- 1Key Laboratory of Yak Breeding Engineering, Lanzhou Institute of Husbandry and Pharmaceutical Sciences, Chinese Academy of Agricultural Sciences, Lanzhou, China
- 2Department of Animal Science, Federal University Dutsin-Ma, Dutsin-Ma, Nigeria
- 3Key Laboratory of Veterinary Pharmaceutical Development, Ministry of Agricultural and Rural Affairs, Lanzhou Institute of Husbandry and Pharmaceutical Sciences, Chinese Academy of Agricultural Sciences, Lanzhou, China
Ruminant animals naturally emit methane gas owing to anaerobic microbial fermentation in the rumen, and these gases are considered major contributors to global warming. Scientists worldwide are attempting to minimize methane emissions from ruminant animals. Some of these attempts include the manipulation of rumen microbes using antibiotics, synthetic chemicals, dietary interventions, probiotics, propionate enhancers, stimulation of acetogens, manipulation of rumination time, vaccination, and genetic selection of animals that produce low methane (CH4). The majority of synthetic additives are harmful to both beneficial rumen microbes and the host or only temporarily affect methanogenesis. Phytogenic feed additives (PFAs) have recently emerged as the best alternatives to antibiotics and synthetic chemicals because of growing public concerns regarding drug resistance and the negative impacts of antibiotics and synthetic chemicals on humans, livestock, and the environment. These additives reduce methane production and improve the volatile fatty acid profile. In this review, we provide an overview of PFA sources and how their bioactive components affect the rumen microbiome to reduce methane emissions. Additionally, we highlight the mechanisms of action of PFAs as a whole, as well as some of their bioactive components. We also review some selected trees, herbs, shrubs, and forages and their roles in reducing methane emissions.
1 Introduction
The world’s human population is anticipated to reach almost 10 billion people by the year 2050; therefore, an increase in ruminant animal production is necessary to meet the demand for animal protein needs of humans by supplying daily meat and dairy products worldwide (1). This has resulted in the intensification of agriculture, especially livestock production, and consequently inflated the global index of methane (CH4) produced by livestock by almost 2.5-fold (2). Methane accounts for 16% of the global greenhouse gas emissions. It is estimated that ruminant animals contribute to 33% of the global methane emissions index (3). Approximately 81 million tons of enteric methane is produced annually by livestock worldwide. It is primarily emitted from the rumen and lower digestive tract when carbohydrates are fermented by microbes (4). These animals are among the largest producers of enteric methane, and they contribute to global warming by adding greenhouse gases to the ozone layer. This process is gaining attention worldwide for identifying rumen microbes that are important for methane production to develop the best methane mitigation strategy (5). Rumen fermentation produces a variety of beneficial products, including methane. Cattle alone contribute 15–20% of the global methane production every year (6). Methane is the most abundant hydrogen sink synthesized by methanogens in the rumen. In addition to contributing to global warming, enteric methane emissions contribute 8–9% of the total energy lost by ruminants, which, if not lost, can be used by animals for growth, meat, and milk production (7).
Most work done to reduce methane emissions in the 1950s focused on reducing feed energy loss, whereas recent efforts have focused on both energy savings and their effects on climate change. Despite the success of manipulating rumen fermentation using antibiotics and ionophores, their use has been limited by environmental and human health concerns (8). Because phytogenic feed additives (PFAs; additives derived from plants) contain many bioactive compounds, unlike antibiotics and ionophores, the global scenario has shifted toward the use of phytogenic feed additives rather than antibiotics or ionophores. This compound is capable of manipulating the microbiota in the rumen through more potent mechanisms of action, including inhibition of the activities of protozoa, methanogenic archaea, and some fiber degraders through its antimicrobial potential and decreasing hydrogen availability (9). PFAs have been reported to manipulate ruminal fermentation and to successfully reduce methane emissions from ruminants (10).
PFAs are increasingly being used in animal nutrition because of the negative effects of antibiotics and synthetic chemicals (11). These additives have sparked interest because of their potential to improve nutrient utilization and promote health (12, 13). PFA comprises various phytochemicals that are biologically active during fermentation. Various metabolic pathways are believed to mediate their antimicrobial, metabolic, immune, and antioxidant effects (14). PFAs have been tested in various ruminant models to manipulate enteric fermentation (15). The use of plant bioactive compounds (PBC) such as tannins, saponins, and essential oils for methane mitigation has been reviewed; however, most studies have focused on PBC rather than providing insight into the sources of these compounds. However, given the current trend and importance of research on climate change and global warming, more research and review are required. In this review, we explore the impact of PFAs on reducing methane emissions, with an emphasis on their effects on rumen ecology as well as the possible underlying mechanisms and factors affecting these effects.
2 Insight into the role of rumen microbial ecology on methanogenesis
Rumen microbes and ruminant animals have a symbiotic relationship. These microbes obtain their substrate when ruminant animals ingest feed and, in return, ferment the feed and supply valuable nutrients to the host, producing methane as a byproduct (Figure 1) (16). The microbial community in the rumen is one of the most diverse gut ecosystems hitherto described in the animal kingdom. It consists of anaerobic bacteria (1010–1011 organisms/mL), archaea (108–109 organisms/mL), ciliated protozoa (105–106 organisms/mL), anaerobic fungi (103–104 organisms/mL), and viral community that is largely uncharacterized (17). To date, only a few of these microbes in microbial ecologies have been cultured and characterized (18). The use of culture-based approaches to study ruminal content has decreased in recent years. However, the introduction of high-throughput sequencing techniques has allowed us to gain a better understanding of the rumen microbiome in different diets, species, and geographical locations (19). These advancements can provide a deeper understanding of the diverse microbial species in the rumen ecosystem. Using metagenomics, it will be much easier to determine which rumen microbial community is responsible for methane production. This information will enable scientists to develop the best methane mitigation strategy, which in turn will reduce the negative impacts of ruminant animals on the environment.
3 Phytogenic tools for reducing methane emissions and their effects on ruminal microbial ecology
Since the 18th century, the loss of energy in the rumen as CH4 has been well-documented in a journal titled “Zeitschrift für Biologie” (Journal of Biology), written by German Scientist Tappeiner in 1884 (20). Since then, scientists worldwide have been working to reduce CH4 emissions without affecting livestock growth and productivity. Owing to the greenhouse gas potential of CH4 and the importance of ruminant contributions, policymakers worldwide are currently seeking effective mitigation strategies. In recent years, numerous studies have been conducted to reduce ruminant CH4 emissions (21).
Recently, PFAs have attracted the attention of researchers worldwide. These additives have been reported to increase feed conversion efficiency; enhance growth, productivity, and animal health; and reduce CH4 emissions (22). PFAs have been tested by scientists and found to significantly reduce CH4 emissions, manipulate rumen microbial ecology, and change the fermentation dynamics of ruminants (Figure 2) (23). These additives include; plants, part of plants, plant oil extracts, trees, shrubs, grasses, and legumes. These PFAs are rich in plant bioactive compounds (PBC) such as saponins, tannins, organosulfur compounds, essential oils, flavonoids, propolis, terpenes, and glycosides.
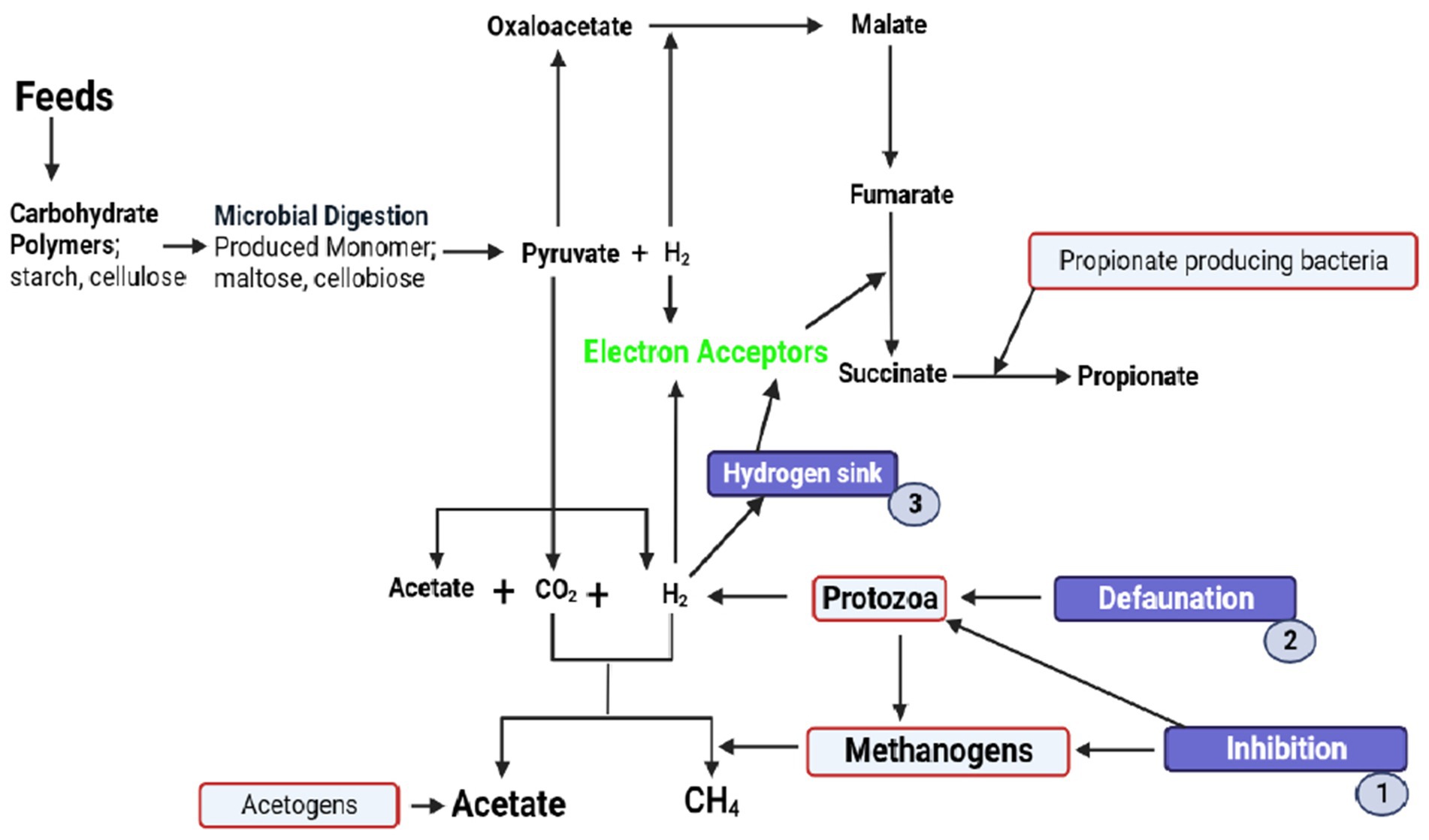
Figure 2. Biochemical pathways of methanogenesis affected by plant bioactive compounds to decrease methane production in the rumen. These bioactive compounds inhibit the activity of methanogens and protozoa (1). This bioactive compound decreases the number of protozoa (defaunation) (2). They also induce the rechanneling of metabolic hydrogen from CH4 to propionate (3).
3.1 Mechanism of action of phytogenic feed additives on rumen microbial cells
Compared to antibiotics, PFAs have a greater potential to modify the ruminal microbiome and reduce methane emissions by disrupting cell membranes, modulating signal transduction and gene expression pathways, inhibiting enzyme activity, and inhibiting bacterial colonization (24). Generally, PFAs increase the permeability and fluidity of cellular membranes, resulting in the efflux of metabolites and ions and leading to cell leakage and microbial death (Figure 3). Moreover, they can manipulate the rumen metabolism by increasing the permeability of a specific group of rumen bacteria (25). There are several possible mechanisms of action, including disruption of the cytoplasmic membrane, disruption of the proton motive force, electron flow, active transport mechanisms, and coagulation of the cell composition (26).
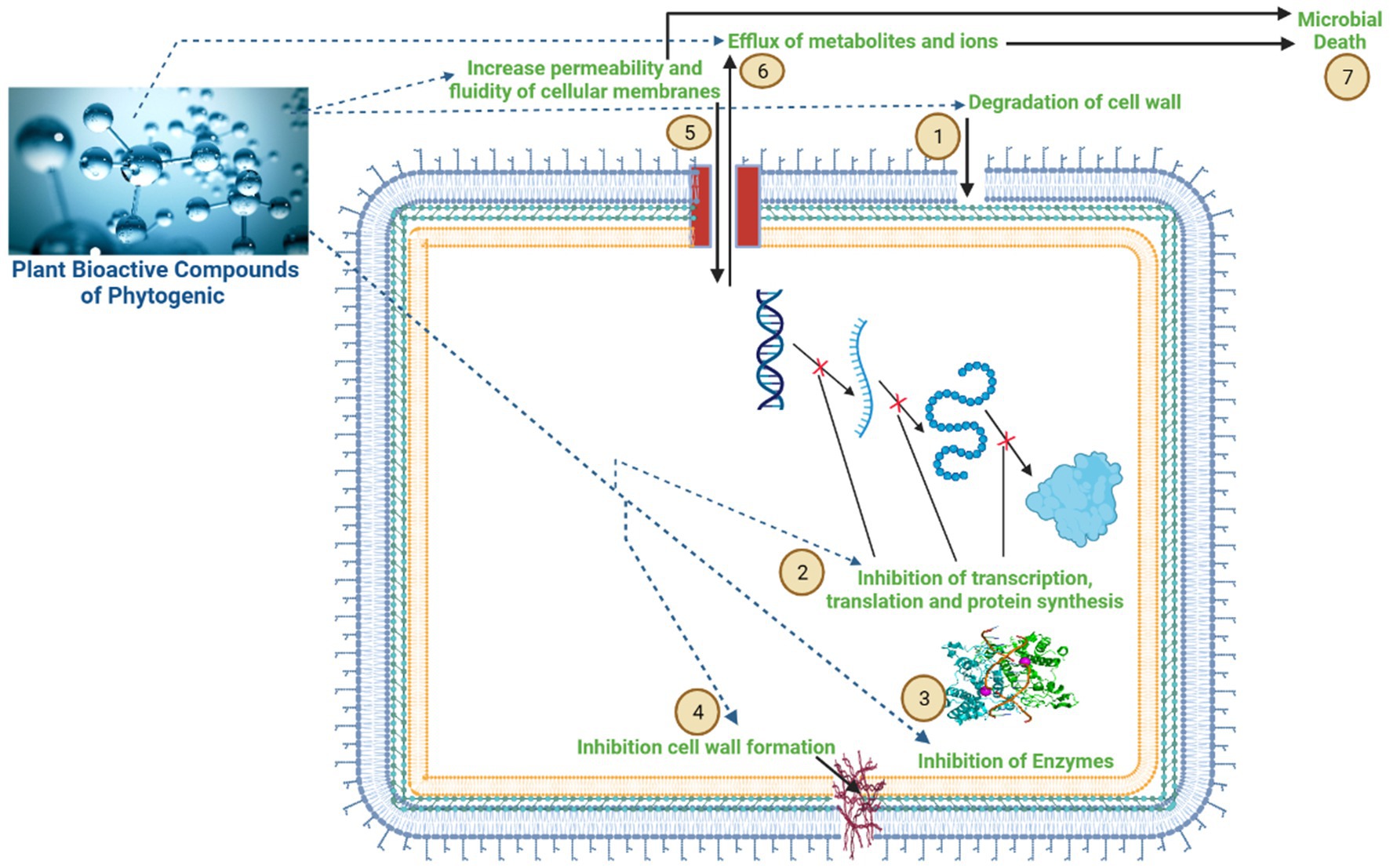
Figure 3. Mechanism of action of the bioactive compounds of phytogenic feed additives (PFAs) on rumen microbial cells. The bioactive compounds of phytogenic feed additives have a greater potential to modulate the ruminal microbiome and reduce CH4 emissions, as indicated in figure: (1) disruption of cell membranes; (2) inhibition of gene transcription, translation, and protein synthesis; (3) inhibition of enzyme activity; (4) inhibition of cell wall formation; (5) increasing permeability and fluidity of cellular membranes; (6) resulting in an influx of metabolites and ions, leading to cell leakage; and (7) microbial death.
3.2 Phytogenic feed additives: sources and effects
3.2.1 Trees, shrubs, and forages
Several trees, shrubs, and forages are used for methane mitigation because they are rich in bioactive compounds that can suppress methanogenic activity. Trees, shrubs, and forages contain many bioactive compounds including terpenes, saponins, cyanogenic glycosides, flavones, isoflavones, tannins, coumarins, and other phenolics (27). In addition to these, trees, shrubs, and forages provide an excellent source of protein. Therefore, feeding trees, shrubs, and forage can be beneficial protein sources and methane reducers (28). Bioactive compounds in these plants can manipulate the rumen microbial ecosystem, thereby reducing methane production (29). The mechanism of action of these bioactive compounds could have direct effects on methanogens, anti-protozoal effects (defaunation), or inhibition of fiber digestion, followed by a lower H2 supply to the methanogens (Table 1).
3.2.2 Trees and shrubs
3.2.2.1 Gliricidia (Gliricidia sepium)
Gliricidia is a member of the family Fabaceae (legume family), subfamily Faboideae (Papilionoideae), and tribe Robinieae. Medium-sized semi-deciduous trees with broad canopies, native to Central America and perhaps northern South America, typically grow to a height of 10 m (occasionally 15 m) (30). Gliricidia foliage is rich in tannins and saponins, making it useful for mitigating methane emissions (31). The effect of long-term supplementation with G. sepium foliage reduced CH4 production in heifers, and this response persisted over time, without affecting the microbial population and VFA concentration and a slight reduction in CPD digestibility (32). Zain et al. (33) reported that 30% supplementation with Gliricidia sepium decreased methane gas production (from 27.22 mM to 13.13 mM) and the number of protozoa (from 6.3 × 105 cell/ml rumen fluid to 4.7 × 105 cell/mL rumen fluid) while increasing digestibility and rumen fermentation parameters. A diet supplemented with 20% Gliricidia sepium leaf meal has the potential to modify rumen fermentation, resulting in improved post-ruminal nutrient utilization (34).
The incorporation of Gliricidia sepium into animal diets reduces in vitro methane production and the population of ruminal protozoa (35).
3.2.2.2 Calliandra (Calliandra calothyrsus)
Calliandra are shrubs native to the American continent that belong to the Mimosoideae family. These shrubs are rich in tannins (36). Calliandra calothyrsus is notable for its high tannin content, making it a significant candidate for mitigating methane emissions (37). Tiemann et al. (38) reported that adding tannin-rich Calliandra plants reduced methane emissions by 24% per day as well as per unit of feed and energy intake. They believed that the mechanism of this reduction was a reduction in the available H2 required for methane production by donating electrons to H2 to form a stable radical. In vitro, supplementation with C. calothyrsus reduces methane production without any negative effects on rumen fermentation parameters (37). According to Ridwan et al. (39), 50% silage containing C. calothyrsus decreased enteric CH4 production by reducing the total number of methanogens and goats supplemented Methanobacteriales but decreased bacterial diversity and organic matter digestibility. PE dairy with C. calothyrsus had reduced enteric methane emissions and improved milk production (40). Mwangi et al. (41) reported that replacing 40% of a protein-deficient basal diet with Calliandra calothyrsus reduces enteric methane emissions in both absolute terms and intensity. Calliandra calothyrsus, when used as a two-thirds replacement for protein in lamb diets, partially reduces methane emissions due to associated reductions in N and energy retention (38).
3.2.2.3 Mulberry (Morus alba)
Mulberry is a fast-growing deciduous tree of the Moraceae family that is native to India and China’s Himalayan foothills (42). The leaves of mulberry trees are widely used as livestock feed because of their high crude protein content and metabolizable energy. In addition, they are rich in flavonoids, a plant bioactive compound known to reduce enteric methane emissions (43). Morus alba is significant in reducing rumen methanogenesis because it contains long-chain unsaturated fatty acids that can effectively decrease methane production in the rumen (44). The in vitro supplementation of mulberry leaf flavonoids at a concentration of 15 mg/100 g decreased methane emission, improved dry matter digestibility, and improved the Total Volatile Fatty Acids (TVFA) profile of sheep (45). Adding 300 g of Morus alba to a dairy cow’s diet changed the microbial community and fermentation process in the rumen, which increased propionate production and reduced methane emissions (46). Morus alba is a promising candidate for reducing enteric methane emissions while providing an optimal level of nitrogen when used as a supplement to low-quality forages (47). Considering its potential, further research is needed to test its effect on reducing methane emissions while improving the TVFA profile of ruminants.
3.2.2.4 Italian plumeless thistle (Carduus pycnocephalus)
This plant belongs to the Astraceae family and genus Carduus (48). This plant is also popularly used in Traditional Chinese Medicine to treat various human diseases, such as colds, rheumatism, and stomachache (49). Carduus contains numerous classes of phytochemicals, including lignans, flavonoids, alkaloids, sterols, triterpenes, coumarins, essential oils, hexadecanoic acid, sterols, and triterpenes (50). The leaves of C. pycnocephalus contain tannins and saponins (51, 52). C. pycnocephalus decreases methane production in a hay-based diet while improving microbial protein synthesis in dairy cattle (51, 52). An in vitro screening of 450 plants for their potential anti-methanogenic effects concluded that C. pycnocephalus was the first among the six selected species, and had the potential to reduce methane emissions by more than 25% without adverse effects on digestibility, total volatile fatty acids, and gas and production (53). Owing to the antimicrobial properties of C. pycnocephalus, its mode of action in reducing methanogenesis may be its effect in reducing the number of rumen methanogens.
3.2.2.5 Chinese peony (Paeonia lactiflora)
Chinese peony (Paeonia lactiflora) is commonly known as chishao (赤芍) in China. More than 1,200 years ago, P. lactiflora root was used in Traditional Chinese Medicine (54). Glucosides of Peony, or Total Glucosides of Peony (TGP), are extracted from P. lactiflora and contain almost 15 components, including albiflorin, benzoyl paeoniflorin, galloylpaeoniflorin, lactoferrin, oxybenzone-paeoniflorin, oxypaeoniflorin, paeony, phenol, phonolite, paeoniflorin, paeoniflorin, paeoniflorin, paeoniflorin, and paeoniflorin (55). The structures of most of these extracts are monoterpene glucosides, among which paeoniflorin is a water-soluble compound, the most abundant (>90%) has a molecular weight of 480.45 and has the highest pharmacological effects among all TGP in both in vitro and in vivo studies (54). P. lactiflora extracts have anti-methanogenic effect (56). P. lactiflora reduces methane emissions by 8–53% in cattle (57). Methane reduction is caused by the inhibition of gram-positive bacteria (58). Considering its potential antimicrobial and anti-methanogenic effects, this plant requires further investigation.
3.2.2.6 Leucaena (Leucaena leucocephala)
The Leucaena tree belongs to the family Mimosaceae, genus; Leucaena and the best-known species is Leucocephala it has many common names Worldwide, in China, it is called “Yin ho huan” (59). Phytochemical analysis of Leucaena leaves revealed the presence of almost 30 compounds including tannins, squalene, phytol, phylobatanins, alkaloids, cardiac glycosides, flavonoids, saponins, and glycosides (60). Phytochemicals in Leucaena have been shown to have several anti-methanogenic effects (60). Leucaena decreased methane production in crossbred cows housed in an open-circuit respiration chamber (61). However, this treatment did not affect the microbial community. Supplementation with Leucaena decreased methane emissions by up to 20% in Colombian Lucerna heifers (62). Another 20% decrease in methane emissions has been reported in grazing cows consuming leucaena pastures in Australia (63). Leucaena is a major source of condensed tannins (CT), and in vitro studies of CT extracts from Leucaena resulted in 99 and 83% reductions in the total number of methanogens and protozoa, respectively (64).
3.2.3 Forages
3.2.3.1 Brassica forages
In temperate countries, Brassica is an annual plant that has been traditionally used in grazing systems to cover periods of feed deficits for ruminants. Brassica forage crops have four main types that are usually used worldwide to provide food for ruminant livestock feeds during shortage, this includes; kale (Brassica oleracea spp. acephala), turnips (Brassica rapa spp. rapa), swedes (Brassica napus spp. napobrassica), and forage rape (Brassica napus spp. biennis) (65). Brassica leaves, stems, bulbs, and roots are used as phytogenic additives (66). They contain bioactive compounds such as S-methyl-cysteine sulfoxide (SMCO) and glucosinolates (65). Both SMCO and glucosinolates reduce the available H2 for methane production through hydrogen sulfide scavenging mechanisms (67). Brassica forages were reported to reduce CH4 emissions in sheep by 37%; however, the experiment did not examine the effect on rumen microbial ecology (68). However, dairy cows fed Brassica forage did not show any methane mitigation effects, and the protozoal count did not significantly differ from those fed 250 g/kg DM grains as a control diet (69). This may be due to species variation or the methane measurement method used. However, Sun (66) reported that Brassica is rich in glucosinolates (GSLs) when ruminants consume Brassica forages, which are broken down in the rumen, resulting in absorption into the blood, which stimulates the secretion of thyroid hormone FT3 in ruminants, and the altered thyroid hormone concentration changes rumen physiology. This would alter the mean retention time of digestion in the rumen, resulting in a reduction in methane emissions.
3.2.3.2 Alfalfa (Medicago sativa L)
Alfalfa (Medicago sativa), also known as lucerne, is a perennial flowering legume belonging to the Fabaceae family. It is the most important legume forage species in the world (70). Alfalfa is rich in saponins, which are known to reduce methane emissions. Kozłowska et al. (71) reported that ensiled Verko and Kometa alfalfa varieties (rich in saponins) reduced methane production without adversely affecting fermentation parameters. Dietary inclusion of alfalfa hay in crossbred Simmental cattle feed improves nitrogen utilization efficiency and reduces methane emissions (72). Sheep-fed alfalfa hay as a substitute for concentrate decreases CH4 emissions, digestibility, and urinary N and NH4 + -N outputs (73). A study conducted by Hironaka et al. (74) indicated that cattle fed pelleted alfalfa hay produced less methane than those fed chopped alfalfa hay.
3.2.3.3 Clover (Trifolium species)
Clover belongs to the Fabaceae family, genus Trifolium, and has approximately 240 species distributed over the temperate and subtropical regions of the Mediterranean Basin, western North America, and eastern Africa (75). Several flavonoids, saponins, chloramines, and phenolic acids have been found in Trifolium plants (76). Methane yield (g/kg DM) was significantly lower in cattle-fed red clover silage (17.8 ± 3.17) than in those fed grass silage (77). A linear increase in dry matter intake (DMI) and reduced methane output per kilogram of DM consumed were observed when white clover was increased in dairy cattle diets (78). The methane yield per kilogram of dry matter and digestible organic matter intake was lower for heifers fed red and white clover silage (79). Based on in vitro experiments, white clover leaves containing soluble CTs of 1.6–2.4% DM reduced methane production by 19% (p ≤ 0.01) and ammonia production by 60% (80). Dairy cattle fed white clover pastures produce less CH4 than those fed ryegrass pastures in small-scale dairy systems (81). Navarro-Villa et al. (82) reported that red clover showed reduced in vitro rumen methane output compared to that of perennial ryegrass. In a study using portable accumulation chambers, sheep that graze subterranean clover produced lower daily CH4 emissions (23.5 g/day) compared with sheep grazing lucerne (27.3 g/day) and perennial ryegrass (32.3 g/day) (83).
3.2.3.4 Chinese Lespedeza (Sericea lespedeza)
Lespedeza is a perennial herb in the family Leguminosae (Fabaceae), which is native to Japan, the Korean Peninsula, China, the Himalayas, Afghanistan, and Malaysia. Lespedeza is rich in condensed tannins and other phenolics (84). Regardless of the feeding level, goats fed CT-containing Lespedeza forage showed decreased CH4 emissions (85). Substituting Eragrostis curvula hay with 60% S. lespedeza on a DM basis resulted in the greatest reduction in CH4 yield (21.4%) compared to a diet of 100% Eragrostis curvula (86). Dietary inclusion of S. lespedeza increases propionate production and reduces CH4 production in the rumen (87). Liu et al. (88) reported that Alpine doelings fed on S. lespedeza forage emit less methane compared to the control. A study was conducted to assess nutrient digestibility, volatile fatty acid (VFA) concentrations, microbial protein synthesis, bacterial nitrogen (N) efficiency, and enteric methane (CH4) production in four grass-legume diets rich in condensed tannins (CT) (alfalfa, birdsfoot trefoil, crown vetch, and S. lespedeza). The results indicated that the lowest total CH4 production was observed in the S. lespedeza diet (89).
3.2.4 Plant oil extracts
Plant oil extracts are high in lipids, making them an excellent option for mitigating methane emissions (Table 1). Numerous studies have indicated that the addition of oils to ruminant diets reduces methane production (90). This supplementation decreases the number of protozoa and methanogens in the rumen and bio-hydrogenates unsaturated fatty acids, thereby reducing methane production (91). The shift from carbohydrates to lipids in ruminant diets modifies gas production in the rumen, reducing CH4 emissions (92, 93).
3.2.4.1 Rapeseed oil
Rapeseed is the third most popular vegetable oil in the world and is extracted from rape (canola) brassica forage. Rapeseed oil is low in erucic acid and glucosinolates (94). The inclusion of rapeseed oil in the diet of dairy cows decreased enteric CH4 emissions and modified the microbial community structure without affecting the total counts of bacteria, archaea, or ciliate protozoa (95). Cattle supplemented with canola oil (rapeseed oil) and nitrate reduce enteric methane emissions and protozoal populations in the rumen (96). Supplementation of nursing dairy cows with 5% rapeseed oil to nursing dairy cows reduced 23% of CH4 emissions with no effect on archaea and bacterial abundance (97). Dietary supplementation of rapeseed (41 g oil/kg DM) decreased daily CH4 emissions from lactating dairy cows by up to 22.5%, which increased the relative abundance of Methanosphaera and Succinivibrionaceae in the rumen and decreased the abundance of Bifidobacteriaceae (98). An in vitro experiment reported a decrease in the population of Thermoplasmata archaea (a methylotrophic methanogen) in the rumen after adding rapeseed oil to silage (99). Growing cattle supplemented with a diet containing 46 g of rapeseed oil/kg of diet DM decreased CH4 emissions, but reduced feed intake (100).
3.2.4.2 Camelina sativa oil
Camelina sativa oil is a rich source of unsaturated fatty acids, particularly linoleic, linolenic, and oleic acids (101). The rich unsaturated fatty acid profile of Camelina sativa oil makes it a very good supplement for ruminants because it has high-quality fat (102). In addition to being rich in unsaturated fatty acids, it also has a rich antioxidant profile (102). Researchers have established that oilseeds are generally one of the most effective ways to reduce enteric CH4 production from ruminants, as they can mitigate CH4 emissions by directly inhibiting rumen protozoa and methanogens while increasing the bio-hydrogenation of polyunsaturated fatty acids to serve as a sink for hydrogen produced by rumen microbes. Supplementation with C. sativa oil at different levels in an in vitro study significantly decreased CH4 production (15). Camelina oil at all levels significantly affected ammonia nitrogen and microbial protein in all rations because it altered the proportions of individual ruminal volatile fatty acids and decreased CH4 production by altering the total number of bacteria, protozoa, and methanogens (103). Dietary supplements of camelina oil in Finnish Ayrshire cows in vivo decreased ruminal CH4 and CO2 production, but there was no change in the total number of bacteria, methanogens, protozoa, and fungi in the rumen (104).
3.2.4.3 Garlic oil
Garlic oil was produced from ground garlic cloves and collected as a distillate from the vapor when the cloves were heated at a temperature of 100°C. Garlic oil is a mixture of various bioactive organosulfur compounds; including diallyl disulfide (C6H10S2), diallyl sulfide (C6H10S), allicin (C6H10S2O), and allyl mercaptan (C3H6S) and others (105). This metabolic profile makes it difficult to determine the exact mechanism of fermentation in the rumen. Both gram-positive and gram-negative bacteria are sensitive to the antibacterial effects of garlic oil (106). In an in vitro experiment, garlic oil was reported to decrease methane emissions by 74%, increase propionate and butyrate proportions, and decrease acetate and branch-chain VFA proportions (107). Garlic oils and their components have been found to modify fermentation parameters in the rumen and inhibit methane production by reducing the abundance of protozoa (108).
3.2.4.4 Palm oil
Palm oil is an edible vegetable oil extracted from the fruit of palm oil trees by squeezing or crushing fleshy fruits or kernels. It contains saturated fatty acids (palmitate, 44.3%; stearate, 4.6%; myristate, 1%), monounsaturated fatty acids (oleic acid, 38.7%), and polyunsaturated fatty acids (linoleic acid, α-linoleic acid 10.5%, and others 0.9%) (109). Recent research on supplementation of three levels of palm oil (20, 40, and 60 g/kg) to heifers fed low-quality grass on enteric CH4 emissions were reported, the results show that total daily CH4 production decreased by 4% for every 10 g/kg palm oil added while feed conversion efficiency, apparent digestibility, intake of nutrients were not affected by the inclusion (110). It has also been reported that the addition of 4% palm oil decreases in vitro methane production and the number of ciliate protozoa (111).
3.3 Mechanism of actions of plant bioactive compounds (PBC) and their role in reducing methane emissions
PBCs are bioactive compounds that have various effects on plants and other living organisms. Many PBCs exhibit anti-methanogenic, antioxidant, antimicrobial, anti-inflammatory, anti-helminthic, anticoagulant, antidiabetic, and lipid-lowering properties (112). They are biologically active metabolites that can exert beneficial effects on methane emission, feed digestion, rumen fermentation productivity, and the health of livestock animals (113).
These compounds were extracted from the plants. These include tannins, saponins, and essential oils that affect methanogenesis by inhibiting the growth, development, and activities of the methanogen population, both directly and indirectly, by reducing the number of protozoa associated with methanogens (Table 2). They may also result in a shift toward propionate production, which reduces hydrogen competition, thereby affecting methanogenesis (23). PBC additives can be used instead of antibiotics in ruminants owing to their antibacterial properties against ruminal bacteria, protozoa, and methanogens (114). These metabolites are believed to have beneficial effects on livestock end products by altering fermentation in the rumen without causing microbial resistance (115).
These phytochemicals can modify the rumen microbiome to alter its physiology because of their excellent antimicrobial activity (116). Numerous experiments on the potential effects of these phytochemicals on fermentation have been conducted both in vitro and in vivo, and have been found to significantly improve feed digestibility and decrease methanogenesis in the rumen (21). Despite various strategies to modify the microbiome of the rumen, PBC has significant potential to replace antibiotics in modifying rumen ecology and decreasing methane production through various mechanisms used by antimicrobial compounds (117). Some of the recognized mechanisms of action include disruption of proton motive force, disruption of cytoplasmic membranes, active transport mechanisms, coagulation of cell composition, and electron flow (26). PBC also significantly affects rumen microflora, resulting in the modification of fermentation and improved productivity of livestock (117).
Several PBCs, including tannins, saponins, essential oils (EO), flavonoids, and propolis have been found to have a significant impact on methanogens, protozoal population, feed conversion efficiency, absorption, and fermentation parameters as well as reducing CH4 emissions from animals (15) (see Table 3).
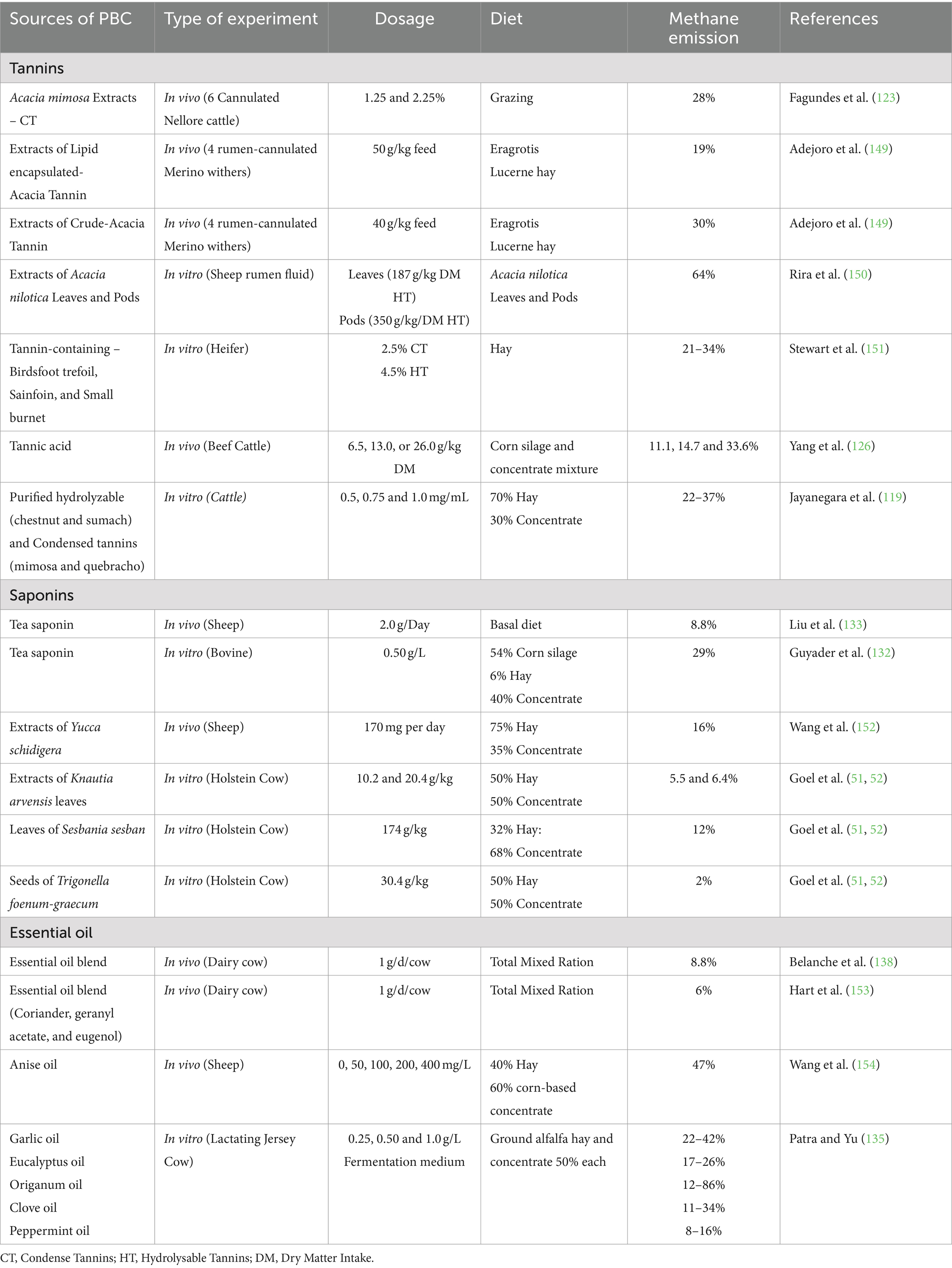
Table 3. Results from recent research on the effect of plant bioactive compounds (PBC) on methane emission in the rumen.
4 Conclusion
This review highlights various phytogenic feed additives capable of changing the rumen microbial ecology and reducing methane production. Trees, shrubs, and legumes are the most effective sources of phytogenic substances that reduce methane while improving the volatile fatty acid profile of ruminants because they contain numerous bioactive compounds. Most of the results in this review are from in vitro experiments; however, to understand the efficiency of phytogenic substances and their effects on methanogenesis, animal performance, animal health and welfare, rumen ecology, safety of phytogenic substances, environmental influence, quantity and quality of animal products, and applicability of phytogenic additives, in vivo studies over a longer period and across various parts of the world are needed. These are paramount to providing livestock farmers, policymakers, and climate change agencies with reliable information on the precise effect of phytogenic feed additives in reducing methane emissions while improving animal production.
Author contributions
IB: Conceptualization, Methodology, Software, Writing – original draft. WX: Writing – review & editing. XD: Funding acquisition, Project administration, Supervision, Writing – review & editing.
Funding
The author(s) declare financial support was received for the research, authorship, and/or publication of this article. This research was funded by the Key projects of the Grant number: 22R5RA037, Gansu Provincial Key Talent Program (No. 220299999999382) and International cooperation and exchange program of the National Natural Science Foundation of China (No. 3231101761).
Acknowledgments
The authors acknowledge the financial support from the Key Laboratory of Yak Breeding Engineering, Lanzhou Institute of Husbandry and Pharmaceutical Sciences, Chinese Academy of Agricultural Sciences, Lanzhou, Gansu Province, People’s Republic of China.
Conflict of interest
The authors declare that the research was conducted in the absence of any commercial or financial relationships that could be construed as a potential conflict of interest.
Publisher’s note
All claims expressed in this article are solely those of the authors and do not necessarily represent those of their affiliated organizations, or those of the publisher, the editors and the reviewers. Any product that may be evaluated in this article, or claim that may be made by its manufacturer, is not guaranteed or endorsed by the publisher.
References
1. Rojas-Downing, MM, Nejadhashemi, AP, Harrigan, T, and Woznicki, SA. Climate change and livestock: impacts, adaptation, and mitigation. Clim Risk Manag. (2017) 16:145–63. doi: 10.1016/j.crm.2017.02.001
2. Van Lingen, HJ, Niu, M, Kebreab, E, Valadares Filho, SC, Rooke, JA, Duthie, C-A, et al. Prediction of enteric methane production, yield and intensity of beef cattle using an intercontinental database. Agric Ecosyst Environ. (2019) 283:106575. doi: 10.1016/j.agee.2019.106575
3. Yusuf, RO, Noor, ZZ, Abba, AH, Hassan, MAA, and Din, MFM. Methane emission by sectors: a comprehensive review of emission sources and mitigation methods. Renew Sust Energ Rev. (2012) 16:5059–70. doi: 10.1016/j.rser.2012.04.008
4. Tyagi, N, Mishra, DB, Vinay, V, and Kumar, S. Feasible strategies for enteric methane mitigation from dairy animals In: S Mahajan and A Varma, editors. Animal manure: agricultural and biotechnological applications. Cham: Springer (2022). 335–54.
5. Black, JL, Davison, TM, and Box, I. Methane emissions from ruminants in Australia: mitigation potential and applicability of mitigation strategies. Animals. (2021) 11:951. doi: 10.3390/ani11040951
6. Houghton, JT, Ding, Y, Griggs, DJ, Noguer, M, van der Linden, PJ, Dai, X, et al. Climate change 2001: The scientific basis: Contribution of working group I to the third assessment report of the intergovernmental panel on climate change. Cambridge, Cambridge University Press: (2001).
7. Morgavi, D, Cantalapiedra-Hijar, G, Eugène, M, Martin, C, Noziere, P, Popova, M, et al. Reducing enteric methane emissions improves energy metabolism in livestock: is the tenet right? Animal. (2023) 17:100830. doi: 10.1016/j.animal.2023.100830
8. McGuffey, R. A 100-year review: metabolic modifiers in dairy cattle nutrition. J Dairy Sci. (2017) 100:10113–42. doi: 10.3168/jds.2017-12987
9. Soltan, YA, Natel, AS, Araujo, R, Morsy, AS, and Abdalla, AL. Progressive adaptation of sheep to a microencapsulated blend of essential oils: ruminal fermentation, methane emission, nutrient digestibility, and microbial protein synthesis. Anim Feed Sci Technol. (2018) 237:8–18. doi: 10.1016/j.anifeedsci.2018.01.004
10. Soltan, YA, and Patra, AK. Ruminal microbiome manipulation to improve fermentation efficiency in ruminants In: AK Patra, editor. Animal feed science and nutrition-production, health and environment. London: IntechOpen (2021)
11. Ahsan, U, Adabi, SG, Sayın Özdemir, Ö, Sevim, Ö, Tatlı, O, Kuter, E, et al. Growth performance, carcass yield and characteristics, meat quality, serum biochemistry, jejunal histomorphometry, oxidative stability of liver and breast muscle, and immune response of broiler chickens fed natural antioxidant alone or in combination with Bacillus licheniformis. Arch Anim Breed. (2022) 65:183–97. doi: 10.5194/aab-65-183-2022
12. Durmic, Z, and Blache, D. Bioactive plants and plant products: effects on animal function, health and welfare. Anim Feed Sci Technol. (2012) 176:150–62. doi: 10.1016/j.anifeedsci.2012.07.018
13. Rochfort, S, Parker, AJ, and Dunshea, FR. Plant bioactives for ruminant health and productivity. Phytochemistry. (2008) 69:299–322. doi: 10.1016/j.phytochem.2007.08.017
14. FrAnKIČ, T, Voljč, M, Salobir, J, and Rezar, V. Use of herbs and spices and their extracts in animal nutrition. Acta Agric Slov. (2009) 94:95–102. doi: 10.14720/aas.2009.94.2.14834
15. Hassan, F-U, Arshad, MA, Ebeid, HM, Rehman, MS-U, Khan, MS, Shahid, S, et al. Phytogenic additives can modulate rumen microbiome to mediate fermentation kinetics and methanogenesis through exploiting diet–microbe interaction. Front Vet Sci. (2020) 7:575801. doi: 10.3389/fvets.2020.575801
16. Mizrahi, I. Rumen symbioses In: E Rosenberg, EF DeLong, S Lory, E Stackebrandt, and F Thompson, editors. The prokaryotes: Prokaryotic biology and symbiotic associations. Berlin Heidelberg: Springer-Verlag (2013). 533–44.
17. Weimer, PJ. Redundancy, resilience, and host specificity of the ruminal microbiota: implications for engineering improved ruminal fermentations. Front Microbiol. (2015) 6:296. doi: 10.3389/fmicb.2015.00296
18. Morgavi, DP, Kelly, W, Janssen, P, and Attwood, G. Rumen microbial (meta) genomics and its application to ruminant production. Animal. (2013) 7:184–201. doi: 10.1017/S1751731112000419
19. Tapio, I, Snelling, TJ, Strozzi, F, and Wallace, RJ. The ruminal microbiome associated with methane emissions from ruminant livestock. J Anim Sci Biotechnol. (2017) 8:1–11. doi: 10.1186/s40104-017-0141-0
20. Armsby, HP. The use of energy values in the computation of rations for farm animals. Washington DC, USA: US Department of Agriculture (1916).
21. Patra, A, Park, T, Kim, M, and Yu, Z. Rumen methanogens and mitigation of methane emission by anti-methanogenic compounds and substances. J Anim Sci Biotechnol. (2017) 8:1–18. doi: 10.1186/s40104-017-0145-9
22. Flachowsky, G, and Lebzien, P. Effects of phytogenic substances on rumen fermentation and methane emissions: a proposal for a research process. Anim Feed Sci Technol. (2012) 176:70–7. doi: 10.1016/j.anifeedsci.2012.07.009
23. Cieslak, A, Szumacher-Strabel, M, Stochmal, A, and Oleszek, W. Plant components with specific activities against rumen methanogens. Animal. (2013) 7:253–65. doi: 10.1017/s1751731113000852
24. Bodas, R, Prieto, N, Garcia-Gonzales, R, Andres, S, Giraldez, FJ, and Lopez, S. Manipulation of rumen fermentation and methane production with plant secondary metabolites. Anim Feed Sci Technol. (2012) 176:78–93. doi: 10.1016/j.anifeedsci.2012.07.010
25. Omojate Godstime, C, Enwa Felix, O, Jewo Augustina, O, and Eze Christopher, O. Mechanisms of antimicrobial actions of phytochemicals against enteric pathogens–a review. J Pharm Chem Biol Sci. (2014) 2:77–85.
26. Lillehoj, H, Liu, Y, Calsamiglia, S, Fernandez-Miyakawa, ME, Chi, F, Cravens, RL, et al. Phytochemicals as antibiotic alternatives to promote growth and enhance host health. Vet Res. (2018) 49:1–18. doi: 10.1186/s13567-018-0562-6
27. Tava, A, Biazzi, E, Ronga, D, Pecetti, L, and Avato, P. Biologically active compounds from forage plants. Phytochem Rev. (2022) 21:471–501. doi: 10.1007/s11101-021-09779-9
28. Wina, E. The use of plant bioactive compounds to mitigate enteric methane in ruminants and its application in Indonesia. Wartazoa. (2012) 22:24–34.
29. Dhanasekaran, DK, Dias-Silva, TP, Filho, ALA, Sakita, GZ, Abdalla, AL, Louvandini, H, et al. Plants extract and bioactive compounds on rumen methanogenesis. Agrofor Syst. (2020) 94:1541–53. doi: 10.1007/s10457-019-00411-6
30. Wafaey, AA, El Hawary, SSE, and Abdelhameed, MF. An overview on Gliricidia sepium in the pharmaceutical aspect: a review article. Egypt J Chem. (2023) 66:479–96.
31. Molina-Botero, IC, Arroyave-Jaramillo, J, Valencia-Salazar, S, Barahona-Rosales, R, Aguilar-Pérez, CF, Burgos, AA, et al. Effects of tannins and saponins contained in foliage of Gliricidia sepium and pods of Enterolobium cyclocarpum on fermentation, methane emissions and rumen microbial population in crossbred heifers. Anim Feed Sci Technol. (2019) 251:1–11. doi: 10.1016/j.anifeedsci.2019.01.011
32. Molina-Botero, IC, Montoya-Flores, MD, Zavala-Escalante, LM, Barahona-Rosales, R, Arango, J, and Ku-Vera, JC. Effects of long-term diet supplementation with Gliricidia sepium foliage mixed with Enterolobium cyclocarpum pods on enteric methane, apparent digestibility, and rumen microbial population in crossbred heifers. J Anim Sci. (2019) 97:1619–33. doi: 10.1093/jas/skz067
33. Zain, M, Putri, E, Rusmana, W, and Erpomena Makmur, M. Effects of supplementing Gliricidia sepium on ration based ammoniated rice straw in ruminant feed to decrease methane gas production and to improve nutrient digestibility (in-vitro). Int J Adv Sci Eng Inf Technol. (2020) 10:724–9. doi: 10.18517/ijaseit.10.2.11242
34. Adelusi, O, Idowu, O, Adebayo, K, Balogun, M, and Oni, A. In vitro degradability of diets containing varying levels of Gliricidia sepium and cassava leaf meals (2022).
35. Galindo, J, González, N, Marrero, Y, Sosa, A, Ruiz, T, Febles, G, et al. Effect of tropical plant foliage on the control of methane production and in vitro ruminal protozoa population. Cuban J Agric Sci. (2014) 48:21–37.
36. Cardoso-Gutierrez, E, Aranda-Aguirre, E, Robles-Jimenez, L, Castelán-Ortega, O, Chay-Canul, A, Foggi, G, et al. Effect of tannins from tropical plants on methane production from ruminants: a systematic review. Vet Anim Sci. (2021) 14:100214. doi: 10.1016/j.vas.2021.100214
37. Muhlisin, M. Calliandra calothyrsus as tannins source for in vitro methane production inhibitor agents. International seminar on tropical animal production (ISTAP) (2017)
38. Tiemann, TT, Lascano, CE, Wettstein, H-R, Mayer, A, Kreuzer, M, and Hess, H. Effect of the tropical tannin-rich shrub legumes Calliandra calothyrsus and Flemingia macrophylla on methane emission and nitrogen and energy balance in growing lambs. Animal. (2008) 2:790–9. doi: 10.1017/S1751731108001791
39. Ridwan, R, Rusmana, I, Widyastuti, Y, Wiryawan, K, Prasetya, B, Sakamoto, M, et al. Methane mitigation and microbial diversity of silage diets containing Calliandra calothyrsus in a rumen in vitro fermentation system. Media Peternakan. (2014) 37:121–8. doi: 10.5398/medpet.2014.37.2.121
40. Sukmawati, M, Permana, I, Thalib, A, and Kompiang, S. Effect of complete rumen modifier (CRM) and Calliandra calothyrus on productivity and enteric methane productions of PE dairy goat. J Anim Vet Sci. (2011) 16:173–83.
41. Mwangi, PM, Eckard, R, Gluecks, I, Merbold, L, Mulat, DG, Gakige, J, et al. Supplementation of a tropical low-quality forage with Calliandra calothyrsus improves sheep health and performance, and reduces methane emission. Front Anim Sci. (2024) 5:1296203. doi: 10.3389/fanim.2024.1296203
42. Rohela, GK, Jogam, P, Mir, MY, Shabnam, AA, Shukla, P, Abbagani, S, et al. Indirect regeneration and genetic fidelity analysis of acclimated plantlets through SCoT and ISSR markers in Morus alba L. cv. Chinese white. Biotechnol Rep (Amst). (2020) 25:e00417. doi: 10.1016/j.btre.2020.e00417
43. Hassan, F-U, Arshad, MA, Li, M, Rehman, MS-U, Loor, JJ, and Huang, J. Potential of mulberry leaf biomass and its flavonoids to improve production and health in ruminants: mechanistic insights and prospects. Animals. (2020) 10:2076. doi: 10.3390/ani10112076
44. González, N, Galindo, J, Aldana, AI, Moreira, O, Sarduy, L, Abdalla, L, et al. Evaluation of different varieties of mulberry (Morus alba) in the control of the methanogenesis in buffalo rumen liquid. Cuban J Agric Sci. (2010) 44:37–41.
45. AL-Bayati, M, and Hassan, A. Effects of in vitro supplementation of mulberry leaf flavonoids on microbial flora, methanogenesis and fermentative products in rumen fluid of sheep. J Res Ecol. (2018) 6:2067–77.
46. Hnokaew, P, Sringarm, K, Chuammitri, P, Arjin, C, Lumsangkul, C, Mekchay, S, et al. Influence of dietary mulberry (Morus alba L.) leaf supplementation on production performance, blood metabolites, rumen fermentation characteristics and ruminal bacteria community in lactating dairy cows. Ital J Anim Sci. (2024) 23:1031–43. doi: 10.1080/1828051X.2024.2375394
47. Tirfessa, G, and Tolera, A. Comparative evaluation of chemical composition, in vitro fermentation and methane production of selected tree forages. Agrofor Syst. (2020) 94:1445–54. doi: 10.1007/s10457-019-00391-7
48. Chaudhary, S. Flora of the Kingdom of Saudi Arabia, II, parts 1–3. Riyadh: Ministry of Agriculture and Water (2000).
49. Esmaeili, A, Rustaiyan, A, Nadimi, M, Masoudi, S, Tadayon, F, Sedaghat, S, et al. Volatile constituents of Centaurea depressa MB and Carduus pycnocephalus L. two compositae herbs growing wild in Iran. J Essent Oil Res. (2005) 17:539–41. doi: 10.1080/10412905.2005.9698988
50. Rustaiyan, A, and Faridchehr, A. Constituents and biological activities of selected genera of the Iranian Asteraceae family. J Herb Med. (2021) 25:100405. doi: 10.1016/j.hermed.2020.100405
51. Goel, G, Makkar, H, and Becker, K. Changes in microbial community structure, methanogenesis and rumen fermentation in response to saponin-rich fractions from different plant materials. J Appl Microbiol. (2008) 105:770–7. doi: 10.1111/j.1365-2672.2008.03818.x
52. Goel, G, Makkar, HP, and Becker, K. Effects of Sesbania sesban and Carduus pycnocephalus leaves and fenugreek (Trigonella foenum-graecum L.) seeds and their extracts on partitioning of nutrients from roughage-and concentrate-based feeds to methane. Anim Feed Sci Technol. (2008) 147:72–89. doi: 10.1016/j.anifeedsci.2007.09.010
53. Bodas, R, López, S, Fernandez, M, García-González, R, Rodríguez, A, Wallace, R, et al. In vitro screening of the potential of numerous plant species as antimethanogenic feed additives for ruminants. Anim Feed Sci Technol. (2008) 145:245–58. doi: 10.1016/j.anifeedsci.2007.04.015
54. He, D-Y, and Dai, S-M. Anti-inflammatory and immunomodulatory effects of Paeonia lactiflora pall., a traditional Chinese herbal medicine. Front Pharmacol. (2011) 2:10.
55. Lim, T. Paeonia lactiflora In: Edible medicinal and non medicinal plants. Dordrecht: Springer (2014). 559–96.
56. Parker, S, May, B, Zhang, C, Zhang, AL, Lu, C, and Xue, CC. A pharmacological review of bioactive constituents of Paeonia lactiflora Pallas and Paeonia veitchii lynch. Phytother Res. (2016) 30:1445–73. doi: 10.1002/ptr.5653
57. Kobayashi, Y. Abatement of methane production from ruminants: trends in the manipulation of rumen fermentation. Asian Australas J Anim Sci. (2010) 23:410–6. doi: 10.5713/ajas.2010.r.01
58. Castillo-González, AR, Burrola-Barraza, ME, Rivas-Martínez, MI, Dominguez-Viveros, J, Ortega-Gutiérrez, JA, and Dominguez-Diaz, D. Relationships between ruminal gram-positive bacteria and methane from lactating dairy cows supplemented with monensin and tallow, alone or in combination. Med Weter. (2016) 72:498–504. doi: 10.21521/mw.5545
59. Shelton, HM, and Brewbaker, JL. Leucaena leucocephala-the most widely used forage tree legume In: RC Gutteridge and HM Shelton, editors. Forage tree legumes in tropical agriculture. Wallingford, UK: CAB International (1994). 15.
60. Zayed, MZ, and Samling, B. Phytochemical constituents of the leaves of Leucaena leucocephala from Malaysia. Int J Pharm Pharm Sci. (2016) 8:174–9. doi: 10.22159/ijpps.2016v8i12.11582
61. Montoya-Flores, MD, Molina-Botero, IC, Arango, J, Romano-Muñoz, JL, Solorio-Sánchez, FJ, Aguilar-Pérez, CF, et al. Effect of dried leaves of Leucaena leucocephala on rumen fermentation, rumen microbial population, and enteric methane production in crossbred heifers. Animals. (2020) 10:300. doi: 10.3390/ani10020300
62. Molina, I, Angarita, E, Mayorga, O, Chará, J, and Barahona-Rosales, R. Effect of Leucaena leucocephala on methane production of Lucerna heifers fed a diet based on Cynodon plectostachyus. Livest Sci. (2016) 185:24–9. doi: 10.1016/j.livsci.2016.01.009
63. Harrison, MT, McSweeney, C, Tomkins, NW, and Eckard, RJ. Improving greenhouse gas emissions intensities of subtropical and tropical beef farming systems using Leucaena leucocephala. Agric Syst. (2015) 136:138–46. doi: 10.1016/j.agsy.2015.03.003
64. Tan, H, Sieo, C, Abdullah, N, Liang, J, Huang, X, and Ho, Y. Effects of condensed tannins from Leucaena on methane production, rumen fermentation and populations of methanogens and protozoa in vitro. Anim Feed Sci Technol. (2011) 169:185–93. doi: 10.1016/j.anifeedsci.2011.07.004
65. Barry, T. The feeding value of forage brassica plants for grazing ruminant livestock. Anim Feed Sci Technol. (2013) 181:15–25. doi: 10.1016/j.anifeedsci.2013.01.012
66. Sun, X. Invited review: Glucosinolates might result in low methane emissions from ruminants fed Brassica forages. Front Vet Sci. (2020) 7:588051. doi: 10.3389/fvets.2020.588051
67. Mageed, AK, Alsaffar, MA, Ghany, MARA, Sukkar, KA, and Ayodele, BV. Advances in synthesis and application of cobalt and nickel-based nanomaterials for catalytic reforming of hydrocarbons and oxygenates to hydrogen-rich syngas. J Ind Eng Chem. (2024) 1–16. doi: 10.1016/j.jiec.2024.09.022
68. Sun, X, Pacheco, D, and Luo, D. Forage brassica: a feed to mitigate enteric methane emissions? Anim Prod Sci. (2016) 56:451–6. doi: 10.1071/AN15516
69. Williams, S, Moate, P, Deighton, M, Hannah, M, Wales, W, and Jacobs, J. Milk production and composition, and methane emissions from dairy cows fed lucerne hay with forage brassica or chicory. Anim Prod Sci. (2016) 56:304–11. doi: 10.1071/AN15528
70. Fu, C, Hernandez, T, Zhou, C, and Wang, Z-Y. Alfalfa (Medicago sativa L.). Methods Mol Biol. (2015) 1223:213–21. doi: 10.1007/978-1-4939-1695-5_17
71. Kozłowska, M, Cieślak, A, Jóźwik, A, El-Sherbiny, M, Stochmal, A, Oleszek, W, et al. The effect of total and individual alfalfa saponins on rumen methane production. J Sci Food Agric. (2020) 100:1922–30. doi: 10.1002/jsfa.10204
72. Du, W, Hou, F, Tsunekawa, A, Kobayashi, N, Ichinohe, T, and Peng, F. Effects of the diet inclusion of common vetch hay versus alfalfa hay on the body weight gain, nitrogen utilization efficiency, energy balance, and enteric methane emissions of crossbred Simmental cattle. Animals. (2019) 9:983. doi: 10.3390/ani9110983
73. Wang, C, Zhang, C, Yan, T, Chang, S, Zhu, W, Wanapat, M, et al. Increasing roughage quality by using alfalfa hay as a substitute for concentrate mitigates CH4 emissions and urinary N and ammonia excretion from dry ewes. J Anim Physiol Anim Nutr. (2020) 104:22–31. doi: 10.1111/jpn.13223
74. Hironaka, R, Mathison, GW, Kerrigan, BK, and Vlach, I. The effect of pelleting of alfalfa hay on methane production and digestibility by steers. Sci Total Environ. (1996) 180:221–7. doi: 10.1016/0048-9697(95)04948-7
75. Lamont, E-J, Zoghlami, A, Hamilton, RS, and Bennett, SJ. Clovers (Trifolium L.) In: N Maxted and SJ Bennett, editors. Plant genetic resources of legumes in the Mediterranean. Dordrecht: Springer (2001). 79–98.
76. Kolodziejczyk-Czepas, J. Trifolium species-derived substances and extracts—biological activity and prospects for medicinal applications. J Ethnopharmacol. (2012) 143:14–23. doi: 10.1016/j.jep.2012.06.048
77. Bica, R, Palarea-Albaladejo, J, Lima, J, Uhrin, D, Miller, GA, Bowen, J, et al. Methane emissions and rumen metabolite concentrations in cattle fed two different silages. Sci Rep. (2022) 12:5441. doi: 10.1038/s41598-022-09108-w
78. Lee, J, Woodward, S, Waghorn, G, and Clark, D. Methane emissions by dairy cows fed increasing proportions of white clover (Trifolium repens) in pasture. Proc NZ Grassland Assoc. (2004) 66:151–5. doi: 10.33584/jnzg.2004.66.2552
79. Parnian-Khajehdizaj, F, Noel, S, Johansen, M, Weisbjerg, M, Hellwing, A, Højberg, O, et al. Methane emission, nutrient digestibility, and rumen microbiota in Holstein heifers fed 14 different grass or clover silages as the sole feed. J Dairy Sci. (2023) 106:4072–91. doi: 10.3168/jds.2022-22638
80. Roldan, MB, Cousins, G, Muetzel, S, Zeller, WE, Fraser, K, Salminen, J-P, et al. Condensed tannins in white clover (Trifolium repens) foliar tissues expressing the transcription factor TaMYB14-1 bind to forage protein and reduce ammonia and methane emissions in vitro. Front Plant Sci. (2022) 12:777354. doi: 10.3389/fpls.2021.777354
81. Arriaga Jordan, C, and González Ronquillo, M. Feeding forage mixtures of ryegrass (Lolium spp.) with clover (Trifolium spp.) supplemented with local feed diets to reduce enteric methane emission efficiency in small-scale dairy systems: a simulated study. Animals (Basel). (2021) 11:946. doi: 10.3390/ani11040946
82. Navarro-Villa, A, O’Brien, M, López, S, Boland, T, and O’kiely, P. In vitro rumen methane output of red clover and perennial ryegrass assayed using the gas production technique (GPT). Anim Feed Sci Technol. (2011) 168:152–64. doi: 10.1016/j.anifeedsci.2011.04.091
83. Muir, S, Kennedy, A, Kearney, G, Hutton, P, Thompson, A, Vercoe, P, et al. Offering subterranean clover can reduce methane emissions compared with perennial ryegrass pastures during late spring and summer in sheep. Anim Prod Sci. (2020) 60:1449–58. doi: 10.1071/AN18624
84. Niyigena, V, Coffey, K, Philipp, D, Savin, M, Zhao, J, Naumann, H, et al. Intake, digestibility, rumen fermentation and nitrogen balance in sheep offered alfalfa silage with different proportions of the tannin-rich legume sericea lespedeza. Anim Feed Sci Technol. (2024) 308:115863. doi: 10.1016/j.anifeedsci.2023.115863
85. Animut, G, Puchala, R, Goetsch, A, Patra, A, Sahlu, T, Varel, V, et al. Methane emission by goats consuming diets with different levels of condensed tannins from lespedeza. Anim Feed Sci Technol. (2008) 144:212–27. doi: 10.1016/j.anifeedsci.2007.10.014
86. Du Toit, CJL, Van Niekerk, WA, Meissner, H, Erasmus, LJ, and Coertze, RJ. Methane emissions from sheep fed Eragrostis curvula hay substituted with Lespedeza cuneata. Anim Prod Sci. (2020) 60:1777–84. doi: 10.1071/AN19257
87. Pech-Cervantes, AA, Terrill, TH, Ogunade, IM, and Estrada-Reyes, ZM. Meta-analysis of the effects of dietary inclusion of sericea lespedeza (Lespedeza cuneata) forage on performance, digestibility, and rumen fermentation of small ruminants. Livest Sci. (2021) 253:104707. doi: 10.1016/j.livsci.2021.104707
88. Liu, H, Puchala, R, LeShure, S, Gipson, TA, Flythe, MD, and Goetsch, AL. Effects of lespedeza condensed tannins alone or with monensin, soybean oil, and coconut oil on feed intake, growth, digestion, ruminal methane emission, and heat energy by yearling alpine doelings. J Anim Sci. (2019) 97:885–99. doi: 10.1093/jas/sky452
89. Roca-Fernández, AI, Dillard, SL, and Soder, KJ. Ruminal fermentation and enteric methane production of legumes containing condensed tannins fed in continuous culture. J Dairy Sci. (2020) 103:7028–38. doi: 10.3168/jds.2019-17627
90. Alvarez-Hess, P, Williams, S, Jacobs, J, Hannah, M, Beauchemin, K, Eckard, R, et al. Effect of dietary fat supplementation on methane emissions from dairy cows fed wheat or corn. J Dairy Sci. (2019) 102:2714–23. doi: 10.3168/jds.2018-14721
91. Patra, AK. The effect of dietary fats on methane emissions, and its other effects on digestibility, rumen fermentation and lactation performance in cattle: a meta-analysis. Livest Sci. (2013) 155:244–54. doi: 10.1016/j.livsci.2013.05.023
92. Haque, MN. Dietary manipulation: a sustainable way to mitigate methane emissions from ruminants. J Anim Sci Technol. (2018) 60:1–10. doi: 10.1186/s40781-018-0175-7
93. Islam, M, and Lee, S-S. Advanced estimation and mitigation strategies: a cumulative approach to enteric methane abatement from ruminants. J Anim Sci Technol. (2019) 61:122–37. doi: 10.5187/jast.2019.61.3.122
94. Piazza, GJ, and Foglia, TA. Rapeseed oil for oleochemical usage. Eur J Lipid Sci Technol. (2001) 103:450–4. doi: 10.1002/1438-9312(200107)103:7<450::AID-EJLT450>3.0.CO;2-D
95. Bayat, AR, Tapio, I, Vilkki, J, Shingfield, K, and Leskinen, H. Plant oil supplements reduce methane emissions and improve milk fatty acid composition in dairy cows fed grass silage-based diets without affecting milk yield. J Dairy Sci. (2018) 101:1136–51. doi: 10.3168/jds.2017-13545
96. Villar, ML, Hegarty, RS, Nolan, JV, Godwin, IR, and McPhee, M. The effect of dietary nitrate and canola oil alone or in combination on fermentation, digesta kinetics and methane emissions from cattle. Anim Feed Sci Technol. (2020) 259:114294. doi: 10.1016/j.anifeedsci.2019.114294
97. Ramin, M, Chagas, JC, Smidt, H, Exposito, RG, and Krizsan, SJ. Enteric and fecal methane emissions from dairy cows fed grass or corn silage diets supplemented with rapeseed oil. Animals. (2021) 11:1322. doi: 10.3390/ani11051322
98. Chagas, JC, Ramin, M, Exposito, RG, Smidt, H, and Krizsan, SJ. Effect of a low-methane diet on performance and microbiome in lactating dairy cows accounting for individual pre-trial methane emissions. Animals. (2021) 11:2597. doi: 10.3390/ani11092597
99. Poulsen, M, Schwab, C, Borg Jensen, B, Engberg, RM, Spang, A, Canibe, N, et al. Methylotrophic methanogenic thermoplasmata implicated in reduced methane emissions from bovine rumen. Nat Commun. (2013) 4:1–9. doi: 10.1038/ncomms2432
100. Beauchemin, K, and McGinn, S. Methane emissions from beef cattle: effects of fumaric acid, essential oil, and canola oil. J Anim Sci. (2006) 84:1489–96. doi: 10.2527/2006.8461489x
101. Flachowsky, G, Langbein, T, Böhme, H, Schneider, A, and Aulrich, K. Effect of false flax expeller combined with short-term vitamin E supplementation in pig feeding on the fatty acid pattern, vitamin E concentration and oxidative stability of various tissues. J Anim Physiol Anim Nutr. (1997) 78:187–95. doi: 10.1111/j.1439-0396.1997.tb00870.x
102. Hurtaud, C, and Peyraud, J-L. Effects of feeding camelina (seeds or meal) on milk fatty acid composition and butter spreadability. J Dairy Sci. (2007) 90:5134–45. doi: 10.3168/jds.2007-0031
103. Ebeid, HM, Hassan, F-U, Li, M, Peng, L, Peng, K, Liang, X, et al. Camelina sativa L. oil mitigates enteric in vitro methane production, modulates ruminal fermentation, and ruminal bacterial diversity in buffaloes [original research]. Front Vet Sci. (2020) 7:1–15. doi: 10.3389/fvets.2020.00550
104. Bayat, A, Kairenius, P, Stefański, T, Leskinen, H, Comtet-Marre, S, Forano, E, et al. Effect of camelina oil or live yeasts (Saccharomyces cerevisiae) on ruminal methane production, rumen fermentation, and milk fatty acid composition in lactating cows fed grass silage diets. J Dairy Sci. (2015) 98:3166–81. doi: 10.3168/jds.2014-7976
105. Lawson, L. The composition and chemistry of garlic cloves and processed garlic In: HP Koch and LD Lawson, editors. Garlic: The science and therapeutic applications of Allium sativum L. and related species. Baltimore, MD: Williams & Wilkins (1996). 37–109.
106. Reuter, HD. Therapeutic effects and applications of garlic and its preparations In: HP Koch and LD Lawson, editors. Garlic: The science and therapeutic applications of Allium sativum L. and related species. Baltimore, MD: Williams & Wilkins (1996)
107. Busquet, M, Calsamiglia, S, Ferret, A, Cardozo, P, and Kamel, C. Effects of cinnamaldehyde and garlic oil on rumen microbial fermentation in a dual flow continuous culture. J Dairy Sci. (2005) 88:2508–16. doi: 10.3168/jds.S0022-0302(05)72928-3
108. Kongmun, P, Wanapat, M, Pakdee, P, and Navanukraw, C. Effect of coconut oil and garlic powder on in vitro fermentation using gas production technique. Livest Sci. (2010) 127:38–44. doi: 10.1016/j.livsci.2009.08.008
109. Sutapa, M, and Analava, M. Health effects of palm oil. J Hum Ecol. (2009) 26:197–203. doi: 10.1080/09709274.2009.11906182
110. Flores-Santiago, E, González-Garduño, R, Vaquera-Huerta, H, Calzada-Marín, JM, Cadena-Villegas, S, Arceo-Castillo, JI, et al. Reduction of enteric methane emissions in heifers fed tropical grass-based rations supplemented with palm oil. Fermentation. (2022) 8:349. doi: 10.3390/fermentation8080349
111. Yilmaz, K, and Kara, K. The effect of vegetable and animal oils added to different forages and concentrates on the in vitro fermentation parameters in ruminants. J Appl Anim Res. (2022) 50:548–59. doi: 10.1080/09712119.2022.2110502
112. Teoh, ES, and Teoh, ES. Secondary metabolites of plants In: Medicinal orchids of Asia. Cham: Springer (2016). 59–73.
113. Kholif, A, Hassan, A, El Ashry, GM, Bakr, M, El-Zaiat, H, Olafadehan, O, et al. Phytogenic feed additives mixture enhances the lactational performance, feed utilization and ruminal fermentation of Friesian cows. Anim Biotechnol. (2021) 32:708–18. doi: 10.1080/10495398.2020.1746322
114. Kholif, AE, and Olafadehan, OA. Essential oils and phytogenic feed additives in ruminant diet: chemistry, ruminal microbiota and fermentation, feed utilization and productive performance. Phytochem Rev. (2021) 20:1087–108. doi: 10.1007/s11101-021-09739-3
115. Wallace, RJ, McEwan, NR, McIntosh, FM, Teferedegne, B, and Newbold, CJ. Natural products as manipulators of rumen fermentation. Asian Australas J Anim Sci. (2002) 15:1458–68. doi: 10.5713/ajas.2002.1458
116. Casamiglia, S, Busquet, M, Cardoza, P, Castillejos, L, and Ferrett, A. Essential oils as modifiers of rumen microbial fermentation: a review. J Dairy Sci. (2017) 90:2580–95. doi: 10.3168/jds.2006-644
117. Reddy, PRK, Elghandour, M, Salem, A, Yasaswini, D, Reddy, PPR, Reddy, AN, et al. Plant secondary metabolites as feed additives in calves for antimicrobial stewardship. Anim Feed Sci Technol. (2020) 264:114469. doi: 10.1016/j.anifeedsci.2020.114469
118. McSweeney, C, Palmer, B, McNeill, D, and Krause, D. Microbial interactions with tannins: nutritional consequences for ruminants. Anim Feed Sci Technol. (2001) 91:83–93. doi: 10.1016/S0377-8401(01)00232-2
119. Jayanegara, A, Goel, G, Makkar, HP, and Becker, K. Divergence between purified hydrolysable and condensed tannin effects on methane emission, rumen fermentation and microbial population in vitro. Anim Feed Sci Technol. (2015) 209:60–8. doi: 10.1016/j.anifeedsci.2015.08.002
120. Patra, AK. An overview of antimicrobial properties of different classes of phytochemicals In: A Patra, editor. Dietary phytochemicals and microbes. Dordrecht: Springer (2012). 1–32.
121. Buccioni, A, Pauselli, M, Viti, C, Minieri, S, Pallara, G, Roscini, V, et al. Milk fatty acid composition, rumen microbial population, and animal performances in response to diets rich in linoleic acid supplemented with chestnut or quebracho tannins in dairy ewes. J Dairy Sci. (2015) 98:1145–56. doi: 10.3168/jds.2014-8651
122. Correa, PS, Mendes, LW, Lemos, LN, Crouzoulon, P, Niderkorn, V, Hoste, H, et al. Tannin supplementation modulates the composition and function of ruminal microbiome in lambs infected with gastrointestinal nematodes. FEMS Microbiol Ecol. (2020) 96:fiaa024. doi: 10.1093/femsec/fiaa024
123. Fagundes, GM, Benetel, G, Welter, KC, Melo, FA, Muir, JP, Carriero, MM, et al. Tannin as a natural rumen modifier to control methanogenesis in beef cattle in tropical systems: friend or foe to biogas energy production? Res Vet Sci. (2020) 132:88–96. doi: 10.1016/j.rvsc.2020.05.010
124. Aboagye, IA, and Beauchemin, KA. Potential of molecular weight and structure of tannins to reduce methane emissions from ruminants: a review. Animals. (2019) 9:856. doi: 10.3390/ani9110856
125. Grainger, C, Clarke, T, Auldist, M, Beauchemin, K, McGinn, S, Waghorn, G, et al. Potential use of Acacia mearnsii condensed tannins to reduce methane emissions and nitrogen excretion from grazing dairy cows. Can J Anim Sci. (2009) 89:241–51. doi: 10.4141/CJAS08110
126. Yang, K, Wei, C, Zhao, G, Xu, Z, and Lin, S. Effects of dietary supplementing tannic acid in the ration of beef cattle on rumen fermentation, methane emission, microbial flora and nutrient digestibility. J Anim Physiol Anim Nutr. (2017) 101:302–10. doi: 10.1111/jpn.12531
127. Volpe, M, Goldfarb, JL, and Fiori, L. Hydrothermal carbonization of Opuntia ficus-indica cladodes: role of process parameters on hydrochar properties. Bioresour Technol. (2018) 247:310–8. doi: 10.1016/j.biortech.2017.09.072
128. Witzig, M, Zeder, M, and Rodehutscord, M. Effect of the ionophore monensin and tannin extracts supplemented to grass silage on populations of ruminal cellulolytics and methanogens in vitro. Anaerobe. (2018) 50:44–54. doi: 10.1016/j.anaerobe.2018.01.012
129. Patra, AK, and Saxena, J. Dietary phytochemicals as rumen modifiers: a review of the effects on microbial populations. Antonie Van Leeuwenhoek. (2009) 96:363–75. doi: 10.1007/s10482-009-9364-1
130. Jayanegara, A, Wina, E, and Takahashi, J. Meta-analysis on methane mitigating properties of saponin-rich sources in the rumen: influence of addition levels and plant sources. Asian Australas J Anim Sci. (2014) 27:1426–35. doi: 10.5713/ajas.2014.14086
131. Ramírez-Restrepo, CA, Tan, C, López-Villalobos, N, Padmanabha, J, Wang, J, and McSweeney, CS. Methane production, fermentation characteristics, and microbial profiles in the rumen of tropical cattle fed tea seed saponin supplementation. Anim Feed Sci Technol. (2016) 216:58–67. doi: 10.1016/j.anifeedsci.2016.03.005
132. Guyader, J, Eugène, M, Doreau, M, Morgavi, D, Gérard, C, and Martin, C. Tea saponin reduced methanogenesis in vitro but increased methane yield in lactating dairy cows. J Dairy Sci. (2017) 100:1845–55. doi: 10.3168/jds.2016-11644
133. Liu, Y, Ma, T, Chen, D, Zhang, N, Si, B, Deng, K, et al. Effects of tea saponin supplementation on nutrient digestibility, methanogenesis, and ruminal microbial flora in dorper crossbred ewe. Animals. (2019) 9:29. doi: 10.3390/ani9010029
134. Tan, C, Ramírez-Restrepo, CA, Shah, AM, Hu, R, Bell, M, Wang, Z, et al. The community structure and microbial linkage of rumen protozoa and methanogens in response to the addition of tea seed saponins in the diet of beef cattle. J Anim Sci Biotechnol. (2020) 11:1–10. doi: 10.1186/s40104-020-00491-w
135. Patra, A, and Yu, Z. Effects of garlic oil, nitrate, saponin and their combinations supplemented to different substrates on in vitro fermentation, ruminal methanogenesis, and abundance and diversity of microbial populations. J Appl Microbiol. (2015) 119:127–38. doi: 10.1111/jam.12819
136. Ye, D, Karnati, S, Wagner, B, Firkins, J, Eastridge, M, and Aldrich, J. Essential oil and monensin affect ruminal fermentation and the protozoal population in continuous culture. J Dairy Sci. (2018) 101:5069–81. doi: 10.3168/jds.2017-13646
137. Lei, Z, Zhang, K, Li, C, Jiao, T, Wu, J, Wei, Y, et al. Ruminal metagenomic analyses of goat data reveals potential functional microbiota by supplementation with essential oil-cobalt complexes. BMC Microbiol. (2019) 19:1–10. doi: 10.1186/s12866-019-1400-3
138. Belanche, A, Newbold, CJ, Morgavi, DP, Bach, A, Zweifel, B, and Yáñez-Ruiz, DR. A meta-analysis describing the effects of the essential oils blend agolin ruminant on performance, rumen fermentation and methane emissions in dairy cows. Animals. (2020) 10:620. doi: 10.3390/ani10040620
139. Zengin, H, and Baysal, AH. Antibacterial and antioxidant activity of essential oil terpenes against pathogenic and spoilage-forming bacteria and cell structure-activity relationships evaluated by SEM microscopy. Molecules. (2014) 19:17773–98. doi: 10.3390/molecules191117773
140. Cobellis, G, Trabalza-Marinucci, M, and Yu, Z. Critical evaluation of essential oils as rumen modifiers in ruminant nutrition: a review. Sci Total Environ. (2016) 545-546:556–68. doi: 10.1016/j.scitotenv.2015.12.103
141. Schären, M, Drong, C, Kiri, K, Riede, S, Gardener, M, Meyer, U, et al. Differential effects of monensin and a blend of essential oils on rumen microbiota composition of transition dairy cows. J Dairy Sci. (2017) 100:2765–83. doi: 10.3168/jds.2016-11994
142. Poudel, P, Froehlich, K, Casper, DP, and St-Pierre, B. Feeding essential oils to neonatal Holstein dairy calves results in increased ruminal Prevotellaceae abundance and propionate concentrations. Microorganisms. (2019) 7:120. doi: 10.3390/microorganisms7050120
143. Evans, JD, and Martin, SA. Effects of thymol on ruminal microorganisms. Curr Microbiol. (2000) 41:336–40. doi: 10.1007/s002840010145
144. Purba, RAP, Paengkoum, S, Yuangklang, C, and Paengkoum, P. Flavonoids and their aromatic derivatives in Piper betle powder promote in vitro methane mitigation in a variety of diets. Ciênc Agrotec. (2020) 44:e012420. doi: 10.1590/1413-7054202044012420
145. Kim, ET, Lee, SJ, Lee, SM, Lee, SS, Lee, ID, Lee, SK, et al. Effects of flavonoid-rich plant extracts on in vitro ruminal methanogenesis, microbial populations and fermentation characteristics. Asian Australas J Anim Sci. (2015) 28:530–7. doi: 10.5713/ajas.14.0692
146. Oskoueian, E, Abdullah, N, and Oskoueian, A. Effects of flavonoids on rumen fermentation activity, methane production, and microbial population. Biomed Res Int. (2013) 2013:1–8. doi: 10.1155/2013/349129
147. Seradj, AR, Abecia, L, Crespo, J, Villalba, D, Fondevila, M, and Balcells, J. The effect of Bioflavex® and its pure flavonoid components on in vitro fermentation parameters and methane production in rumen fluid from steers given high concentrate diets. Anim Feed Sci Technol. (2014) 197:85–91. doi: 10.1016/j.anifeedsci.2014.08.013
148. Morsy, A, Soltan, Y, El-Zaiat, H, Alencar, S, and Abdalla, A. Role of bee propolis extract on diet digestibility, purine derivatives, mitigating methane formation, and blood metabolites in late pregnant ewes. Anim Feed Sci Technol. (2021) 273:114834. doi: 10.1016/j.anifeedsci.2021.114834
149. Adejoro, FA, Hassen, A, and Akanmu, AM. Effect of lipid-encapsulated acacia tannin extract on feed intake, nutrient digestibility and methane emission in sheep. Animals. (2019) 9:863. doi: 10.3390/ani9110863
150. Rira, M, Morgavi, DP, Genestoux, L, Djibiri, S, Sekhri, I, and Doreau, M. Methanogenic potential of tropical feeds rich in hydrolyzable tannins. J Anim Sci. (2019) 97:2700–10. doi: 10.1093/jas/skz199
151. Stewart, EK, Beauchemin, KA, Dai, X, MacAdam, JW, Christensen, RG, and Villalba, JJ. Effect of tannin-containing hays on enteric methane emissions and nitrogen partitioning in beef cattle. J Anim Sci. (2019) 97:3286–99. doi: 10.1093/jas/skz206
152. Wang, C, Wang, S, and Zhou, H. Influences of flavomycin, ropadiar, and saponin on nutrient digestibility, rumen fermentation, and methane emission from sheep. Anim Feed Sci Technol. (2009) 148:157–66. doi: 10.1016/j.anifeedsci.2008.03.008
153. Hart, KJ, Jones, HG, Waddams, KE, Worgan, HJ, Zweifel, B, and Newbold, CJ. An essential oil blend decreases methane emissions and increases milk yield in dairy cows. Open J Anim Sci. (2019) 9:259–67. doi: 10.4236/ojas.2019.93022
Keywords: global warming, microbial fermentation, phytogenic feed additives, rumen microbiome, rumen manipulation, rumen microbe, bioactive material
Citation: Bature I, Xiaohu W and Ding X (2024) The roles of phytogenic feed additives, trees, shrubs, and forages on mitigating ruminant methane emission. Front. Vet. Sci. 11:1475322. doi: 10.3389/fvets.2024.1475322
Edited by:
Sadarman Sadarman, State Islamic University of Sultan Syarif Kasim Riau, IndonesiaReviewed by:
Moyosore Joseph Adegbeye, University of Africa, Toru-Orua, NigeriaDewi Febrina, State Islamic University of Sultan Syarif Kasim Riau, Indonesia
Copyright © 2024 Bature, Xiaohu and Ding. This is an open-access article distributed under the terms of the Creative Commons Attribution License (CC BY). The use, distribution or reproduction in other forums is permitted, provided the original author(s) and the copyright owner(s) are credited and that the original publication in this journal is cited, in accordance with accepted academic practice. No use, distribution or reproduction is permitted which does not comply with these terms.
*Correspondence: Xuezhi Ding, ZGluZ3h1ZXpoaUBjYWFzLmNu