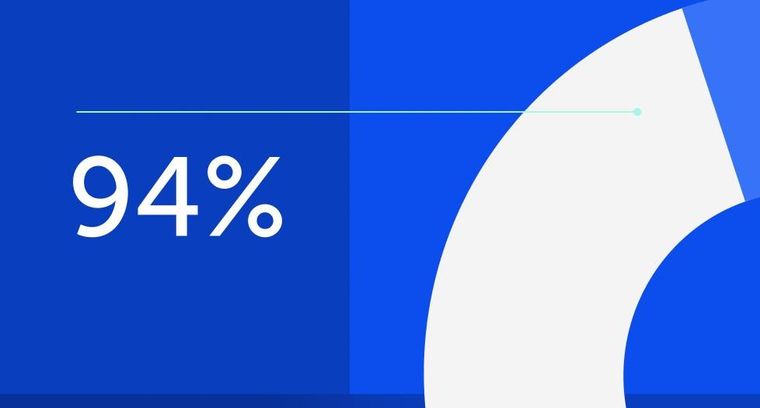
94% of researchers rate our articles as excellent or good
Learn more about the work of our research integrity team to safeguard the quality of each article we publish.
Find out more
REVIEW article
Front. Aging Neurosci., 15 June 2023
Sec. Cellular and Molecular Mechanisms of Brain-aging
Volume 15 - 2023 | https://doi.org/10.3389/fnagi.2023.1179988
This article is part of the Research TopicSystem Approaches to Advance Drug Discovery Against Aging and Age-Related DiseasesView all 6 articles
The glymphatic system is a brain-wide perivascular pathway driven by aquaporin-4 on the endfeet of astrocytes, which can deliver nutrients and active substances to the brain parenchyma through periarterial cerebrospinal fluid (CSF) influx pathway and remove metabolic wastes through perivenous clearance routes. This paper summarizes the composition, overall fluid flow, solute transport, related diseases, affecting factors, and preclinical research methods of the glymphatic system. In doing so, we aim to provide direction and reference for more relevant researchers in the future.
It is traditionally believed that the lymphatic system doesn’t exist in the central nervous system (Nedergaard and Goldman, 2016). Thus, cell debris, potential neurotoxic proteins, and other metabolites with large molecular weight are considered to be removed in a different clearance pathway than brain vasculature. In 2012, the Nedergaard (Iliff et al., 2012) group found that cerebrospinal fluid (CSF) can enter brain parenchyma and exchange with brain interstitial fluid (ISF) in the presence of aquaporin-4 (AQP4) on astrocytes. Likewise, when the mixed fluid was drained from the brain, Amyloid-β (Aβ) was transported along with the outflow. Since the function of this “drainage” pathway is similar to that of the lymphatic system and supported by astrocytes, it was named the glymphatic system sooner after glia (Jessen et al., 2015).
Since last decade, many researchers in the field of neurology, neurodegenerative diseases and physiology have aimed to study and develop the glymphatic system, providing a brand-new perspective for us to understand brain diseases: the overall fluid flow of the brain rather than a specific lesion or structure. Herein, we summarized the components of the glymphatic system, the fluid circulation mode within this system, how pathogenic solutes are transported in certain diseases, affecting factors of its function, and by what means we can study the glymphatic system. All above may provide direction and reference for brain diseases and medical researchers.
The glymphatic system is a brain-wide perivascular pathway driven by AQP4 on astrocytic endfeet, which can deliver nutrients and neuroactive substances to the brain parenchyma through peri-arterial CSF influx pathway and remove metabolic wastes through peri-venous clearance routes (Gouveia-Freitas and Bastos-Leite, 2021). This system is mainly composed of three elements: peri-arterial CSF influx realized by AQP4 on astrocytes, infusion of CSF and ISF in the brain parenchyma, and peri-venous clearance routes (Szczygielski et al., 2021). Therefore, the function of the glymphatic system is closely related to two structures: the Perivascular Space (PVS) and AQP4 on astrocytes.
In the 19th century, Rudolf Virchow and Charles Robin found annular tunnel spaces around penetrating arterioles in the brain parenchyma and named them Virchow-Robin spaces (VRS). Subsequently, researchers found that all arterioles, capillaries, and venules in the brain parenchyma were surrounded by this structure resembling a donut-shaped tunnel, and called it Perivascular spaces (PVS) (Zhang et al., 1990). The inner wall of PVS comprises vascular cells (mostly endothelial cells and smooth muscle cells), while the outer wall is built by perivascular astrocytic endfeet (Yao D. et al., 2022). VRS is connected with space around pial arteries, and the liquid it is composed of is CSF (Jessen et al., 2015). As the penetrating arterioles narrow deeper down in the brain parenchyma, the CSF-containing PVS becomes continuous with the basal lamina. The basal lamina mainly comprises laminin, fibronectin, type IV collagen, and heparin sulfate proteoglycan. Due to the loose structure of the extracellular matrix of these cells, the basal lamina has the least resistance to CSF influx. CSF will flow downwards from the VRS along the peri-arterial space, enter the basal layer surrounding capillaries, and exit along the perivenous space (Jessen et al., 2015). The structure of PVS is shown in Figure 1.
Figure 1. The structure of VRS. VRS is an annular tunnel space around penetrating arterioles in the brain parenchyma, filled with CSF. CSF can enter the brain parenchyma through PVS.
In 2012, researchers injected fluorescent tracers with different molecular weights into the cisterna magna of anesthetized mice, followed by in vivo two-photon imaging and immunofluorescence techniques to track the CSF circulation through the brain interstitial space. Results from this study demonstrated that CSF entered brain parenchyma along the PVS and rapidly exchanged with ISF. Then the mixture of CSF and ISF was cleared along the perivenous drainage pathways (Iliff et al., 2012). Notably, this process was supported by AQP4 in astrocytes.
Astrocytes provide a link between blood vessels and neurons. They can transmit neural activities from synapses to the basal lamina, where vascular endothelial cells, pericytes, and astrocytes are located (Lu and Gao, 2022). The increase of Ca2+ in astrocytes caused by neurotransmitters leads to the synthesis and release of vasoactive metabolites such as TGF-β, glial-derived neurotrophic factor (GDNF), b-fibroblast growth factor (bFGF), interleukin-6 (IL-6) and angiopoietins, prostaglandin E2 (PGE2), epoxyeicosatrienoic acids (EETs), and 20-hydroxyeicosatetraenoic acid (20-HETE) (Bicker et al., 2014; MacVicar and Newman, 2015), leading to vasoconstriction and vasorelaxation (Burn et al., 2021), further causing changes in PVS space. In addition, there are 20 nm clefts between the endfeet of astrocytes around PVS, allowing macromolecular solutes to pass through (Czigler et al., 2020).
AQP4s are located in surfaces of the blood–brain barrier and CSF–brain barrier and are expressed in astrocyte endfeet processes surrounding capillaries. Astrocyte processes comprise the subpial and subependymal glial-limiting membranes and the perivascular astrocytic end-foot processes that circumscribe the entirety of the cerebrovasculature (Zhao Z. et al., 2022). The distribution of AQP4 is shown in Figure 2. As one of the essential components of astrocyte endfeet that constitutes the outer wall of PVS, AQP4 mediates fluid and small molecular substances (molecular weight:0∼18; diameter:0∼0.38 nm) in CSF to enter the brain parenchyma (Peng et al., 2023). By injecting several CSF tracers with different molecular weights and contrast medium into AQP4 knock-out (KO) mice or mice that lack perivascular AQP4 (Snta1 KO), there is a significant decrease in CSF tracer transport in KO mice and rats compared to controls. Notably, these findings suggest that AQP4 on the astrocytes supports perivascular CSF influx and ISF outflow in the glymphatic system (Mestre et al., 2018).
Figure 2. The distribution of AQP-4. AQP4 is located at the ependymal cells and the astrocyte processes (which face CSF–brain and blood–brain barriers).
Moreover, the glymphatic system is not only a specific tissue or structure but also a functional generalization of the flow and transportation of fluid in the brain. After CSF enters the brain parenchyma, it mixes with ISF, exchanges substances, and clears the metabolites of brain tissue through the perivenular outflow channel. A functional diagram of the glymphatic system is shown in Figure 3. The proposal of the glymphatic system has updated our understanding of fluid circulation in the brain, which is of significant importance.
Figure 3. Functional diagram of the glymphatic system. CSF delivers nutrients and neuroactive substances to the brain parenchyma through the peri-arterial spaces pathway and mixes with ISF in the brain parenchyma. The mix of CSF and ISF removes metabolic wastes through peri-venous clearance routes.
There are four kinds of fluid in the brain: blood, CSF, ISF, and intracellular fluid. Blood in vessels and ISF in brain parenchyma are separated by the blood-brain barrier (BBB), while blood and CSF are separated by the blood-cerebrospinal fluid barrier (B-CSFB) (Markowicz-Piasecka et al., 2022). The composition of BBB and B-CSFB is shown in Figure 4. Blood generates CSF through choroid plexus capillary endothelial cells distributed in the lateral ventricle, third ventricle, and fourth ventricle and enters the ventricles and subarachnoid space for storage (Saunders et al., 2023). The process of CSF production requires the Na/K-ATPase and aquaporin 1 (AQP1) scattered on the choroid plexus epithelial cells (de Laurentis et al., 2021). Simultaneously, some solutes and water in the blood can enter the brain tissue through the BBB and become parts of ISF. With pial arteries in the CSF-containing subarachnoid space becoming penetrating arteries upon diving into the brain parenchyma (Iliff et al., 2013a), CSF enters the brain parenchyma through the perivascular spaces of penetrating arteries, which AQP4 drives on the endfeet of astrocytes (Mogensen et al., 2021). In brain parenchyma, CSF mixes with ISF and exchanges substances. This indicates that CSF becomes an additional source of ISF through the glymphatic system (Nedergaard and Goldman, 2016). Notably, CSF and ISF exit the brain parenchyma through three pathways: (1) Perineural sheaths surrounding the head and face. CSF enters the nasal mucosa along the olfactory nerve’s nerve sheath towards the nasal mucosa’s lymphatic vessels. From here, the CSF is drained to the cervical lymph nodes. Other perineural efflux pathways in rodents are the trigeminal, glossopharyngeal, vagal, and spinal accessory nerves (Lundgaard et al., 2017). (2) Dural lymphatic vessels. Dural lymphatic vessels distribute on the dura mater, the sigmoid sinus, the retro glenoid vein, the middle meningeal artery, and the pterygopalatine artery (Louveau et al., 2015). Dural lymphatic vessels absorb CSF from the adjacent subarachnoid space and ISF from the glymphatic system and transport fluid into deep cervical LNs (dcLNs) via foramina at the base of the skull (Aspelund et al., 2015). (3) Arachnoid granulations. CSF in the subarachnoid space flows from the arachnoid granulations into the sagittal sinus and is directly discharged into the blood (Pollay, 2010). The fluid circulation is shown in Figure 5.
Figure 4. The composition of BBB and B-CSFB. The composition of BBB: A layer of endothelial cells interconnected through tight junctions and not containing fenestrations, basal membrane consisting of the basal lamina of astrocytes and basal lamina of endothelial cells, protrusions of astrocytes (Zhang et al., 2022). The composition of B-CSFB: choroidal epithelial cells interconnected by tight junctions (which are more permeable than the junctions between the endothelial cells of brain capillaries), basal membrane, endothelium of the pia mater capillaries containing fenestrations (Jessen et al., 2015).
Figure 5. (A) The fluid circulation in the glymphatic system (Rasmussen et al., 2018). Blood generates CSF through choroid plexus capillary endothelial cells distributed in the lateral, third, and fourth ventricle. CSF flows from the ventricular system to the subarachnoid space of the brain and spinal cord. CSF in the subarachnoid space enters brain parenchyma through the perivascular spaces of penetrating arteries, which AQP4 drives on the end feet of astrocytes. Additionally, CSF mixes with ISF in brain parenchyma. The mixture of CSF and ISF subsequently enters the perivenous space. Egress sites of cranial fluid (orange arrows) fall into three functional categories: the perineural sheaths surrounding cranial and spinal nerves, dural lymphatic vessels, and arachnoid granulations. Finally, the mixture of CSF and ISF is drained to the cervical lymph nodes. (B) The fluid circulation in the glymphatic system.
Traditionally, fluid circulation in the glymphatic system is thought to be driven by pressure difference, respiration, and arterial pulsation (Nakada and Kwee, 2019). CSF is constantly generated in the choroid plexus, creating a pressure that causes fluid to flow from the ventricular to the subarachnoid space. Breathing also plays a vital role in the circulation of CSF. Researchers have found that during deep breathing, each inhalation will cause high CSF flow, and breath holding will inhibit it. Notably, this may be related to the reduction of chest pressure during inhalation (Djukic et al., 2022). When the internal carotid artery was ligated unilaterally, the arterial pulsation was reduced by about 50%, and the rate of CSF-ISF exchange was slowed down. When the adrenergic agonist Dobutamine is used throughout the body, the pulsation of the penetrating artery increases by about 60%, and the rate of CSF-ISF exchange in perivascular is accelerated (Iliff et al., 2013b). These findings indicate that arterial pulsation promotes the flow of CSF in the glymphatic system through the perivascular space. In recent years, the discovery of parenchymal border macrophages (PBMs) located in pia mater and rain arterial tree orders has updated fluid flow dynamics in the brain. The subpopulation of PBMs that expresses high levels of CD163 and LYVE1 (scavenger receptor protein) regulates arterial brain movement and changes CSF flow (Drieu et al., 2022). Pharmacological or genetic depletion of PBMs led to obstructed CSF and accumulated extracellular matrix proteins (Drieu et al., 2022).
The glymphatic system complements the problems that cannot be explained in the traditional CSF circulation theory. For example, in traditional theory, CSF is mainly produced by the choroid plexus (Thompson et al., 2022). In this case, removing the choroid plexus should have a certain effect on patients with hydrocephalus. Still, the clinical data found that removing the choroid has no obvious effect on the treatment of hydrocephalus (Oresković and Klarica, 2010). The concept of a glymphatic system gives the hypothesis that ISF enters the subarachnoid space through PVS and becomes one of the CSF sources. In traditional CSF circulation theory, the elimination of metabolites in the brain is mainly completed by diffusion. But the production and transport of metabolites in the brain are rapid, which is difficult to explain by diffusion (Cserr et al., 1981). Bulk flow in the glymphatic system can properly supplement the traditional theory so that people can understand why substances with different molecular weights are injected into the caudate nucleus and can be eliminated from the brain at almost the same speed.
There are two categories of solutes transported in the glymphatic system: (1) Nutrients (such as glucose and lipids) (Lundgaard et al., 2015) and neuroactive substances (such as transthyretin and apolipoprotein E) (Kassem et al., 2006; Xu et al., 2006); (2) Metabolites in the central nervous system, such as amyloid β(Aβ) and α-Synuclein. Studies have also shown that glucose, lipids, growth factors, neurotransmitters, neuroactive substances, and medicine injected into CSF (such as anti-tumor medicine) can be transported to the brain through the influx of CSF (Kassem et al., 2006; Xu et al., 2006; Rangroo Thrane et al., 2013; Lundgaard et al., 2015). Especially in lipid transport, the glymphatic system plays a central role in the brain. Metabolites in the central nervous system, such as Aβ, α-Synuclein, tau protein, and lactic acid, can also be discharged from the brain through the glymphatic system (Rasmussen et al., 2022). Aβ exists in the brain interstitium, and its abnormal precipitation is closely related to neurodegenerative diseases such as Alzheimer’s (Zhao J. et al., 2022). From investigations, the fluorescence-labeled Aβ is transported along the glymphatic system pathway in the central nervous system. When AQP4 is knocked out, the clearance of Aβ in the central nervous system slows down due to fluids congestion because Aβ is mainly removed by bulk flow along the gliovascular clearance system rather than locally across the BBB (Iliff et al., 2012). For solutes that cannot pass through the BBB, the fluxion of CSF in the glymphatic system may be the only medium for them to inflow and outflow the brain parenchyma. Therefore, the study of the glymphatic system is conducive to exploring how medicine molecules enter the brain parenchyma without passing through the BBB and promote the clearance of brain pathogenic factors, which may benefit neurodegenerative disease therapies.
Alzheimer’s disease (AD) is a neurodegenerative disease mainly characterized by memory impairment and decreases in brain volume (Choi and Tanzi, 2023). The pathogenesis of AD is related to the formation of senile plaques by Aβ (Ma et al., 2017), neurofibrillary tangles caused by abnormal accumulation of tau protein (Reeves et al., 2020), and depolarization of AQP4(AQP4 polarization: AQP4 is mainly expressed in astrocytes’ endfeet; AQP4 depolarization: AQP4 expression is transferred from astrocytes’ endfeet to the whole astrocytes cell body) on the astrocytes (Lynch et al., 2022). In APP/PS1 mice (a model of AD), toxic Aβ (such as soluble oligomers) accumulation is related to malfunction of glymphatic system transport, which is quantified by the solute inflow into the brain from CSF and clearance from the brain by radio-labeled tracers. Notably, decreased glymphatic system transport is associated with Aβ40 entering and accumulating in the perivascular spaces, which is related to AQP4 depolarization (Iliff et al., 2012). Thus, a malfunction of the glymphatic system preceded significant amyloid-β deposits, which may be an early signal of AD (Uchida, 2022).
Parkinson’s disease (PD) is a chronic neurodegenerative disease related to unbalanced production and clearance of α-syn (Scott-Massey et al., 2022). When meningeal lymphatic drainage was blocked by ligating the deep cervical lymph nodes of A53T mice (PD model), the glymphatic inflow of CSF tracer in the mice’s brain was reduced, which resulted in more severe α-syn accumulation, neuroglia activation, inflammation, loss of dopaminergic neurons and dyskinesia (Zou et al., 2019). The deletion of AQP4 inhibited the clearance of α-syn in the brain, manifested by the increase of protein monomers but not the increase of oligomers (Bobela et al., 2015). These indicate that AQP4 and meningeal lymphatic drainage are partially responsible for clearance of α-syn soluble monomers, suggesting a promising direction for PD symptom alleviation.
Stroke is mainly divided into hemorrhagic and ischemic stroke, characterized by local nerve dysfunction due to cerebral blood circulation disorder (Xu et al., 2023). Hemorrhagic stroke includes cerebral and subarachnoid hemorrhage, and ischemic stroke includes cerebral infarction and cerebral thrombosis (Li and Chen, 2022). After 24h of subarachnoid hemorrhage, immunohistochemical results showed that fibrin clots blocked the perivascular spaces of cerebral cortical vessels (Pu et al., 2019), and the glymphatic system function could be repaired by intraventricular injection of tissue plasminogen activator (t-PA) (Bosche et al., 2020). This indicates that subarachnoid hemorrhage leads to disorders of the glymphatic system and related pathological damage. Notably, targeted improvement of cerebral glymphatic clearance may become a new strategy for treating hemorrhagic stroke. In the ischemic stroke model, pathological changes similar to subarachnoid hemorrhagic stroke can be observed, such as the expansion of perivascular space, AQP4 polarity distribution changed from normal perivascular pattern to scattered parenchymal pattern (Back et al., 2017; Wang et al., 2017). Therefore, protecting and maintaining the normal function of glymphatic system will help attenuate stroke injury and also help improve cognitive impairment due to stroke.
Brain edema is a pathological phenomenon of brain tissue damage caused by increased brain volume and cranial pressure due to excessive intracellular or intercellular fluid. Brain edema is traditionally divided into two phases: early cytotoxic and later vasogenic phases (Mestre et al., 2020). In cytotoxic edema, energy deficiency caused by hypoxia promotes CSF influx in the glymphatic system and inhibits ISF efflux (Thrane et al., 2014). Additionally, AQP4 protein expression positively correlates with the degree of brain edema (Mamtilahun et al., 2019). Moreso, vasogenic brain edema is mainly caused by the destruction of the BBB and the increase of capillary permeability, resulting in a large amount of liquid entering the extracellular fluid. Importantly, AQP4 is considered the mechanism of water removal from the interstitial spaces, and vasogenic edema and intracranial pressure can be improved by regulating the role of AQP4 (Zhou et al., 2022). The conclusions of AQP4 in the cytotoxic and vasogenic edema models are entirely different, suggesting that AQP4 may be a selective two-way water channel. Moreover, liquid flow direction depends on whether the BBB is intact: when the BBB remains intact, AQP4 transports water into the ISF, and when the BBB is damaged, it becomes reverse transport (Assentoft et al., 2015). However, the mechanism of this process needs to be explored.
Traumatic brain injury (TBI) is caused by brain tissue trauma, which leads to brain dysfunction (Sivandzade et al., 2020). Following brain injury, astrocytes can release several vasoactive substances, such as isoprostanes (vasoconstrictors of cerebral arterioles) and endothelin 1 (causes vasoconstriction related to calcium influx), which could cause decreased cerebral perfusion (Jullienne et al., 2016). Notably, TBI causes the accumulation of Aβ and tau protein (Hicks et al., 2022), which is a risk factor for neurodegenerative diseases (such as dementia, AD, etc.) (Gao et al., 2022). Likewise, injury in mice’s brain parenchyma causes the loss of polarity distribution of AQP4 on the endfeet of astrocytes. As a result, the function of the glymphatic system decreases by about 60% and lasts for at least one month (Iliff et al., 2014). These indicate that chronic injury of the glymphatic system after TBI may be a key factor leading to tau protein aggregation and neurodegenerative attacks in the brain after trauma. Therefore, further exploration of the glymphatic system can help find ways to alleviate TBI and prevent the transformation to degenerative diseases after TBI.
Multiple sclerosis (MS) is a chronic immune dysfunction disease characterized by inflammation and demyelinating plaques (Dobson and Giovannoni, 2019). The characteristic pathological marker of MS is perivenous inflammatory lesions, causing demyelinating plaques (Karussis, 2014). Non-invasive diffusion MRI was used to calculate the diffusion along the perivascular space index (a proxy for glymphatic function). Notably, results showed that the diffusion along the PVS in patients with multiple sclerosis was lower, and this lower diffusion index was associated with more severe clinical disabilities and longer disease duration (Carotenuto et al., 2022; Verghese et al., 2022). Research has also found that MS patients are more likely to detect enlarged perivascular spaces in MRI and suggested that using CSF diversion to decongest perivascular spaces, which possibly improves glymphatic function and interstitial removal of proinflammatory molecules from CSF, finally downregulating local inflammation (Granberg et al., 2020; Scollato et al., 2022). The depolarization of AQP4 was found in the cuprizone-induced demyelination model and chronic MS lesions of post-mortem human subjects, which may be related to metabolic injury to the brain (Rohr et al., 2020). These findings suggest that the function of the glymphatic system is impaired during the process of MS, particularly in progressive stages, which implies that the glymphatic system is deeply involved in multiple sclerosis.
Amyotrophic lateral sclerosis (ALS) is a progressive degenerative motor neuron disease characterized by the selective death of motor neurons (Giovannelli et al., 2022). Although the underlying cause of ALS is not yet fully understood, its pathological mechanisms involve proteostasis, glutamate excitotoxicity, dysregulation of RNA metabolism, nuclear-cytoplasmic transport, and autophagy. More than 97% of ALS cases show the presence of insoluble proteins such as TDP-43 and tau protein in the brain. Furthermore, tau protein elevation in CSF indicates upper motor neuron degeneration, leading to ALS (Süssmuth et al., 2010). Therefore, promoting the clearance of tau protein in the glymphatic system may be an effective way to treat ALS. When astrocytes are exposed to ALS-CSF, their morphology is changed and accompanied by increased GFAP reactivity, further acquiring neuroinflammatory features (Mishra et al., 2016). Sleep, which plays an essential role in the clearance of the glymphatic system, is also disrupted in patients with amyotrophic lateral sclerosis (Boentert, 2019). Thus, these findings suggest that further exploring the functional mechanism of the glymphatic system will be helpful to better explain the physiological and pathological mechanism of ALS.
Diabetic patients often have cognitive and memory decline, and are at higher risk of vascular diseases and AD (Li Z. et al., 2022). MRI analysis of Type-2 diabetic rats showed the clearance rate of contrast agent Gd-DTPA in CSF from interstitial space in the hippocampus was three times slower than that of non-DM rats (diabetes rat model), which was confirmed by fluorescence imaging analysis (Jiang et al., 2017). These results indicate Type-2 diabetes can inhibit the exchange flow of CSF and ISF, leading to impaired glymphatic system function. The impairment may be due to the expansion of the perivascular space. The metabolic wastes accumulated in expanded perivascular space will trigger an inflammatory reaction, thus causing further expansion of perivascular space (Yao X. Y. et al., 2022). The study of the glymphatic system provides a new explanation for cognitive impairment caused by diabetes, but its mechanism is still unclear and requires further experiments to warrant.
A migraine is a recurrent unilateral or bilateral fluctuating headache. Cortical spreading depression (CSD) is a potential signal that waveform can be characterized by an intense neuronal activity that slowly progresses over the cortex and a period of neuronal inactivity (Pi et al., 2022). Acute puncture and KCl were used to induce transient CSD in mice. CSD can induce quick closure of penetrating periarterial and perivenous space on the cortical surface, lasting for several minutes and gradually recovering within 30 min (Schain et al., 2017). During CSD, the common occurrence of white matter lesions in headaches results from liquid and waste retention in the interstitial space in the distended perivascular space (Toriello et al., 2021). Therefore, accelerating the flow of CSF to improve the clearance of wastes in the perivascular space may be a new direction for treating migraine.
The discovery of the glymphatic system gives us a new understanding of the efficient fluid transport and metabolite clearance of the central nervous system, which can help us better understand the pathogenesis of diseases and find new ways to treat them. Notably, the abnormal accumulation of Aβ (Ma et al., 2017), tau protein (Reeves et al., 2020), and depolarization of AQP4 on the astrocytes(the decrease of AQP4 on the endfeet of paravascular astrocytes) (Back et al., 2017; Wang et al., 2017) may be involved in the occurrence and aggravation of the diseases. Thus, further approaches will be explored to address these disease conditions.
The function of the glymphatic system is partly influenced by the sleep-wake cycle. In the sleep state, the function of the glymphatic system is significantly enhanced than in the awake. CSF inflow of mice in sleep and awake states were compared by in vivo two-photon imaging technology, and results showed that, within 30 minutes after CSF tracer injecting, the tracer inflow of the same mice in the awake state was 95% lower than that in the sleep state (Xie et al., 2013). Moreover, the volume fraction of brain interstitial space in sleeping mice (22∼24%) was significantly higher than that in awake mice (13∼15%) (Xie et al., 2013). The inhibition of CSF inflow during wakefulness may be partly related to noradrenaline (NE) secretion. NE is a neuromodulator that regulates the activity of neuronal and non-neuronal cells (Murtazina et al., 2021). Notably, it can reduce the interstitial space by increasing the cell volume, thus increasing the resistance of the inflow of CSF in the interstitial space (Sherpa et al., 2016; Hablitz et al., 2019). In addition, sleep deficiency can also lead to a decrease in the clearance rate of metabolic proteins in the brain parenchyma, resulting in protein deposition such as Aβ and tau protein (Shokri-Kojori et al., 2018). In short, increasing sleep time or improving sleep quality can enhance CSF inflow and metabolite clearance.
Many neurodegenerative diseases (such as AD, PD, ALS, and so on) often occur in middle and old age, and their pathogenesis is associated with the accumulation of Aβ and tau protein (Jessen et al., 2015). Aβ and tau protein can be cleared from brain parenchyma with CSF outflow in the glymphatic system. The clearance rate of interstitial solutes was compared between young (2–3 months old) and old (18–20 months old) wild-type mice, and results showed that Aβ clearance was reduced by 40%, accompanied by a 27% decrease in the pulsatility of the vascular wall of the arterioles in the old mice cortex, as well as a widespread loss of AQP4 polarization along the penetrating artery (Kress et al., 2014). In addition, aging leads to artery stiffening, which can reduce arterial pulsatility in the cerebral cortex of the elderly, in turn inhibiting CSF inflow from the PVS to the brain parenchyma (Zieman et al., 2005). This indicates that with the growth of age, the functions of the glymphatic system will deteriorate, affecting the clearance of metabolic wastes in the brain.
The function of the glymphatic system is closely related to the anesthetics selected in the experiment, and different anesthetics play a role in promoting or inhibiting. In the awake state, CSF tracers injected into mice cisterna magma hardly enters brain parenchyma (Benveniste et al., 2019). However, after intraperitoneal injection of ketamine/xylazine (KX), CSF rapidly rushed into the brain parenchyma along the periarterial space, with speed comparable to that of naturally sleeping mice, and CSF inflow increased significantly (Xie et al., 2013). Moreso, the effects of different anesthetics were compared. The CSF tracer influx was highest under K/X, followed by isoflurane (ISO) supplemented with dexmedetomidine and pentobarbital. The mice anesthetized with α-chloralose, Avertin, or ISO exhibited low CSF tracer influx. Additionally, it was found that under anesthesia, CSF inflow in the glymphatic system correlates positively with cortical delta power (neuronal network oscillations) in electroencephalogram (EEG) recordings and negatively with heart rate (Hablitz et al., 2019). Additionally, the cortical delta power may contribute to the fluid influx into the brain parenchyma and clearance of waste from the brain, and increased in naturally sleeping animals (Benveniste et al., 2017). Therefore, in the design of CSF flow experiments, we should pay attention to the selection and use of anesthetics to avoid side effects.
Different body positions can also affect the function of the glymphatic system during sleep or anesthesia. The transport efficiency of the glymphatic system in the lateral position of anesthetized rats was higher than that in the supine and prone positions by dynamic-contrast-enhanced MRI and kinetic modeling. The tracer in the brain of prone rats entered and cleared slowly in the glymphatic system (Lee et al., 2015; Muccio et al., 2021). Optical imaging and radiotracer studies also confirmed that the transport of the glymphatic system and the clearance rate of Aβ were faster in the lateral and supine positions (Lee et al., 2015). The changes in body position may affect the function of the cardiovascular and respiratory systems and subsequently impact the pulsation leading to CSF/ISF circulation (Muccio et al., 2021). This mechanism needs to be further explored. These findings suggest that the lateral position during sleep/anesthesia may be advantageous in removing brain metabolic wastes, including Aβ. In the future, we should also pay attention to the placement of the test animals in the experimental design of the glymphatic system.
Alcohol influences the glymphatic system in two different ways. Glymphatic system function was significantly inhibited after mice were exposed to 1.5 g/kg (binge level) ethanol acutely and chronically (Lundgaard et al., 2018). Chronic exposure to 1.5 g/kg ethanol increased GFAP expression (associated with astrocyte reactive phenotype and reduced glymphatic function (Iliff et al., 2014; Kress et al., 2014)) and induced mislocation of the astrocyte-specific AQP4. The function of the glymphatic system increased in mice treated with 0.5 g/kg (low dose) ethanol following acute exposure. After one month of chronic exposure, low doses of chronic ethanol intake were associated with a significant decrease in GFAP expression (Lundgaard et al., 2018). Low-dose ethanol also has a vasodilatory effect, which may promote the interaction of endothelial, smooth muscle cells by activating the production of endothelial-specific nitric oxide synthase (eNOS) and vasodilator nitric oxide, thus promoting the diffusion of metabolic wastes in the central nervous system to the perivascular space (Cheng et al., 2019). Therefore, low-dose intake of alcohol can be potentially beneficial to the function of the glymphatic system. In contrast, long-term excessive intake of alcohol will inhibit the function of the glymphatic system.
Sports can improve cognitive ability, especially in patients with vascular degeneration and neurodegenerative diseases. The glymphatic system function of voluntary wheel running and sedentary mice were compared. CSF inflow continuously increased in voluntary exercise mice during the awake period, mainly concentrated in the hypothalamus and ventral parts of the cortex. However, it also occurred in the middle cerebral artery territory. Voluntary exercise increases CSF flux in a wide range of brain regions in mice, which may be related to the role of exercise in promoting cognition (von Holstein-Rathlou et al., 2018). Another study demonstrated that voluntary wheel running accelerated the protein clearance of the glymphatic system rather than the protein penetration of the BBB (He et al., 2017). It also improved the expression and polarization of AQP4 in astrocytes, thereby protecting mice from synaptic dysfunction and spatial cognitive decline, indicating a possible mechanism of exercise-induced neuroprotection in the aging brain (He et al., 2017).
In short, these influencing factors affect the fluid inflow and outflow of the glymphatic system mainly by regulating the cardiovascular and respiratory systems. When arterial pulsation slows down, or respiration is inhibited, the flow of CSF is inhibited, affecting the clearance of macromolecular metabolic proteins in the brain. The accumulation of metabolic proteins in the brain parenchyma further affects the size of the brain interstitial space, thus increasing the resistance to CSF inflow. However, the specific mechanisms involved in this process still need further exploration. These influencing factors suggest that attention should be paid to the patient’s daily sleep, drinking, and exercise behaviors to prevent brain-related diseases. When designing experiments related to the glymphatic system, attention should be paid to the choice of anesthesia, the position, and the age of experimental animals.
The study of the glymphatic system can be divided into in vivo and ex vivo techniques. Imaging ex vivo is usually performed with light sheet fluorescence microscopy of an observed brain or spinal cord sections from the optically cleared brains of mice receiving CSF tracer injections while under anesthesia (Hablitz et al., 2020; Hauglund et al., 2020). This way, high-resolution (up to 200 nm) 3D imaging of tracer distribution within the cells and in perivascular spaces can be observed (Lohela et al., 2022). Brain or spinal cord fixed coronal sections are usually combined with immunohistochemistry to compare CSF flow with the expression pattern of related proteins. However, in recent years, Panoptic imaging of transparent mice has also been used to analyze the distribution of tracers in the brain (Bèchet et al., 2020). Imaging in vivo is mainly utilized to observe the flow of CSF in the brain by injecting a CSF tracer into the cisterna magna and using two-photon fluorescence imaging (TPI) (Iliff et al., 2013b; Tithof et al., 2019), near-infrared fluorescence (NIRF) (Myllylä et al., 2018), transcranial optical imaging (Plog et al., 2018), magnetic resonance imaging(MRI) (Bai et al., 2022; Li C. et al., 2022) and so on. TPI has high spatial resolution and can observe 240μm below the cortical surface, which is conducive to observing the diffusion of the CSF tracer along the outside of cortical surface arteries and penetrating arterioles (Iliff et al., 2012). However, TPI is invasive to the study object and damages the skull. Its narrow field of vision and shallow imaging area limit the observation of subcortical brain areas, so it is impossible to observe the glymphatic system from the whole brain field (Palczewska et al., 2023). Compared with TPI, transcranial optical imaging does not damage animals’ skull in the experimental process. Transcranial optical imaging can distinguish the region 1∼2 mm below the surface of the skull and can detect the fluorophore 5 ∼ 6mm below the surface of the cortex. It can complete the wide-area imaging of CSF flow in the dorsal perivascular of the cerebral cortex of living mice (Li and Wan, 2021). However, it can only observe the dorsal region of the cerebral cortex and cannot image the ventral area of the cerebral cortex (Sweeney et al., 2019). MRI can dynamically monitor the whole brain in real-time and facilitate studying CSF flow in the glymphatic system. The recently discovered NIRF can perform non-invasive measurement of human brain fluid dynamics, which is conducive to long-term brain monitoring (including sleep time) and can be combined with different magnetic neuroimaging technologies (Liu Y. et al., 2022). It can perform real-time dynamic detection of CSF tracer in vivo, but its low spatial resolution makes it impossible to analyze the fluid flow in perivascular space (Gakuba et al., 2018). MRI (Iliff et al., 2013a; Stanton et al., 2021), single-photon emission computerized tomography (SPECT), and positron emission tomography (PET) coupled with computerized tomography (Benveniste et al., 2021) can be used to obtain brain-wide 4D images of tracer movement in vivo in experimental animals. Notably, these methods are readily translatable to clinical neuroimaging studies. Details on preclinical methods to study the glymphatic system are shown in Table 1.
The concept of the glymphatic system enriches the theory of fluid circulation and waste clearance in the brain. In the past, CSF was thought to be produced from the choroid plexus and circulated in subarachnoid space. However, the glymphatic system provides the hypothesis that CSF enters brain parenchyma and exchanges with ISF dynamically. When the exchange balance is influenced, ISF might be another CSF reserve (Jessen et al., 2015). In the past, researchers thought there was no brain lymphatic system, and the brain’s removal of wastes in the brain was mainly completed through CSF circulation and its addition to blood circulation (Syková and Nicholson, 2008). In fact, the brain parenchyma does not come into direct contact with CSF, and CSF circulation may not be efficient enough due to the limitation of the molecular weight of metabolites. The glymphatic system establishes a bridge between CSF and brain parenchyma, which can help explain brain waste’s clearance pathway.
The discovery of the glymphatic system provides a new direction for understanding brain diseases, which shifts the focus from the changes in the specific structure of the brain to the overall fluid circulation in the brain. A case in point is the critical role of the glymphatic system in understanding the occurrence of brain edema. Traditionally, brain edema is thought to be formed entirely by fluid accumulation in the intravascular compartment (Go, 1984). However, relevant studies of the glymphatic system have found that CSF is the primary source of initial brain edema rather than blood leakage (Mestre et al., 2020). CSF enters the brain parenchyma along the perivascular space within a few minutes after ischemic injury (Mestre et al., 2020). This process is initiated by ischemic spreading depolarization and subsequent vasoconstriction, which expands the perivascular space and doubles the rate of glymphatic inflow. The entry of CSF through the PVS may promote the swelling of astrocytic endfeet and pericyte contraction, which further reduces blood flow and induces the development of an infarction area (Ito et al., 2011; Khennouf et al., 2018). Moreover, the concept of the glymphatic system coincides with the holistic perspective and integrity of the therapeutic effect of traditional Chinese medicine. Traditional Chinese medicine often uses two or more herbs through herbal compatibility to treat diseases, different herb components play different roles in treating brain diseases and finally have a good curative effect when combined. With the knowledge of the glymphatic system, traditional Chinese medicine may better explain multi-ingredient multi-target efficacy. Traditional Chinese medicine formulas may treat diseases by regulating vasoconstriction and relaxation, changing PVS space (Song et al., 2018), and impacting the polar distribution of AQP4 (Yang et al., 2019) and astrocyte swelling.
The proposal of the glymphatic system also provides a new direction for delivering medication. The existence of the BBB and B-CSFB helps to prevent pathogens and toxic substances from entering the brain. However, they also prevent the entry and clearance of medicine for treating brain-related diseases. New ways of medicine delivery have emerged based on the understanding of the glymphatic system. Indocyanine green (ICG) - loaded PLGA nanoparticles were injected near the regional lymph node in the neck of mice. The particles would first converge in the regional deep cervical lymph nodes, then be efficiently transported to the brain through meningeal lymphatic vessels, significantly inhibiting glioma growth in mice and prolonging the survival period of treatment. The uptake of drugs injected with this method in the brain is 44 times higher than that by intravenous injection (Zhao et al., 2020). Drug delivery systems (DDS) are established to conquer the BBB and transport drugs into the brain. However, the elimination route of DDS is unclear. In an experiment, nano-sized DDS in the brain was systematically tracked, and it suggested that it was critically drained by the glymphatic system from the blood vessel basement membrane to periphery circulations (Liu R. et al., 2022). Although their studies are at the beginning stage and drug administration or clearance is affected by many factors (the polarity and safety of drugs are difficult to control), these studies still provide a new direction for medicine delivery.
However, the theory of the glymphatic system is still controversial. Firstly, some researchers believe that the function of the glymphatic system is exaggerated. For instance, several studies have proved that CSF flows along the peripheral spaces of large vessels in convective flow or dispersion and then regulates CSF / ISF exchange in diffusion. This is inconsistent with the fact that CSF / ISF exchange is realized through bulk flow in the glymphatic system (Asgari et al., 2016; Holter et al., 2017; Abbott et al., 2018; Hannocks et al., 2018). Secondly, the role of capillaries in CSF/ISF exchange between arterioles and venules was neglected in glymphatic system theory. Some experiments have proposed that CSF/ISF convection around capillaries occurs in the basal lamina, connects the periarteriolar and the perivenous space, and forms an adequate convective circulation pathway (Hannocks et al., 2018; Pizzo et al., 2018). This structure is more efficient for maintaining neuronal microenvironment homeostasis and promotes substance exchange in CSF / ISF (Wolak and Thorne, 2013). Last but not least, the study on the functional regulation of the glymphatic system is only based on rodent animal models and has not been applied to humans. Thus, we need follow-up experiments to prove that the glymphatic system is also important for the steady-state maintenance of the human brain by supplementing subsequent experiments.
Moreover, there are still some situations that the glymphatic system has not explained. In some diseases like AD or PD, it is not clear whether the malfunction of the glymphatic system occurs first or whether the relevant pathological changes like Aβ or tau-protein deposit happen in the first place. This is crucial to understanding the etiology of diseases. Besides the exchange between CSF/ISF, will the balance be influenced by diseases or altered in some scenarios?
In a word, the proposal of the glymphatic system breaks the traditional understanding that there is no lymphatic system in the brain, complements the theory of fluid circulation and metabolic pathways of brain wastes, and draws a lot of attention to the critical role of fluid circulation in maintaining the homeostasis of the brain environment, which is of great significance. However, there are also many controversies and unsolved problems in the glymphatic system, which requires more researchers to engage in the field for further study, constantly innovate and improve the research paradigm and methods, and make better use of the results of the glymphatic system, to play a more significant role in treating related diseases.
All authors listed have made a substantial, direct, and intellectual contribution to the work, and approved it for publication.
This work was supported by the Funds of the scientific and technological innovation project of the Chinese Academy of Traditional Chinese Medicine CI2021A04614.
The authors thank AiMi Academic Services (www.aimieditor.com) for English language editing and review services.
The authors declare that the research was conducted in the absence of any commercial or financial relationships that could be construed as a potential conflict of interest.
All claims expressed in this article are solely those of the authors and do not necessarily represent those of their affiliated organizations, or those of the publisher, the editors and the reviewers. Any product that may be evaluated in this article, or claim that may be made by its manufacturer, is not guaranteed or endorsed by the publisher.
Abbott, N. J., Pizzo, M. E., Preston, J. E., Janigro, D., and Thorne, R. G. (2018). The role of brain barriers in fluid movement in the CNS: is there a ‘glymphatic’ system? Acta Neuropathol. 135, 387–407. doi: 10.1007/s00401-018-1812-4
Asgari, M., de Zélicourt, D., and Kurtcuoglu, V. (2016). Glymphatic solute transport does not require bulk flow. Sci. Rep. 6:38635. doi: 10.1038/srep38635
Aspelund, A., Antila, S., Proulx, S. T., Karlsen, T. V., Karaman, S., Detmar, M., et al. (2015). A dural lymphatic vascular system that drains brain interstitial fluid and macromolecules. J. Exp. Med. 212, 991–999. doi: 10.1084/jem.20142290
Assentoft, M., Larsen, B. R., and MacAulay, N. (2015). Regulation and function of AQP4 in the central nervous system. Neurochem. Res. 40, 2615–2627. doi: 10.1007/s11064-015-1519-z
Back, D. B., Kwon, K. J., Choi, D. H., Shin, C. Y., Lee, J., Han, S. H., et al. (2017). Chronic cerebral hypoperfusion induces post-stroke dementia following acute ischemic stroke in rats. J. Neuroinflammation 14:216. doi: 10.1186/s12974-017-0992-5
Bai, Y., Yuan, M., Mi, H., Zhang, F., Liu, X., Lu, C., et al. (2022). Hypothermia reduces glymphatic transportation in traumatic edematous brain assessed by intrathecal dynamic contrast-enhanced MRI. Front. Neurol. 13:957055. doi: 10.3389/fneur.2022.957055
Bèchet, N. B., Kylkilahti, T. M., Mattsson, B., Petrasova, M., Shanbhag, N. C., and Lundgaard, I. (2020). Light sheet fluorescence microscopy of optically cleared brains for studying the glymphatic system. J. Cereb. Blood Flow Metab. 40, 1975–1986. doi: 10.1177/0271678X20924954
Benveniste, H., Heerdt, P. M., Fontes, M., Rothman, D. L., and Volkow, N. D. (2019). Glymphatic system function in relation to anesthesia and sleep states. Anesth. Analg. 128, 747–758. doi: 10.1213/ANE.0000000000004069
Benveniste, H., Lee, H., Ding, F., Sun, Q., Al-Bizri, E., Makaryus, R., et al. (2017). Anesthesia with dexmedetomidine and low-dose isoflurane increases solute transport via the glymphatic pathway in rat brain when compared with high-dose isoflurane. Anesthesiology 127, 976–988. doi: 10.1097/ALN.0000000000001888
Benveniste, H., Lee, H., Ozturk, B., Chen, X., Koundal, S., Vaska, P., et al. (2021). Glymphatic cerebrospinal fluid and solute transport quantified by MRI and PET imaging. Neuroscience 474, 63–79. doi: 10.1016/j.neuroscience.2020.11.014
Bicker, J., Alves, G., Fortuna, A., and Falcão, A. (2014). Blood-brain barrier models and their relevance for a successful development of CNS drug delivery systems: a review. Eur. J. Pharm. Biopharm. 87, 409–432. doi: 10.1016/j.ejpb.2014.03.012
Bobela, W., Aebischer, P., and Schneider, B. L. (2015). A lpha-synuclein as a mediator in the Interplay between aging and Parkinson’s disease. Biomolecules 5, 2675–2700. doi: 10.3390/biom5042675
Boentert, M. (2019). Sleep disturbances in patients with amyotrophic lateral sclerosis: current perspectives. Nat. Sci. Sleep 11, 97–111. doi: 10.2147/NSS.S183504
Bosche, B., Mergenthaler, P., Doeppner, T. R., Hescheler, J., and Molcanyi, M. (2020). Complex clearance mechanisms after intraventricular hemorrhage and rt-PA treatment-a review on clinical trials. Transl. Stroke Res. 11, 337–344. doi: 10.1007/s12975-019-00735-6
Burn, L., Gutowski, N., Whatmore, J., Giamas, G., and Pranjol, M. Z. I. (2021). The role of astrocytes in brain metastasis at the interface of circulating tumour cells and the blood brain barrier. Front. Biosci. 26:590–601. doi: 10.52586/4969
Cai, R., Pan, C., Ghasemigharagoz, A., Todorov, M. I., Förstera, B., Zhao, S., et al. (2019). Panoptic imaging of transparent mice reveals whole-body neuronal projections and skull-meninges connections. Nat. Neurosci. 22, 317–327. doi: 10.1038/s41593-018-0301-3
Carotenuto, A., Cacciaguerra, L., Pagani, E., Preziosa, P., Filippi, M., and Rocca, M. A. (2022). Glymphatic system impairment in multiple sclerosis: relation with brain damage and disability. Brain 145, 2785–2795. doi: 10.1093/brain/awab454
Cheng, Y., Liu, X., Ma, X., Garcia, R., Belfield, K., and Haorah, J. (2019). Alcohol promotes waste clearance in the CNS via brain vascular reactivity. Free Radic. Biol. Med. 143, 115–126. doi: 10.1016/j.freeradbiomed.2019.07.029
Choi, S. H., and Tanzi, R. E. (2023). Adult neurogenesis in Alzheimer’s disease. Hippocampus 219, 78–89. doi: 10.1002/hipo.23504
Cserr, H. F., Cooper, D. N., Suri, P. K., and Patlak, C. S. (1981). Efflux of radiolabeled polyethylene glycols and albumin from rat brain. Am. J. Physiol. 240, F319–F328. doi: 10.1152/ajprenal.1981.240.4.F319
Czigler, A., Toth, L., Szarka, N., Szilágyi, K., Kellermayer, Z., Harci, A., et al. (2020). Prostaglandin E(2) a postulated mediator of neurovascular coupling, at low concentrations dilates whereas at higher concentrations constricts human cerebral parenchymal arterioles. Prostaglandins Other Lipid Mediat. 146:106389. doi: 10.1016/j.prostaglandins.2019.106389
de Laurentis, C., Cristaldi, P., Arighi, A., Cavandoli, C., Trezza, A., Sganzerla, E. P., et al. (2021). Role of aquaporins in hydrocephalus: what do we know and where do we stand? a systematic review. J. Neurol. 268, 4078–4094. doi: 10.1007/s00415-020-10122-z
Djukic, M., Lange, P., Erbguth, F., and Nau, R. (2022). Spatial and temporal variation of routine parameters: pitfalls in the cerebrospinal fluid analysis in central nervous system infections. J. Neuroinflammation 19:174. doi: 10.1186/s12974-022-02538-3
Dobson, R., and Giovannoni, G. (2019). Multiple sclerosis - a review. Eur. J. Neurol. 26, 27–40. doi: 10.1111/ene.13819
Drieu, A., Du, S., Storck, S. E., Rustenhoven, J., Papadopoulos, Z., Dykstra, T., et al. (2022). Parenchymal border macrophages regulate the flow dynamics of the cerebrospinal fluid. Nature 611, 585–593. doi: 10.1038/s41586-022-05397-3
Gakuba, C., Gaberel, T., Goursaud, S., Bourges, J., Di Palma, C., Quenault, A., et al. (2018). General anesthesia inhibits the activity of the “Glymphatic system”. Theranostics 8, 710–722. doi: 10.7150/thno.19154
Gao, F., Hu, M., Zhang, J., Hashem, J., and Chen, C. (2022). TDP-43 drives synaptic and cognitive deterioration following traumatic brain injury. Acta Neuropathol. 144, 187–210. doi: 10.1007/s00401-022-02449-w
Giovannelli, I., Higginbottom, A., Kirby, J., Azzouz, M., and Shaw, P. J. (2022). Prospects for gene replacement therapies in amyotrophic lateral sclerosis. Nat. Rev. Neurol. 19, 39–52. doi: 10.1038/s41582-022-00751-5
Go, K. G. (1984). Pathophysiological aspects of brain edema. Clin. Neurol. Neurosurg. 86, 77–80. doi: 10.1016/0303-8467(84)90069-6
Gouveia-Freitas, K., and Bastos-Leite, A. J. (2021). Perivascular spaces and brain waste clearance systems: relevance for neurodegenerative and cerebrovascular pathology. Neuroradiology 63, 1581–1597. doi: 10.1007/s00234-021-02718-7
Granberg, T., Moridi, T., Brand, J. S., Neumann, S., Hlavica, M., Piehl, F., et al. (2020). Enlarged perivascular spaces in multiple sclerosis on magnetic resonance imaging: a systematic review and meta-analysis. J. Neurol. 267, 3199–3212. doi: 10.1007/s00415-020-09971-5
Hablitz, L. M., Plá, V., Giannetto, M., Vinitsky, H. S., Stæger, F. F., Metcalfe, T., et al. (2020). Circadian control of brain glymphatic and lymphatic fluid flow. Nat. Commun. 11:4411. doi: 10.1038/s41467-020-18115-2
Hablitz, L. M., Vinitsky, H. S., Sun, Q., Stæger, F. F., Sigurdsson, B., Mortensen, K. N., et al. (2019). Increased glymphatic influx is correlated with high EEG delta power and low heart rate in mice under anesthesia. Sci. Adv. 5:eaav5447. doi: 10.1126/sciadv.aav5447
Hannocks, M. J., Pizzo, M. E., Huppert, J., Deshpande, T., Abbott, N. J., Thorne, R. G., et al. (2018). Molecular characterization of perivascular drainage pathways in the murine brain. J. Cereb. Blood Flow Metab. 38, 669–686. doi: 10.1177/0271678X17749689
Hauglund, N. L., Kusk, P., Kornum, B. R., and Nedergaard, M. (2020). Meningeal lymphangiogenesis and enhanced glymphatic activity in mice with chronically implanted EEG electrodes. J. Neurosci. 40, 2371–2380. doi: 10.1523/JNEUROSCI.2223-19.2020
He, X. F., Liu, D. X., Zhang, Q., Liang, F. Y., Dai, G. Y., Zeng, J. S., et al. (2017). Voluntary exercise promotes glymphatic clearance of amyloid beta and reduces the activation of astrocytes and microglia in aged mice. Front. Mol. Neurosci. 10:144. doi: 10.3389/fnmol.2017.00144
Hicks, A. J., Ponsford, J. L., Spitz, G., Dore, V., Krishnadas, N., Roberts, C., et al. (2022). β-Amyloid and tau imaging in chronic traumatic brain injury: a cross-sectional study. Neurology 99, e1131–e1141. doi: 10.1212/WNL.0000000000200857
Holter, K. E., Kehlet, B., Devor, A., Sejnowski, T. J., Dale, A. M., Omholt, S. W., et al. (2017). Interstitial solute transport in 3D reconstructed neuropil occurs by diffusion rather than bulk flow. Proc. Natl. Acad. Sci. U S A. 114, 9894–9899. doi: 10.1073/pnas.1706942114
Iliff, J. J., Chen, M. J., Plog, B. A., Zeppenfeld, D. M., Soltero, M., Yang, L., et al. (2014). Impairment of glymphatic pathway function promotes tau pathology after traumatic brain injury. J. Neurosci. 34, 16180–16193. doi: 10.1523/JNEUROSCI.3020-14.2014
Iliff, J. J., Lee, H., Yu, M., Feng, T., Logan, J., Nedergaard, M., et al. (2013a). Brain-wide pathway for waste clearance captured by contrast-enhanced MRI. J. Clin. Invest 123, 1299–1309. doi: 10.1172/JCI67677
Iliff, J. J., Wang, M., Zeppenfeld, D. M., Venkataraman, A., Plog, B. A., Liao, Y., et al. (2013b). Cerebral arterial pulsation drives paravascular CSF-interstitial fluid exchange in the murine brain. J. Neurosci. 33, 18190–18199. doi: 10.1523/JNEUROSCI.1592-13.2013
Iliff, J. J., Wang, M., Liao, Y., Plogg, B. A., Peng, W., Gundersen, G. A., et al. (2012). A paravascular pathway facilitates CSF flow through the brain parenchyma and the clearance of interstitial solutes, including amyloid β. Sci. Transl. Med. 4, 147ra111. doi: 10.1126/scitranslmed.3003748
Ito, U., Hakamata, Y., Kawakami, E., and Oyanagi, K. (2011). Temporary [corrected] cerebral ischemia results in swollen astrocytic end-feet that compress microvessels and lead to delayed [corrected] focal cortical infarction. J. Cereb. Blood Flow Metab. 31, 328–338. doi: 10.1038/jcbfm.2010.97
Jessen, N. A., Munk, A. S., Lundgaard, I., and Nedergaard, M. (2015). The glymphatic system: a beginner’s guide. Neurochem. Res. 40, 2583–2599. doi: 10.1007/s11064-015-1581-6
Jiang, Q., Zhang, L., Ding, G., Davoodi-Bojd, E., Li, Q., Li, L., et al. (2017). Impairment of the glymphatic system after diabetes. J. Cereb. Blood Flow Metab. 37, 1326–1337. doi: 10.1177/0271678X16654702
Jullienne, A., Obenaus, A., Ichkova, A., Savona-Baron, C., Pearce, W. J., and Badaut, J. (2016). Chronic cerebrovascular dysfunction after traumatic brain injury. J. Neurosci. Res. 94, 609–622. doi: 10.1002/jnr.23732
Karussis, D. (2014). The diagnosis of multiple sclerosis and the various related demyelinating syndromes: a critical review. J. Autoimmun. 48-49, 134–142. doi: 10.1016/j.jaut.2014.01.022
Kassem, N. A., Deane, R., Segal, M. B., and Preston, J. E. (2006). Role of transthyretin in thyroxine transfer from cerebrospinal fluid to brain and choroid plexus. Am. J. Physiol. Regul. Integr. Comp. Physiol. 291, R1310–R1315. doi: 10.1152/ajpregu.00789.2005
Khennouf, L., Gesslein, B., Brazhe, A., Octeau, J. C., Kutuzov, N., Khakh, B. S., et al. (2018). Active role of capillary pericytes during stimulation-induced activity and spreading depolarization. Brain 141, 2032–2046. doi: 10.1093/brain/awy143
Kress, B. T., Iliff, J. J., Xia, M., Wang, M., Wei, H. S., Zeppenfeld, D., et al. (2014). Impairment of paravascular clearance pathways in the aging brain. Ann. Neurol. 76, 845–861. doi: 10.1002/ana.24271
Lee, H., Xie, L., Yu, M., Kang, H., Feng, T., Deane, R., et al. (2015). The effect of body posture on brain glymphatic transport. J. Neurosci. 35, 11034–11044. doi: 10.1523/JNEUROSCI.1625-15.2015
Li, C., Lin, L., Sun, C., Hao, X., Yin, L., Zhang, X., et al. (2022). Glymphatic system in the thalamus, secondary degeneration area was severely impaired at 2nd week after transient occlusion of the middle cerebral artery in rats. Front. Neurosci. 16:997743. doi: 10.3389/fnins.2022.997743
Li, Z., Wang, S., Liu, S., Gong, X., Wang, Y., Wu, D., et al. (2022). Synergistic impact of diabetes and cognitive impairment on all-cause and cause-specific mortality in Chinese older adults: a prospective population-based cohort study. Front. Endocrinol. 13:997260. doi: 10.3389/fendo.2022.997260
Li, J., and Wan, C. (2021). Non-invasive detection of intracranial pressure related to the optic nerve. Quant. Imaging Med. Surg. 11, 2823–2836. doi: 10.21037/qims-20-1188
Li, X., and Chen, G. (2022). CNS-peripheral immune interactions in hemorrhagic stroke. J. Cereb. Blood Flow Metab. 43, 185–197. doi: 10.1177/0271678X221145089
Liu, R., Jia, W., Wang, Y., Hu, C., Yu, W., Huang, Y., et al. (2022). Glymphatic system and subsidiary pathways drive nanoparticles away from the brain. Research 2022:9847612. doi: 10.34133/2022/9847612
Liu, Y., Zhuang, D., Wang, J., Huang, H., Li, R., Wu, C., et al. (2022). Recent advances in small molecular near-infrared fluorescence probes for a targeted diagnosis of the Alzheimer disease. Analyst 147, 4701–4723. doi: 10.1039/D2AN01327D
Lohela, T. J., Lilius, T. O., and Nedergaard, M. (2022). The glymphatic system: implications for drugs for central nervous system diseases. Nat. Rev. Drug Discov. 21, 763–779. doi: 10.1038/s41573-022-00500-9
Louveau, A., Smirnov, I., Keyes, T. J., Eccles, J. D., Rouhani, S. J., Peske, J. D., et al. (2015). Structural and functional features of central nervous system lymphatic vessels. Nature 523, 337–341. doi: 10.1038/nature14432
Lu, H. J., and Gao, Y. J. (2022). Astrocytes in chronic pain: cellular and molecular mechanisms. Neurosci. Bull. 39, 425–439. doi: 10.1007/s12264-022-00961-3
Lundgaard, I., Li, B., Xie, L., Kang, H., Sanggaard, S., Haswell, J. D., et al. (2015). Direct neuronal glucose uptake heralds activity-dependent increases in cerebral metabolism. Nat. Commun. 6:6807. doi: 10.1038/ncomms7807
Lundgaard, I., Lu, M. L., Yang, E., Peng, W., Mestre, H., Hitomi, E., et al. (2017). Glymphatic clearance controls state-dependent changes in brain lactate concentration. J. Cereb. Blood Flow Metab. 37, 2112–2124. doi: 10.1177/0271678X16661202
Lundgaard, I., Wang, W., Eberhardt, A., Vinitsky, H. S., Reeves, B. C., Peng, S., et al. (2018). Beneficial effects of low alcohol exposure, but adverse effects of high alcohol intake on glymphatic function. Sci. Rep. 8:2246. doi: 10.1038/s41598-018-20424-y
Lynch, M., Pham, W., Sinclair, B., O’Brien, T. J., Law, M., and Vivash, L. (2022). Perivascular spaces as a potential biomarker of Alzheimer’s disease. Front. Neurosci. 16:1021131. doi: 10.3389/fnins.2022.1021131
Ma, Q., Ineichen, B. V., Detmar, M., and Proulx, S. T. (2017). Outflow of cerebrospinal fluid is predominantly through lymphatic vessels and is reduced in aged mice. Nat. Commun. 8:1434. doi: 10.1038/s41467-017-01484-6
MacVicar, B. A., and Newman, E. A. (2015). Astrocyte regulation of blood flow in the brain. Cold Spring Harb. Perspect. Biol. 7:a020388. doi: 10.1101/cshperspect.a020388
Mamtilahun, M., Tang, G., Zhang, Z., Wang, Y., Tang, Y., and Yang, G. Y. (2019). Targeting water in the brain: role of Aquaporin-4 in ischemic brain edema. Curr. Drug Targets 20, 748–755. doi: 10.2174/1389450120666190214115309
Markowicz-Piasecka, M., Darłak, P., Markiewicz, A., Sikora, J., Kumar Adla, S., Bagina, S., et al. (2022). Current approaches to facilitate improved drug delivery to the central nervous system. Eur. J. Pharm. Biopharm. 181, 249–262. doi: 10.1016/j.ejpb.2022.11.003
Mestre, H., Du, T., Sweeney, A. M., Liu, G., Samson, A. J., Peng, W., et al. (2020). Cerebrospinal fluid influx drives acute ischemic tissue swelling. Science 367:eaax7171. doi: 10.1126/science.aax7171
Mestre, H., Hablitz, L. M., Xavier, A. L., Feng, W., Zou, W., Pu, T., et al. (2018). Aquaporin-4-dependent glymphatic solute transport in the rodent brain. Elife 7:e40070. doi: 10.7554/eLife.40070
Mishra, P. S., Dhull, D. K., Nalini, A., Vijayalakshmi, K., Sathyaprabha, T. N., Alladi, P. A., et al. (2016). Astroglia acquires a toxic neuroinflammatory role in response to the cerebrospinal fluid from amyotrophic lateral sclerosis patients. J. Neuroinflammation 13:212. doi: 10.1186/s12974-016-0698-0
Mogensen, F. L., Delle, C., and Nedergaard, M. (2021). The glymphatic system (En)during inflammation. Int. J. Mol. Sci. 22:7491. doi: 10.3390/ijms22147491
Muccio, M., Chu, D., Minkoff, L., Kulkarni, N., Damadian, B., Damadian, R. V., et al. (2021). Upright versus supine MRI: effects of body position on craniocervical CSF flow. Fluids Barriers CNS 18:61. doi: 10.1186/s12987-021-00296-7
Murtazina, A. R., Bondarenko, N. S., Pronina, T. S., Chandran, K. I., Bogdanov, V. V., Dilmukhametova, L. K., et al. (2021). A comparative analysis of CSF and the blood levels of monoamines as neurohormones in rats during ontogenesis. Acta Naturae 13, 89–97. doi: 10.32607/actanaturae.11516
Myllylä, T., Harju, M., Korhonen, V., Bykov, A., Kiviniemi, V., and Meglinski, I. (2018). Assessment of the dynamics of human glymphatic system by near-infrared spectroscopy. J. Biophotonics 11:e201700123. doi: 10.1002/jbio.201700123
Nakada, T., and Kwee, I. L. (2019). Fluid dynamics inside the brain barrier: current concept of interstitial flow, glymphatic flow, and cerebrospinal fluid circulation in the brain. Neuroscientist 25, 155–166. doi: 10.1177/1073858418775027
Nedergaard, M., and Goldman, S. A. (2016). Brain drain. Sci. Am. 314, 44–49. doi: 10.1038/scientificamerican0316-44
Oresković, D., and Klarica, M. (2010). The formation of cerebrospinal fluid: nearly a hundred years of interpretations and misinterpretations. Brain Res. Rev. 64, 241–262. doi: 10.1016/j.brainresrev.2010.04.006
Palczewska, G., Wojtkowski, M., and Palczewski, K. (2023). From mouse to human: Accessing the biochemistry of vision in vivo by two-photon excitation. Prog. Retin. Eye Res. 93:101170. doi: 10.1016/j.preteyeres.2023.101170
Peng, S., Liu, J., Liang, C., Yang, L., and Wang, G. (2023). Aquaporin-4 in glymphatic system, and its implication for central nervous system disorders. Neurobiol. Dis. 179:106035. doi: 10.1016/j.nbd.2023.106035
Pi, C., Tang, W., Li, Z., Liu, Y., Jing, Q., Dai, W., et al. (2022). Cortical pain induced by optogenetic cortical spreading depression: from whole brain activity mapping. Mol. Brain 15:99. doi: 10.1186/s13041-022-00985-w
Pizzo, M. E., Wolak, D. J., Kumar, N. N., Brunette, E., Brunnquell, C. L., Hannocks, M. J., et al. (2018). Intrathecal antibody distribution in the rat brain: surface diffusion, perivascular transport and osmotic enhancement of delivery. J. Physiol. 596, 445–475. doi: 10.1113/JP275105
Plog, B. A., Mestre, H., Olveda, G. E., Sweeney, A. M., Kenney, H. M., Cove, A., et al. (2018). Transcranial optical imaging reveals a pathway for optimizing the delivery of immunotherapeutics to the brain. JCI Insight 3:e120922. doi: 10.1172/jci.insight.120922
Pollay, M. (2010). The function and structure of the cerebrospinal fluid outflow system. Cerebrospinal Fluid Res. 7:9. doi: 10.1186/1743-8454-7-9
Pu, T., Zou, W., Feng, W., Zhang, Y., Wang, L., Wang, H., et al. (2019). Persistent malfunction of glymphatic and meningeal lymphatic drainage in a mouse model of subarachnoid hemorrhage. Exp. Neurobiol. 28, 104–118. doi: 10.5607/en.2019.28.1.104
Rangroo Thrane, V., Thrane, A. S., Plog, B. A., Thiyagarajan, M., Iliff, J. J., Deane, R., et al. (2013). Paravascular microcirculation facilitates rapid lipid transport and astrocyte signaling in the brain. Sci. Rep. 3:2582 doi: 10.1038/srep02582
Rasmussen, M. K., Mestre, H., and Nedergaard, M. (2018). The glymphatic pathway in neurological disorders. Lancet Neurol. 17, 1016–1024. doi: 10.1016/S1474-4422(18)30318-1
Rasmussen, M. K., Mestre, H., and Nedergaard, M. (2022). Fluid transport in the brain. Physiol. Rev. 102, 1025–1151. doi: 10.1152/physrev.00031.2020
Reeves, B. C., Karimy, J. K., Kundishora, A. J., Mestre, H., Cerci, H. M., Matouk, C., et al. (2020). Glymphatic system impairment in Alzheimer’s disease and idiopathic normal pressure hydrocephalus. Trends Mol. Med. 26, 285–295. doi: 10.1016/j.molmed.2019.11.008
Rohr, S. O., Greiner, T., Joost, S., Amor, S., Valk, P. V., Schmitz, C., et al. (2020). Aquaporin-4 expression during toxic and autoimmune demyelination. Cells 9:2187. doi: 10.3390/cells9102187
Saunders, N. R., Dziegielewska, K. M., Fame, R. M., Lehtinen, M. K., and Liddelow, S. A. (2023). The choroid plexus: a missing link in our understanding of brain development and function. Physiol. Rev. 103, 919–956. doi: 10.1152/physrev.00060.2021
Schain, A. J., Melo-Carrillo, A., Strassman, A. M., and Burstein, R. (2017). Cortical spreading depression closes paravascular space and impairs glymphatic flow: implications for migraine headache. J. Neurosci. 37, 2904–2915. doi: 10.1523/JNEUROSCI.3390-16.2017
Scollato, A., Lolli, F., Lastrucci, G., Repice, A., De Santis, G., Nicoletti, C., et al. (2022). Case report: a multiple sclerosis patient with imaging features of glymphatic failure benefitted from CSF flow shunting. Front. Neurosci. 16:863117. doi: 10.3389/fnins.2022.863117
Scott-Massey, A., Boag, M. K., Magnier, A., Bispo, D., Khoo, T. K., and Pountney, D. L. (2022). Glymphatic system dysfunction and sleep disturbance may contribute to the pathogenesis and progression of parkinson’s disease. Int. J. Mol. Sci. 23:12928. doi: 10.3390/ijms232112928
Sherpa, A. D., Xiao, F., Joseph, N., Aoki, C., and Hrabetova, S. (2016). Activation of β-adrenergic receptors in rat visual cortex expands astrocytic processes and reduces extracellular space volume. Synapse 70, 307–316. doi: 10.1002/syn.21908
Shokri-Kojori, E., Wang, G. J., Wiers, C. E., Demiral, S. B., Guo, M., Kim, S. W., et al. (2018). β-Amyloid accumulation in the human brain after one night of sleep deprivation. Proc. Natl. Acad. Sci. U S A. 115, 4483–4488. doi: 10.1073/pnas.1721694115
Sivandzade, F., Alqahtani, F., and Cucullo, L. (2020). Traumatic brain injury and blood-brain barrier (BBB): underlying pathophysiological mechanisms and the influence of cigarette smoking as a premorbid condition. Int. J. Mol. Sci. 21:2721. doi: 10.3390/ijms21082721
Song, H., Chen, X., Yu, Y., and Zhang, L. (2018). Xingnao Kaiqiao acupuncture combined with Angong Niuhuang Wan for a patient under persistent vegetative state: a case report. Front. Med. 12, 334–339. doi: 10.1007/s11684-017-0539-2
Stanton, E. H., Persson, N., Gomolka, R. S., Lilius, T., Sigurðsson, B., Lee, H., et al. (2021). Mapping of CSF transport using high spatiotemporal resolution dynamic contrast-enhanced MRI in mice: effect of anesthesia. Magn. Reson. Med. 85, 3326–3342. doi: 10.1002/mrm.28645
Süssmuth, S. D., Sperfeld, A. D., Hinz, A., Brettschneider, J., Endruhn, S., Ludolph, A. C., et al. (2010). CSF glial markers correlate with survival in amyotrophic lateral sclerosis. Neurology 74, 982–987. doi: 10.1212/WNL.0b013e3181d5dc3b
Sweeney, A. M., Plá, V., Du, T., Liu, G., Sun, Q., Peng, S., et al. (2019). In vivo imaging of cerebrospinal fluid transport through the intact mouse skull using fluorescence macroscopy. J. Vis. Exp. 149:10.3791/59774. doi: 10.3791/59774
Syková, E., and Nicholson, C. (2008). Diffusion in brain extracellular space. Physiol. Rev. 88, 1277–1340. doi: 10.1152/physrev.00027.2007
Szczygielski, J., Kopańska, M., Wysocka, A., and Oertel, J. (2021). Cerebral microcirculation, perivascular unit, and glymphatic system: role of Aquaporin-4 as the gatekeeper for water homeostasis. Front. Neurol. 12:767470. doi: 10.3389/fneur.2021.767470
Thompson, D., Brissette, C. A., and Watt, J. A. (2022). The choroid plexus and its role in the pathogenesis of neurological infections. Fluids Barriers CNS 19:75. doi: 10.1186/s12987-022-00372-6
Thrane, A. S., Rangroo Thrane, V., and Nedergaard, M. (2014). Drowning stars: reassessing the role of astrocytes in brain edema. Trends Neurosci. 37, 620–628. doi: 10.1016/j.tins.2014.08.010
Tithof, J., Kelley, D. H., Mestre, H., Nedergaard, M., and Thomas, J. H. (2019). Hydraulic resistance of periarterial spaces in the brain. Fluids Barriers CNS 16:19. doi: 10.1186/s12987-019-0140-y
Toriello, M., González-Quintanilla, V., Pérez-Pereda, S., Fontanillas, N., and Pascual, J. (2021). The potential role of the glymphatic system in headache disorders. Pain Med. 22, 3098–3100. doi: 10.1093/pm/pnab137
Uchida, K. (2022). Waste clearance in the brain and neuroinflammation: a novel perspective on biomarker and drug target discovery in Alzheimer’s disease. Cells 11:919. doi: 10.3390/cells11050919
Verghese, J. P., Terry, A., de Natale, E. R., and Politis, M. (2022). Research Evidence of the Role of the Glymphatic System and Its Potential Pharmacological Modulation in Neurodegenerative Diseases. J. Clin. Med. 11:6964. doi: 10.3390/jcm11236964
von Holstein-Rathlou, S., Petersen, N. C., and Nedergaard, M. (2018). Voluntary running enhances glymphatic influx in awake behaving, young mice. Neurosci. Lett. 662, 253–258. doi: 10.1016/j.neulet.2017.10.035
Wang, M., Ding, F., Deng, S., Guo, X., Wang, W., Iliff, J. J., et al. (2017). Focal solute trapping and global glymphatic pathway impairment in a murine model of multiple microinfarcts. J. Neurosci. 37, 2870–2877. doi: 10.1523/JNEUROSCI.2112-16.2017
Wolak, D. J., and Thorne, R. G. (2013). Diffusion of macromolecules in the brain: implications for drug delivery. Mol. Pharm. 10, 1492–1504. doi: 10.1021/mp300495e
Xie, L., Kang, H., Xu, Q., Chen, M. J., Liao, Y., Thiyagarajan, M., et al. (2013). Sleep drives metabolite clearance from the adult brain. Science 342, 373–377. doi: 10.1126/science.1241224
Xu, Q., Bernardo, A., Walker, D., Kanegawa, T., Mahley, R. W., and Huang, Y. (2006). Profile and regulation of apolipoprotein E (ApoE) expression in the CNS in mice with targeting of green fluorescent protein gene to the ApoE locus. J. Neurosci. 26, 4985–4994. doi: 10.1523/JNEUROSCI.5476-05.2006
Xu, Y., Li, K., Zhao, Y., Zhou, L., Liu, Y., and Zhao, J. (2023). Role of ferroptosis in stroke. Cell Mol. Neurobiol. 43, 205–222. doi: 10.1007/s10571-022-01196-6
Yang, W. T., Wang, Y., Shi, Y. H., Fu, H., Xu, Z., Xu, Q. Q., et al. (2019). Herbal compatibility of ginseng and rhubarb exerts synergistic neuroprotection in cerebral ischemia/reperfusion injury of rats. Front. Physiol. 10:1174. doi: 10.3389/fphys.2019.01174
Yao, D., Zhang, R., Xie, M., Ding, F., Wang, M., and Wang, W. (2022). Updated understanding of the glial-vascular unit in central nervous system disorders. Neurosci Bull. 39, 503–518. doi: 10.1007/s12264-022-00977-9
Yao, X. Y., Gao, M. C., Bai, S. W., Xie, L., Song, Y. Y., Ding, J., et al. (2022). Enlarged perivascular spaces, neuroinflammation and neurological dysfunction in NMOSD patients. Front. Immunol. 13:966781. doi: 10.3389/fimmu.2022.966781
Zhang, E. T., Inman, C. B., and Weller, R. O. (1990). Interrelationships of the pia mater and the perivascular (Virchow-Robin) spaces in the human cerebrum. J. Anat. 170, 111–123.
Zhang, S., Gan, L., Cao, F., Wang, H., Gong, P., Ma, C., et al. (2022). The barrier and interface mechanisms of the brain barrier, and brain drug delivery. Brain Res. Bull. 190, 69–83. doi: 10.1016/j.brainresbull.2022.09.017
Zhao, J., Yang, J., Ding, L., Wang, F., and Lin, L. (2022). A review of the pathogenesis and chinese medicine intervention of Alzheimer’s disease. J. Integr. Neurosci. 22:2. doi: 10.31083/j.jin2201002
Zhao, Z., He, J., Chen, Y., Wang, Y., Wang, C., Tan, C., et al. (2022). The pathogenesis of idiopathic normal pressure hydrocephalus based on the understanding of AQP1 and AQP4. Front. Mol. Neurosci. 15:952036. doi: 10.3389/fnmol.2022.952036
Zhao, P., Le, Z., Liu, L., and Chen, Y. (2020). Therapeutic delivery to the brain via the lymphatic vasculature. Nano Lett. 20, 5415–5420. doi: 10.1021/acs.nanolett.0c01806
Zhou, Z., Zhan, J., Cai, Q., Xu, F., Chai, R., Lam, K., et al. (2022). The water transport system in Astrocytes-aquaporins. Cells 11:2564. doi: 10.3390/cells11162564
Zieman, S. J., Melenovsky, V., and Kass, D. A. (2005). Mechanisms, pathophysiology, and therapy of arterial stiffness. Arterioscler. Thromb. Vasc. Biol. 25, 932–943. doi: 10.1161/01.ATV.0000160548.78317.29
Keywords: the glymphatic system, cerebrospinal fluid, perivascular spaces, astrocytes, AQP-4
Citation: Ding Z, Fan X, Zhang Y, Yao M, Wang G, Dong Y, Liu J and Song W (2023) The glymphatic system: a new perspective on brain diseases. Front. Aging Neurosci. 15:1179988. doi: 10.3389/fnagi.2023.1179988
Received: 05 March 2023; Accepted: 29 May 2023;
Published: 15 June 2023.
Edited by:
Alexey Moskalev, Komi Scientific Center (RAS), RussiaReviewed by:
Claudio Nicoletti, University of Siena, ItalyCopyright © 2023 Ding, Fan, Zhang, Yao, Wang, Dong, Liu and Song. This is an open-access article distributed under the terms of the Creative Commons Attribution License (CC BY). The use, distribution or reproduction in other forums is permitted, provided the original author(s) and the copyright owner(s) are credited and that the original publication in this journal is cited, in accordance with accepted academic practice. No use, distribution or reproduction is permitted which does not comply with these terms.
*Correspondence: Wenting Song, d2VudGluZ19zb25nMUAxNjMuY29t
Disclaimer: All claims expressed in this article are solely those of the authors and do not necessarily represent those of their affiliated organizations, or those of the publisher, the editors and the reviewers. Any product that may be evaluated in this article or claim that may be made by its manufacturer is not guaranteed or endorsed by the publisher.
Research integrity at Frontiers
Learn more about the work of our research integrity team to safeguard the quality of each article we publish.