided, PErsonalized, MOtor-Cognitive exergame training on cognitive functions and gait in chronic Stroke—a randomized, controlled trial. Front. Aging Neurosci. 17:1514594. doi: 10.3389/fnagi.2025.1514594
Received: 21 October 2024; Accepted: 27 February 2025;
Published: 13 March 2025.
Edited by:
Ankit Vijayvargiya, Dublin City University, IrelandReviewed by:
Matthieu Gallou-Guyot, The University of Tokyo, JapanCopyright © 2025 Huber, Knols, Held, Betschart, Gartmann, Nauer and de Bruin. This is an open-access article distributed under the terms of the Creative Commons Attribution License (CC BY). The use, distribution or reproduction in other forums is permitted, provided the original author(s) and the copyright owner(s) are credited and that the original publication in this journal is cited, in accordance with accepted academic practice. No use, distribution or reproduction is permitted which does not comply with these terms.
*Correspondence: E. D. de Bruin, ZWxpbmcuZGVicnVpbkBoZXN0LmV0aHouY2g=
Disclaimer: All claims expressed in this article are solely those of the authors and do not necessarily represent those of their affiliated organizations, or those of the publisher, the editors and the reviewers. Any product that may be evaluated in this article or claim that may be made by its manufacturer is not guaranteed or endorsed by the publisher.
Research integrity at Frontiers
Learn more about the work of our research integrity team to safeguard the quality of each article we publish.