- Laboratory of Gastrointestinal Microbiology, National Center for International Research on Animal Gut Nutrition, Nanjing Agricultural University, Nanjing, China
Introduction: Infection by pathogenic bacteria during weaning is a common cause of diarrhea and intestinal inflammation in piglets. Supplementing the diet with synbiotics is beneficial for animal health. The strain of Lactiplantibacillus plantarum L47 (L47) isolated in our lab exhibited good probiotic properties when combined with inulin. Here, the effectiveness of combining L47 and inulin (CLN) in protecting against enterotoxigenic Escherichia coli (ETEC) induced colon and liver inflammation in weaned piglets was evaluated.
Methods: Twenty-eight piglets aged 21 days were randomly assigned into 4 groups: CON (control), LI47 (oral CLN culture fluid, 1010 CFU/d of L47 and 1 g/d of inulin), ECON (oral ETEC culture fluid, 1010 CFU/d), and ELI47 (oral CLN and ETEC culture fluid). After 24 days, the colon and liver samples were collected for further analysis.
Results and discussion: CLN alleviated colon damage caused by ETEC challenge, as evidenced by an increase of colonic crypt depth, mRNA expression of tight junction Claudin-1 and Occludin, GPX activity, the concentration of IL-10 and sIgA (p < 0.05). Moreover, there was a decrease in MDA activity, the load of E. coli, the concentration of LPS, gene expression of TLR4, and the concentration of TNF-α and IL-6 (p < 0.05) in colonic mucosa. Additionally, CLN counteracted liver damage caused by ETEC challenge by modulating pathways associated with immunity and disease occurrence (p < 0.05).
Conclusion: Supplementing with CLN alleviated colon inflammation induced by ETEC challenge by decreasing the E. coli/LPS/TLR4 pathway and regulating hepatic immune response and disease-related pathways, suggesting that CLN could protect intestinal and liver health in animals.
1 Introduction
Post-weaning diarrhea in piglets presents a significant challenge for intensive pig farms, resulting in substantial economic losses due to high morbidity rates (1, 2). Reports indicate that mortality among piglets affected by post-weaning diarrhea ranges from 20 to 30% (3). Enterotoxigenic Escherichia coli (ETEC) is a pathogen causing diarrhea in piglets, exerting its pathogenic effects by releasing adhesins and enterotoxins (4), thereby instigating intestinal inflammation, compromising intestinal barrier function (5), and consequently reducing gut microbiota diversity (6).
Probiotics are widely used to alleviate post-weaning stress in piglets due to their environmental friendliness, safety, and effectiveness (7, 8). For example, Lactobacillus plantarum has been found to preserve intestinal mucosal barrier function, thereby shielding intestinal epithelia from exogenous stimuli (9). Lactobacillus plantarum LLY-606 restored gut microbiota dysbiosis and reduced inflammation by inhibiting the activation of the TLR4/MYD88/NF-kB signaling pathway (10). Prebiotics are utilized by specific gut microbiota, for example, Bifidobacterium and Lactobacillus metabolize them into acetate and lactate, thereby providing nourishment for other microbes (11, 12). Inulin, a soluble dietary fiber categorized as a prebiotic, is found in the roots and stems of plants such as Asteraceae and Campanulaceae, and is metabolized into short-chain fatty acids (SCFAs) by colon microbes (13). Reports indicate that inulin relies on gut microbiota to alleviate inflammation, enhance intestinal integrity, and boost host immunity (14–16). Liu et al. found that the combination of Lactobacillus rhamnosus and inulin increased the abundance and diversity of the colonic microbiota, enhancing the levels of beneficial bacteria such as Lactobacillus and Alistipes, and alleviating dextran sulfate (DSS) induced ulcerative colitis (17). Ayala-Monter demonstrated that the combined use of inulin and Lactobacillus casei increased the weaning weight of nursing lambs while reducing the abundance of E. coli in fecal samples and the incidence of diarrhea (18). In our previous study with mice, we observed similar results, where the combination of Lactiplantibacillus plantarum L47 and inulin (CLN) increased the production of SCFAs in the colon, decreased the abundance of E. coli, and reduced the expression of inflammatory factors, thereby alleviating DSS-induced colitis (19).
Growing evidence indicates a correlation between gut microbiota dysbiosis and the onset of metabolic liver diseases (20, 21). Lipopolysaccharides (LPS) produced by Gram-negative bacteria can reach the liver and contribute to the development of chronic hepatitis (22). Therefore, we hypothesized that CLN might mitigate damage to the colon and liver by regulating gut microbes in ETEC-challenged weaned piglets, and we aimed to preliminarily explore its mechanisms of action.
2 Materials and methods
This research followed the animal welfare regulations in China for animal experimentation and received authorization from the Animal Ethics Committee at Nanjing Agricultural University (SYXK-2021-0086).
2.1 Preparation of CLN mixture and ETEC culture fluid
The strain of L47, isolated from healthy pig intestines, was preserved in our lab (23). Its probiotic properties and synbiotic effect with inulin in vitro were evaluated in our previous studies (24). After thawing, the strain was inoculated into MRS liquid medium and activated at 37°C for 24 h. This activation process was repeated twice. The concentration of L47 in the activated culture was 1.0 × 109 CFU/mL, then mixed with inulin at a 10:1 (v/w) ratio. Additionally, the E. coli K88 strain CVCC224 was provided by the China Institute of Veterinary Drugs Control, and the concentration of ETEC culture fluid was 1.0 × 109 CFU/mL.
2.2 Animal experimental design
A total of 28 castrated male piglets, aged 21 days and of the Duroc × Landrace × Yorkshire breed (6.80 ± 0.84 kg), were allocated into 4 distinct groups, designated as the CON, LI47, ECON and ELI47 groups. The experimental period was separated into two stages, totaling 24 days, and all piglets were fed a basal diet throughout the experiment. In the first stage (d 0–21), the piglets were orally administrated with 10 mL PBS (CON and ECON group) or a mixture of CLN (LI47 and ELI47 group) daily. In the second stage (d 22–24), the piglets received a single oral administration of 10 mL PBS (CON and LI47 group) or ETEC culture fluid (ECON and ELI47 group) on d 22 of the experiment. The detailed procedure is shown in Figure 1A.
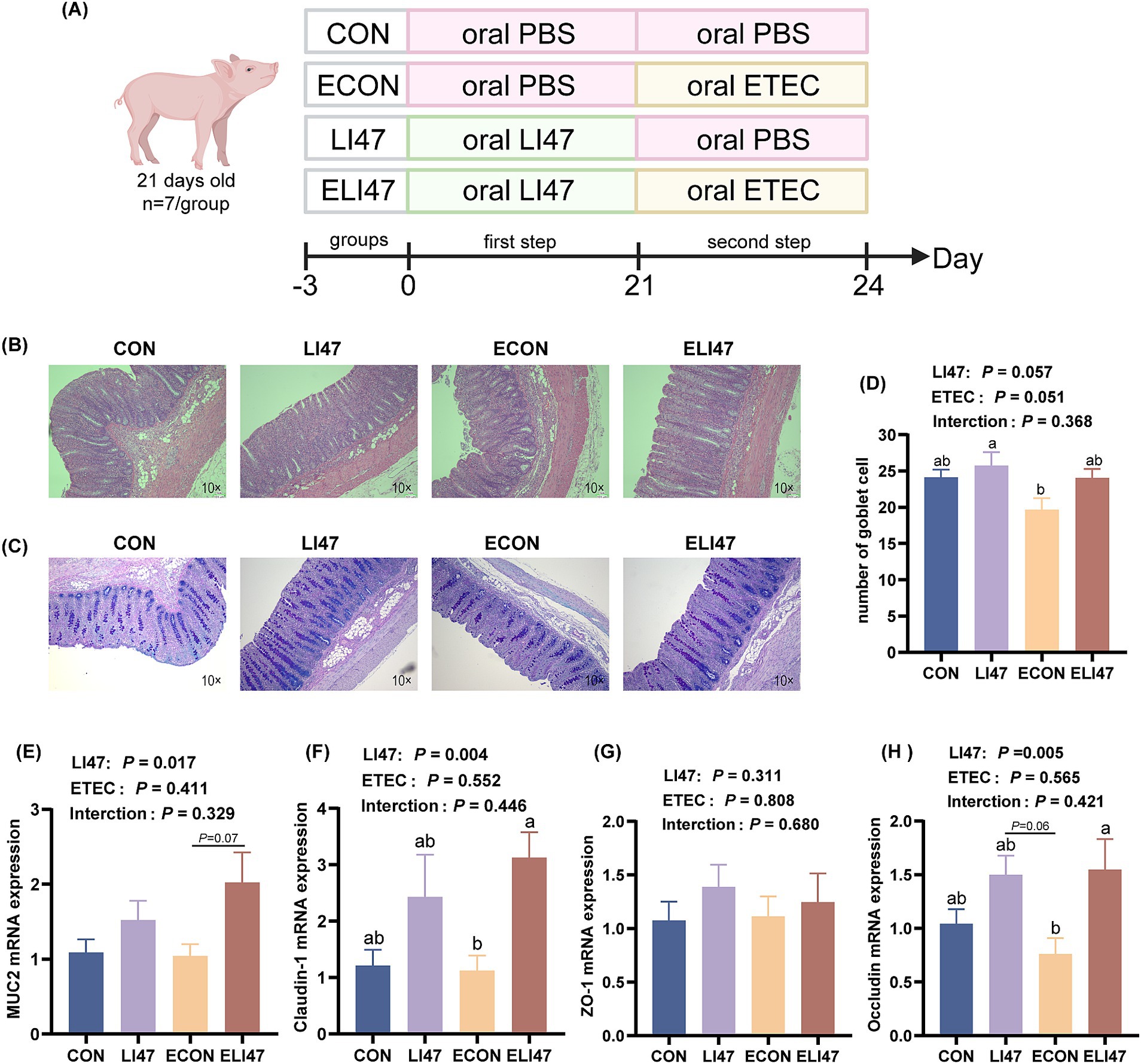
Figure 1. CLN alleviates colon morphology and barrier function damage. (A) Experimental design diagram. Colon tissues stained with (B) H&E and (C) AB-PAS. Scale bar, 100 μm. (D) Number of goblet cells in the colon under each field of view (n = 7). Gene expressions of (E) MUC2, (F) claudin-1, (G) ZO-1 and (H) occluding in the colon mucosa. Results are expressed as mean ± SEM (n = 7), and shoulder markings without the same letter indicate differences (p < 0.05). CON, PBS intervention. LI47, a mixture of CLN intervention. ECON, PBS intervention and challenged by ETEC. ELI47, a mixture of CLN intervention and challenged by ETEC. The p-value of LI47 < 0.05 indicates a significant difference between the groups with CLN treatment (LI47 and ELI47) and those without CLN treatment (CON and ECON). The p-value of ETEC <0.05 indicates a significant difference between the groups treated with ETEC (ECON and ELI47) and those without ETEC (CON and LI74). The p-value of interaction <0.05 indicates a significant interaction between these two factors (CLN and ETEC) (as shown in the following figure).
2.3 Experimental diet and management
All piglets were free to access an antibiotic-free basic diet, which was formulated following the NRC (2012) guidelines to fulfill the nutritional needs of weaning piglets, as detailed in Supplementary Table 1 (25). The nutritional level of dry matter in the diet was measured according to the method described by Zhang (26). The piglets were raised in individual cages with controlled temperatures (27 ± 2°C) and had free access to water.
2.4 Sample collection
The piglets were weighed and slaughtered on d 24 of the experiment. Blood from the neck artery was collected into centrifuge tubes, centrifuged at 4°C (3,500 × g, 10 min) and the serum was carefully transferred to new cryo-tubes.
The entire colon and liver were collected, and their weight and length were measured. A 1.5 cm segment of the middle colon and 1 cm × 1 cm liver tissue sample were carefully dissected using a surgical knife and forceps, then fixed in a 4% paraformaldehyde solution for a minimum of 24 h. The liver tissue and the colonic contents were then collected. The colon tissue was gently rinsed with pre-cooled physiological saline, and the colonic mucosa was collected by scraping it using a glass slide (27, 28).
2.5 Serum biochemistry
The serum samples were prepared and preprocessed according to the protocol of the kit manufacturer (Angle Gene, Nanjing, China) to determine the levels of the target serum markers (Table 1).
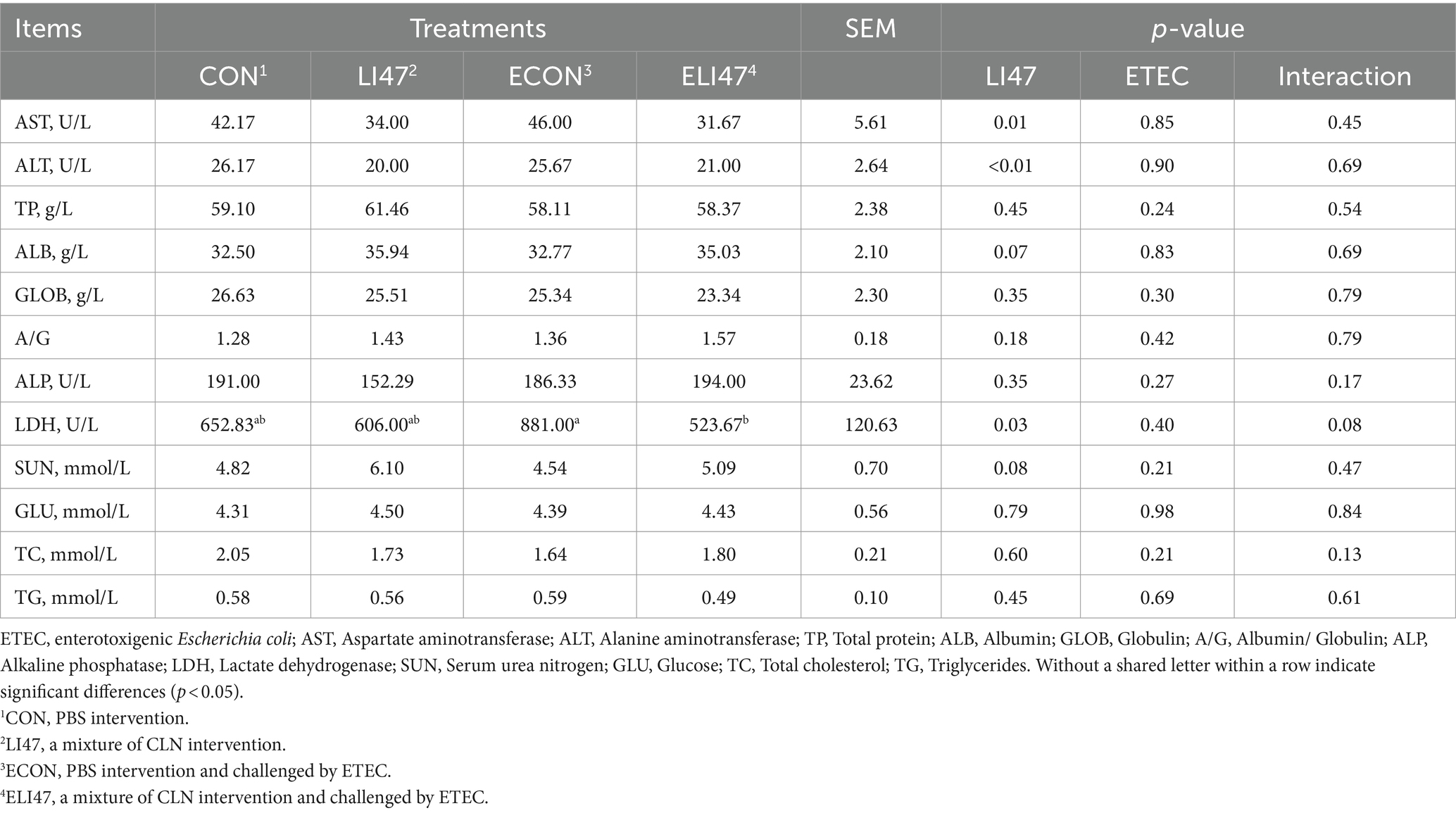
Table 1. The effects on serum biochemical markers of ETEC challenged piglets with the treatment of CLN.
2.6 Morphological observation
The fixed colon and liver tissue samples (as described by 2.4) were embedded in paraffin. Cross-sections of each sample were prepared and stained using either H&E or AB-PAS staining methods, then sealed with neutral resin (29, 30). Ultimately, the Image-Pro Plus 6.0 image system was utilized to measure the crypt depth and count goblet cells in the colon samples, as well as to examine the liver samples. Eight different fields were randomly selected per slide, and the average values were calculated as single-slide data.
2.7 Colon antioxidant activity
A 1:9 ratio of the colonic mucosa sample and pre-cooled 0.9% physiological saline were mixed and then centrifuged at 4°C (3,500 × g/10 min). The protein concentration was determined by utilizing a kit (Beyotime, Shanghai, China). Finally, the concentrations of total antioxidant capacity (T-AOC), malondialdehyde (MDA), glutathione peroxidase (GPX), catalase (CAT), and total superoxide dismutase (T-SOD) were determined following the kit instructions (Nanjing Jiancheng, China).
2.8 16S rRNA sequencing
The total DNA was obtained from colon digest using a kit from Omega Bio-Tek (Norcross, GA, USA), and primers 515F (5′- brocade-GTGCCGCCAGCMGCCGG-3′) and 907R (5′- CCGTCAATTCMTTTRAGT-TT-3′) were used to amplified the V4-V5 region of the 16S rRNA gene, where brocade is the unique eight base sequence for each sample. Subsequently, following the Illumina genomic DNA library preparation protocol, the merged DNA products were used to construct an Illumina paired-end library. The amplicon library was then sequenced on the Illumina MiSeq platform (Shanghai BIOZERON Co., Ltd) according to the standard protocol using paired-end sequencing (2 × 250). The Illumina PE250 sequencing reads were processed to obtain valid sequences for all samples based on the barcode, and quality control filtering was performed on the reads, followed by optimization and statistical analysis of the data. Operational taxonomic units (OTUs) were defined at a 97% similarity level for bioinformatics statistical analysis and alpha diversity assessment.
2.9 Colon short-chain fatty acids
A previously described method was used to determine the contents of SCFAs (31). Firstly, the pre-treated sample was mixed with a 25% (w/v) metaphosphate acid solution and then stored overnight at −20°C. After thawing, the mixture was centrifuged to obtain the supernatant, which was then filtered through a 0.22 μm filter.
2.10 RNA-seq analysis in liver
According to the manufacturer’s instructions (Invitrogen), total RNA was extracted from liver tissue using TRIzol reagent, followed by the removal of rRNA and enrichment of mRNA. The Illumina TruSeq™ RNA Sample Prep Kit was then employed to construct a cDNA library by reverse transcribing the mRNA into cDNA. The purified double-stranded cDNA underwent end repair, A-tailing, and adapter ligation, followed by the selection of approximately 200 bp cDNA fragments. These fragments were PCR amplified and purified again to obtain the final library. Quality control of the library was performed using agarose gel electrophoresis, and RNA concentration, purity, and integrity were assessed. After passing quality control, sequencing was conducted using the Illumina TruSeq SBS Kit (300 cycles). The raw data underwent quality control to generate valid data, which were aligned to the reference genome to identify differentially expressed genes (DEGs, p < 0.05) among the samples. The Kyoto Encyclopedia of Genes and Genomes (KEGG) was used for annotation and functional enrichment of the DEGs.
2.11 Enzyme-Linked Immunosorbent Assay
The concentrations of immune cytokines, tumor necrosis factor (TNF)-α, secretory immunoglobulin A (sIgA), and LPS in the colonic mucosa were determined using ELISA kits supplied by Nanjing Jiancheng (Nanjing, China).
2.12 Quantitative real-time PCR
Total RNA was extracted from both colonic mucosa and liver tissue using a kit from Accurate (Hunan, China), and the RNA quality was assessed using the ND-2000 (Thermo Scientific, Wilmington, United States). Subsequently, PCR detection was performed using a kit (Accurate, Hunan, China). The primers utilized in the study are listed in Table 2. Finally, the expression levels of the target genes were assessed using the 2-△△Ct method (32).
2.13 Statistical analysis
Presented the data as mean ± standard error of the mean (SEM) and analyzed by SPSS 25.0 software (SPSS Inc., Chicago, IL, United States). p-value <0.05 means statistical difference, 0.05 < p < 0.10 means a trend. The general linear model (GLM) procedure with a two-factor (LI47 and ETEC) analysis of variance (ANOVA) design was employed. Significant differences were conducted by Tukey’s multiple range test. Different bacterial populations were compared using the Mann–Whitney U test, and multiple comparisons were corrected using the Benjamini-Hochberg false discovery rate. Finally, graphs were formed using GraphPad Prism 8.0.2 (La Jolla, CA, United States).
3 Results
3.1 CLN improves colon morphology induced by ETEC-challenged
As shown in Table 3, treatment with CLN did not affect either colonic length or weight. Figures 1B,C shows that ETEC challenge decreased the colonic crypt depth, while treatment with CLN increased it (p < 0.05). An interaction effect on crypt depth was observed between ETEC challenge and treatment with CLN (p < 0.05).
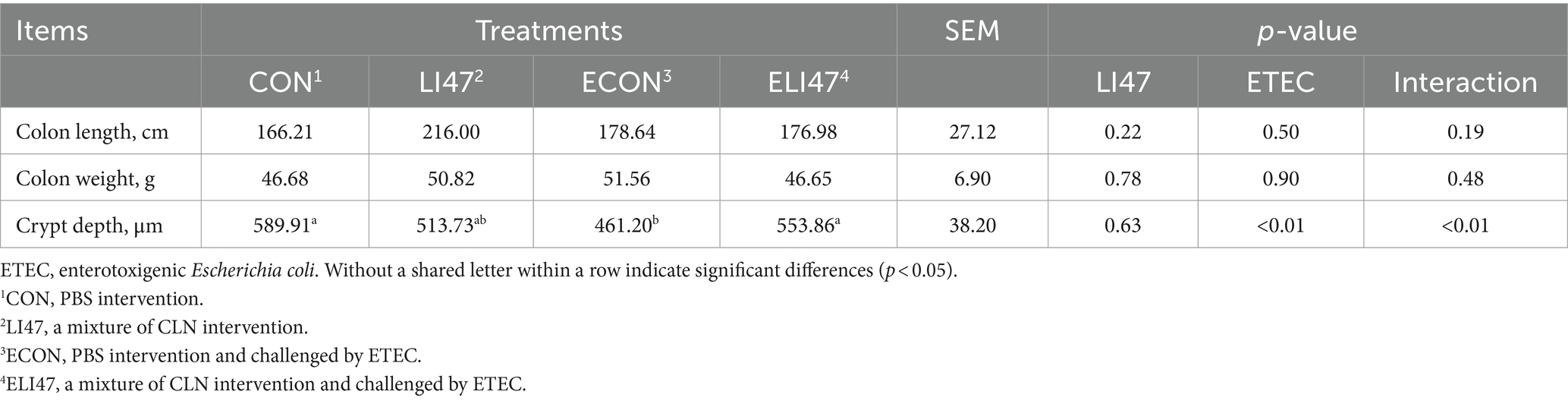
Table 3. The effects on the intestinal morphology of ETEC-challenged piglets with the treatment of CLN.
3.2 CLN enhances colon barrier function
The goblet cell count was decreased by ETEC challenge, but treatment with CLN increased it (Figure 1D, p > 0.05). Additionally, treatment with CLN increased the mRNA expression of MUC2 (Figure 1E, 0.05 < p < 0.1), Occludin and Claudin-1 (Figures 1F–H, p < 0.05). No interaction was found in the goblet cell count and the mRNA expression of MUC2, Occludin, Claudin-1, and ZO-1 between ETEC challenge and CLN treatment (p > 0.05).
3.3 CLN alleviates colonic inflammation in ETEC-challenged piglets
ETEC challenge increased the concentration of TNF-α and IL-6 in the colonic mucosa (p < 0.05), while treatment with CLN showed the opposite effect (Figures 2A,B, p < 0.05). Additionally, ETEC challenge decreased the concentration of IL-10 and secretory immunoglobulin A (sIgA) in the colonic mucosa, whereas treatment with CLN increased their levels (Figures 2D,E, p < 0.05). No difference was observed in IL-1β across the four groups (Figure 2C, p > 0.05). An interaction effect on the concentration of TNF-α, IL-6, IL-10, and sIgA was found between treatment with CLN and ETEC challenge (p < 0.05), while no interaction was observed in IL-1β (p > 0.05).
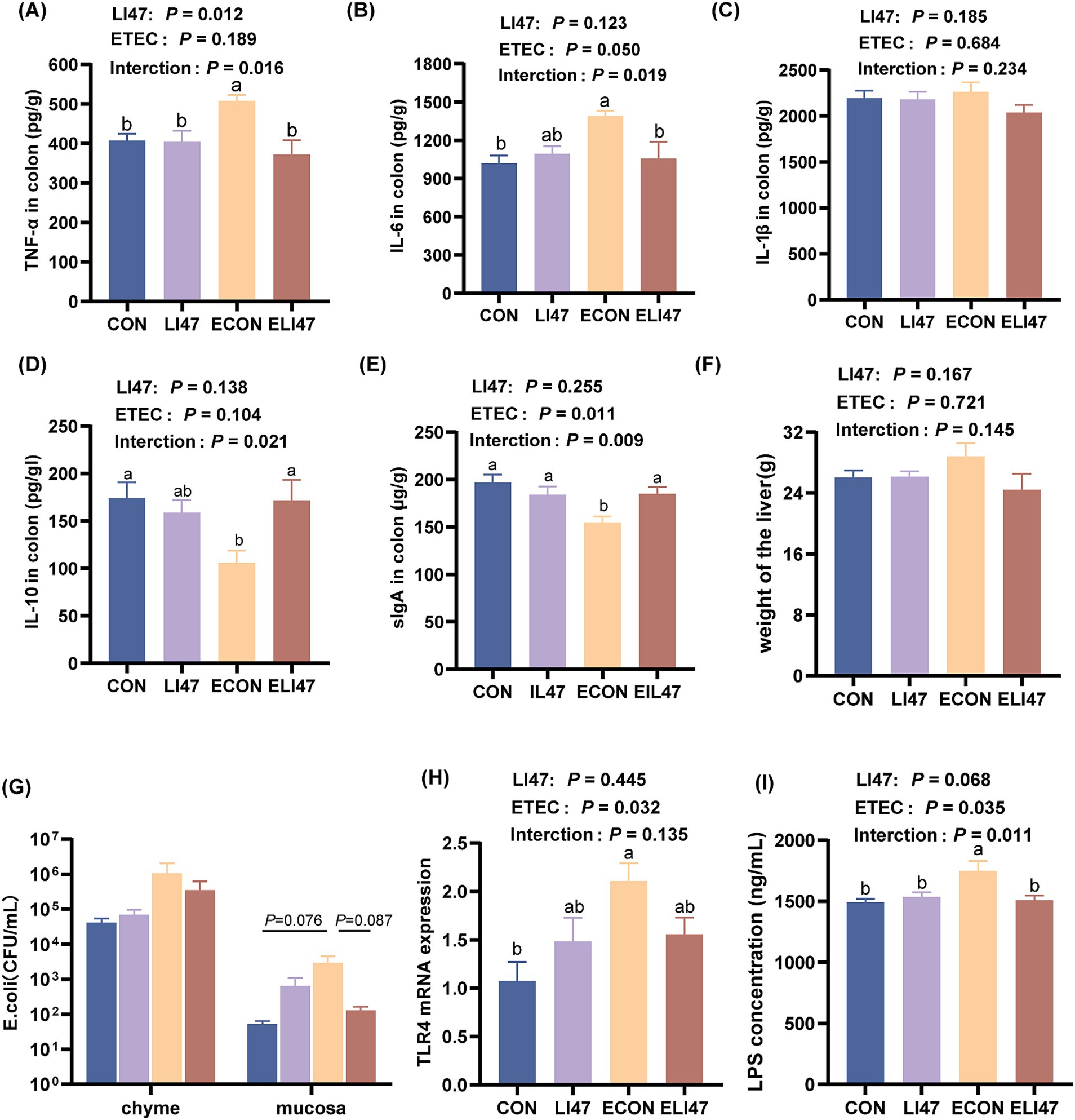
Figure 2. Effects of the treatment with CLN on the cytokines and the level of E. coli/LPS/TLR4 in the colon mucosa and the liver weight. Concentration of (A) TNF-α, (B) IL-6, (C) IL-1β, (D) IL-10, (E) sIgA in the colon mucosa. (F) The weight of the liver. (G) The colonization level of E. coli in colon chyme and mucosa. (H) The concentration of LPS and (I) gene expression of TLR4 in colon mucosa. Results are expressed as mean ± SEM (n = 7), and shoulder markings without the same letter indicate differences (p < 0.05). CON, PBS intervention. LI47, a mixture of CLN intervention. ECON, PBS intervention and challenged by ETEC. ELI47, a mixture of CLN intervention and challenged by ETEC.
3.4 CLN improves the colonic antioxidant capacity in ETEC-challenged piglets
As shown in Table 4, ETEC challenge decreased the concentration of GPX (p < 0.05) and increased MDA (p < 0.05), both of which were reversed by treatment with CLN (p < 0.05). Additionally, ETEC challenge decreased the concentration of CAT. There was an interaction found in the concentration of MDA between treatment with CLN and ETEC challenge (p < 0.05), while no interaction was found on T-AOC, T-SOD, GPX, and CAT (p > 0.05).
3.5 CLN improves the colonic microbiota in ETEC-challenged piglets
In the ELI47 group, ACE and Chao index were lower compared to the CON and LI47 groups (Figure 3A, p < 0.05). However, no differences were observed in Shannon and Simpson index across the 4 groups (Figure 3B, p > 0.05). Firmicutes and Bacteroidota were the most abundant phylum (Figure 3C). Treatment with CLN increased the relative abundance of Firmicutes (p < 0.05) while showing a trend toward decreasing the level of Bacteroidota (Figure 3D, 0.05 < p < 0.10). The proportions of Desulfobacterota and Campylobacterota were decreased by ETEC challenge (Figure 3D, 0.05 < p < 0.10), while CLN treatment showed an increasing trend of Desulfobacterota (Figure 3D, 0.05 < p < 0.10).
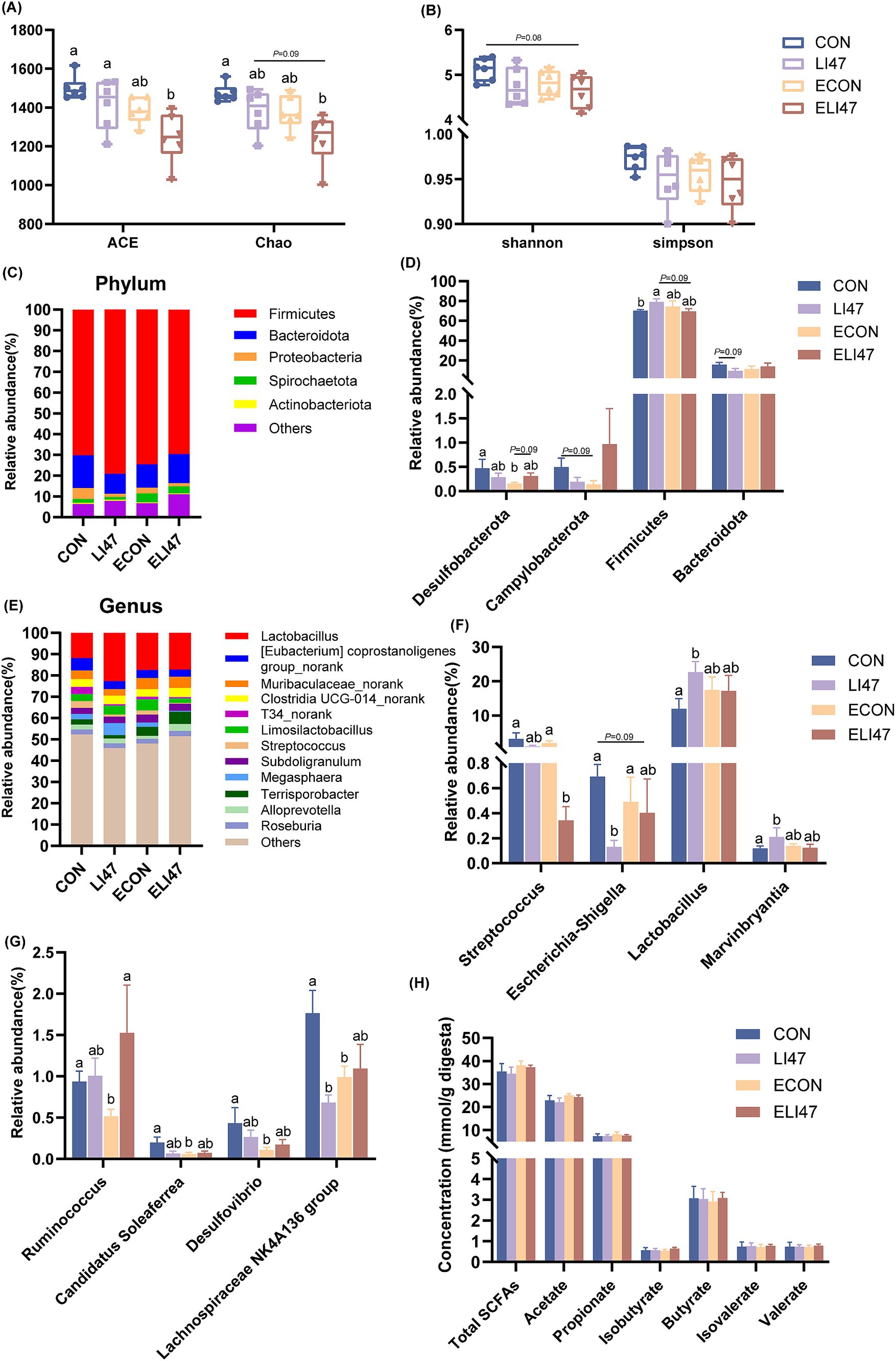
Figure 3. CLN regulates the composition of colonic digest microbiota and SCFAs concentration in piglets. (A) ACE, Chao, (B) Shannon and Simpson index among four groups. Relative abundance of the microbial (C) phylum and (E) genus, (D) different phyla and (F–G) different genera. (H) The concentrations of SCFAs among four groups. Results are expressed as mean ± SEM (n = 7), and shoulder markings without the same letter indicate differences (p < 0.05). CON, PBS intervention. LI47, a mixture of CLN intervention. ECON, PBS intervention and challenged by ETEC. ELI47, a mixture of CLN intervention and challenged by ETEC.
Lactobacillus was the most abundant genus in the colon (Figure 3E), and CLN treatment promoted the proportions of Lactobacillus and Marvinbryantia (Figure 3F, P < 0.05), while decreasing the levels of Lachnospiraceae NK4A136 group, Escherichia-Shigella and Streptococcus (Figures 3F,G, p < 0.05). Lower abundances of Ruminococcus, Candidatus Soleaferrea, Desulfovibrio, and Lachnospiraceae NK4A136 group were observed following ETEC challenge (Figure 3G, p < 0.05). However, no differences in the SCFAs were found among the 4 groups (Figure 3H, p > 0.05).
3.6 CLN inhibits the Escherichia coli/LPS/TLR4 pathway in ETEC-challenged piglets
ETEC challenge resulted in an increased E. coli load (0.05 < p < 0.1), a higher LPS concentration (p < 0.05), and an elevated level of TLR4 (p < 0.05) in the colonic mucosa (Figures 2G–I), while these were decreased in ELI47 group (0.05 < p < 0.10). However, there was no difference in the load of E. coli in the colonic digest among the 4 groups (Figure 2G, p > 0.05).
3.7 CLN alleviates the expression of liver injury markers in serum in ETEC-challenged piglets
Compared with the ECON group, the concentration of LDH (p < 0.05) and AST (p = 0.08) decreased in the ELI47 group (Table 1). However, there was no difference in the concentrations of ALT, TP, TC, TG, SUN, etc. among the four groups (Table 1, p > 0.05). An interaction was revealed in LDH level between ETEC-challenge and treatment with CLN (p < 0.05).
3.8 CLN has no effects on liver morphology and inflammation
There were no differences in liver weight and liver tissue sections among the 4 groups, as all exhibited intact liver tissue structure with clear and complete hepatic lobules and hepatic cords, well-arranged hepatocytes, and no visible lipid droplet accumulation (Figures 2F, 4A). Additionally, the mRNA expression of TNF-α, IL-1β, IL-6, and IL-10 showed no differences among the 4 groups (Figures 4B–E, p > 0.05). No interaction was observed on TNF-α, IL-1β, IL-6, and IL-10 levels between ETEC-challenged and the supplementation of CLN (p > 0.05).
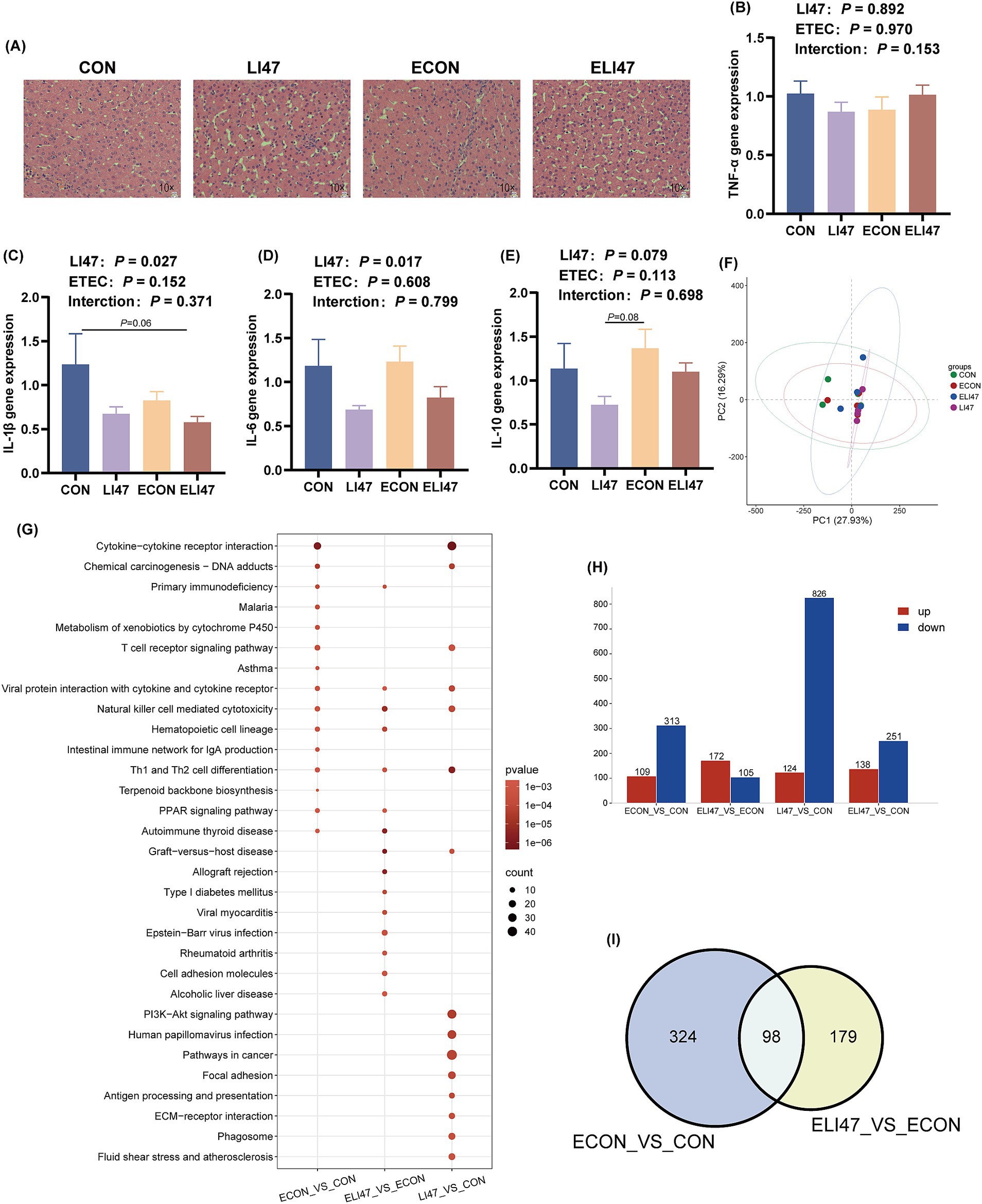
Figure 4. Effects of the treatment with CLN on the liver morphology and RNA-seq of ETEC-challenged piglets. (A) Liver tissues stained with H&E. Scale bar, 100 μm. Gene expression of (B) TNF-α, (C) IL-1β, (D) IL-6, (E) IL-10 in liver tissues (n = 7). (F) PCA analysis, (H) DEGs, (I) Venn diagram and (G) KEGG pathway enrichment analysis of DEGs among four groups (n = 4). Results are expressed as mean ± SEM. CON, PBS intervention. LI47, a mixture of CLN intervention. ECON, PBS intervention and challenged by ETEC. ELI47, a mixture of CLN intervention and challenged by ETEC.
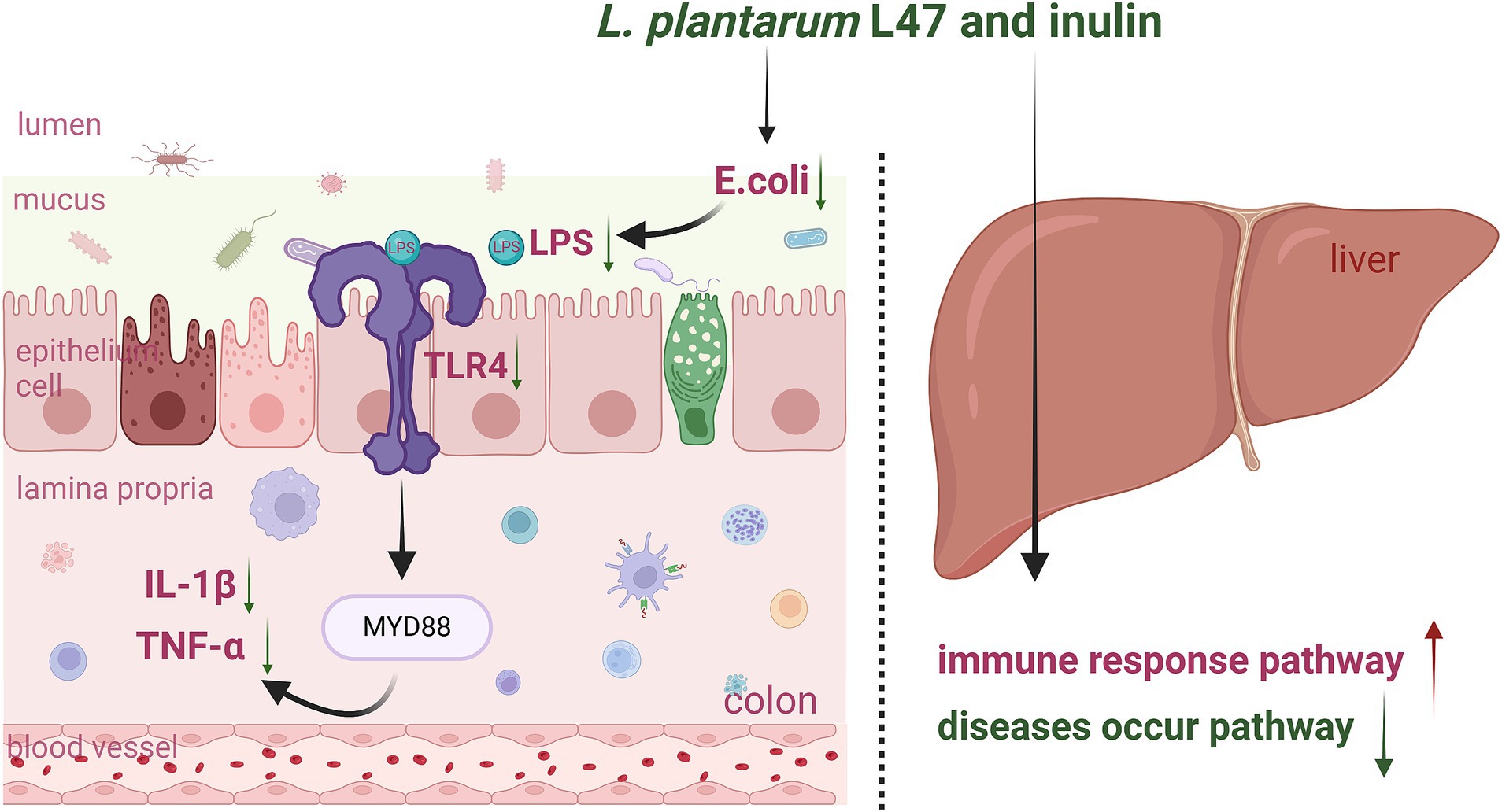
Figure 5. Graphic Summary: Effects of the treatment with CLN on the colon and liver inflammation of ETEC-challenged piglets (by Biorender). LI47, L. plantarum L47 and inulin; E. coli, Escherichia coli; LPS, lipopolysaccharide; TLR4, toll like receptor 4; MYD88, myeloid differentiation primary response gene (88); IL, interleukin; TNF-α, tumor necrosis factor-α.
3.9 CLN regulates pathways related to immunity and disease occurrence
The PCA analysis presented no obvious separation trend among the 4 groups (Figure 4F). ETEC challenge resulted in 422 differentially expressed genes (DEGs) (109 up-regulated, 313 down-regulated) compared to the CON group (Figure 4H), while treatment with CLN (ELI47 group) showed 277 DEGs (172 up-regulated, 105 down-regulated) (Figure 4H). Furthermore, 940 DEGs (124 upregulated, 826 downregulated) were identified in the LI47 group compared to the CON group (Figure 4H). Both ETEC-challenge and treatment with CLN modulated 98 DEGs (Figure 4I). KEGG enrichment analysis based on DEGs was performed (Figure 4G). Pathways associated with immune regulation, including Hematopoietic cell lineage, Intestinal immune network for IgA production, and Th1 and Th2 cell differentiation were down-regulated by ETEC challenge (Figure 4G). Besides, treatment with CLN up-regulated pathways such as Th1 and Th2 cell differentiation, Hematopoietic cell lineage, while down-regulating pathways like Alcoholic liver disease, Focal adhesion, and Fluid shear stress and atherosclerosis, etc. (Figure 4G).
4 Discussion
The intestinal barrier, formed by numerous epithelial cells, serves the crucial role of distinguishing the external environment from the internal host system (33). The intestine is a complex and crucial organ involved in digesting and absorbing nutrients, managing metabolic processes, and regulating immune functions (34). However, ETEC infection induces intestinal damage such as villous atrophy, inflammation, oxidative stress, and dysbiosis (5). Therefore, it is of practical significance to explore effective strategies to protect intestinal health. Synbiotics, which are composed of probiotics and prebiotics, maintain or enhance intestinal health through various mechanisms (35–37). Here, we aim to explore the potential benefits of dietary CLN supplementation against ETEC colonic injury.
The function of intestinal morphology and the integrity of the barrier are closely associated with the occurrence of intestinal inflammation and oxidative stress (38–43). The intestine is lined with numerous intestinal epithelial cells (IECs) that secrete mucins (such as MUC2) and antimicrobial proteins to maintain the chemical barrier, while intercellular tight junctions maintain the physical barrier of the intestine (33, 44, 45). Alexia’s study suggests that the synbiotic combination of Lactobacillus acidophilus W37 and inulin can directly stimulate IECs or immune cells, thereby activating immune receptors involved in immune regulation (46). In this study, ETEC-challenge induced colonic injury, while CLN supplementation exhibited protective and restorative effects. This is consistent with previous research (17, 47, 48), indicating that CLN has the potential to protect intestinal health, and its protective mechanism warrants further investigation.
Recently, there has been a growing interest in altering the intestinal microbiota using synbiotics (22, 49, 50). Robin supplemented piglets’ diets with Pediococcus acidilactici and lactulose, which increased the proportion of beneficial microbes like Lactobacillus and Prevotella, and alleviated the intestinal inflammation induced by Shiga toxin-producing Escherichia coli (51). Similarly, our previous study indicated that intervention with CLN altered the fermentation environment of the simulated pig colon and increased the production of SCFAs in vitro (24). Firmicutes are Gram-positive bacteria, including the genera Bacillus, Clostridium, and Lactobacillus (52). The increase in abundance of Firmicutes by supplementing CLN may be attributed to the increase in abundance of Lactobacillus, it reported that the Lactobacillus genus promoted intestinal epithelial cell proliferation, maintained intestinal barrier function, and reduced intestinal inflammatory responses (53, 54). Lachnospiraceae are considered a beneficial bacterium in the gut in numerous studies, involved in fiber digestion and SCFAs production, however, Lachnospiraceae may also be associated with the occurrence of metabolic diseases (55–57). In addition, supplementation with CLN decreased the proportion of harmful bacteria like Escherichia-Shigella and Streptococcus in the colon. This suggests that CLN might exert anti-inflammatory and antioxidant properties through its impact on intestinal microbiota composition. Several signaling pathways are involved in regulating inflammation and oxidative stress, including AHR/HTAT3 (58), TLR4/NOD (19), AHR/Nrf2 (59), and NF-kB/MAPK (60). LPS is a component released upon the death of Gram-negative bacteria like E. coli, and it plays a role in inflammation and can even induce sepsis (61, 62). The recognition and binding of LPS to the TLR4 receptor can activate intracellular signaling via MyD88-dependent or -independent pathways, thereby inducing inflammation (63, 64). In this study, ETEC-challenge induced increases in the expression of E. coli/LPS/TLR4, while CLN supplementation reduced these levels. This suggests that treatment with CLN may alleviate colonic inflammation by decreasing E. coli abundance in colonic mucosa and inhibiting LPS/TLR4 recognition and binding.
LDH is present in various organs, including the liver, heart, lymph nodes, spleen, lungs, and pancreas. It participates in the glycolytic pathway and catalyzes the redox reaction between pyruvate and lactate. When these tissues are damaged, LDH is released into the bloodstream in large quantities (65, 66). Therefore, the elevated serum concentration of LDH in the ECON group may indicate that an organ has suffered damage. Interestingly, the treatment with CLN could prevent such damage from occurring. The liver is a crucial metabolic organ, and AST and ALT are commonly used as biomarkers for liver injury (67). Compared to the ECON group, the ELI47 group exhibited a trend toward reduced AST concentration, suggesting that CLN may have a protective effect against liver injury. Currently, an increasing number of studies are focusing on the relationship between gut microbiota and liver diseases (68). For instance, excessive exposure to copper has been shown to impair intestinal barrier function and disrupt microbial communities, leading to increased production of LPS and activation of the TLR4/NF-kB signaling pathway, resulting in liver inflammation (69). Additionally, Chen demonstrated that Lactobacillus plantarum Lp2 could inhibit LPS-induced liver injury (70). Consistent with these studies, treatment with CLN demonstrates the ability to inhibit LPS/TLR4 production and has the potential to prevent liver injury. To further explore the effects of CLN on the liver, we conducted transcriptome analysis, which revealed significant enrichment of the Th1 and Th2 cell differentiation pathways. Th1 cells primarily induce the production of interferon-γ (IFN-γ), which is critical for the host’s autoimmune response. Th2 cells induce the secretion of cytokines such as IL-4, IL-5, and IL-13, promoting the production of immunoglobulin (Ig) A and Ig E, thereby regulating humoral immune responses and allergic diseases (71, 72). It is noteworthy that the balance between Th1 and Th2 cells is crucial for maintaining a normal immune response (73). A study indicated that ginsenoside Rh2 regulates Th1 differentiation and the Th1/Th2 immune balance through LDC (74). In this study, ETEC challenge downregulated the pathway of Th1 and Th2 cell differentiation, while CLN treatment upregulated this pathway. Additionally, CLN treatment inhibited several signaling pathways associated with disease development. The transcriptome results suggest that CLN may play a role in maintaining normal immune responses and inhibiting disease development. However, further studies are needed to validate the roles of these pathways. In conclusion, this study demonstrates that treatment with CLN can serve as an effective protective measure for promoting animal health.
5 Conclusion
Supplementation with CLN may reduce colonic inflammation induced by ETEC in weaned piglets by inhibiting the E. coli/LPS/TLR4 pathway (Figure 5). Neither ETEC challenge nor the intervention of CLN impacted the liver phenotype, such as maintaining intact liver tissue structure, well-arranged hepatocytes, and no visible lipid droplet accumulation. However, both interventions presented effects on LDH and AST levels in the serum and modulated pathways associated with immunity and disease occurrence in the liver.
Data availability statement
The datasets of 16S rRNA gene sequence and transcriptome sequence presented in the study are deposited in the NCBI repository, accession number are PRJNA1185907 and PRJNA1185914.
Ethics statement
The animal study was approved by SYXK-2021-0086, the Animal Ethics Committee at Nanjing Agricultural University. The study was conducted in accordance with the local legislation and institutional requirements.
Author contributions
JM: Data curation, Formal analysis, Investigation, Methodology, Supervision, Visualization, Writing – original draft, Writing – review & editing. LC: Investigation, Methodology, Supervision, Writing – review & editing. HZ: Data curation, Methodology, Supervision, Writing – review & editing. MH: Data curation, Supervision, Writing – review & editing. JW: Supervision, Writing – review & editing. SH: Funding acquisition, Investigation, Project administration, Supervision, Writing – review & editing.
Funding
The author(s) declare that financial support was received for the research, authorship, and/or publication of this article. We appreciate the support from the National Key Research and Development Program of China for this study (2021YFD1300301-5).
Conflict of interest
The authors declare that the research was conducted in the absence of any commercial or financial relationships that could be construed as a potential conflict of interest.
Publisher’s note
All claims expressed in this article are solely those of the authors and do not necessarily represent those of their affiliated organizations, or those of the publisher, the editors and the reviewers. Any product that may be evaluated in this article, or claim that may be made by its manufacturer, is not guaranteed or endorsed by the publisher.
Supplementary material
The Supplementary material for this article can be found online at: https://www.frontiersin.org/articles/10.3389/fvets.2024.1496893/full#supplementary-material
References
1. Bonetti, A, Tugnoli, B, Piva, A, and Grilli, E. Towards zero zinc oxide: feeding strategies to manage post-weaning diarrhea in piglets. Animals. (2021) 11:642. doi: 10.3390/ani11030642
2. Dang, DX, Choi, SY, Choi, YJ, Lee, JH, Castex, M, Chevaux, E, et al. Probiotic, paraprobiotic, and hydrolyzed yeast mixture supplementation has comparable effects to zinc oxide in improving growth performance and ameliorating post-weaning diarrhea in weaned piglets. Probiotics Antimicrob Proteins. (2023) 16:249–58. doi: 10.1007/s12602-022-10008-8
3. Cremonesi, P, Biscarini, F, Castiglioni, B, Sgoifo, CA, Compiani, R, and Moroni, P. Gut microbiome modifications over time when removing in-feed antibiotics from the prophylaxis of post-weaning diarrhea in piglets. PLoS One. (2022) 17:e0262199. doi: 10.1371/journal.pone.0262199
4. Roussel, C, De Paepe, K, Galia, W, de Bodt, J, Chalancon, S, Denis, S, et al. Multi-targeted properties of the probiotic saccharomyces cerevisiae CNCM I-3856 against enterotoxigenic escherichia coli (ETEC) H10407 pathogenesis across human gut models. Gut Microbes. (2021) 13:1953246. doi: 10.1080/19490976.2021.1953246
5. Guo, P, Wang, Z, Lv, X, Wang, X, Yu, J, Tian, X, et al. Changyanning regulates gut microbiota and metabolism to ameliorate intestinal injury induced by ETEC K88. Front Microbiol. (2023) 14:1098818. doi: 10.3389/fmicb.2023.1098818
6. Gresse, R, Chaucheyras-Durand, F, Fleury, MA, Van de Wiele, T, Forano, E, and Blanquet-Diot, S. Gut microbiota dysbiosis in postweaning piglets: understanding the keys to health. Trends Microbiol. (2017) 25:851–73. doi: 10.1016/j.tim.2017.05.004
7. Barba-Vidal, E, Martín-Orúe, SM, and Castillejos, L. Review: are we using probiotics correctly in post-weaning piglets? Animal. (2018) 12:2489–98. doi: 10.1017/s1751731118000873
8. Ma, T, Huang, W, Li, Y, Jin, H, Kwok, L-Y, Sun, Z, et al. Probiotics alleviate constipation and inflammation in late gestating and lactating sows. NPJ Biofilms and Microbiomes. (2023) 9:70. doi: 10.1038/s41522-023-00434-z
9. Yang, J, Qiu, Y, Hu, S, Zhu, C, Wang, L, Wen, X, et al. Lactobacillus plantarum inhibited the inflammatory response induced by enterotoxigenic Escherichia coli K88 via modulating MAPK and NF-κB signalling in intestinal porcine epithelial cells. J Appl Microbiol. (2020) 130:1684–94. doi: 10.1111/jam.14835
10. Shi, R, Ye, J, Fan, H, Xiao, C, Wang, D, Xia, B, et al. Lactobacillus plantarum LLY-606 supplementation ameliorates hyperuricemia via modulating intestinal homeostasis and relieving inflammation. Food Funct. (2023) 14:5663–77. doi: 10.1039/d2fo03411e
11. Sanders, ME, Merenstein, DJ, Reid, G, Gibson, GR, and Rastall, RA. Probiotics and prebiotics in intestinal health and disease: from biology to the clinic. Nat Rev Gastroenterol Hepatol. (2019) 16:605–16. doi: 10.1038/s41575-019-0173-3
12. Oniszczuk, A, Oniszczuk, T, Gancarz, M, and Szymańska, J. Role of gut microbiota, probiotics and prebiotics in the cardiovascular diseases. Molecules. (2021) 26:1172. doi: 10.3390/molecules26041172
13. Birkeland, E, Gharagozlian, S, Birkeland, KI, Valeur, J, Måge, I, Rud, I, et al. Prebiotic effect of inulin-type fructans on faecal microbiota and short-chain fatty acids in type 2 diabetes: a randomised controlled trial. Eur J Nutr. (2020) 59:3325–38. doi: 10.1007/s00394-020-02282-5
14. Beisner, J, Filipe, RL, Kaden-Volynets, V, Stolzer, I, Günther, C, and Bischoff, SC. Prebiotic inulin and sodium butyrate attenuate obesity-induced intestinal barrier dysfunction by induction of antimicrobial peptides. Front Immunol. (2021) 12:678360. doi: 10.3389/fimmu.2021.678360
15. Wang, Y, Nan, X, Zhao, Y, Jiang, L, Wang, H, Zhang, F, et al. Dietary supplementation of inulin ameliorates subclinical mastitis via regulation of rumen microbial community and metabolites in dairy cows. Microbiol Spectr. (2021) 9:e0010521. doi: 10.1128/spectrum.00105-21
16. Bao, T, He, F, Zhang, X, Zhu, L, Wang, Z, Lu, H, et al. Inulin exerts beneficial effects on non-alcoholic fatty liver disease via modulating gut microbiome and suppressing the lipopolysaccharide-toll-like receptor 4-Mψ-nuclear factor-κB-nod-like receptor protein 3 pathway via gut-liver axis in mice. Front Pharmacol. (2020) 11:558525. doi: 10.3389/fphar.2020.558525
17. Liu, Z, Liu, F, Wang, W, Sun, C, Gao, D, Ma, J, et al. Study of the alleviation effects of a combination of Lactobacillus rhamnosus and inulin on mice with colitis. Food Funct. (2020) 11:3823–37. doi: 10.1039/c9fo02992c
18. Ayala-Monter, MA, Hernández-Sánchez, D, González-Muñoz, S, Pinto-Ruiz, R, Martínez-Aispuro, JA, Torres-Salado, N, et al. Growth performance and health of nursing lambs supplemented with inulin and Lactobacillus casei. Anim Biosci. (2019) 32:1137–44. doi: 10.5713/ajas.18.0630
19. Zhu, H, Wang, H, Wang, S, Tu, Z, Zhang, L, Wang, X, et al. Flaxseed oil attenuates intestinal damage and inflammation by regulating necroptosis and TLR4/NOD signaling pathways following lipopolysaccharide challenge in a piglet model. Mol Nutr Food Res. (2018) 62:e1700814. doi: 10.1002/mnfr.201700814
20. An, L, Wirth, U, Koch, D, Schirren, M, Drefs, M, Koliogiannis, D, et al. The role of gut-derived lipopolysaccharides and the intestinal barrier in fatty liver diseases. J Gastrointest Surg. (2021) 26:671–83. doi: 10.1007/s11605-021-05188-7
21. Mouzaki, M, Comelli, EM, Arendt, BM, Bonengel, J, Fung, SK, Fischer, SE, et al. Intestinal microbiota in patients with nonalcoholic fatty liver disease. Hepatology. (2013) 58:120–7. doi: 10.1002/hep.26319
22. Roy, S, and Dhaneshwar, S. Role of prebiotics, probiotics, and synbiotics in management of inflammatory bowel disease: current perspectives. World J Gastroenterol. (2023) 29:2078–100. doi: 10.3748/wjg.v29.i14.2078
23. Li, X, Wang, C, Yu, D, Ding, L, Zhu, W, and Hang, S. Isolation, identification and characterization of lactic acid bacteria from swine. Acta Microbiol Sin. (2017) 57:1879–87. doi: 10.13343/j.cnki.wsxb.20170004
24. Zhu, X, Zhang, Z, Cui, L, Zhu, W, and Hang, S. Combination screening of lactobacillus spp. with prebiotics and analysis of its in vitro fermentation characteristics. Acta Microbiol Sin. (2021) 61:104–14. doi: 10.13343/j.cnki.wsxb.20200102
25. Cui, L, Zeng, H, Hou, M, Li, Z, Mu, C, Zhu, W, et al. Lactiplantibacillus plantarum L47 and inulin alleviate enterotoxigenic Escherichia coli induced ileal inflammation in piglets by upregulating the levels of α-linolenic acid and 12,13-epoxyoctadecenoic acid. Animl Nutr. (2023) 14:370–82. doi: 10.1016/j.aninu.2023.06.008
26. Zhang, L. Feed analysis and feed quality testing technology. Beijing: china agricultural university press (2007).
27. Ding, H, Zhao, X, Azad, MAK, Ma, C, Gao, Q, He, J, et al. Dietary supplementation with Bacillus subtilis and xylo-oligosaccharides improves growth performance and intestinal morphology and alters intestinal microbiota and metabolites in weaned piglets. Food Funct. (2021) 12:5837–49. doi: 10.1039/d1fo00208b
28. Renner, L, Kahlert, S, Tesch, T, Bannert, E, Frahm, J, Barta-Böszörményi, A, et al. Chronic DON exposure and acute LPS challenge: effects on porcine liver morphology and function. Mycotoxin Res. (2017) 33:207–18. doi: 10.1007/s12550-017-0279-9
29. Brown, DC, Maxwell, CV, Erf, GF, Davis, ME, Singh, S, and Johnson, ZB. The influence of different management systems and age on intestinal morphology, immune cell numbers and mucin production from goblet cells in post-weaning pigs. Vet Immunol Immunopathol. (2006) 111:187–98. doi: 10.1016/j.vetimm.2005.12.006
30. Bubnov, RV, Babenko, LP, Lazarenko, LM, Mokrozub, VV, Demchenko, OA, Nechypurenko, OV, et al. Comparative study of probiotic effects of Lactobacillus and Bifidobacteria strains on cholesterol levels, liver morphology and the gut microbiota in obese mice. EPMA J. (2017) 8:357–76. doi: 10.1007/s13167-017-0117-3
31. Li, Z, Ding, L, Zhu, W, and Hang, S. Effects of the increased protein level in small intestine on the colonic microbiota, inflammation and barrier function in growing pigs. BMC Microbiol. (2022) 22:172. doi: 10.1186/s12866-022-02498-x
32. Schmittgen, TD, and Livak, KJ. Analyzing real-time PCR data by the comparative C(T) method. Nat Protoc. (2008) 3:1101–8. doi: 10.1038/nprot.2008.73
33. Peterson, LW, and Artis, D. Intestinal epithelial cells: regulators of barrier function and immune homeostasis. Nat Rev Immunol. (2014) 14:141–53. doi: 10.1038/nri3608
34. Elmentaite, R, Kumasaka, N, Roberts, K, Fleming, A, Dann, E, King, HW, et al. Cells of the human intestinal tract mapped across space and time. Nature. (2021) 597:250–5. doi: 10.1038/s41586-021-03852-1
35. Mounir, M, Ibijbijen, A, Farih, K, Rabetafika, HN, and Razafindralambo, HL. Synbiotics and their antioxidant properties, mechanisms, and benefits on human and animal health: a narrative review. Biomol Ther. (2022) 12:1443. doi: 10.3390/biom12101443
36. Markowiak, P, and Śliżewska, K. Effects of probiotics, prebiotics, and synbiotics on human health. Nutrients. (2017) 9:1021. doi: 10.3390/nu9091021
37. Gibson, GR, and Roberfroid, MB. Dietary modulation of the human colonic microbiota: introducing the concept of prebiotics. J Nutr. (2019) 125:1401–12. doi: 10.1093/jn/125.6.1401
38. Qin, L, Ji, W, Wang, J, Li, B, Hu, J, and Wu, X. Effects of dietary supplementation with yeast glycoprotein on growth performance, intestinal mucosal morphology, immune response and colonic microbiota in weaned piglets. Food Funct. (2019) 10:2359–71. doi: 10.1039/c8fo02327a
39. Terciolo, C, Dobric, A, Ouaissi, M, Siret, C, Breuzard, G, Silvy, F, et al. Saccharomyces boulardii CNCM I-745 restores intestinal barrier integrity by regulation of E-cadherin recycling. J Crohns Colitis. (2017) 11:999–1010. doi: 10.1093/ecco-jcc/jjx030
40. Liu, N, Zhou, L, Fang, J, Jiang, H, and Liu, G. Effects of IQW and IRW on inflammation and gut microbiota in ETEC-induced diarrhea. Mediat Inflamm. (2021) 2021:2752265. doi: 10.1155/2021/2752265
41. Traserra, S, Casabella-Ramón, S, Vergara, P, and Jimenez, ME. Coli infection disrupts the epithelial barrier and activates intrinsic neurosecretory reflexes in the pig colon. Front Physiol. (2023) 14:1170822. doi: 10.3389/fphys.2023.1170822
42. Li, L, Peng, P, Ding, N, Jia, W, Huang, C, and Tang, Y. Oxidative stress, inflammation, gut dysbiosis: what can polyphenols do in inflammatory bowel disease? Antioxidants. (2023) 12:967. doi: 10.3390/antiox12040967
43. Chen, H, Li, Y, Wang, J, Zheng, T, Wu, C, Cui, M, et al. Plant polyphenols attenuate DSS-induced ulcerative colitis in mice via antioxidation, anti-inflammation and microbiota regulation. Int J Mol Sci. (2023) 24:10828. doi: 10.3390/ijms241310828
44. Nyström, EEL, Martinez-Abad, B, Arike, L, Birchenough, GMH, Nonnecke, EB, Castillo, PA, et al. An intercrypt subpopulation of goblet cells is essential for colonic mucus barrier function. Science. (2021) 372:eabb1590. doi: 10.1126/science.abb1590
45. Capaldo, CT, Powell, DN, and Kalman, D. Layered defense: how mucus and tight junctions seal the intestinal barrier. J Mol Med. (2017) 95:927–34. doi: 10.1007/s00109-017-1557-x
46. Lépine, A, and de Vos, P. Synbiotic effects of the dietary fiber long-chain inulin and probiotic Lactobacillus acidophilus W37 can be caused by direct, synergistic stimulation of immune toll-like receptors and dendritic cells. Mol Nutr Food Res. (2018) 62:e1800251. doi: 10.1002/mnfr.201800251
47. Zheng, Y, Zhang, Z, Tang, P, Wu, Y, Zhang, A, Li, D, et al. Probiotics fortify intestinal barrier function: a systematic review and meta-analysis of randomized trials. Front Immunol. (2023) 14:1143548. doi: 10.3389/fimmu.2023.1143548
48. Ye, W, Chen, Z, He, Z, Gong, H, Zhang, J, Sun, J, et al. Lactobacillus plantarum-derived postbiotics ameliorate acute alcohol-induced liver injury by protecting cells from oxidative damage, improving lipid metabolism, and regulating intestinal microbiota. Nutrients. (2023) 15:845. doi: 10.3390/nu15040845
49. Li, H-Y, Zhou, D-D, Gan, R-Y, Huang, S-Y, Zhao, C-N, Shang, A, et al. Effects and mechanisms of probiotics, prebiotics, synbiotics, and postbiotics on metabolic diseases targeting gut microbiota: a narrative review. Nutrients. (2021) 13:3211. doi: 10.3390/nu13093211
50. Zhang, X, Guan, X, Tang, Y, Sun, J, Wang, X, Wang, W-D, et al. Clinical effects and gut microbiota changes of using probiotics, prebiotics or synbiotics in inflammatory bowel disease: a systematic review and meta-analysis. Eur J Nutr. (2021) 60:2855–75. doi: 10.1007/s00394-021-02503-5
51. Guevarra, RB, Kim, ES, Cho, JH, Song, M, Cho, JH, Lee, JH, et al. Gut microbial shifts by synbiotic combination of Pediococcus acidilactici and lactulose in weaned piglets challenged with Shiga toxin-producing Escherichia coli. Front Vet Sci. (2023) 9:1101869. doi: 10.3389/fvets.2022.1101869
52. Rinninella, E, Raoul, P, Cintoni, M, Franceschi, F, Miggiano, GAD, Gasbarrini, A, et al. What is the healthy gut microbiota composition? A changing ecosystem across age, environment, diet, and diseases. Microorganisms. (2019) 7:14. doi: 10.3390/microorganisms7010014
53. Liu, Y, Zhang, H, Xie, A, Sun, J, Yang, H, Li, J, et al. Lactobacillus rhamnosus and L. plantarum combination treatment ameliorated colitis symptoms in a mouse model by altering intestinal microbial composition and suppressing inflammatory response. Mol Nutr Food Res. (2023) 67:e2200340. doi: 10.1002/mnfr.202200340
54. Wu, H, Xie, S, Miao, J, Li, Y, Wang, Z, Wang, M, et al. Lactobacillus reuteri maintains intestinal epithelial regeneration and repairs damaged intestinal mucosa. Gut Microbes. (2020) 11:997–1014. doi: 10.1080/19490976.2020.1734423
55. Grecco, GG, Gao, Y, Gao, H, Liu, Y, and Atwood, BK. Prenatal opioid administration induces shared alterations to the maternal and offspring gut microbiome: a preliminary analysis. Drug Alcohol Depend. (2021) 227:108914. doi: 10.1016/j.drugalcdep.2021.108914
56. Thipart, K, Gruneck, L, Phunikhom, K, Sharpton, TJ, Sattayasai, J, and Popluechai, S. Dark-purple rice extract modulates gut microbiota composition in acetic acid– and indomethacin-induced inflammatory bowel disease in rats. Int Microbiol. (2022) 26:423–34. doi: 10.1007/s10123-022-00309-x
57. Vacca, M, Celano, G, Calabrese, FM, Portincasa, P, Gobbetti, M, and De Angelis, M. The controversial role of human gut Lachnospiraceae. Microorganisms. (2020) 8:573. doi: 10.3390/microorganisms8040573
58. Wang, Q, Wang, F, Zhou, Y, Li, X, Xu, S, Jin, Q, et al. Bacillus amyloliquefaciens SC06 relieving intestinal inflammation by modulating intestinal stem cells proliferation and differentiation via AhR/STAT3 pathway in LPS-challenged piglets. J Agric Food Chem. (2024) 72:6096–109. doi: 10.1021/acs.jafc.3c05956
59. Xun, W, Fu, Q, Shi, L, Cao, T, Jiang, H, and Ma, Z. Resveratrol protects intestinal integrity, alleviates intestinal inflammation and oxidative stress by modulating AhR/Nrf2 pathways in weaned piglets challenged with diquat. Int Immunopharmacol. (2021) 99:107989. doi: 10.1016/j.intimp.2021.107989
60. Tian, M, Li, L, Tian, Z, Zhao, H, Chen, F, Guan, W, et al. Glyceryl butyrate attenuates enterotoxigenic Escherichia coli-induced intestinal inflammation in piglets by inhibiting the NF-κB/MAPK pathways and modulating the gut microbiota. Food Funct. (2022) 13:6282–92. doi: 10.1039/d2fo01056a
61. Trent, MS, Stead, CM, Tran, AX, and Hankins, JV. Diversity of endotoxin and its impact on pathogenesis. J Endotoxin Res. (2006) 12:205–23. doi: 10.1179/096805106x118825
62. Hsu, RYC, Chan, CHF, Spicer, JD, Rousseau, MC, Giannias, B, Rousseau, S, et al. LPS-induced TLR4 signaling in human colorectal cancer cells increases beta1 integrin-mediated cell adhesion and liver metastasis. Cancer Res. (2011) 71:1989–98. doi: 10.1158/0008-5472.can-10-2833
63. Qu, Y, Li, X, Xu, F, Zhao, S, Wu, X, Wang, Y, et al. Kaempferol alleviates murine experimental colitis by restoring gut microbiota and inhibiting the LPS-TLR4-NF-κB axis. Front Immunol. (2021) 12:679879. doi: 10.3389/fimmu.2021.679897
64. Lu, Y, Yeh, W, and Ohashi, PS. LPS/TLR4 signal transduction pathway. Cytokine. (2008) 42:145–51. doi: 10.1016/j.cyto.2008.01.006
65. Hicks, KG, Cluntun, AA, Schubert, HL, Hackett, SR, Berg, JA, Leonard, PG, et al. Protein-metabolite interactomics of carbohydrate metabolism reveal regulation of lactate dehydrogenase. Science. (2023) 379:996–1003. doi: 10.1126/science.abm3452
66. Ashraf, A, Liaquat, A, Shabbir, S, Bokhari, SA, Tariq, Z, Furrukh, Z, et al. High level of lactate dehydrogenase and ischaemia–reperfusion injury regulate the multiple organ dysfunction in patients with COVID-19. Postgrad Med J. (2022) 99:576–81. doi: 10.1136/postgradmedj-2022-141573
67. Evans, C, MacKenzie, F, and Marrington, R. Variation in liver function testing and the effect of pyridoxal-5-phosphate on ALT, AST and FIB-4 results. Ann Clin Biochem. (2024) 61:459–68. doi: 10.1177/00045632241269741
68. Tan, W, Mao, L, Yu, S, Huang, J, Xie, Q, Hu, M, et al. DHA and EPA improve liver IR in HFD-induced IR mice through modulating the gut microbiotas-LPS-liver axis. J Funct Foods. (2023) 112:105917. doi: 10.1016/j.jff.2023.105917
69. Gu, T, Kong, M, Duan, M, Chen, L, Tian, Y, Xu, W, et al. Cu exposure induces liver inflammation via regulating gut microbiota/LPS/liver TLR4 signaling axis. Ecotox Environ Safe. (2024) 278:116430. doi: 10.1016/j.ecoenv.2024.116430
70. Chen, Y, Guan, W, Zhang, N, Wang, Y, Tian, Y, Sun, H, et al. Lactobacillus plantarum Lp2 improved LPS-induced liver injury through the TLR-4/MAPK/NFκB and Nrf2-HO-1/CYP2E1 pathways in mice. Food Nutr Res. (2022) 66:5459. doi: 10.29219/fnr.v66.5459
71. Daďová, P, Mikulová, A, Jaroušek, R, Chorvátová, M, Uldrijan, S, and Kubala, L. A forskolin-mediated increase in cAMP promotes T helper cell differentiation into the Th1 and Th2 subsets rather than into the Th17 subset. Int Immunopharmacol. (2023) 125:111166. doi: 10.1016/j.intimp.2023.111166
72. Qin, L, Song, Y, Zhang, F, Wang, R, Zhou, L, Jin, S, et al. CRL4B complex-mediated H2AK119 monoubiquitination restrains Th1 and Th2 cell differentiation. Cell Death Differ. (2023) 30:1488–502. doi: 10.1038/s41418-023-01155-8
73. Tang, D, Liu, S, Sun, H, Qin, X, Zhou, N, Zheng, W, et al. All-trans-retinoic acid shifts Th1 towards Th2 cell differentiation by targeting NFAT1 signalling to ameliorate immune-mediated aplastic anaemia. Br J Haematol. (2020) 191:906–19. doi: 10.1111/bjh.16871
Keywords: weaned piglet, Lactiplantibacillus plantarum L47, inulin, enterotoxigenic Escherichia coli, colon, liver
Citation: Miao J, Cui L, Zeng H, Hou M, Wang J and Hang S (2024) Lactiplantibacillus plantarum L47 and inulin affect colon and liver inflammation in piglets challenged by enterotoxigenic Escherichia coli through regulating gut microbiota. Front. Vet. Sci. 11:1496893. doi: 10.3389/fvets.2024.1496893
Edited by:
Iram Liaqat, Government College University, PakistanReviewed by:
Neeta Agarwal, Indian Veterinary Research Institute (IVRI), IndiaShaukat Ali, Government College University, Pakistan
Irfana Liaqat, Government College University, Pakistan
Copyright © 2024 Miao, Cui, Zeng, Hou, Wang and Hang. This is an open-access article distributed under the terms of the Creative Commons Attribution License (CC BY). The use, distribution or reproduction in other forums is permitted, provided the original author(s) and the copyright owner(s) are credited and that the original publication in this journal is cited, in accordance with accepted academic practice. No use, distribution or reproduction is permitted which does not comply with these terms.
*Correspondence: Suqin Hang, c3VxaW5oYW5nNjlAbmphdS5lZHUuY24=