- 1Department of Respiratory Medicine, NUTRIM–School of Nutrition and Translational Research in Metabolism, Maastricht University Medical Center+, Maastricht, Netherlands
- 2Department of Precision Medicine, GROW–School for Oncology and Developmental Biology, Maastricht University Medical Center+, Maastricht, Netherlands
- 3Danone Nutricia Research, Utrecht, Netherlands
Cancer cachexia is a complex metabolic syndrome characterized by involuntary skeletal muscle loss and is associated with poor clinical outcome, decreased survival and negatively influences cancer therapy. No curative treatments are available for cancer cachexia, but nutritional intervention is recommended as a cornerstone of multimodal therapy. Optimal nutritional care is pivotal in the treatment of cancer cachexia, and the effects of nutrients may extend beyond provision of adequate energy uptake, targeting different mechanisms or metabolic pathways that are affected or deregulated by cachexia. The evidence to support this notion derived from nutritional intervention studies in experimental models of cancer cachexia is systematically discussed in this review. Moreover, experimental variables and readout parameters to determine skeletal muscle wasting and cachexia are methodologically evaluated to allow critical comparison of similar studies. Single- and multinutrient intervention studies including qualitative modulation of dietary protein, dietary fat, and supplementation with specific nutrients, such as carnitine and creatine, were reviewed for their efficacy to counteract muscle mass loss and its underlying mechanisms in experimental cancer cachexia. Numerous studies showed favorable effects on impaired protein turnover and related metabolic abnormalities of nutritional supplementation in parallel with a beneficial impact on cancer-induced muscle wasting. The combination of high quality nutrients in a multitargeted, multinutrient approach appears specifically promising, preferentially as a multimodal intervention, although more studies investigating the optimal quantity and combination of nutrients are needed. During the review process, a wide variation in timing, duration, dosing, and route of supplementation, as well as a wide variation in animal models were observed. Better standardization in dietary design, and the development of experimental models that better recapitulate the etiology of human cachexia, will further facilitate successful translation of experimentally-based multinutrient, multimodal interventions into clinical practice.
Introduction
Cancer cachexia is a debilitating syndrome characterized by involuntary weight loss that not only affects adipose tissue but also leads to wasting and weakness of skeletal muscle. Cachexia is associated with an abnormal energy and substrate metabolism that cannot be reversed by conventional nutritional support (1). This differentiates the syndrome from (semi)starvation during which energy expenditure and protein turnover is reduced. Cachexia is highly prevalent in advanced cancers. One third of all patients with cancer loses more than 5% of their original body weight, which is a common screening criterion for cancer cachexia (1, 2). In particular, about 70% of gastric cancer patients, 80% of lung cancer patients, and 90% of liver and pancreatic cancer patients are at risk of developing cachexia (2). Cachexia is associated with poor clinical outcome, decreased survival (3) and negatively influences tumor therapy, as is illustrated by increased postoperative mortality and decreased response to radiation-, chemo-, and immunotherapy (4–6). Muscle wasting is an important contributing factor to muscle weakness in cachexia, which adversely affects performance status, quality of life and hospitalization risk of cancer patients (7).
Many factors contribute to cancer-induced weight loss including anorexia, altered protein and energy metabolism, and chronic inflammation. The anorexia associated with cancer cachexia is likely caused by the activity of pro-inflammatory cytokines, such as tumor necrosis factor-α (TNF-α), interleukin-1 (IL-1), interleukin-6 (IL-6) and growth differentiation factor 15, that are produced either by the tumor or by the host in response to the tumor, which interfere with appetite signals within the anterior hypothalamus (8, 9). Some of these cytokines may also increase the metabolic rate in cancer cachexia (10). In addition to hypermetabolism, one of the major metabolic changes contributing to cancer cachexia is the alteration in protein metabolism. These changes are characterized by a net protein breakdown and an increased oxidation of branched-chain amino acids (BCAAs), especially in the skeletal muscle, to support energy production (gluconeogenesis) and to supply amino acids for elevated hepatic synthesis of acute-phase proteins (11). The breakdown of the host protein is partly stimulated by tumor-secreted factors, including proteolysis-inducing factor and lipid-mobilizing factor, and host-derived inflammatory cytokines such as TNF- α and IL-6 (12).
Undernutrition is a common problem in patients with cancer cachexia and can be a consequence of both reduced dietary intake, poor dietary quality and hypermetabolism (13). The reduced food intake is thought to be explained by tumor-induced symptoms, such as mechanical interference with nutritional intake or absorption, treatment-related side effects, including mucositis, nausea and vomiting, and altered taste. Undernutrition not only affects the macronutrients that supply energy but also the micronutrients that are essential cofactors in metabolism and vital to preserve body mass Therefore, undernutrition is one of the main reasons that patients with cancer cachexia have an inadequate micronutrient status (14). Accordingly, inadequate micronutrient intake negatively influences the course of the disease and increases the risk of complications.
For a long time, undernutrition and cachexia remained neglected medical conditions for which a clear therapeutic strategy was lacking. A multidisciplinary approach is currently considered the best option to tackle cancer cachexia, in which nutritional intervention is recommended as an integral part of the multimodal therapy (15). Adequate nutritional care is pivotal to provide the essential building blocks to maintain and rebuild tissue in cachectic patients. Furthermore, nutrition is also crucial to supply energy and micronutrients that are vital to fuel and catalyze metabolic processes. In addition to the nourishing of the cachectic patients, various nutrients have been implied in the regulation and normalization of metabolic processes underlying the wasting in cachectic patients. Most of the metabolically active nutrients have different functions in the various intertwined processes that may be deregulated in cachexia. For nutrients and their metabolites that are rate limiting in key metabolic pathways, preventing any deficiencies will help preserve or restore metabolic homeostasis. Furthermore, an approach where multiple nutrients are combined in a well-balanced multi-nutrient intervention is likely most appropriate, as it is well-recognized that nutritional modulation includes alteration of intermediates, precursors, catalyzers and regulators of many metabolic pathways. Therefore, nutritional interventions have the potential to simultaneously affect multiple targets that may be involved in the cancer cachexia process including energy intake, anorexia, inflammation and anabolic signaling (Figure 1). However, identification of an optimal nutritional intervention requires systematic experimental evaluation of the specific as well as combined effects of individual components.
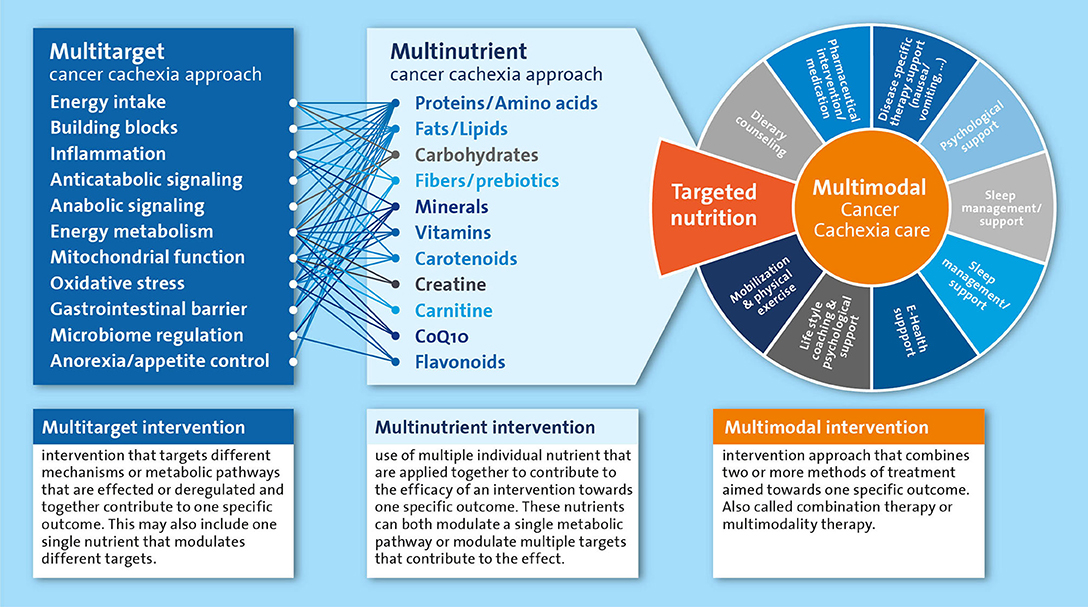
Figure 1. Simplified representation of the multitarget and multinutrient approach and their mutual interactions as part of the multimodal cancer cachexia care. All components listed under “multinutrient cancer cachexia approach” are systematically reviewed as single nutrients or as part of multinutrient approaches in the text.
Nutritional support in patients with cancer cachexia aims to counteract the negative energy balance as well as the net protein breakdown, without stimulating tumor growth or negatively influencing anti-tumor therapy. To establish a net positive protein balance, specific nutrients mitigating catabolic and stimulating anabolic signals should be considered. To create an anabolic environment, adequate caloric intake, and nutrient composition (e.g., quantity and quality of nutrients) is instrumental, because without sufficient nutrient availability, anabolic triggers will not lead to muscle maintenance or an increase in muscle mass. Several nutritional agents have been proposed to tackle cancer cachexia, however, clear evidence of their efficacy is limited. A better understanding of specific nutrients' contribution to muscle anabolism in these patients can lead to the development of specialized nutritional products focusing on halting muscle mass loss in cancer cachexia.
Experimental animal models are used extensively to study mechanisms underlying cancer cachexia and evaluate potential treatments. In this narrative review, based on a systematic evaluation of the current literature (Supplementary Table 1), we provide an overview of preclinical studies focused on nutritional interventions in cancer cachexia, and discuss the gaps and highlight opportunities in current experimental models.
Single Nutrient Interventions
Dietary Protein
Adequate supply of dietary protein is a prerequisite for maintenance or gain of skeletal muscle mass. A positive protein balance is required to increase skeletal muscle mass, and elevated plasma levels of essential amino acids from dietary protein are considered an effective anabolic stimulus (16). However, fundamental evidence on the sufficient and optimal quantity and quality of protein intake for treating low muscle mass is lacking. The ESPEN guidelines on nutrition in cancer patients suggest a protein intake in the range of 1.0–1.5 g/kg/day (17). Importantly, these guidelines are recommendations and evidence-based studies to support the optimal quantity are largely missing. Evidence concerning the quality of proteins, regarding the optimal amino acid composition and their digestibility (availability) in the context of cancer cachexia, is also lacking. In contrast to their suitability for defining the optimal quantity of protein resulting from translational challenges, experimental models of cancer cachexia can readily be deployed to study optimal amino acid composition and quality of proteins to prevent or treat cancer cachexia.
Branched-Chain Amino Acids
Branched-chain amino acids (BCAAs) have been hypothesized to exert a therapeutic effect in diseases accompanied by muscle wasting since they are integral components of skeletal muscle proteins and their critical role in stimulating protein synthesis (18). BCAAs may decrease proteolysis and increase protein synthesis in skeletal muscle, primarily through activation of the mTOR pathway and modulation of inflammation through glutamine production (19, 20). Of the BCAAs, leucine is most potent in stimulating muscle protein synthesis, whereas isoleucine and valine are less effective (20). In MAC16 tumor bearing mice, administration of leucine and valine significantly reversed the loss in body weight. Only leucine produced a significant recovery in muscle wet weight by increasing protein synthesis and decreasing degradation (20). In C26 tumor-bearing mice, leucine supplemented diet increased plasma amino acid concentration and counteracted muscle mass loss dose-dependently, while no effect of leucine-rich diet on body weight and/or anorexia was observed (21). In rats bearing the Walker 256 tumor, multiple studies have demonstrated that the loss of skeletal muscle mass induced by cancer cachexia was attenuated by leucine supplementation (22–26). Leucine supplementation did attenuate protein degradation, potentially through their modulatory effects on proteasome subunits; and improved protein synthesis, via activation of the mTOR pathway and downstream kinases. These actions were related to cachexia amelioration but did not increase survival time or reduce tumor growth (22, 23, 26, 27). Furthermore, a different cytokine profile was observed in tumor bearing rats fed a leucine-rich diet after 14 days. Both, the pro- inflammatory cytokines TNF-α, IL-6 and IFNγ and anti-inflammatory cytokines IL-10 and IL-4 were enhanced in the serum compared to tumor bearing control, indicating a cytokine modulatory effect (25). Altogether, these data suggest that leucine supplementation has a beneficial effect on experimental cancer cachexia. The BCAA leucine may attenuate muscle wasting by modulating protein synthesis and proteolysis.
β-Hydroxy-β-Methylbutyrate
β-hydroxy-β-methylbutyrate (HMB) is synthesized in the human body through metabolism of L-leucine (28). Under normal conditions about 5% of dietary leucine is converted into HMB (29, 30). This leucine metabolite is thought to modulate protein turnover, primarily by suppressing protein degradation (28). In mice bearing the MAC16 tumor, HMB supplementation attenuated the development of weight loss accompanied by a small reduction in tumor weight (31, 32). HMB caused a significant increase in the wet weight of soleus muscle and a reduction in protein degradation. Furthermore, Smith et al. showed that HMB was not only capable of attenuating protein degradation in skeletal muscle of cachectic mice but also stimulated protein synthesis (32). In rats bearing the Walker 256 tumor, HMB supplementation significantly decreased tumor weight. These rats maintained body weight, blood metabolic parameters (glucose, lactate, triacylglycerol, and cholesterol) and tissue glycogen levels comparable to non-tumor-bearing rats (33). Similar effects of HMB were obtained in rats bearing the Yoshida AH-130 tumor fed a 4% HMB-enriched diet (34). HMB supplementation led to a significant increase in body weight and a significantly attenuated gastrocnemius loss. Although, protein synthesis was not measured, HMB treatment increased phosphorylation of mTOR and p70S6k compared to both sham and tumor-bearing control, suggesting a direct modulatory effect on muscle protein anabolism (34). Given the beneficial effects of HMB on muscle protein turnover and the observed anti-tumor effect, HMB could be a useful supplement as part of the treatment of muscle wasting in cancer cachexia. However, further exploration of the efficacy of lower doses will be required, as the HMB doses used in these studies are supra-physiological and may not be feasible as intervention in a clinical setting.
Glutamine
Glutamine is the most abundant, non-essential, amino acid in the body that plays a role in a variety of physiological and biochemical processes (35). Glutamine is considered the main metabolic fuel for gastrointestinal enterocytes maintaining the normal integrity of the intestinal mucosa. Furthermore, glutamine plays an essential role as a precursor of peptide, protein, glucose and lipid synthesis (36, 37). Moreover, glutamine is one of the precursor amino acids of glutathione, which is a major antioxidant and a vital component of host defense (38). Although glutamine is the most abundant amino acid in the body, a marked glutamine depletion is observed in many patients with cancer (35). Consequently, glutamine might be useful to treat cancer cachexia. Supplementation with 2% L-glutamine showed to attenuate cancer-induced cachexia, indicated as preserved body weight loss and a lower cachexia index, in rats bearing the Walker 256 tumor (39–43). Furthermore, tumor growth was inhibited in tumor-bearing rats supplemented with L-glutamine (39–42). In cachectic rats, Walker 256 tumor growth caused considerable changes in small intestine metabolism (44). L-Glutamine supplementation restored the intestinal mucosa in the duodenum and jejunum by enhancing cell proliferation as well as increasing villous height, crypt depth, and total height of the intestinal wall (40). Furthermore, L-glutamine supplementation was associated with a significant elevation of glucose and insulin levels compared to control. The resulting hyperglycemia is probably attributable to the increased activity of gluconeogenic enzymes in the small intestine due to the increased availability of glutamine as a glucose precursor (41). All together, these studies suggest a beneficial effect of glutamine on cancer cachexia via enhancing intestinal health and energy metabolism.
Glycine
Glycine is a non-essential amino acid and is often considered biologically neutral. However, studies have shown that L-glycine has effective anti-inflammatory, immunomodulatory and cytoprotective properties (45). The underlying mechanisms responsible for the protective effects of glycine are not completely clear, but may include suppression of calcium signaling, inhibition of inflammatory cell activation and decreased formation of free radicals and other toxic compounds (45). In addition, dietary glycine was also reported to inhibit the growth of certain types of tumors, such as liver tumors (46) and melanoma tumors (47). Only one study investigated the effect of glycine treatment in an experimental model of cancer cachexia. In mice bearing the C26 tumor, glycine administration reduces tumor growth and attenuates cancer-induced cachexia (48). Glycine treatment partially prevented the tumor-induced loss in skeletal muscle mass and cross-sectional area. This protective effect of glycine was associated with preserved muscle function. In skeletal muscle, glycine normalized IL-6 and F4/80, a marker for macrophage in filtration, mRNA expression and reduced oxidative stress. In addition, glycine treatment attenuated Atrogin-1 and MuRF1 mRNA expression. In accordance, protein expression of the initiation factor eIF3-f, a major target of Atrogin-1 and an important regulator of protein synthesis, was preserved (48). This is the first and only study to demonstrate a beneficial effect of glycine on cancer-induced cachexia. Additional research is required to confirm these promising results and to further unravel the anti-tumor and anti-cachectic effects.
Arginine
Arginine is a conditionally essential amino acid, i.e., the body can synthesize sufficient amounts of arginine to meet physiological demands under well-nourished, healthy conditions (49). Arginine is involved in a number of biological processes including cell growth survival and protein synthesis. It is also a precursor in the biosynthesis of nitric oxide (50). Nitric oxide is a ubiquitous cellular messenger that stimulates the release of certain hormones, such as insulin and growth hormone, and is an important regulator of blood flow, tissue oxygenation, and immune function. In addition, arginine may enhance T cell natural killer cell activity, which inhibits tumor growth (51). Therefore, supplementation with arginine could be beneficial for patients with cancer cachexia through modulation of the endogenous anti-tumor response. However, up till now, there are no data in experimental cancer cachexia models published. This could be related to the concern that arginine, or its metabolites, interfere with metabolic pathways that can induce growth of some tumors (52, 53). These findings emphasize the importance that not all nutrients that envision positive effects on muscle anabolism are applicable in the treatment of cancer cachexia.
Dietary Fat
Dietary fat is an important source of energy and contributes a significant caloric value to our diet. In Western diets, dietary fat may constitute 40–45% of the total caloric intake. Dietary fat is not just a source of energy, it also functions as structural component of cell membranes, carries fat soluble vitamins, plays an important role in signal transduction and is a precursor for inflammatory mediators (54).
High-Fat Ketogenic Diet
Currently, there is limited research available to substantiate an optimal energy percentage of dietary fat in cancer cachexia but the effect of ketogenic diets have been investigated in cancer. The ketogenic diet is a high-fat, low-carbohydrate diet designed to increase the blood concentration of free fatty acids and ketone bodies as alternative sources of energy to glucose (55). As tumor cells mostly rely on glucose as a substrate for anaerobic energy production, i.e., Warburg effect (56), ketogenic diets aim to reduce energy sources for the tumor, while providing free fatty acids and ketone bodies as an energy source for the muscle. Consequently, a high fat diet might be expected to prevent host catabolism during cachexia, mainly by tumor growth reduction. Only a few studies investigated the anti-cachectic effects of ketogenic diets in experimental models of cancer cachexia. In mice bearing the MAC16 tumor, Tisdale et al. showed that the cachectic phenotype can be partly reversed by a ketogenic diet [80% of calories supplied as medium chain triglycerides (MCT)], causing reduced tumor growth and an inhibition of body weight loss (57). Body composition analysis showed retention of both fat and fat-free carcass mass in animals fed high levels of MCT. In another study, an 80% MCT-based high-fat diet reduced both tumor weight and host weight loss and restored both nitrogen balance and urea excretion to that of non-tumor-bearing mice (58). Furthermore, amino acid concentrations in plasma were also restored to control levels, suggesting that excessive nitrogen catabolism during cachexia can be prevented. More recently, the effect of an infant formula with a ketogenic composition used to treat patients with refractory epilepsy was investigated on cancer cachexia in C26 tumor-bearing mice (59). Mice receiving the ketogenic formula showed reduced body weight loss and muscle mass loss. Tumor growth and plasma IL-6 levels were significantly decreased in mice receiving the ketogenic formula compared to tumor-bearing control. It seems that the ketogenic diet inhibits tumor growth and thereby prevents host catabolism. Collectively, these studies suggest that the ketogenic diet with adequate amounts of proteins has beneficial effects on the development of cancer cachexia. However, there is some debate concerning the use of ketogenic diets and the development of dyslipidemia. Some (60, 61) but not all (62) studies indicate that a ketogenic diet produce significant increases in the plasma levels of total cholesterol and triglycerides. Elevated levels of plasma triglycerides and cholesterol are often detected in patients suffering from cancer cachexia (63), as a result of increased lipolysis (64, 65). This tumor induced dyslipidemia, in turn causes lipotoxic effects in other tissues including skeletal muscle (65, 66). Considering the potential impact of dyslipidemia to cancer cachexia, this potential effect should be further investigated to probe the feasibility of the ketogenic diets in patients with cancer cachexia.
Polyunsaturated Fatty Acids
Dietary long chain polyunsaturated fatty acids (lcPUFAs) have an effect on diverse physiological processes affecting normal health and chronic diseases (67–69). The n-3 and n-6 lcPUFA families are derived from the desaturation and elongation of the essential lcPUFAs α-linolenic and linoleic acids that are ingested as components of food. The principal members of the n-3 lcPUFA family are eicosapentaenoic acid (EPA) and docosahexaenoic acid (DHA), whereas arachidonic acid is the main derivate of the n-6 lcPUFA family. It is known that eicosanoids derived from n-6 lcPUFAs have pro-inflammatory and immune-active functions, whereas n-3 lcPUFA-derived eicosanoids have anti-inflammatory properties, attributable to their ability to inhibit the formation of n-6 lcPUFA-derived eicosanoids.
Diet supplementation with fish oil, which is rich in n-3 lcPUFAs EPA and DHA, has been investigated to preserve skeletal muscle mass in various experimental animal models of cancer cachexia. Most of these studies show that fish oil is an effective nutritional intervention to induce body weight gain, reduce tumor growth rate and reverse food intake. In 1990, Tisdale et al. showed for the first time that a diet enriched in fish oil reduced both tumor growth and weight loss produced by the MAC16 adenocarcinoma (70). Reversal of host body weight loss was associated with an increase in total body fat and muscle mass. Although the amount of fish oil required for optimal activity was high (50% of total calories), no toxicities were observed (70). Comparable results were found in Walker 256 tumor-bearing rats receiving an n-3 fish oil diet. Tumor weight gain in fish oil fed rats was reduced and these animals gained body weight and maintained blood metabolic parameters (glucose, lactate, triacylglycerol and cholesterol) similar to non-tumor-bearing rats (71). Fish oil supplementation via oral gavage reduced body weight loss and tumor weight gain to a similar extent (72). Finally, some studies investigated the effect of lifelong supplementation of the diet with fish oil on cancer cachexia (73, 74). In these studies, the diet of pregnant and lactating rats and subsequent male offspring was supplemented with fish oil. Lifelong supplementation of the diet with fish oil significantly decreased tumor growth, increased survival, reversed food intake and prevented body weight loss. Furthermore, fish oil supplementation partly preserved blood metabolic parameters in tumor-bearing rats compared to control (73, 74). However, it is difficult to predict if the effects found in these lifelong supplementation studies can be translated into a treatment regimen relevant to cancer cachexia.
While most studies reported clinically and statistically significant effects of fish oil supplementation on preventing body weight loss, Dumas et al. have found no effect (75). In rats with peritoneal carcinosis, fish oil-enriched diet delayed the occurrence of anorexia compared to the control diet. Furthermore, ascites production was lower in fish oil treated rats. However, no difference in body weight gain, fat mass and muscle mass was reported (75). The discrepancies could be due to differences in the design of the study. In the aforementioned studies, in both the treatment arm and control arm relative weight gain of animals and tissues were assessed at the same time point, whereas Dumas et al. assessed the final measurements when anorexia was evident in all animals, ensuing different time points per group.
Besides fish oil, shark liver oil is a common dietary supplement rich in n-3 lcPUFAs. Prophylactic supplementation with shark liver oil promoted gain in body weight, reduction of tumor weight and maintained blood metabolic parameters in Walker 256 tumor bearing rats (76, 77). However, shark liver oil supplementation showed less potent effects compared to fish oil (77).
The anti-cachectic and anti-tumor effect of purified EPA has also been investigated in various experimental animal models. In mice bearing the cachexia-inducing MAC16 adenocarcinoma, EPA was found to stabilize weight loss, delay growth of the tumor, and increase the overall survival (78–80). Such anti-cachectic effect was not achieved by the use of DHA or linoleic acid alone (79). Supplementation with EPA inhibited tumor-induced lipolysis and reduced protein degradation without an effect on protein synthesis (78, 81, 82). In mice bearing the cachexia-inducing S180 ascites tumor, oral administration of EPA prevented body weight loss by preserving the white adipose tissue mass (83). Furthermore, EPA administration suppressed plasma levels of pro-inflammatory cytokines such as TNF-α and IL-6. Lastly, EPA treatment also preserved several key lipolytic factors and raised the mRNA levels of some adipogenic factors in the white adipose tissue (83). A higher body weight gain in response to EPA supplementation has also been reported in Apc(min/+) mice (84), rats implanted with MCA sarcoma (85), and rats treated with MAT-LyLu prostate tumor cells (86).
Taken together, these studies suggest that n-3 lcPUFAs, in particular EPA, are effective in preventing body weight loss and tumor growth in experimental animal models of cancer cachexia. However, little is known on the underlying mechanisms of n-3 lcPUFAs/EPA in cancer cachexia. The aforementioned data suggest that n-3 lcPUFAs/EPA is able to suppress lipolysis, probably by downregulating lipid mobilizing factors (87). Whether the anti-lipolytic activity is a direct effect of n-3 lcPUFAs/EPA or related to its anti-inflammatory properties through inhibiting pro-inflammatory cytokines, such as TNF- α and IL-6, needs to be further elucidated.
Conjugated Linoleic Acid
Conjugated linoleic acid (CLA) is a group of at least 28 isomers of linoleic acid found mostly in red meat and dairy products derived from ruminants (88). CLA is marketed as a weight-loss supplement to reduce body fat and promote lean muscle growth (e.g., Tonalin®). Experimental studies have shown that CLA has anti-carcinogenic effects (89, 90). Furthermore, CLA was protective against TNF- α and LPS induced muscle wasting (91, 92). However, data on the effect of dietary supplementation of CLA in the treatment of cancer cachexia is contradictory. In mice bearing the C26 tumor, Graves et al. showed that a diet containing 0.5% CLA preserved skeletal muscle mass and reduced TNF receptor type 1 levels in muscle homogenates (93). In mice bearing the LLC tumor, the same diet reduced skeletal muscle wasting, but had no effect on skeletal muscle levels of TNF receptor type 1 (94). In mice bearing the B16 melanoma, 0.5% CLA did not affect skeletal muscle mass or TNF receptor type 1 levels in skeletal muscle (94). The notion that CLA has beneficial effects in cancer cachexia was also not supported by Tian et al. (95). In this study, a 1% CLA enriched diet did not affect skeletal muscle mass and adipose tissue mass. CLA supplementation did not inhibit the induction of proteolytic markers. Instead, MuRF1 expression was significantly higher in C26 tumor-bearing mice receiving a diet containing 1% CLA. In skeletal muscle, CLA enhanced tumor- induced gene expression of inflammatory markers TNF- α, IL-6 receptor and F4/80. Moreover, in epididymal adipose tissue, tumor driven lipolysis was aggravated by CLA supplementation (95). More recently, in rats bearing the Walker 256 tumor, CLA treatment aggravated cachexia symptoms, including increased inflammatory status, steatosis and hyperlipidemia (96). Collectively, these results do not provide strong support for CLA in the treatment of cancer cachexia.
Other Nutrients
Carnitine
Carnitine is a trimethylamine, which is synthesized in the liver and kidney via the conversion of two essential amino acids, lysine and methionine. Carnitine plays a major role in the import of long chain fatty acids from the cytosol into the mitochondrial matrix for subsequent β-oxidation (97, 98). Inefficiency of this bioenergetics process results in increased oxidative stress, contributing to the development of metabolic abnormalities and the release of pro-inflammatory cytokines. Consequently, supplementation of carnitine to enhance mitochondrial β-oxidation may attenuate oxidative stress and inflammation, resulting in beneficial clinical outcomes. Carnitine supplementation has been studied in various experimental models of cancer cachexia. In rats bearing the Yoshida AH-130 tumor, L-carnitine treatment resulted in greater food intake (99, 100). Protein levels of carnitine palmitoyl transferase-1 (CPT-1) enzyme, a marker of carnitine effects, were higher in the muscles of tumor-bearing rats treated with L-carnitine compared with the non-treated cachectic animals (100). This was accompanied by an inhibition of tumor-induced muscle wasting (99, 100) and an increase in physical activity (99). Moreover, L-carnitine treatment resulted in a down-regulation of atrogin-1 and MuRF1 and a decrease in the proteasome activity in gastrocnemius muscle (99, 100). In addition, the pro-apoptotic marker caspase-3 in skeletal muscle tended to be decreased in muscle of tumor-bearing rats treated with L-carnitine (99). In mice bearing the C26 tumor, L-carnitine significantly increased food intake, muscle mass and epididymis fat weight through the upregulation of CPT (101). In addition, the increased CPT activity was associated with reduced plasma levels of IL-6 and TNF-α (101). In cachectic rats bearing the Walker 256 tumor, CPT activity was reduced and liver and plasma triacylglycerol content was increased. L-Carnitine treatment restored these measures back to control values, showing that L-carnitine preserves hepatic lipid metabolism in experimental cancer cachexia (102). Current evidence suggests that carnitine might help to ameliorate muscle wasting in cancer, although more molecular studies investigating the exact working mechanism are needed.
Creatine
Creatine is a non-protein amino acid that can be endogenously synthesized in the liver, kidney and pancreas and is mainly stored and utilized in the skeletal muscle. Creatine can be phosphorylated by creatine kinase to form phosphocreatine, which plays an important bioenergetics role by providing rapid energy during muscle contraction, where phosphocreatine donates a phosphate group to adenosine diphosphate to resynthesize adenosine triphosphate (103, 104). Given that oral creatine supplementation augments its intramuscular content and has the capacity to effectively enhance muscle strength and lean body mass (105–107), makes it an interesting supplement to treat muscle-wasting diseases. Indeed, creatine supplementation has been successfully used as an adjuvant treatment in numerous myopathies (108–110). Furthermore, creatine supplementation has shown antioxidant capacities as well as effectiveness to counteract pro-inflammatory cytokines (111, 112). Despite the promising results, only a few studies have investigated the effects of creatine supplementation in experimental cancer cachexia. In rats bearing the Walker 256 tumor, creatine supplementation attenuated body weight loss and tumor growth was decreased (113–115). Cancer-induced skeletal muscle atrophy was attenuated by creatine, as evidenced by the increase in muscle fiber cross-sectional area. Creatine also prevented cancer-induced increase in Atrogin-1 and MuRF1 protein levels. Furthermore, creatine supplementation prevented the increase in plasma TNF-α and IL-6 (113, 114), while it increased plasma IL-10 (114). However, mean survival time was not different compared to tumor-bearing control (113, 115). Given the beneficial effects of creatine supplementation on skeletal muscle mass maintenance in experimental cancer cachexia and the promising results in other muscle wasting diseases, creatine could be a useful supplement to treat muscle wasting in cancer cachexia and should be the objective for future studies. It should be pointed out however that creatine supplementation may increase urinary creatinine levels, which complicates interpretation of the latter as a marker of renal dysfunction in patients with a history or risk of renal disease.
Flavonoids
Flavonoids are a large group of polyphenolic compounds and are ubiquitously expressed in plants. Fruits and vegetables are the main dietary sources of flavonoids for humans, along with tea and wine. As a dietary component, flavonoids are thought to have health-promoting properties due to their antioxidant, hepatoprotective, anti-inflammatory and anti-carcinogenic properties (116). Despite several known effects of flavonoids on health and disease, research into the effects of flavonoids on cachexia prevention has been limited to date. In Apc(min/+) mice, quercetin supplementation attenuated the progression of cancer cachexia (117). Quercetin significantly attenuated body weight loss, but did not affect the loss of epididymal fat in Apc(min/+) mice. After 3 weeks of supplementation, the loss of muscle mass and grip strength shown in Apc(min/+) mice was significantly attenuated by quercetin. Furthermore, increased plasma IL-6 levels were completely mitigated by quercetin in this model of intestinal cancer. In this study, no effect of quercetin supplementation on tumor number was observed, while a reduction of the tumor weight was found in rats bearing the Walker 256 tumor (118). Epigallocatechin-3-gallate (EGCG), the principal polyphenolic component in green tea, effectively attenuates skeletal muscle atrophy in mice bearing the Lewis lung carcinoma (LLC) tumor (119). EGCG supplementation inhibited tumor growth and the loss of body weight was significantly slowed down. Furthermore, it was shown that EGCG positively regulates the expression of muscle-specific ubiquitin ligase genes encoding MuRF-1 and Atrogin-1 (119). Another study examined the effect of isoflavones derived from soy extracts on muscle atrophy in LLC-bearing mice (120). The isoflavone diet attenuated the tumor-induced loss in wet weight and myofiber size of the gastrocnemius muscle. Moreover, the increased expression of MuRF-1 and Atrogin-1 was significantly suppressed by the supplementation of isoflavones. In parallel, the isoflavone diet significantly inhibited the phosphorylation of ERK in skeletal muscle of tumor-bearing mice. No effect of dietary isoflavones on tumor mass or pro-inflammatory cytokines IL-6 and TNF-α were observed (120). Morin, another flavonoid, was able to suppress tumor-induced skeletal muscle wasting in LLC-bearing mice (121). Dietary morin prevented the reduction of muscle wet weight and myofiber size. Moreover, the tumor mass in mice fed the morin diet was significant lower compared to mice fed the normal diet. It was suggested that morin indirectly prevents muscle atrophy by suppressing tumor growth via targeting ribosomal protein S10. The anti-proliferative effect of morin had a cell specific action that was only apparent in tumor cells, but not in muscle cells (121). Because of the variation in flavonoids and limited number of studies, there is not enough evidence to recommend flavonoids as a potential supplement to treat muscle wasting in cancer. However, the available data merits further investigation of their potential to modulate cancer cachexia.
Resveratrol
Resveratrol (3,5,4′-trihydroxystilbene) is a phytoalexin, a class of compounds produced by many plants when they are infected or physically harmed (122); it has been reported to have anti-tumor effects in rats (123). Research on the potential positive effects of resveratrol in cancer cachexia is limited and contradictory. In mice bearing the MAC16 tumor with established weight loss, resveratrol partly blocked weight loss by interfering with NF-κB activity in skeletal muscle and this was accompanied by inhibition of tumor growth (124). Another study demonstrated that resveratrol inhibited skeletal muscle atrophy induced by the C26 adenocarcinoma tumor through its inhibition of NF-κB activity in skeletal muscle (125), without affecting tumor growth. In contrast to these studies, administration of resveratrol did not attenuate skeletal muscle mass loss or body weight loss in both rats bearing the Yoshida AH-130 tumor and mice bearing the LLC (126). In fact, in rats, administration of resveratrol exaggerated cancer cachexia (126). On the contrary, resveratrol was able to decrease proteolysis in vitro (126). After absorption, resveratrol undergoes rapid and extensive metabolism leading to low bioavailability (127, 128). With respect to the poor bioavailability it might be not surprising that resveratrol did not prevent muscle wasting, as the likelihood to reach effective levels in plasma or muscle is very low. Taking these aforementioned studies into consideration, resveratrol is not likely able to ameliorate tumor induced cachexia.
Prebiotic Non-digestible Oligosaccharides
A prebiotic is “a selectively fermented ingredient that allows specific changes, both in the composition and/or activity in the gastrointestinal microflora that confers benefits upon host well-being and health” (129). Prebiotic oligosaccharides such as galacto-oligosaccharides (GOS) and fructo-oligosaccharides (FOS) have shown to have immune modulating activities, observed in several animal experiments, and clinical trials (130–132). These oligosaccharides have been associated with improved gut barrier function (133). It stimulates bifidobacteria, lactobacilli and other healthy bacteria, while it reduces harmful bacteria in the gut. Moreover, these oligosaccharides may block or activate specific receptors on immune cells leading to improved immune responses. In addition, oligosaccharides are fermented by colonic bacteria into short-chain fatty acids (133). Short-chain fatty acids have been shown to exert anti-carcinogenic as well as anti-inflammatory properties (134). Although, the exact role of the microbiome in cancer cachexia is not clear yet, animal studies suggest that the composition of the microbiota and intestinal barrier function is affected by the presence of a tumor and the development of cancer cachexia (135). These findings support the rational to target the gut microbiota in cancer cachexia using prebiotic non-digestible oligosaccharides. The therapeutic potential of prebiotic non-digestible oligosaccharides in cancer cachexia have been barely explored. In mice bearing the C26 tumor, diet supplemented with GOS and FOS (weight ratio 9:1) significantly reduced skeletal muscle mass loss (136). Similar results were obtained when the FOS was replaced by additional GOS (136). Administration of a prebiotic candidate, pectic oligosaccharide (POS), to leukemic mice mitigated the cachectic phenotype, by delaying anorexia and preserving fat mass (137). In addition, POS increased the total number of bacteria and induced a drastic change in microbial diversity and populations. No effect on inflammation was observed (137). Due to limited research, the impact of prebiotic non-digestible oligosaccharides on cancer cachexia is currently unclear and would warrants further investigation.
Multinutrient Interventions
As discussed in section Single nutrient interventions, numerous nutrients led to positive results in experimental models of cancer cachexia, targeting different aspects of the wasting syndrome. Combining such high quality nutrients in a “multinutrient approach” to treat cachexia, is expected to have a superior impact compared to single nutrients. A multinutrient approach is aimed at targeting specific factors involved in cancer cachexia, e.g., anorexia, altered fat, and protein metabolism, and systemic inflammation, but also the replenishment of nutrient deficiencies.
Several of these multi-nutrient approaches have been studied in experimental models of cancer cachexia. In mice bearing the MAC16 tumor, Smith et al. investigated the effect EPA combined with protein (casein), amino acid mixture (leucine, arginine and methionine), and carbohydrate on protein synthesis and degradation in gastrocnemius muscle (82). Treatment with only EPA significantly reduced protein degradation, but had no effect on protein synthesis. Combination of EPA with casein tended to increase protein synthesis. However, when combined with the amino acid mixture, protein synthesis almost doubled. The addition of carbohydrate to stimulate insulin release had no additional effect (82). In another study, Van Norren et al. examined the effect of dietary supplementation with a specific combination of high protein (100% casein), leucine and fish oil (EPA and DHA in a ratio of 2.2:1) on body composition in mice bearing the C26 tumor (138). The multinutrient intervention targeting catabolism, anabolism and essential amino acid supply significantly reduced loss of carcass, muscle and fat mass. Addition of the single nutrients to the diets resulted in no or marginal effects (138). In a second experiment, the effect of a more humanized diet on weight loss, muscle function and physical activity was studied, referred to as Specific Nutritional Composition, containing high protein (68% casein and 32% whey), leucine, fish oil (EPA and DHA in a ratio of 2.2:1), and the oligosaccharides GOS and FOS. The specific nutritional composition diet significantly reduced loss of body, carcass, muscle and fat mass. Furthermore, tumor weight was significantly lower compared to tumor-bearing control. Muscle performance was improved and total daily activity was normalized after intervention with the specific nutritional composition diet (138, 139). The specific nutritional composition diet also showed beneficial immune modulatory effects. The nutritional combination significantly improved the Th1 immunity and plasma levels of IL-6, TNF-α and PGE2 were significantly reduced (139). Furthermore, this combination attenuated weight loss and inflammatory markers, and reduced pathogen levels and bacterial translocation in a chemotherapy model (140). In mice bearing the B16 melanoma, the effect of a multi-nutrient intervention with BCAAs, citric acid, L-carnitine, coenzyme Q10 and various vitamins and minerals was investigated on the development of cancer cachexia (141). The mice receiving the intervention diet showed a higher cumulative food intake compared to tumor-bearing control. In addition, tumor weight was significantly lower and lung metastasis of the B16 melanoma cells were absent in the intervention group. However, attenuation of muscle tissue loss was only observed for the suprahyoid muscles in the neck but not for gastrocnemius or soleus muscles in the lower leg (141). More recently, the effect of total nutrition formula on LLC-bearing mice was investigated (142). Total nutritional formula is an energy- and protein-dense oral nutritional supplement fortified with micronutrients such as vitamin D, vitamin A, coenzyme Q10 and selenium. Daily oral supplementation with total nutritional formula significantly suppressed tumor growth, while body weight loss and gastrocnemius muscle mass loss were significantly attenuated. Furthermore, the plasma levels of TNF-α and IL-6 were significantly decreased in LLC-bearing mice supplemented with total nutritional formula (142).
Clearly, the few studies in which the combination of nutrients was systemically compared to single nutrients alone strongly support the concept of a multinutrient approach. More experimental dietary intervention studies should focus on multi-nutrient interventions targeting multiple aspects of the disease. Furthermore, future studies should define optimal selection and balance of nutrients that work in concert to produce a health benefit that is greater than the sum of the single nutrients.
Nutritional Interventions: Gaps and Opportunities
All of the nutritional intervention studies included in this review describe effects on phenotypic measures of cachexia, such as body weight loss and/or muscle mass loss. However, only few of the studies contribute to understanding the underlying mechanisms by which beneficial effects of nutritional interventions are mediated. Of all reviewed nutrients, only L-leucine and its metabolite HMB have been shown to attenuate tumor-induced muscle wasting by (directly) modulating muscle protein synthesis and proteolysis. For many other nutrients, the anti-cachectic effect is frequently associated with reduced tumor growth, modulation of inflammatory signaling or other extra-muscular alterations that may contribute to cachexia. In these studies, it is difficult to discriminate whether these nutrients act directly on skeletal muscle metabolism, or impact indirectly on cachexia. A better understanding of the beneficial actions of nutritional interventions will not only aid their clinical implementation, but also the systematic evaluation of additive or synergistic effects of rationally selected nutritional combinations with distinct or complementary actions.
Standardization in Experimental Design
Given the heterogeneity of cancer-induced cachexia, the results obtained in the reviewed studies must be considered in the context of the specific models that were used, and variations in dietary regiments that were applied. Although these variations may cause discrepancies between results across different animal models, and laboratory groups, preclinical studies per se have provided advantages and results that merit their application in cachexia research. The experimental models of cancer cachexia allow analysis of different tissues affected by cancer cachexia, and provide valuable insights in the underlying mechanisms of tested interventions. In addition, safety and efficacy parameters that are relevant and translatable to clinical practice are defined in experimental models, including identification of potentially harmful interactions with other treatment modalities. Nevertheless, various aspects in the experimental design would benefit from standardization. When evaluating the potential beneficial effects of the nutritional interventions in experimental cancer cachexia, a wide variation in timing, duration, dosing and route of supplementation was observed, as well as in animal models used (Supplementary Table 1).
Intervention as Source of Variation
Dietary intake, composition and dose is an often overlooked source of variation in experimental animal studies of cancer cachexia (143). The majority of the studies reviewed provide diets ad libitum, while only a few studies control daily food intake. However, it should be kept in mind that anorexia is an important contributing factor to cancer-induced muscle wasting and that dietary intake is closely related to cachexia-related outcome measures. This stresses the importance of dietary standardization, recording of food intake or pair feeding the control group, to correlate the amount of nutrient intake with outcome measures. Details on dietary intake are often provided, however, the exact composition of the experimental diets is often not described. Many of the studies reviewed used standard laboratory chow. Standard laboratory chow diets do not have a standardized macronutrient composition and often vary from batch to batch (144). Furthermore, the ingredients of laboratory chow are rarely defined, therefore it might contain unknown nutrients that may impact study endpoints. The use of purified or semi-purified diets should be the new standard. In comparison to standard laboratory chow, the ingredients included in purified and semi-purified diets are open formulas and well-characterized (145). In addition, the formulation of the semi-purified diet can be altered by the researcher according to the research objective. Besides the choice of the background diet, the control diet and intervention diet should be matched for calories and nitrogen content. The proportions of the nutrients in relation to human intake should also be considered, improving the translatability to the clinic. Furthermore, the dose of the supplemented nutrients is a point of attention. Some of the intervention studies use supraphysiological doses that may not be feasible for a nutritional intervention. For example, a diet with 4% HMB is rather unbalanced considering that normal rodent chow contains only 12.5% protein.
The timing and duration of supplementation as well as the route of administration vary between studies and is another source of variation. The majority of the studies started supplementation before or with tumor inoculation, while only a few initiate supplementation when animals show evidence of cachexia. In both study designs, the metabolic state of the animals at the start of the intervention is different, each of which may require a different formulation of an effective nutritional intervention. Besides, nutrients might have a fundamental different action in such models, for example by affecting tumor take or growth. In addition to the timing, the duration of the intervention studies varies substantially, ranging from 24 h to 34 days after tumor inoculation. This suggests that the cachectic state at the end of the study differs between these studies, making it difficult to compare phenotypical and biochemical data between studies.
In addition, different routes of administration were applied in the reviewed experimental intervention studies. Only a few administrated the intervention via intra-peritoneal injection, subcutaneous injection or drinking water. A significant number of studies supplemented the nutrients via oral gavage. Similar to the injections, the advantage of administration via oral gavage is that an exact amount of the nutrient is delivered irrespective of the food intake, which minimizes variation between animals. However, by administration via oral gavage the nutrient appears as a bolus and is not part of the food matrix. Furthermore, administration via oral gavage may have impact on the food intake and the intervention diet is often not isonitrogenous and isocaloric to the control diet. In the majority of the studies the nutrients are administered via the diet, either by modification of semi-purified diets, or by incorporation into the standard laboratory chow. This allows to explore the full metabolic potential of nutritional interventions, as it takes its processing through the complete digestive tract into consideration. Moreover, from a translational perspective this may be the preferred route of administration, as its clinical application will be accordingly.
Combined, systematic attention to the aspects discussed above will contribute to further standardization in experimental design and reporting of experimental details. In turn, this will greatly stimulate the speed to explore promising leads and turn these into reproducible preclinical intervention diets, with robust translational potential in cancer cachexia.
Heterogeneity Between Animal Models of Cancer Cachexia
Numerous well-established animal models of cancer cachexia are used (Table 1). However, there is substantial variability between them in terms of cell type, site of inoculation/implantation, tumor growth (speed and size), development of metastasis, the overall dynamics of the wasting process, and putative underlying mechanisms (e.g., anorexia, inflammation) (146). This makes it difficult to compare results across animal models. Furthermore, these animal models often do not recapitulate all major clinical characteristics present in cancer cachexia, which may complicate direct translation of the findings to the clinic. For example, the kinetics by which cachexia develops in these models differs from that in patients. The effectiveness of some treatments may be of transient nature and lost in patients with chronic cachexia, while treatments that do not prove effective in acute models of cachexia might be useful for treating chronic cachexia. Furthermore, the majority of the animal models have ectopically growing tumors. Although, these animal models have been useful in investigating the underlying mechanisms of cancer cachexia, animal models of cancer cachexia with orthotopically growing tumors may more closely represent clinical cancer cachexia. Orthotopic tumor growth provides tumor cells its original stroma and microenvironment, both of these elements may determine the etiology of cachexia and affect the outcome of interventions. Indeed, recently, it has been demonstrated for a murine model of pancreatic cancer cachexia that orthotopic implantation of tumor cells on the location of interest much better recapitulates the clinical characteristics of cancer cachexia compared to subcutaneous tumor models (147). The growth monitoring capabilities for palpable, subcutaneously grown tumors is no longer a rationale for avoiding the use of orthotopic models, as recent studies have demonstrated the routine deployment of non-invasive imaging in various models, including orthotopically grown lung and brain tumors, to follow up tumor growth (148–150). Moreover, micro-CT imaging-based automated 3D contouring algorithms have been developed to simultaneously determine muscle mass changes in a model of orthotopic lung cancer cachexia (151). Finally, orthotopic tumor models provide an opportunity to study the interaction between conventional cancer therapies (e.g., chemo-, immune-, and radiation therapy) and adjuvant interventions targeting cachexia. Taken together, the development of more humanized models to study the effectiveness of interventions to treat cancer cachexia may lead to a more rapid translation into clinical trials.
Combining Nutrition With Anti-cancer Therapy and Cachexia Interventions
Although not in the scope of this review, in addition to tumor activity and host responses that may drive cachexia, there is increasing clinical evidence that treatments directed at the tumor may actually contribute to the development and progression of cachexia (152, 153). Studies in healthy mice and rats have also demonstrated that some chemotherapeutic agents induce muscle wasting (154, 155). Recently, it was for the first time demonstrated that distinct metabolic derangements are present in cancer-induced and chemotherapy-induced cachexia (156). This implies that different therapeutic strategies to treat cachexia may be required. A better understanding of the tumor-, host-, and treatment-induced drivers of cachexia in combination with in-depth knowledge on the mechanism of nutritional interventions is crucial to develop effective therapeutic interventions for cancer-induced and treatment-induced cachexia. Moreover, as in practice nutritional interventions will be used in combination with anti-cancer therapies such as chemo-, radiation- and immunotherapy, it is necessary to investigate if such combinations are safe, and whether they modulate the therapeutic effectiveness. Some nutrients, such as lcPUFAs, may improve the adherence to and efficacy of chemotherapy (157), while others, including carnitine and glutamine are suggested to reduce the toxicity of anti-cancer therapy in cachectic patients (158).
Considering cancer cachexia is a multifactorial syndrome, it is expected that a multimodal approach targeting multiple aberrant pathophysiologic pathways simultaneously will be most efficient. In addition to optimized nutritional care, other therapeutic strategies, including exercise training and pharmacological interventions, could effectively contribute to attenuate cancer related muscle wasting. Although not all treatment modalities can be modeled in pre-clinical experimental set ups, efficacy, synergy and relevance of some combined treatment modalities have been evaluated. In particular, exercise training has some experimental evidence of benefit to mitigate skeletal muscle loss and therefore should be seen as potential tool to maximize outcomes and quality of life of patients with cancer cachexia (159–162). Exercise training stimulates the increase of muscle mass and strength and might improve cancer-associated skeletal muscle wasting by stimulating anabolic pathways as well as by down-regulating the activity of pro-inflammatory cytokines (163). In addition, exercise training has a beneficial effect on counteracting fatigue, which is one of the most severe symptoms in cancer patients (164). Combining exercise training and nutritional intervention was shown to be more effective than either one alone, highlighting the potential of multimodal interventions with a nutritional component (159, 161, 162). Besides exercise training, pharmacological agents such as appetite stimulants, anabolic steroids, and non-steroidal anti-inflammatory drugs could effectively contribute to the treatment of cachexia (165). Carefully designed combination therapy may maximize the impact on treating muscle wasting while minimizing the chance for drug toxicities. Various combinations of single nutrients with exercise or pharmacological agents have been studied in experimental cancer cachexia, with some success. However, the number of studies is limited and therefore more research is needed to find the optimal combination. In future studies, attention should be paid on the interaction between the different components of the multimodal approach. It is important that the combination is safe and contributes to the total therapy effectiveness.
Conclusion
Targeted nutrition is pivotal in preserving muscle mass in cancer cachexia. A large number of studies demonstrated beneficial effects of nutritional interventions on muscle wasting. However, evidenced-based studies that indicate the optimal quantity and quality of the nutrients are often missing. Furthermore, the combination of multiple nutrients is expected to have a superior impact compared to single nutrients alone. More studies investigating the optimal quantity, quality and combination of nutrients are needed. Rationally-designed nutrition intervention studies, performed in well-characterized experimental models are an essential approach in the design of multimodal therapies targeting cancer cachexia.
Author Contributions
WW has drafted the manuscript. WW, AS, JT, AH, and RL edited and revised the manuscript. All authors listed have approved it for publication.
Conflict of Interest
AH was employed by Nutricia Research.
The remaining authors declare that the research was conducted in the absence of any commercial or financial relationships that could be construed as a potential conflict of interest.
Supplementary Material
The Supplementary Material for this article can be found online at: https://www.frontiersin.org/articles/10.3389/fnut.2020.601329/full#supplementary-material
References
1. Fearon K, Strasser F, Anker SD, Bosaeus I, Bruera E, Fainsinger RL, et al. Definition and classification of cancer cachexia: an international consensus. Lancet Oncol. (2011) 12:489–95. doi: 10.1016/S1470-2045(10)70218-7
2. Anker MS, Holcomb R, Muscaritoli M, von Haehling S, Haverkamp W, Jatoi A, et al. Orphan disease status of cancer cachexia in the USA and in the European Union: a systematic review. J Cachexia Sarcopenia Muscle. (2019) 10:22–34. doi: 10.1002/jcsm.12402
3. Prado CM, Lieffers JR, McCargar LJ, Reiman T, Sawyer MB, Martin L, et al. Prevalence and clinical implications of sarcopenic obesity in patients with solid tumours of the respiratory and gastrointestinal tracts: a population-based study. Lancet Oncol. (2008) 9:629–35. doi: 10.1016/S1470-2045(08)70153-0
4. Dewys WD, Begg C, Lavin PT, Band PR, Bennett JM, Bertino JR, et al. Prognostic effect of weight loss prior to chemotherapy in cancer patients. Eastern cooperative oncology group. Am J Med. (1980) 69:491–7. doi: 10.1016/S0149–2918(05)80001–3
5. Vaughan VC, Martin P, Lewandowski PA. Cancer cachexia: impact, mechanisms and emerging treatments. J Cachexia Sarcopenia Muscle. (2013) 4:95–109. doi: 10.1007/s13539-012-0087-1
6. Turner DC, Kondic AG, Anderson KM, Robinson AG, Garon EB, Riess JW, et al. Pembrolizumab exposure-response assessments challenged by association of cancer cachexia and catabolic clearance. Clin Cancer Res. (2018) 24:5841–9. doi: 10.1158/1078-0432.CCR-18-0415
7. Aapro M, Arends J, Bozzetti F, Fearon K, Grunberg SM, Herrstedt J, et al. Early recognition of malnutrition and cachexia in the cancer patient: a position paper of a European school of oncology task force. Annals Oncol. (2014) 25:1492–9. doi: 10.1093/annonc/mdu085
8. Ezeoke CC, Morley JE. Pathophysiology of anorexia in the cancer cachexia syndrome. J Cachexia Sarcopenia Muscle. (2015) 6:287–302. doi: 10.1002/jcsm.12059
9. Johnen H, Lin S, Kuffner T, Brown DA, Tsai VW, Bauskin AR, et al. Tumor-induced anorexia and weight loss are mediated by the TGF-beta superfamily cytokine MIC-1. Nat Med. (2007) 13:1333–40. doi: 10.1038/nm1677
10. Vazeille C, Jouinot A, Durand JP, Neveux N, Boudou-Rouquette P, Huillard O, et al. Relation between hypermetabolism, cachexia, and survival in cancer patients: a prospective study in 390 cancer patients before initiation of anticancer therapy. Am J Clin Nutr. (2017) 105:1139–47. doi: 10.3945/ajcn.116.140434
11. Baracos VE, Mackenzie ML. Investigations of branched-chain amino acids and their metabolites in animal models of cancer. J Nutr. (2006) 136(1 Suppl):237s-42s. doi: 10.1093/jn/136.1.237S
12. Argilés JM. Cancer-associated malnutrition. Eur J Oncol Nurs. (2005) 9(Suppl 2):S39–50. doi: 10.1016/j.ejon.2005.09.006
13. von Meyenfeldt M. Cancer-associated malnutrition: an introduction. Eur J Oncol Nurs. (2005) 9(Suppl 2):S35–8. doi: 10.1016/j.ejon.2005.09.001
14. Grober U, Holzhauer P, Kisters K, Holick MF, Adamietz IA. Micronutrients in oncological intervention. Nutrients. (2016) 8:163. doi: 10.3390/nu8030163
15. Prado CM, Purcell SA, Laviano A. Nutrition interventions to treat low muscle mass in cancer. J Cachexia Sarcopenia Muscle. (2020) 11:366–80. doi: 10.1002/jcsm.12525
16. Fujita S, Dreyer HC, Drummond MJ, Glynn EL, Cadenas JG, Yoshizawa F, et al. Nutrient signalling in the regulation of human muscle protein synthesis. J Physiol. (2007) 582(Pt 2):813–23. doi: 10.1113/jphysiol.2007.134593
17. Arends J, Bachmann P, Baracos V, Barthelemy N, Bertz H, Bozzetti F, et al. ESPEN guidelines on nutrition in cancer patients. Clin Nutrit. (2017) 36:11–48. doi: 10.1016/j.clnu.2016.07.015
18. Kobayashi H, Kato H, Hirabayashi Y, Murakami H, Suzuki H. Modulations of muscle protein metabolism by branched-chain amino acids in normal and muscle-atrophying rats. J Nutrition. (2006) 136(1 Suppl):234S-6S. doi: 10.1093/jn/136.1.234S
19. Nicastro H, da Luz CR, Chaves DF, Bechara LR, Voltarelli VA, Rogero MM, et al. Does branched-chain amino acids supplementation modulate skeletal muscle remodeling through inflammation modulation? Possible mechanisms of action. J Nutr Metab. (2012) 2012:136937. doi: 10.1155/2012/136937
20. Eley HL, Russell ST, Tisdale MJ. Effect of branched-chain amino acids on muscle atrophy in cancer cachexia. Biochem J. (2007) 407:113–20. doi: 10.1042/BJ20070651
21. Peters SJ, van Helvoort A, Kegler D, Argiles JM, Luiking YC, Laviano A, et al. Dose-dependent effects of leucine supplementation on preservation of muscle mass in cancer cachectic mice. Oncol Rep. (2011) 26:247–54. doi: 10.3892/or.2011.1269
22. Ventrucci G, Mello MA, Gomes-Marcondes MC. Leucine-rich diet alters the eukaryotic translation initiation factors expression in skeletal muscle of tumour-bearing rats. BMC Cancer. (2007) 7:42. doi: 10.1186/1471-2407-7-42
23. Cruz B, Gomes-Marcondes MC. Leucine-rich diet supplementation modulates foetal muscle protein metabolism impaired by Walker-256 tumour. Reproduct Biol Endocrinol. (2014) 12:2. doi: 10.1186/1477-7827-12-2
24. Viana LR, Canevarolo R, Luiz AC, Soares RF, Lubaczeuski C, Zeri AC, et al. Leucine-rich diet alters the (1)H-NMR based metabolomic profile without changing the Walker-256 tumour mass in rats. BMC Cancer. (2016) 16:764. doi: 10.1186/s12885-016-2811-2
25. Cruz B, Oliveira A, Gomes-Marcondes MCC. L-leucine dietary supplementation modulates muscle protein degradation and increases pro-inflammatory cytokines in tumour-bearing rats. Cytokine. (2017) 96:253–60. doi: 10.1016/j.cyto.2017.04.019
26. Ventrucci G, Ramos Silva LG, Roston Mello MA, Gomes Marcondes MC. Effects of a leucine-rich diet on body composition during nutritional recovery in rats. Nutrition. (2004) 20:213–7. doi: 10.1016/j.nut.2003.10.014
27. Gomes-Marcondes MC, Ventrucci G, Toledo MT, Cury L, Cooper JC. A leucine-supplemented diet improved protein content of skeletal muscle in young tumor-bearing rats. Brazilian J Med Biol Res. (2003) 36:1589–94. doi: 10.1590/S0100-879X2003001100017
28. Nissen SL, Abumrad NN. Nutritional role of the leucine metabolite β-hydroxy β-methylbutyrate (HMB). J Nutr Biochem. (1997) 8:300–11. doi: 10.1016/S0955-2863(97)00048-X
29. Van Koevering M, Nissen S. Oxidation of leucine and alpha-ketoisocaproate to beta-hydroxy-beta-methylbutyrate in vivo. Am J Physiol. (1992) 262(1 Pt 1):E27–31. doi: 10.1152/ajpendo.1992.262.1.E27
30. Wilson GJ, Wilson JM, Manninen AH. Effects of beta-hydroxy-beta-methylbutyrate (HMB) on exercise performance and body composition across varying levels of age, sex, and training experience: a review. Nutr Metab. (2008) 5:1. doi: 10.1186/1743-7075-5-1
31. Mirza KA, Pereira SL, Voss AC, Tisdale MJ. Comparison of the anticatabolic effects of leucine and Ca-beta-hydroxy-beta-methylbutyrate in experimental models of cancer cachexia. Nutrition. (2014) 30:807–13. doi: 10.1016/j.nut.2013.11.012
32. Smith HJ, Mukerji P, Tisdale MJ. Attenuation of proteasome-induced proteolysis in skeletal muscle by {beta}-hydroxy-{beta}-methylbutyrate in cancer-induced muscle loss. Cancer Res. (2005) 65:277–83.
33. Nunes EA, Kuczera D, Brito GA, Bonatto SJ, Yamazaki RK, Tanhoffer RA, et al. Beta-hydroxy-beta-methylbutyrate supplementation reduces tumor growth and tumor cell proliferation ex vivo and prevents cachexia in Walker 256 tumor-bearing rats by modifying nuclear factor-kappaB expression. Nutr Res. (2008) 28:487–93. doi: 10.1016/j.nutres.2008.04.006
34. Aversa Z, Bonetto A, Costelli P, Minero VG, Penna F, Baccino FM, et al. beta-hydroxy-beta-methylbutyrate (HMB) attenuates muscle and body weight loss in experimental cancer cachexia. Int J Oncol. (2011) 38:713–20. doi: 10.3892/ijo.2010.885
35. Bode BP, Fischer C, Abcouwer S, Wasa M, Souba WW. Glutamine and Cancer Cachexia. Protein and Amino Acid Metabolism in Cancer Cachexia. Berlin: Springer Berlin Heidelberg (1996). p. 139–70. doi: 10.1007/978-3-662-22346-8_11
36. Newsholme P, Lima MM, Procopio J, Pithon-Curi TC, Doi SQ, Bazotte RB, et al. Glutamine and glutamate as vital metabolites. J Med Biol Res. (2003) 36:153–63. doi: 10.1590/S0100-879X2003000200002
37. Newsholme P, Procopio J, Lima MM, Pithon-Curi TC, Curi R. Glutamine and glutamate–their central role in cell metabolism and function. Cell Biochem Funct. (2003) 21:1–9. doi: 10.1002/cbf.1003
38. Yu JC, Jiang ZM, Li DM. Glutamine: a precursor of glutathione and its effect on liver. World J Gastroenterol. (1999) 5:143–6. doi: 10.3748/wjg.v5.i2.143
39. Fracaro L, Frez F, Silva B, Vicentini G, de Souza S, Martins H, et al. Walker 256 tumor-bearing rats demonstrate altered interstitial cells of Cajal. Effects on ICC in the Walker 256 tumor model. Neurogastroenterol Motility. (2016) 28:101–15. doi: 10.1111/nmo.12702
40. Martins HA, Sehaber CC, Hermes-Uliana C, Mariani FA, Guarnier FA, Vicentini GE, et al. Supplementation with l-glutamine prevents tumor growth and cancer-induced cachexia as well as restores cell proliferation of intestinal mucosa of Walker-256 tumor-bearing rats. Amino Acids. (2016) 48:2773–84. doi: 10.1007/s00726-016-2313-1
41. Martins HA, Bazotte RB, Vicentini GE, Lima MM, Guarnier FA, Hermes-Uliana C, et al. l-Glutamine supplementation promotes an improved energetic balance in Walker-256 tumor-bearing rats. Tumour Biol. (2017) 39:1010428317695960. doi: 10.1177/1010428317695960
42. Vicentini GE, Fracaro L, de Souza SR, Martins HA, Guarnier FA, Zanoni JN. Experimental cancer cachexia changes neuron numbers and peptide levels in the intestine: partial protective effects after dietary supplementation with L-Glutamine. PLoS ONE. (2016) 11:e0162998. doi: 10.1371/journal.pone.0162998
43. Vicentini GE, Martins HA, Fracaro L, de Souza SRG, da Silva Zanoni KP, Silva TNX, et al. Does l-glutamine-supplemented diet extenuate NO-mediated damage on myenteric plexus of Walker 256 tumor-bearing rats? Food Res Int. (2017) 101:24–34. doi: 10.1016/j.foodres.2017.08.054
44. Lima MMR, de Mello MAR, Curi R. Walker 256 tumour growth causes marked changes of glutamine metabolism in rat small intestine. Cell Biochem Funct. (2002) 20:107–13. doi: 10.1002/cbf.961
45. Zhong Z, Wheeler MD, Li X, Froh M, Schemmer P, Yin M, et al. L-Glycine: a novel antiinflammatory, immunomodulatory, and cytoprotective agent. Curr Opin Clin Nutr Metabolic Care. (2003) 6:229–40. doi: 10.1097/00075197-200303000-00013
46. Rose ML, Cattley RC, Dunn C, Wong V, Li X, Thurman RG. Dietary glycine prevents the development of liver tumors caused by the peroxisome proliferator WY-14,643. Carcinogenesis. (1999) 20:2075–81. doi: 10.1093/carcin/20.11.2075
47. Rose ML, Madren J, Bunzendahl H, Thurman RG. Dietary glycine inhibits the growth of B16 melanoma tumors in mice. Carcinogenesis. (1999) 20:793–8. doi: 10.1093/carcin/20.5.793
48. Ham DJ, Murphy KT, Chee A, Lynch GS, Koopman R. Glycine administration attenuates skeletal muscle wasting in a mouse model of cancer cachexia. Clin Nutr. (2014) 33:448–58. doi: 10.1016/j.clnu.2013.06.013
49. Morris SM Jr. Regulation of enzymes of urea and arginine synthesis. Annu Rev Nutr. (1992) 12:81–101. doi: 10.1146/annurev.nu.12.070192.000501
50. Delage B, Fennell DA, Nicholson L, McNeish I, Lemoine NR, Crook T, et al. Arginine deprivation and argininosuccinate synthetase expression in the treatment of cancer. Int J Cancer. (2010) 126:2762–72. doi: 10.1002/ijc.25202
51. Crowell JA, Steele VE, Sigman CC, Fay JR. Is inducible nitric oxide synthase a target for chemoprevention? Mol Cancer Ther. (2003) 2:815–23.
52. Szende B, Tyihák E, Trézl L. Role of arginine and its methylated derivatives in cancer biology and treatment. Cancer Cell Int. (2001) 1:3. doi: 10.1186/1475-2867-1-3
53. Yerushalmi HF, Besselsen DG, Ignatenko NA, Blohm-Mangone KA, Padilla-Torres JL, Stringer DE, et al. Role of polyamines in arginine-dependent colon carcinogenesis in ApcMin/+ mice. Mol Carcinogen. (2006) 45:764–73. doi: 10.1002/mc.20246
54. Carreiro AL, Buhman KK. Absorption of Dietary Fat and Its Metabolism in Enterocytes. In: Patel VB, editor. The Molecular Nutrition of Fats. Academic Press (2019). p. 33–48. doi: 10.1016/B978-0-12-811297-7.00003-2
55. Allen BG, Bhatia SK, Anderson CM, Eichenberger-Gilmore JM, Sibenaller ZA, Mapuskar KA, et al. Ketogenic diets as an adjuvant cancer therapy: history and potential mechanism. Redox Biol. (2014) 2:963–70. doi: 10.1016/j.redox.2014.08.002
56. Liberti MV, Locasale JW. The warburg effect: how does it benefit cancer cells? Trends Biochem Sci. (2016) 41:211–8. doi: 10.1016/j.tibs.2015.12.001
57. Tisdale MJ, Brennan RA, Fearon KC. Reduction of weight loss and tumour size in a cachexia model by a high fat diet. Br J Cancer. (1987) 56:39–43. doi: 10.1038/bjc.1987.149
58. Beck SA, Tisdale MJ. Nitrogen excretion in cancer cachexia and its modification by a high fat diet in mice. Cancer Res. (1989) 49:3800–4.
59. Nakamura K, Tonouchi H, Sasayama A, Ashida K. A ketogenic formula prevents tumor progression and cancer cachexia by attenuating systemic inflammation in Colon 26 tumor-bearing mice. Nutrients. (2018) 10. doi: 10.3390/nu10020206
60. Kwiterovich J, Peter O, Vining EPG, Pyzik P, Skolasky J, Richard, Freeman JM. Effect of a high-fat ketogenic diet on plasma levels of lipids, lipoproteins, and apolipoproteins in children. JAMA. (2003) 290:912–20. doi: 10.1001/jama.290.7.912
61. Creighton BC, Hyde PN, Maresh CM, Kraemer WJ, Phinney SD, Volek JS. Paradox of hypercholesterolaemia in highly trained, keto-adapted athletes. BMJ Open Sport Exercise Med. (2018) 4:e000429. doi: 10.1136/bmjsem-2018-000429
62. Groesbeck DK, Bluml RM, Kossoff EH. Long-term use of the ketogenic diet in the treatment of epilepsy. Dev Med Child Neurol. (2006) 48:978–81. doi: 10.1111/j.1469-8749.2006.tb01269.x
63. Tisdale MJ. Mechanisms of cancer cachexia. Physiol Rev. (2009) 89:381–410. doi: 10.1152/physrev.00016.2008
64. Das SK, Hoefler G. The role of triglyceride lipases in cancer associated cachexia. Trends Mol Med. (2013) 19:292–301. doi: 10.1016/j.molmed.2013.02.006
65. Das SK, Eder S, Schauer S, Diwoky C, Temmel H, Guertl B, et al. Adipose triglyceride lipase contributes to cancer-associated cachexia. Science. (2011) 333:233–8. doi: 10.1126/science.1198973
66. Stephens NA, Skipworth RJ, Macdonald AJ, Greig CA, Ross JA, Fearon KC. Intramyocellular lipid droplets increase with progression of cachexia in cancer patients. J Cachexia Sarcopenia Muscle. (2011) 2:111–7. doi: 10.1007/s13539-011-0030-x
67. Fernandes G, Venkatraman JT. Role of omega-3 fatty acids in health and disease. Nutr Res. (1993) 13:S19–45. doi: 10.1016/S0271-5317(05)80282-9
68. Sijben JW, Calder PC. Differential immunomodulation with long-chain n-3 PUFA in health and chronic disease. Proc Nutr Soc. (2007) 66:237–59. doi: 10.1017/S0029665107005472
69. Yates CM, Calder PC, Ed Rainger G. Pharmacology and therapeutics of omega-3 polyunsaturated fatty acids in chronic inflammatory disease. Pharmacol Therapeut. (2014) 141:272–82. doi: 10.1016/j.pharmthera.2013.10.010
70. Tisdale MJ, Dhesi JK. Inhibition of weight loss by omega-3 fatty acids in an experimental cachexia model. Cancer Res. (1990) 50:5022–6.
71. Pizato N, Bonatto S, Yamazaki RK, Aikawa J, Nogata C, Mund RC, et al. Ratio of n6 to n-3 fatty acids in the diet affects tumor growth and cachexia in Walker 256 tumor-bearing rats. Nutr Cancer. (2005) 53:194–201. doi: 10.1207/s15327914nc5302_8
72. Coelho I, Casare F, Pequito DC, Borghetti G, Yamazaki RK, Brito GA, et al. Fish oil supplementation reduces cachexia and tumor growth while improving renal function in tumor-bearing rats. Lipids. (2012) 47:1031–41. doi: 10.1007/s11745-012-3715-9
73. Togni V, Ota CC, Folador A, Junior OT, Aikawa J, Yamazaki RK, et al. Cancer cachexia and tumor growth reduction in Walker 256 tumor-bearing rats supplemented with N-3 polyunsaturated fatty acids for one generation. Nutrit Cancer. (2003) 46:52–8. doi: 10.1207/S15327914NC4601_07
74. Fernandez R, Piechnik J, Fabris R, Malnic G, Fernandes LC. Effect of chronic fish oil supplementation on renal function of normal and cachectic rats. Brazil J Med Biol Res. (2004) 37:1481–9. doi: 10.1590/S0100-879X2004001000006
75. Dumas JF, Goupille C, Pinault M, Fandeur L, Bougnoux P, Servais S, et al. n-3 PUFA-enriched diet delays the occurrence of cancer cachexia in rat with peritoneal carcinosis. Nutr Cancer. (2010) 62:343–50. doi: 10.1080/01635580903407080
76. Iagher F, de Brito Belo SR, Naliwaiko K, Franzoi AM, de Brito GA, Yamazaki RK, et al. Chronic supplementation with shark liver oil for reducing tumor growth and cachexia in walker 256 tumor-bearing rats. Nutr Cancer. (2011) 63:1307–15. doi: 10.1080/01635581.2011.607540
77. Iagher F, de Brito Belo SR, Souza WM, Nunes JR, Naliwaiko K, Sassaki GL, et al. Antitumor and anti-cachectic effects of shark liver oil and fish oil: comparison between independent or associative chronic supplementation in Walker 256 tumor-bearing rats. Lipids Health Dis. (2013) 12:146. doi: 10.1186/1476-511X-12-146
78. Beck SA, Smith KL, Tisdale MJ. Anticachectic and antitumor effect of eicosapentaenoic acid and its effect on protein turnover. Cancer Res. (1991) 51:6089–93.
79. Tisdale MJ, Beck SA. Inhibition of tumour-induced lipolysis in vitro and cachexia and tumour growth in vivo by eicosapentaenoic acid. Biochem Pharmacol. (1991) 41:103–7. doi: 10.1016/0006-2952(91)90016-X
80. Hudson EA, Tisdale MJ. Comparison of the effectiveness of eicosapentaenoic acid administered as either the free acid or ethyl ester as an anticachectic and antitumour agent. Prostaglandins Leukotr Essential Fatty Acids. (1994) 51:141–5. doi: 10.1016/0952-3278(94)90090-6
81. Whitehouse AS, Smith HJ, Drake JL, Tisdale MJ. Mechanism of attenuation of skeletal muscle protein catabolism in cancer cachexia by eicosapentaenoic acid. Cancer Res. (2001) 61:3604–9.
82. Smith HJ, Greenberg NA, Tisdale MJ. Effect of eicosapentaenoic acid, protein and amino acids on protein synthesis and degradation in skeletal muscle of cachectic mice. Br J Cancer. (2004) 91:408–12. doi: 10.1038/sj.bjc.6601981
83. Du L, Yang YH, Wang YM, Xue CH, Kurihara H, Takahashi K. EPA-enriched phospholipids ameliorate cancer-associated cachexia mainly via inhibiting lipolysis. Food Funct. (2015) 6:3652–62. doi: 10.1039/C5FO00478K
84. Fini L, Piazzi G, Ceccarelli C, Daoud Y, Belluzzi A, Munarini A, et al. Highly purified eicosapentaenoic acid as free fatty acids strongly suppresses polyps in Apc(Min/+) mice. Clin Cancer Res. (2010) 16:5703–11. doi: 10.1158/1078-0432.CCR-10-1990
85. Jho DH, Babcock TA, Tevar R, Helton WS, Espat NJ. Eicosapentaenoic acid supplementation reduces tumor volume and attenuates cachexia in a rat model of progressive non-metastasizing malignancy. J Parenteral Enteral Nutr. (2002) 26:291–7. doi: 10.1177/0148607102026005291
86. Dagnelie PC, Bell JD, Williams SC, Bates TE, Abel PD, Foster CS. Effect of fish oil on cancer cachexia and host liver metabolism in rats with prostate tumors. Lipids. (1994) 29:195–203. doi: 10.1007/BF02536729
87. Russell ST, Tisdale MJ. Effect of eicosapentaenoic acid (EPA) on expression of a lipid mobilizing factor in adipose tissue in cancer cachexia. Prostagland Leukotr Essential Fatty Acids. (2005) 72:409–14. doi: 10.1016/j.plefa.2005.03.002
88. Pariza MW, Ha YL. Conjugated dienoic derivatives of linoleic acid: a new class of anticarcinogens. Med Oncol Tumor Pharmacother. (1990) 7:169–71.
89. Lee KW, Lee HJ, Cho HY, Kim YJ. Role of the conjugated linoleic acid in the prevention of cancer. Crit Rev Food Sci Nutr. (2005) 45:135–44. doi: 10.1080/10408690490911800
90. Ip MM, Masso-Welch PA, Ip C. Prevention of mammary cancer with conjugated linoleic acid: role of the stroma and the epithelium. J Mammary Gland Biol Neoplasia. (2003) 8:103–18. doi: 10.1023/A:1025739506536
91. Pariza MW, Park Y, Cook ME. Conjugated linoleic acid and the control of cancer and obesity. Toxicol Sci. (1999) 52(2 Suppl):107–10. doi: 10.1093/toxsci/52.2.107
92. Yang M, Cook ME. Dietary conjugated linoleic acid decreased cachexia, macrophage tumor necrosis factor-alpha production, and modifies splenocyte cytokines production. Exp Biol Med. (2003) 228:51–8. doi: 10.1177/153537020322800107
93. Graves E, Hitt A, Pariza MW, Cook ME, McCarthy DO. Conjugated linoleic acid preserves gastrocnemius muscle mass in mice bearing the colon-26 adenocarcinoma. Res Nursing Health. (2005) 28:48–55. doi: 10.1002/nur.20052
94. McCarthy DO, Graves E. Conjugated linoleic acid preserves muscle mass in mice bearing the Lewis lung carcinoma, but not the B16 melanoma. Res Nursing Health. (2006) 29:98–104. doi: 10.1002/nur.20115
95. Tian M, Kliewer KL, Asp ML, Stout MB, Belury MA. c9t11-Conjugated linoleic acid-rich oil fails to attenuate wasting in colon-26 tumor-induced late-stage cancer cachexia in male CD2F1 mice. Mol Nutrit Food Res. (2011) 55:268–77. doi: 10.1002/mnfr.201000176
96. Gonçalves DC, Lira FS, Yamashita AS, Carnevali Junior LC, Eder R, Laviano A, et al. Liver lipid metabolism disruption in cancer cachexia is aggravated by cla supplementation -induced inflammation. Clin Nutr. (2019) 38:2219–30. doi: 10.1016/j.clnu.2018.09.023
97. Bremer J. Carnitine–metabolism and functions. Physiol Rev. (1983) 63:1420–80. doi: 10.1152/physrev.1983.63.4.1420
98. Longo N, Frigeni M, Pasquali M. Carnitine transport and fatty acid oxidation. Biochim Biophys Acta. (2016) 1863:2422–35. doi: 10.1016/j.bbamcr.2016.01.023
99. Busquets S, Serpe R, Toledo M, Betancourt A, Marmonti E, Orpi M, et al. L-Carnitine: an adequate supplement for a multi-targeted anti-wasting therapy in cancer. Clin Nutr. (2012) 31:889–95. doi: 10.1016/j.clnu.2012.03.005
100. Busquets S, Perez-Peiro M, Salazar-Degracia A, Argiles JM, Serpe R, Rojano-Toimil A, et al. Differential structural features in soleus and gastrocnemius of carnitine-treated cancer cachectic rats. J Cell Physiol. (2020) 235:526–37. doi: 10.1002/jcp.28992
101. Liu S, Wu H-J, Zhang Z-Q, Chen Q, Liu B, Wu J-P, et al. L-carnitine ameliorates cancer cachexia in mice by regulating the expression and activity of carnitine palmityl transferase. Cancer Biol Ther. (2011) 12:125–30. doi: 10.4161/cbt.12.2.15717
102. Silverio R, Laviano A, Rossi Fanelli F, Seelaender M. L-Carnitine induces recovery of liver lipid metabolism in cancer cachexia. Amino Acids. (2012) 42:1783–92. doi: 10.1007/s00726-011-0898-y
103. Wallimann T, Tokarska-Schlattner M, Schlattner U. The creatine kinase system and pleiotropic effects of creatine. Amino Acids. (2011) 40:1271–96. doi: 10.1007/s00726-011-0877-3
104. Gualano B, Roschel H, Lancha-Jr AH, Brightbill CE, Rawson ES. In sickness and in health: the widespread application of creatine supplementation. Amino Acids. (2012) 43:519–29. doi: 10.1007/s00726-011-1132-7
105. Harris RC, Söderlund K, Hultman E. Elevation of creatine in resting and exercised muscle of normal subjects by creatine supplementation. Clin Sci. (1992) 83:367–74. doi: 10.1042/cs0830367
106. Terjung RL, Clarkson P, Eichner ER, Greenhaff PL, Hespel PJ, Israel RG, et al. American college of sports medicine roundtable. The physiological and health effects of oral creatine supplementation. Med Sci Sports Exerc. (2000) 32:706–17. doi: 10.1097/00005768-200003000-00024
107. Buford TW, Kreider RB, Stout JR, Greenwood M, Campbell B, Spano M, et al. International Society of Sports Nutrition position stand: creatine supplementation and exercise. J Int Soc Sports Nutr. (2007) 4:6. doi: 10.1186/1550-2783-4-6
108. Louis M, Lebacq J, Poortmans JR, Belpaire-Dethiou MC, Devogelaer JP, Van Hecke P, et al. Beneficial effects of creatine supplementation in dystrophic patients. Muscle Nerve. (2003) 27:604–10. doi: 10.1002/mus.10355
109. Chung Yl, Alexanderson H, Pipitone N, Morrison C, Dastmalchi M, Ståhl-Hallengren C, et al. Creatine supplements in patients with idiopathic inflammatory myopathies who are clinically weak after conventional pharmacologic treatment: six-month, double-blind, randomized, placebo-controlled trial. Arthritis Care Res. (2007) 57:694–702. doi: 10.1002/art.22687
110. Kley R, Tarnopolsky M, Vorgerd M. Creatine treatment in muscle disorders: a meta-analysis of randomised controlled trials. J Neurol Neurosurg Psychiatr. (2008) 79:366–7. doi: 10.1136/jnnp.2007.127571
111. Santos R, Bassit R, Caperuto E, Rosa LC. The effect of creatine supplementation upon inflammatory and muscle soreness markers after a 30km race. Life Sci. (2004) 75:1917–24. doi: 10.1016/j.lfs.2003.11.036
112. Bassit R, Curi R, Rosa LC. Creatine supplementation reduces plasma levels of pro-inflammatory cytokines and PGE 2 after a half-ironman competition. Amino Acids. (2008) 35:425–31. doi: 10.1007/s00726-007-0582-4
113. Campos-Ferraz PL, Gualano B, das Neves W, Andrade IT, Hangai I, Pereira RT. Exploratory studies of the potential anti-cancer effects of creatine. Amino Acids. (2016) 48:1993–2001. doi: 10.1007/s00726-016-2180-9
114. Cella PS, Marinello PC, Borges FH, Ribeiro DF, Chimin P, Testa MTJ, et al. Creatine supplementation in Walker-256 tumor-bearing rats prevents skeletal muscle atrophy by attenuating systemic inflammation and protein degradation signaling. Eur J Nutr. (2020) 59:661–9. doi: 10.1007/s00394-019-01933-6
115. Deminice R, Cella PS, Padilha CS, Borges FH, da Silva LE, Campos-Ferraz PL, et al. Creatine supplementation prevents hyperhomocysteinemia, oxidative stress and cancer-induced cachexia progression in Walker-256 tumor-bearing rats. Amino Acids. (2016) 48:2015–24. doi: 10.1007/s00726-016-2172-9
116. Kumar S, Pandey AK. Chemistry and biological activities of flavonoids: an overview. Sci World J. (2013) 2013:162750. doi: 10.1155/2013/162750
117. Velázquez KT, Enos RT, Narsale AA, Puppa MJ, Davis JM, Murphy EA, et al. Quercetin supplementation attenuates the progression of cancer cachexia in ApcMin/+ mice. J Nutrit. (2014) 144:868–75. doi: 10.3945/jn.113.188367
118. Camargo CA, da Silva ME, da Silva RA, Justo GZ, Gomes-Marcondes MC, Aoyama H. Inhibition of tumor growth by quercetin with increase of survival and prevention of cachexia in Walker 256 tumor-bearing rats. Biochem Biophys Res Commun. (2011) 406:638–42. doi: 10.1016/j.bbrc.2011.02.111
119. Wang H, Lai YJ, Chan YL, Li TL, Wu CJ. Epigallocatechin-3-gallate effectively attenuates skeletal muscle atrophy caused by cancer cachexia. Cancer Lett. (2011) 305:40–9. doi: 10.1016/j.canlet.2011.02.023
120. Hirasaka K, Saito S, Yamaguchi S, Miyazaki R, Wang Y, Haruna M, et al. Dietary Supplementation with isoflavones prevents muscle wasting in tumor-bearing mice. J Nutrit Sci Vitaminol. (2016) 62:178–84. doi: 10.3177/jnsv.62.178
121. Yoshimura T, Saitoh K, Sun L, Wang Y, Taniyama S, Yamaguchi K, et al. Morin suppresses cachexia-induced muscle wasting by binding to ribosomal protein S10 in carcinoma cells. Biochem Biophys Res Commun. (2018) 506:773–9. doi: 10.1016/j.bbrc.2018.10.184
122. Sales JM, Resurreccion AV. Resveratrol in peanuts. Crit Rev Food Sci Nutr. (2014) 54:734–70. doi: 10.1080/10408398.2011.606928
123. Carbó N, Costelli P, Baccino FM, López-Soriano FJ, Argilés JM. Resveratrol, a natural product present in wine, decreases tumour growth in a rat tumour model. Biochem Biophys Res Commun. (1999) 254:739–43. doi: 10.1006/bbrc.1998.9916
124. Wyke SM, Russell ST, Tisdale MJ. Induction of proteasome expression in skeletal muscle is attenuated by inhibitors of NF-kappaB activation. Br J Cancer. (2004) 91:1742–50. doi: 10.1038/sj.bjc.6602165
125. Shadfar S, Couch ME, McKinney KA, Weinstein LJ, Yin X, Rodriguez JE, et al. Oral resveratrol therapy inhibits cancer-induced skeletal muscle and cardiac atrophy in vivo. Nutr Cancer. (2011) 63:749–62. doi: 10.1080/01635581.2011.563032
126. Busquets S, Fuster G, Ametller E, Olivan M, Figueras M, Costelli P, et al. Resveratrol does not ameliorate muscle wasting in different types of cancer cachexia models. Clin Nutr. (2007) 26:239–44. doi: 10.1016/j.clnu.2006.12.001
127. Sergides C, Chirilă M, Silvestro L, Pitta D, Pittas A. Bioavailability and safety study of resveratrol 500 mg tablets in healthy male and female volunteers. Exp Ther Med. (2016) 11:164–70. doi: 10.3892/etm.2015.2895
128. Almeida L, Vaz-da-Silva M, Falcão A, Soares E, Costa R, Loureiro AI, et al. Pharmacokinetic and safety profile of trans-resveratrol in a rising multiple-dose study in healthy volunteers. Mol Nutr Food Res. (2009) 53(Suppl 1):S7–15. doi: 10.1002/mnfr.200800177
129. Roberfroid M. Prebiotics: the concept revisited. J Nutr. (2007) 137:830S-7S. doi: 10.1093/jn/137.3.830S
130. Vos AP, Haarman M, Buco A, Govers M, Knol J, Garssen J, et al. A specific prebiotic oligosaccharide mixture stimulates delayed-type hypersensitivity in a murine influenza vaccination model. Int Immunopharmacol. (2006) 6:1277–86. doi: 10.1016/j.intimp.2006.03.010
131. Vos AP, M'Rabet L, Stahl B, Boehm G, Garssen J. Immune-modulatory effects and potential working mechanisms of orally applied nondigestible carbohydrates. Crit Rev Immunol. (2007) 27:97–140. doi: 10.1615/CritRevImmunol.v27.i2.10
132. Bruzzese E, Volpicelli M, Squeglia V, Bruzzese D, Salvini F, Bisceglia M, et al. A formula containing galacto- and fructo-oligosaccharides prevents intestinal and extra-intestinal infections: an observational study. Clin Nutr. (2009) 28:156–61. doi: 10.1016/j.clnu.2009.01.008
133. Xiao L, van't Land B, van de Worp WRPH, Stahl B, Folkerts G, Garssen J. Early-life nutritional factors and mucosal immunity in the development of autoimmune diabetes. Front Immunol. (2017) 8:1219. doi: 10.3389/fimmu.2017.01219
134. den Besten G, van Eunen K, Groen AK, Venema K, Reijngoud DJ, Bakker BM. The role of short-chain fatty acids in the interplay between diet, gut microbiota, and host energy metabolism. J Lipid Res. (2013) 54:2325–40. doi: 10.1194/jlr.R036012
135. Bindels LB, Neyrinck AM, Claus SP, Le Roy CI, Grangette C, Pot B, et al. Synbiotic approach restores intestinal homeostasis and prolongs survival in leukaemic mice with cachexia. ISME J. (2016) 10:1456–70. doi: 10.1038/ismej.2015.209
136. Gorselink M, van Helvoort ALB, Hageman RJJ, inventors, Nutricia NV. Use of Dietary Fibres Against Muscle Wasting (2006).
137. Bindels LB, Neyrinck AM, Salazar N, Taminiau B, Druart C, Muccioli GG, et al. Non digestible oligosaccharides modulate the gut microbiota to control the development of leukemia and associated cachexia in mice. PLoS ONE. (2015) 10:e0131009. doi: 10.1371/journal.pone.0131009
138. van Norren K, Kegler D, Argiles JM, Luiking Y, Gorselink M, Laviano A, et al. Dietary supplementation with a specific combination of high protein, leucine, and fish oil improves muscle function and daily activity in tumour-bearing cachectic mice. Br J Cancer. (2009) 100:713–22. doi: 10.1038/sj.bjc.6604905
139. Faber J, Vos P, Kegler D, van Norren K, Argiles JM, Laviano A, et al. Beneficial immune modulatory effects of a specific nutritional combination in a murine model for cancer cachexia. Br J Cancer. (2008) 99:2029–36. doi: 10.1038/sj.bjc.6604785
140. Faber J, van Limpt K, Kegler D, Luiking Y, Garssen J, van Helvoort A, et al. Bacterial translocation is reduced by a specific nutritional combination in mice with chemotherapy-induced neutropenia. J Nutr. (2011) 141:1292–8. doi: 10.3945/jn.110.136986
141. Awa H, Futamura A, Higashiguchi T, Ito A, Mori N, Murai M, et al. Effects of combined treatment with branched-chain amino acids, citric acid, L-Carnitine, Coenzyme Q10, Zinc, and various vitamins in tumor-bearing mice. Biol Pharmaceut Bull. (2017) 40:266–71. doi: 10.1248/bpb.b16-00638
142. Liu YM, Chan YL, Wu TH, Li TL, Hsia S, Chiu YH, et al. Antitumor, inhibition of metastasis and radiosensitizing effects of total nutrition formula on lewis tumor-bearing mice. Nutrients. (2019) 11. doi: 10.3390/nu11081944
143. Giles K, Guan C, Jagoe TR, Mazurak V. Diet composition as a source of variation in experimental animal models of cancer cachexia. J Cachexia Sarcopenia Muscle. (2016) 7:110–25. doi: 10.1002/jcsm.12058
144. Abramson EC, Kukla LJ, Shevrin DH, Lad TE, McGuire WP, Kukreja SC. A model for malignancy-associated humoral hypercalcemia. Calcified Tissue Int. (1984) 36:563–7. doi: 10.1007/BF02405367
145. The Importance of a Proper Control Diet [press release]. www.ResearchDiets.com (2013).
146. Suzuki T, Von Haehling S, Springer J. Promising models for cancer-induced cachexia drug discovery. Exp Opin Drug Discov. (2020) 15:627–37. doi: 10.1080/17460441.2020.1724954
147. Michaelis KA, Zhu X, Burfeind KG, Krasnow SM, Levasseur PR, Morgan TK, et al. Establishment and characterization of a novel murine model of pancreatic cancer cachexia. J Cachexia Sarcopenia Muscle. (2017) 8:824–38. doi: 10.1002/jcsm.12225
148. Kirsch DG, Grimm J, Guimaraes AR, Wojtkiewicz GR, Perez BA, Santiago PM, et al. Imaging primary lung cancers in mice to study radiation biology. Int J Radiat Oncol Biol Phys. (2010) 76:973–7. doi: 10.1016/j.ijrobp.2009.11.038
149. Iglesias VS, Hoof SJv, Vaniqui A, Schyns LE, Lieuwes N, Yaromina A, et al. An orthotopic non-small cell lung cancer model for image-guided small animal radiotherapy platforms. Br J Radiol. (2019) 92:20180476. doi: 10.1259/bjr.20180476
150. Park SS, Chunta JL, Robertson JM, Martinez AA, Oliver Wong CY, Amin M, et al. MicroPET/CT imaging of an orthotopic model of human glioblastoma multiforme and evaluation of pulsed low-dose irradiation. Int J Radiat Oncol Biol Phys. (2011) 80:885–92. doi: 10.1016/j.ijrobp.2011.01.045
151. van der Heyden B, van de Worp WR, van Helvoort A, Theys J, Schols AM, Langen RC, et al. Automated CT-derived skeletal muscle mass determination in lower hind limbs of mice using a 3D U-Net deep learning network. J Appl Physiol. (2020) 128:42–9. doi: 10.1152/japplphysiol.00465.2019
152. Op den Kamp CM, De Ruysscher DK, van den Heuvel M, Elferink M, Houben RM, Oberije CJ, et al. Early body weight loss during concurrent chemo-radiotherapy for non-small cell lung cancer. J Cachexia Sarcopenia Muscle. (2014) 5:127–37. doi: 10.1007/s13539-013-0127-5
153. Sanders KJC, Hendriks LE, Troost EGC, Bootsma GP, Houben RMA, Schols AMWJ, et al. Early Weight loss during chemoradiotherapy has a detrimental impact on outcome in NSCLC. J Thoracic Oncol. (2016) 11:873–9. doi: 10.1016/j.jtho.2016.02.013
154. Le Bricon T, Gugins S, Cynober L, Baracos VE. Negative impact of cancer chemotherapy on protein metabolism in healthy and tumor-bearing rats. Metabolism. (1995) 44:1340–8. doi: 10.1016/0026-0495(95)90040-3
155. Damrauer JS, Stadler ME, Acharyya S, Baldwin AS, Couch ME, Guttridge DC. Chemotherapy-induced muscle wasting: association with NF-κB and cancer cachexia. Eur J Transl Myol. (2018) 28:7590. doi: 10.4081/ejtm.2018.7590
156. Pin F, Barreto R, Couch ME, Bonetto A, O'Connell TM. Cachexia induced by cancer and chemotherapy yield distinct perturbations to energy metabolism. J Cachexia Sarcopenia Muscle. (2019) 10:140–54. doi: 10.1002/jcsm.12360
157. Murphy RA, Mourtzakis M, Chu QS, Baracos VE, Reiman T, Mazurak VC. Supplementation with fish oil increases first-line chemotherapy efficacy in patients with advanced nonsmall cell lung cancer. Cancer. (2011) 117:3774–80. doi: 10.1002/cncr.25933
158. Savarese DMF, Savy G, Vahdat L, Wischmeyer PE, Corey B. Prevention of chemotherapy and radiation toxicity with glutamine. Cancer Treat Rev. (2003) 29:501–13. doi: 10.1016/S0305-7372(03)00133-6
159. Penna F, Busquets S, Pin F, Toledo M, Baccino FM, López-Soriano FJ, et al. Combined approach to counteract experimental cancer cachexia: eicosapentaenoic acid and training exercise. J Cachexia Sarcopenia Muscle. (2011) 2:95–104. doi: 10.1007/s13539-011-0028-4
160. Deuster P, Morrison S, Ahrens R. Endurance exercise modifies cachexia of tumor growth in rats. Med Sci Sports Exerc. (1985) 17:385–92. doi: 10.1249/00005768-198506000-00015
161. Salomao EM, Gomes-Marcondes MC. Light aerobic physical exercise in combination with leucine and/or glutamine-rich diet can improve the body composition and muscle protein metabolism in young tumor-bearing rats. J Physiol Biochem. (2012) 68:493–501. doi: 10.1007/s13105-012-0164-0
162. Salomao EM, Toneto AT, Silva GO, Gomes-Marcondes MC. Physical exercise and a leucine-rich diet modulate the muscle protein metabolism in Walker tumor-bearing rats. Nutr Cancer. (2010) 62:1095–104. doi: 10.1080/01635581.2010.492082
163. Hardee JP, Counts BR, Carson JA. Understanding the role of exercise in cancer cachexia therapy. Am J Lifestyle Med. (2019) 13:46–60. doi: 10.1177/1559827617725283
164. Kessels E, Husson O, van der Feltz-Cornelis CM. The effect of exercise on cancer-related fatigue in cancer survivors: a systematic review and meta-analysis. Neuropsychiatr Dis Treat. (2018) 14:479–94. doi: 10.2147/NDT.S150464
Keywords: cachexia, cancer, undernutrition, nutrition, multinutrient, multitarget, multimodal
Citation: van de Worp WRPH, Schols AMWJ, Theys J, van Helvoort A and Langen RCJ (2020) Nutritional Interventions in Cancer Cachexia: Evidence and Perspectives From Experimental Models. Front. Nutr. 7:601329. doi: 10.3389/fnut.2020.601329
Received: 31 August 2020; Accepted: 02 December 2020;
Published: 22 December 2020.
Edited by:
Marilia Seelaender, University of São Paulo, BrazilReviewed by:
Fabio Penna, University of Turin, ItalyMiguel Luiz Batista Júnior, University of Mogi das Cruzes, Brazil
Copyright © 2020 van de Worp, Schols, Theys, van Helvoort and Langen. This is an open-access article distributed under the terms of the Creative Commons Attribution License (CC BY). The use, distribution or reproduction in other forums is permitted, provided the original author(s) and the copyright owner(s) are credited and that the original publication in this journal is cited, in accordance with accepted academic practice. No use, distribution or reproduction is permitted which does not comply with these terms.
*Correspondence: Wouter R. P. H. van de Worp, dy52YW5kZXdvcnBAbWFhc3RyaWNodHVuaXZlcnNpdHkubmw=