- 1Department of Pharmacy, University of Agriculture, Faisalabad, Pakistan
- 2Department of Veterinary Medicine, College of Agriculture and Veterinary Medicine, Qassim University, Buraidah, Saudi Arabia
Bacterial pathogens of animals impact food production and human health globally. Food animals act as the major host reservoirs for pathogenic bacteria and thus are highly prone to suffer from several endemic infections such as pneumonia, sepsis, mastitis, and diarrhea, imposing a major health and economical loss. Moreover, the consumption of food products of infected animals is the main route by which human beings are exposed to zoonotic bacteria. Thus, there is excessive and undue administration of antibiotics to fight these virulent causative agents of food-borne illness, leading to emergence of resistant strains. Thus, highprevalence antibiotic-resistant resistant food-borne bacterial infections motivated the researchers to discover new alternative therapeutic strategies to eradicate resistant bacterial strains. One of the successful therapeutic approach for the treatment of animal infections, is the application of cell membrane-coated nanoparticles. Cell membranes of several different types of cells including platelets, red blood cells, neutrophils, cancer cells, and bacteria are being wrapped over the nanoparticles to prepare biocompatible nanoformulations. This diversity of cell membrane selection and together with the possibility of combining with an extensive range of nanoparticles, has opened a new opportunistic window for the development of more potentially effective, safe, and immune evading nanoformulations, as compared to conventionally used bare nanoparticle. This article will elaborately discuss the discovery and development of novel bioinspired cell membrane-coated nanoformulations against several pathogenic bacteria of food animals such as Klebsiella pneumoniae, Escherichia coli, Staphylococcus aureus, Salmonella enteritidis, Campylobacter jejuni, Helicobacter pylori, and Group A Streptococcus and Group B Streptococcus.
Introduction
Livestock and aquatic animals are the major sources of income, food, fertilizer, clothing, and building materials for a human being (1, 2). The continuous progression and growth of livestock sector offer significant opportunities for food security gains, poverty decline, better human nutrition, and agricultural development (3, 4). Farm animals constitute 40% of total agriculture output globally (5). These production animals support the livelihoods and the income of almost 1.3 billion people in the world (6). However, disease outbreak in livestock and aquaculture species may significantly reduce this quantum (7, 8). Highly contagious diseases especially the bacterial diseases of animals may have ripple effects on the revenue, food supply, trade, and even human health as these may lead to reduced animal fertility, low yields (milk, eggs, etc.), animal death, and disease transfer in humans (9). There is a need to better understand the emerging animal diseases and their impact on the environment and society, as well as the development of innovative and potential therapeutic and preventive methodologies for their management.
Several therapeutic approaches have been considered to control livestock and fish bacterial diseases including the use of chemicals and antibiotic drugs (10–16). The use of antibiotics has become a vital input in food animal species to achieve the ultimate goal of successful farming with high production (17–19). Yet, the extensive use of antibiotics has raised the prevalence of antimicrobial resistant bacteria (20). In addition, the existence of drug residues in food animals further exaggerates the alarming situation for human beings (21–23). This lethal condition motivates scientists to search and develop new innovative nano-therapeutic agents which may successfully treat the resistant infections and avoid the undue excessive use of inaffective antibiotic drugs (24, 25). Several groups reported the development of nanoparticles which have been comprehensively analyzed in therapeutic context (26, 27). These tiny particles offer advantages of improved delivery of drug to target site (28) as well as controlled release of cargo (29). However, despite of these benefits, food and drug administration (FDA) approved only a few nanoparticles for clinical use. As our body system can easily recognize and rapidly remove the foreign substances via reticuloendothelial system, complex circulatory proteins and immune cells (30). Moreover, another strategy has been considered for surface modification of nanoparticles, that is the coating of Poly(ethylene glycol) (PEG) onto nanoparticles (31). Though pegylation was not a successful solution, as the studies showed that subsequent dosing of PEG-modified nanoparticles induce the rapid clearance by the hepatic phenomenon of accelerated blood clearance (32) via IgM antibodies production and complement system activation against PEG (33). Hence, a protective shield for cargo was needed in the form of an optimal delivery system to avoid rapid degradation and to offer a targeted and controlled drug delivery (34). Hence, the idea of development of biomimetic system start gaining attention to functionalize the nanoparticles with therapeutic potential. The cell membrane coating technology involves use of the cell membrane as drug-carrier, facilitating the controlled and targeted delivery of core nanoparticles irrespective to properties of nanomaterial. The cell membrane coated nano-moieties replicate the shape, surface composition, and movement of normal physiological cells (35).
A recent approach is the use of biomimetic nanoparticles in medical science for rapid diagnosis and biocontrol or treatment of bacterial diseases (36–39). Application of biomimetic nanoparticle can provide a sustainable and natural solution to control resistant bacterial infectionsin the livestock and fish farming industry (40, 41). The bioinspired nanotherapy may present a viable antibiotics alternative (40). The cell membrane coatings over nanoparticles give the appearance of surfaces of naturally occurring cells in the body, that when correctly selected and prepared do not have any risk of immune system activation and attack (38). The biomimetic therapeutic design demonstrates several therapeutic benefits like capability of biointerfacing (42, 43), biocompatibility (44), prolonged duration of circulation (45), immune evasion (46), and protection of the entrapped drug from active targeting and degradation (47).
A number of biomimetic nanoformulations have been designed using wide range of natural cell membranes coated over a variety of nanoparticles to ascertain antibacterial potential (48–52). Studies indicated that there is a significant medical importance of biomimetic and bioinspired nanoparticles to fight against the pathogenic bacteria of food animal host (45). However, our understanding of the design strategies for fabricating potentially effective and economically viable antibacterial nano-moieties remains limited. In this review, we will highlight the recent progress on the antibacterial activity of combo of different nanoparticles with natural cell-derived surfaces. Moreover, we will discuss recent research studies regarding successful development of novel biomimetic and biodegradable antibacterial nanomedicines against major bacterial pathogens responsible for veterinary bacterial diseases.
Concept of cell membrane coating over nanoparticles
The conception of coating several types of nanoparticles with plasma membranes of cells was raised in view of the troubleshooting of the functionalization of synthesized nanoparticles (53). Therefore, scientists developed new nature inspired nano-transporters, so that the nanoparticles may not be degraded by the internal environment of the organism and may easily reach their therapeutic targets (54). The synthesis of biomimetic nanoparticles combines the properties and specifications of cell membranes (external surface) and nanoparticles (core) (55, 56). This new emerging cell membranes coating technology allows the designing of biomimetic nanotransporters with covering surfaces which may directly imitate complicated functionalities of the cells, needed to have precise physiological interaction with other living cells and tissues (57, 58).
The plasma membrane coating concept was first reported in 2011, Hu and his coworkers (59) first employed red blood cell membranes for coating poly (lactic-co-glycolic acid) (PLGA) nanoparticles. The RBCs were first chosen for coating as they have extended circulation period of 120 days so the nanocarriers may stay longer in the blood circulation, the property which is highly needed for the nanocarriers. Since then, for this technology, wide variety of cell types have been considered including mesenchymal stem cells, bacterial cells, platelets, white blood cells, and cancer cells (60, 61).
Cell membrane coating techniques
To produce cell membrane-coated nanoparticles, the specific cell membrane must be coated over the nanoparticle core (62). The most frequently mentioned technique for cell membrane coating is membrane extrusion and ultra-sound to date (63). Membrane extrusion is one of the primary cell membrane-coating techniques. Physical membrane extrusion is performed so that both the cell membranes and nanoparticles may move through membranes of various pore sizes concurrently, leading to the wrapping of the membranes over the nanoparticles (64). This method is beneficial as uniformly sized particles are obtained by using this methodology (65). The strategies employed for extrusion of membranes are membrane emulsification, vesicle extrusion and precipitation extrusion for emulsions, liposomes and nanoparticles/nanofibers respectively. The extrusion technique was first reported for uniform coating of PLGA nanoparticle core with red blood cell membrane (59). After that successful coating several research groups have stated this method for coating different types of cell membranes with varying pore size (66–68); however, mechanical forces may affect the structure of the membrane in this protocol (59).
Consistent and stable cell membrane-coated nanoparticles (CMCNPs) can be obtained by ultrasound technique. This technique also encourages the membrane and nanoparticles to naturally form a core-shell structure with a little loss of substantial material; however it may destroy the nanoparticles (69). Several researchers have reported to use this technique for assembling different types of cell membranes such as assembling or coating of membranes of platelet (54), stem cell (70), neutrophil (71) onto PLGA nanoparticles; Moreover, coating of cardiac stem cell membrane over microparticles of PLGA (72). Another example is assembling of a hybrid of platelet and RBC membranes over gold nanowires (73).
In another technique, nanoparticles and cell membrane vesicles are first mixed in the microfluidic system with the S and Y-shaped channels. Then, instantaneous pores are created on the vesicles by using electrical pulses at the exit of the channels and thus allowing nanoparticles to penetrate the vesicles, this technique is known as electroporation (74). An example of application of this technique is successful coating of RBC membranes onto ferric oxide (Fe3O4) nanoparticles resulting in uniform fabrication of RBC-Fe3O4 nanoparticles of good colloidal stability (75).
Another technique involves co-incubation of nanoparticles and live cells followed by the addition of serum-free media for secretion and production of exosomes (76). While wrapping the cell membrane over nanoparticles, it is vital to keep in mind that the coating process makes stable homogenous nanoparticles that do not interfere with either the function of the nanoparticles or the cellular membrane (77).
Concerning the membrane coating methods, the easiest strategy for coating among the above mentioned methods, is based on subsequent extrusion technique. Moreover, ultrasounds produce sonication forces to coat membrane surfaces over nanoparticles with better efficacy (69). Microfluidic technology is highly recommended for coating magnetic nanoparticles with good control as fine-tuning may be obtained via the flow speed (75).
Bioinspired nanotherapies against principal food borne pathogens
Biomimetic manufacturing of nanoparticles is a successful and rapidly developing field of nanotechnology (58). Depicting the concept of specific attachment sites and translocation protocols by pathogenic bacteria and host mammalian cells, the biomimetic nanoparticles showed several pharmacological functions like improved accumulation at site of infection/inflammation (78), efficient drug delivery to the target (79, 80), prolonged duration of circulation (81), and eliminate off-target adverse effects in the healthy host tissues (82). Thus, resulting in development of more effective and targeted nanoplatforms. All over the world, researchers have been working on bioengineered coated nanoparticles with special focus on antibacterial therapy (83, 84). The effectiveness, long-term safety, biopharmaceutical, and pharmacokinetic profiles of newly developed biomimetic nanotherapies are being confirmed by comprehensive anti-bacterial studies (85) (Table 1, Figure 1).
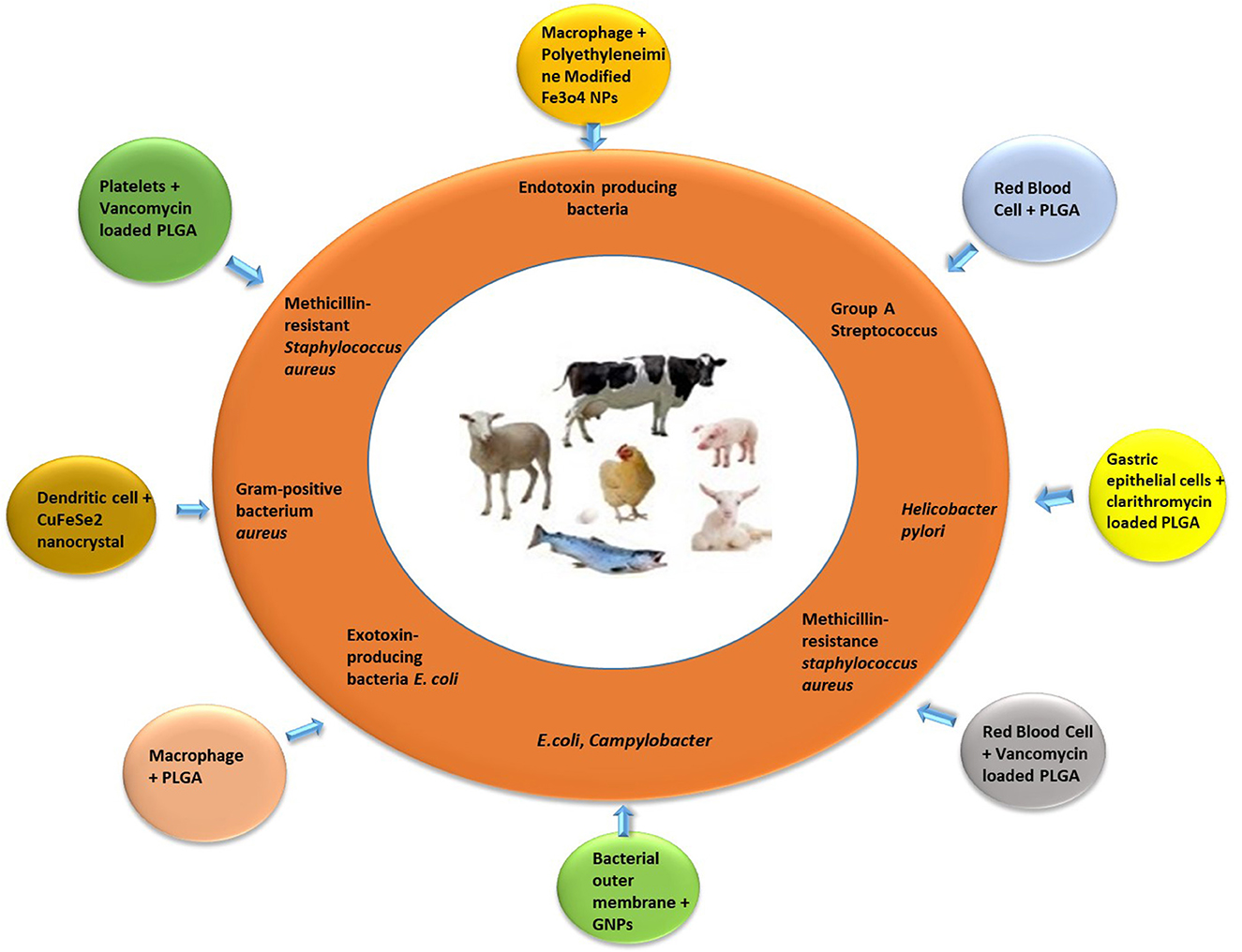
Figure 1. Bioinspired and biomimetic nanotherapies against specific bacterial pathogens of food animals.
Major food animal pathogens treated through cell membrane-coated nanoparticles
Klebsiella pneumoniae
Klebsiella pneumoniae is commonly considered a responsible pathogen for nosocomial and community-acquired infections, including liver abscesses, infections of urinary tract and lower respiratory tract (102). The causative bacteria may be found in several niches like soil, meat, feaces, skin and intestine of livestock, and sea food animals (103). Antimicrobial-resistant species of K. pneumoniae have been found in various food products, including raw meat (bovine, chicken, and pork), sea food, vegetables, and ready-to-eat meals (104–108). World Health Organization (WHO) has listed cephalosporins and carbapenems resistant K. pneumoniae strain, as one of the top threats to public health globally. Thus emphasizing the urgent need for the discovery and development of new therapeutic agents (109, 110). Sepsis is a systemic inflammatory response syndrome caused by infection, with high incidence and mortality. Therefore, it is necessary to carry out an effective anti-infection treatment. In a recent research work, the scientists designed and developed a new biomimetic nanomedicine and named it γ3-RBCNPs. This bioinspired antibacterial agent involves the loading of ciprofloxacin into PLGA nanoparticles and then wrapping with RBCM. These functionalized nanoformulations specifically target intercellular adhesion molecule-1 (ICAM-1) via γ3 peptide on their surface and ultimately eradicate the K. pneumoniae with the help of loaded ciprofloxacin. The RBC membrane coating over the nanoparticles offers the properties of prolonged circulation time and immune escape (45, 46). Further investigations indicated that γ3-RBCNPs have good antibacterial efficacy, good bio-safety, and long half-life in mice mode (51).
Salmonella enteritidis
It is a foodborne pathogen, possess several zoonotic serotypes as well as a wide range of host organisms including poultry, ovine, bovine, and humans (111). It is the most common type of infectious agent, causing animal and human Salmonellosis (112, 113). S. enteritidis is responsible for a reduction in egg production in poultry, leading economic losses to poultry industry (114, 115). Notably, S. enteritidis-infected meat and contaminated eggs are the primary cause of human Salmonellosis, so the control and treatment of Salmonellosis in the food animals would definitely lead to decline in cases of human Salmonellosis (116, 117). Moreover, the multi-drug resistant (MDR) strains of salmonella are emerging and their prevalence is also increasing day by day (118–120). In Egypt, the percentage of prevalence of multi drug resistant S. enterica was found to be 69.8% in the marketed meat samples in 2010, and 82.4 to 100% in 2020 (121–123).
Several therapeutic and preventive strategies have been considered to control Salmonella shedding in poultry and other livestock animals (124). It is expected that novel non-antibiotic therapeutic agents may reduce burden of bacterial colonization, environmental contamination and public health risk (125). Currently, Salmonella vaccines have been developed by coating of bacterial cell membranes over the chitosan nanoparticles. Scientists utilized S. enterica outer membrane proteins, flagellin proteins, and chitosan nanoparticles for the development of nano-vaccine against Salmonella (CNP-vaccine). Two experiments were performed, Experiment I was done to evaluate the optimal dose of CNP-vaccine to get a protective response against bacterial infection of S. Enteritidis. Whereas the Experiment II was performed to investigate the cross protection of nanoformulations against bacterial infection of S. Heidelberg. The results indicated that CNP-Salmonella vaccine was capable to raise the level of IgA and IgG in S. Enteritidis or S. Heidelberg infected broilers, thus showed cross-protection against both serovars of S. enterica (49).
Methicillin-resistant Staphylococcus aureus (MRSA)
This is a highly dangerous drug-resistant pathogen which is responsible for a wide range of infections such as endocarditis, sepsis and bacteremia leading to high rate of mortality (126–128). In food animals MRSA was first detected in the early 1970s in Belgium and induced bovine mastitis (129). After that, several cases have been reported on MRSA infection and colonization in other food-chain animals such as poultry, cattle, sheep, goat and pigs, intimating MRSA as an imperative veterinary and zoonotic pathogen (130–132).
Molecular typing of S. aureus indicated that certain animal lineages are specific to host while some are able to colonize or infect a wide variety of animals (133). The most notable case is ST398, which was primarily detected among pigs, and afterwards was found in various food-chain animals as well as in humans. The isolates stated to date and carrying mecC mainly belonged to lineages in cattle. However, mec C has also been indicated in other food animals such as sheep and rabbits as well as among various companion animals (cats, guinea pigs, horses, and dogs) (134, 135).
The pathogenesis of S. aureus pathogenesis is fueled by lethal secretion of toxins including α-toxin, which damages the membrane by forming pores and targets epithelium and endothelium of leukocytes and platelets (136). Most antibiotics are ineffective in treating MRSA infections as MRSA has a good survival strategy and self-preservation (137, 138). Nano-drug delivery systems have emerged as a new method to overcome this barrier (135). Recently, a study was conducted to synthesize an innovative nano-drug delivery system against MRSA infection. The researchers developed silver metal-organic frame structure (Ag-MOF) of methylimidazole and silver nitrate. Then the framework was loaded with Vancomycin (Vanc) to form Ag-MOF-Vanc complex and finally coated with platelet vesicles to get platelet membrane covered nanoparticles PLT@Ag-MOF-Vanc. The prepared nanoformulations killed MRSA through various strategies, such as interfering with bacterial metabolism, inhibiting production of reactive oxygen species, destructing the structure of cell membrane, and inhibiting formation of biofilm. The coating of the platelet membrane buttoned up to the surface of the pathogenic bacteria (MRSA) and the sites of MRSA infection. The results indicated good therapeutic effect in the mouse MRSA pneumonia model, and showed no toxic effect (139).
A biomimetic nanodecoy strategy was developed to capture and neutralize S. aureus toxins. The Platelets membranes were isolated and coated over PLGA nanoparticles and the resulting formulation of nanospongee inhibit the platelet and macrophage damage, induced by toxins of S. aureus, thereby supporting activation of platelets, nitric oxide production, macrophage oxidative burst, and bactericidal activity. Moreover, nanosponge also helped in release of neutrophils which are trapped in the extracellular fluid by a pathogenic organism. Thus, the prepared platelet membrane coated nanoparticles (PNPs) provided the therapeutic benefits of cytoprotection and enhanced host resistance to S. aureus infection (50).
The cell membrane-coated nanoparticles have great potential for treatment of bacterial infections. However, infection of bones involved inflammation and loss of bone mass, so the treatment must have anti-inflammatory and osteoconductive agents (39). The membrane coating through ultrasonication and extrusion strategies reduced the functionality of plasma membrane, so for coating the nanoparticles, another protocol was introduced in 2021, which involved electroporation procedure to help in retaining the functionality of cell membranes. A composite of nanoparticles with bactericidal TiO2 and osteoconductive Ca3(PO4)2 properties was assembled and coated by macrophage membrane. The resulting macrophage membrane coated nanoparticles possess better functional membranous coating and showed significant bactericidal and anti-inflammatory potential against methicillin resistant S. aureus infection (39).
Escherichia coli
Escherichia coli (E. coli) is both commensal and pathogenic type of bacteria existing in humans and animals (140). Shiga-toxin producing E. coli (STEC), known as E. coli 0157 is responsible for causing resistant infectious disease (141–144). The symptoms of disease include fever, nausea, cramps, vomiting, bloody or watery diarrhea and sometimes kidney failure leading to death. Cows especially calves, sheep, goats, pig and deer may transfer the infection to humans. It is a food-borne pathogen and people got infection by either having contaminated food, including unpasteurized milk and undercooked beef or by having direct contact with E. coli O157 from stool of calves and cattle (145, 146). Animals carry E. coli O157 in the intestine and then shed in stool but still appear healthy and clean. The germs can quickly contaminate the animals' skin, fur, feathers, and the areas where they live and roam. Animals can lead healthy life but may potentially spread E. coli O157 infection to other animals and humans. E. coli may cause bovine mastitis in cattle (147, 148) and infection can be graded from being a subclinical illness of bovine mammary gland to a serious systemic infection. The age and lactation stage of the cows are the major factors to be considered for determining the severity of coliform mastitis.
The currently available antimicrobial agents are not effective enough for treatment of E. coli mastitis. However, cephalosporins and fluoroquinolones have shown partial therapeutic benefits for treatment of E. coli mastitis (149). The use of both drugs is restricted to a limit in food animals with special instructions (150). In E. coli mastitis, a potential therapeutic agent is needed, scientists are working to design and develop biomimetic nanoformulations for the successful treatment of the E. coli instigated disease. The disguised nanoparticles were prepared by using macrophage membranes to specifically neutralize and deactivate lipopolysaccharides secreted by E. coli, pathogenic bacteria. The in vivo experimentation in endotoxemia-induced mice model demonstrated that the coated nanoparticles reduced immune response and the inflammatory reaction. In addition, the nanoformulation improved the survival rate. These macrophage membranes coated nanoparticles are broadly considered for the treatment of LPS associated infectious diseases (95).
Helicobacter pylori
Helicobacter pylori is a spiral shaped gram negative bacterium which mainly colonize in the gastric environment (151). This highly virulent bacteria specie is responsible to cause ulcer, lymphoma and gastric cancer (152). The transmission route of the bacteria is still not known. However, studies were conducted in the past to evaluate the probability of zoonotic transmission. In a study, scientists isolated H. pylori from pigs, sheep, cow, and camel milk (153, 154) and the results showed that H. pylori may opt these animals as reservoirs. In another study, the prevalence and virulence of H. pylori was determined in the stomach of sheep, cow and goat. The zoonotic transmission of H. pylori was also examined. It was demonstrated in the results that cows, sheep, and human beings samples were found H. pylori positive; however the bacterium was not detected in goat samples. Moreover, the virulent H. pylori genotype (vacA s1a/m1a) linked to epithelial cell injury, was the predominant H. pylori genotype in cow, and goat population (155). H. pylori infection is the major cause of gastric and peptic ulcer, gastritis and gastric cancer, thus it is highly essential to treat the infection of food animals and humans with potential therapeutic agents. Currently, clarithromycin, amoxicillin and metronidazole are recommended for the treatment. However, mutations in H. pylori genes have led to the treatment failure (156).
Recently, a novel targeted nanotherapeutic agent was developed, inspired by the idea of natural interaction and adhesion of host and the bacteria. The plasma membranes of gastric epithelial cells are coated over chlarithromycin-loaded polymeric nanoparticles, the resulting biomimetic nanoparticles have the same surface properties as the epithelial cells and thus adhere to H. pylori bacteria. Thus, the nanoformulations provided the targeted drug delivery platform against colonized pathogenic bacteria. Thus, the bioinspired nanotherapeutic approach strategy reported here represented a novel drug delivery system to treat infectious disease of H. pylori (92).
Another versatile targeted therapeutic agent was synthesized by Zhang and his coworkers (157). The bioinspired moieties interfere with adhesion of bacteria to the host and thus evade the decorum of bacterial growth inhibition or killing, which may reduce the resistance development. The polymeric cores were wrapped with bacterial outer membranes resulting in synthesis of H-pylori-mimicking nanoparticles which compete with source bacteria for binding to gastric epithelial cells. Treatment of H-pylori-mimicking nanoparticles with gastric epithelial cells enhance the target adhesion and the efficacy is dependent on coated nanoparticles concentration and dosing (157).
Campylobacter jejuni
Campylobacter jejuni is highly responsible for foodborne zoonosis and is associated with handling and consumption of the poultry meat. The bacteria colonizes in the intestine of chicken and thus slaughtering process resulted in fecal contamination of the carcasses (158). One strategy to control C. jejuni infection is to halt the bacterial colonization of broilers (159).
The extent of contamination is directly linked to the number of bacteria in the cecal content of broiler chicken and the number of bacteria on the carcasses and cut meat pieces (160). Therefore, Campylobacter load is reduced at poultry production level before slaughtering (161). Several antibiotic drugs have been considered for the treatment and control of the infection but still the infection prevails due to the existence of resistant strains of bacteria.
Several therapeutic and preventive strategies have been developed against C. jejuni colonization in poultry (159, 160, 162–164). In a study, biodegradable and biocompatible poly (lactide-co-glycolide) (PLGA) compound was used to prepare nanoparticle and then the synthesized nanoparticles were wrapped with outer membrane proteins of C. jejuni. The prepared coated nanoparticles were then administered through oral/subcutaneous routes. The results were interpreted based on intestinal colonization of C. jejuni in chicken. The C. jejuni colonization in cecal and cloacal contents was reduced post administration of prepared nano vaccine. It was concluded that nanoparticles encapsulated with outer membrane proteins of C. jejuni may be considered as a potential candidate vaccine for controlling the colonization of C. jejuni in chickens (88).
Considering the dynamic interaction of pathogen with host as well as understanding the versatile functionality of bacterial outer membrane proteins for intestinal adherence and invasion, bacterial outer-membrane vesicles have become a potential candidate for vaccine targeting against C. jejuni (165). The chitosan-nanoparticles were coated with outer-membrane vesicles and then evaluated in mouse model for induction of specific immune response against C. jejuni. The results indicated that intragastric delivery of chitosan-coated outer-membrane vesicles impart significant immune protection (166).
Further, immunization with the outer membrane vesicles resulted in potent cellular responses with an increased CD4+ and CD8+ T cell population. Moreover, significant upregulation of IFN-γ and IL-6 gene expression suggests that mucosal delivery of outer membrane vesicles promotes a Th1/Th2 mixed-type immune response. Together, as an acellular and nonreplicating canonical end product of bacterial secretion, mucosal delivery of outer membrane vesicles may represent a promising platform for developing an effective vaccine against C. jejuni (166).
Group A streptococcus and Group B streptococcus
The strains of Streptococcus cause mild to severe bacterial disease in animals as well as in human beings. The pathogenic organism is not host-specific as some strains which are associated with animals can be frequently found in humans (167, 168). Streptococcus infection may rapidly develop into a lethal disease despite administration of commercially available drugs (antibiotics and palliative medication). The streptococcus bacteria possess detrimental virulence factors, such as Group A streptococcus pore-forming streptolysin O and Group B streptococcus pore-forming toxin β-hemolysin/cytolysin. The toxins adversely effects the host tissues including failing of blood brain barrier, injury of lung cells, and apoptosis of immune cells (90). Many zoonotic cases of streptococci are sporadic, but some fish-linked strain of S. agalactiae are involved in outbreaks (169, 170). Pig associated strain of S. suis, has emerged as a major environmental pathogen causing deadly shock-like syndrome, septicemia, streptoccoccal meningitis, and other human diseases after contact with infected animals or derived food products, especially in the Asian countries (171–174). Moreover, in case of cattle, streptococci are declared to be the virulent bacteria causing bovine mastitis throughout the world (175, 176).
Several classes of antibiotic drugs are considered for the treatment of infected animals. First-line treatment for streptococci infection is penicillins either alone or in combo with tetracyclines, aminoglycosides, lincosamides, macrolides and fluoroquinolones (177). However, antibiotic-resistant phenotypes of streptococci have been reported in infected animals all over the world (178). New treatment strategies are being considered against streptococcal infection (179, 180), including a titratable detoxification therapy by a wraping of polymeric cores with donor red blood cell membranes to create biomimetic “nanosponges”. The polymeric cores of biomimetic nanosponge retain the same repertoire of receptors over the cell membrane and proposed a non-specific toxin decoy stratagem to neutralize and sequester several bacterial toxins and pro-inflammatory chemokines of the host. The prepared nanosponge was evaluated to neutralize and sequester streptolysin O toxin of Group A Streptococcus (GAS) and inhibit the functioning of later virulent bacteria. Moreover, this therapeutic intervention inhibited toxin-induced apoptosis of macrophage and enhanced neutrophil trapping process leading to enhanced killing of pathogenic bacteria by phagocytic cells. In vivo studies of biomimetic nanosponge showed that the local administration of the prepared nanosponge reduced lesion size and bacterial colony-forming unit in the GAS-infected mouse model. Thus, the application of a toxin decoy assisted in the inactivation of secreted toxin and presented a novel approach to directly target virulence in severe GAS infections (89).
In the recent research work, red blood cell membrane vesicles were isolated and coated over polymeric nanoparticles to formulate biomimetic nanosponge. The prepared nanosponges proved to be successfully alleviate a sequence of toxic events like the hemolytic activity of living Goup B streptococcus bacteria, injury of lung epithelial and production of streptococcus -induced macrophage IL-1β. The results also indicated that red blood cell membrane-coated nanoparticles might be considered a first-in-class treatment option for streptococcal bacterial infection (90).
Conclusion
Traditional anti-infection therapeutic strategies mainly involved administration of antibiotics. However, emergence of drug-resistant bacteria and increasing endemic of lethal bacterial infections raised the importance of development of novel therapeutic agents for infectious diseases. Recent advances in nanotechnology, resulted in synthesis of a variety of nanoscale cell-membrane bagged nanoparticles. Several efforts have been directed toward nanoparticle functionalization by successful protection during the interaction of bioinspired nanoparticles with pathogens or exo/endotoxin. Food-borne zoonotic pathogens have been targeted by biomimetic nanoparticles and showed potential antibacterial activity.
Author contributions
SA contributed to the design/plan of the work. KA and SA collected and interpreted the data and drafted, revised, and approved the final version of the article to be published.
Acknowledgments
The researchers would like to thank the Deanship of Scientific Research, Qassim University, Saudi Arabia for funding the publication of this project.
Conflict of interest
The authors declare that the research was conducted in the absence of any commercial or financial relationships that could be construed as a potential conflict of interest.
Publisher's note
All claims expressed in this article are solely those of the authors and do not necessarily represent those of their affiliated organizations, or those of the publisher, the editors and the reviewers. Any product that may be evaluated in this article, or claim that may be made by its manufacturer, is not guaranteed or endorsed by the publisher.
References
1. Alao BO, Falowo AB, Chulayo A, Muchenje V. The potential of animal by-products in food systems: production, prospects and challenges. Sustain. (2017) 9:1089–99. doi: 10.3390/su9071089
2. Hampton JO, Hyndman TH, Allen BL, Fischer B. Animal harms and food production: informing ethical choices. J Anim. (2021) 11:1225–39. doi: 10.3390/ani11051225
3. Enahoro D, Lannerstad M, Pfeifer C, Dominguez-Salas P. Contributions of livestock-derived foods to nutrient supply under changing demand in low- and middle-income countries. Glob Food Sec. (2018) 19:1–10. doi: 10.1016/j.gfs.2018.08.002
4. Leroy F, Abraini F, Beal T, Dominguez-Salas P, Gregorini P, Manzano P, et al. Animal board invited review: animal source foods in healthy, sustainable, and ethical diets—an argument against drastic limitation of livestock in the food system. animal. (2022) 16:100457–86. doi: 10.1016/j.animal.2022.100457
5. Lawal-Adebowale OA. Farm animals' health behaviours: an essential communicative signal for farmers' veterinary care and sustainable production. Livest Heal Farming. (2020) 1–25. doi: 10.5772/intechopen.89738
6. Chineke CA. Alleviating poverty through ruminant production : the nigerian gains. World J Agric Sci. (2022) 21:13–25. doi: 10.4314/gjass.v21i1.3
7. Chen R, Gardiner E, Quigley A. Foot mouth disease outbreak in Indonesia: summary and implications. Glob Biosecurity. (2022) 4:1–21. doi: 10.31646/gbio.175
8. Sanches-Fernandes GM, Correia I, Costa, R. Vibriosis outbreaks in aquaculture: addressing environmental and public health concerns and preventive therapies using gilthead seabream farming as a model system. Front Microbiol. (2022) 13:1–25. doi: 10.3389/fmicb.2022.904815
9. McElwain TF, Thumbi SM. Animal pathogens and their impact on animal health, the economy, food security, food safety and public health. Rev Sci Tech. (2019) 36:423–33. doi: 10.20506/rst.36.2.2663
10. Gulaydin O, Gurturk K, Ekin IH, Ozturk C. Determination of MIC values of various antimicrobial agents and presence of resistance genes in pasteurella multocida strains isolated from bovine. Kafkas Üniversitesi Veteriner Fakültesi Dergisi. (2021) 27:217–24.
11. Okonkwo IF, Achilike KM. Comparative assessment of antimicrobial activities of Allium cepa (onions) extract. Agrobiol Rec. (2022) 9:73–9. doi: 10.47278/journal.abr/2022.012
12. Rafay M, Usman Ghaffar M, Abid M, Malik Z, Madnee M. Phytochemicals analysis and antimicrobial activities of echinops echinatus from cholistan desert, Pakistan. Agrobiol Rec. (2021) 5:21–7. doi: 10.47278/journal.abr/2021.001
13. Landers TF, Cohen B, Wittum TE, Larson EL. A review of antibiotic use in food animals: perspective, policy, and potential. Public Health Rep. (2012) 127:4–26. doi: 10.1177/003335491212700103
14. Okocha RC, Olatoye IO, Adedeji OB. Food safety impacts of antimicrobial use and their residues in aquaculture. Public Health Rev. (2018) 39:1–22. doi: 10.1186/s40985-018-0099-2
15. Sharma C, Rokana N, Chandra M, Singh BP, Gulhane RD, Gill JPS, et al. Antimicrobial resistance: its surveillance, impact, and alternative management strategies in dairy animals. Front Vet Sci. (2018) 4:237–52. doi: 10.3389/fvets.2017.00237
16. Ferri G, Lauteri C, Vergara A. Antibiotic resistance in the finfish aquaculture industry: a review. Antibiot. (2022) 11:1574–82. doi: 10.3390/antibiotics11111574
17. Myers J, Hennessey M, Arnold JC, McCubbin KD, Lembo T, Mateus A, et al. Crossover-use of human antibiotics in livestock in agricultural communities: a qualitative cross-country comparison between Uganda, Tanzania and India. Antibiotics. (2022) 11:1–32. doi: 10.3390/antibiotics11101342
18. Wallinga D, Smit LAM, Davis MF, Casey JA, Nachman KE. A review of the effectiveness of current us policies on antimicrobial use in meat and poultry production. Curr Environ Heal Reports. (2022) 9:339–54. doi: 10.1007/s40572-022-00351-x
19. Ur Rahman S, Mohsin M. The under reported issue of antibiotic-resistance in food-producing animals in Pakistan. Pak Vet J. (2019) 39:323–8. doi: 10.29261/pakvetj/2019.037
20. Xu C, Kong L, Gao H, Cheng X, Wang X. A review of current bacterial resistance to antibiotics in food animals. Front Microbiol. (2022) 13:1458–75. doi: 10.3389/fmicb.2022.822689
21. Ahmed MH, Riad EM, Diab OM, Mansour HA, El-Mossalami M. Antibiotic residues in locally marketed fresh and frozen livers in Cairo and Giza, Egypt. Int J Vet Sci. (2022) 11:37–42. doi: 10.47278/journal.ijvs/2021.073
22. Desmarchelier A, Bessaire T, Savoy MC, Tarres A, Mujahid C, Beck A, et al. Screening of 154 veterinary drug residues in foods of animal origin using LC–MS/MS: first action. AOAC Int. (2021) 104:650–681. doi: 10.1093/jaoacint/qsaa168
23. Piñeiro SA, Cerniglia CE. Antimicrobial drug residues in animal-derived foods: Potential impact on the human intestinal microbiome. J Vet Pharmacol Ther. (2021) 44:215–22. doi: 10.1111/jvp.12892
24. Jiang L, Lee HW, Loo SCJ. Therapeutic lipid-coated hybrid nanoparticles against bacterial infections. RSC Adv. (2020) 10:8497–524. doi: 10.1039/C9RA10921H
25. Kaur K, Reddy S, Barathe P, Shriram V, Anand U, Proćków J, et al. Combating drug-resistant bacteria using photothermally active nanomaterials: a perspective review. Front Microbiol. (2021) 12:3405–28. doi: 10.3389/fmicb.2021.747019
26. Doane T, Burda C. Nanoparticle mediated non-covalent drug delivery. Adv Drug Deliv Rev. (2013) 65:607–21. doi: 10.1016/j.addr.2012.05.012
27. Fang RH, Zhang L. Nanoparticle-based modulation of the immune system. Annu Rev Chem Biomol Eng. (2016) 7:305–26. doi: 10.1146/annurev-chembioeng-080615-034446
28. Peng JQ, Fumoto S, Suga T, Miyamoto H, Kuroda N, Kawakami S, et al. Targeted co-delivery of protein and drug to a tumor in vivo by sophisticated RGD-modified lipid-calcium carbonate nanoparticles. J Control Release. (2019) 302:42–53. doi: 10.1016/j.jconrel.2019.03.021
29. Peralta ME, Jadhav SA, Magnacca G, Scalarone D, Mártire DO, Parolo ME, et al. Synthesis and in vitro testing of thermoresponsive polymer-grafted core-shell magnetic mesoporous silica nanoparticles for efficient controlled and targeted drug delivery. J Colloid Interface Sci. (2019) 544:198–205. doi: 10.1016/j.jcis.2019.02.086
30. Parodi A, Molinaro R, Sushnitha M, Evangelopoulos M, Martinez JO, Arrighetti N, et al. Bio-inspired engineering of cell- and virus-like nanoparticles for drug delivery. Biomaterials. (2017) 147:155–68. doi: 10.1016/j.biomaterials.2017.09.020
31. Wang JL, Du XJ, Yang JX, Shen S, Li HJ, Luo YL, et al. The effect of surface poly(ethylene glycol) length on in vivo drug delivery behaviors of polymeric nanoparticles. Biomaterials. (2018) 182:104–13. doi: 10.1016/j.biomaterials.2018.08.022
32. Shiraishi K, Hamano M, Ma H, Kawano K, Maitani Y, Aoshi T, et al. Hydrophobic blocks of PEG-conjugates play a significant role in the accelerated blood clearance (ABC) phenomenon. J Control Release. (2013) 165:183–90. doi: 10.1016/j.jconrel.2012.11.016
33. Wan X, Zhang J, Yu W, Shen L, Ji S, Hu T. Effect of protein immunogenicity and PEG size and branching on the anti-PEG immune response to PEGylated proteins. Process Biochem. (2017) 52:183–91. doi: 10.1016/j.procbio.2016.09.029
34. Thanuja MY, Anupama C, Ranganath SH. Bioengineered cellular and cell membrane-derived vehicles for actively targeted drug delivery: So near and yet so far. Adv Drug Deliv Rev. (2018) 132:57–80. doi: 10.1016/j.addr.2018.06.012
35. Meyer RA, Sunshine JC, Green JJ. Biomimetic particles as therapeutics. Trends Biotechnol. (2015) 33:514–39. doi: 10.1016/j.tibtech.2015.07.001
36. Rao L, Tian R, Chen X. Cell-membrane-mimicking nanodecoys against infectious diseases. ACS Nano. (2020) 14:2569–74. doi: 10.1021/acsnano.0c01665
37. Altaf S, Muhammad F, Aslam B, Faisal MN. Cell membrane enveloped polymeric nanosponge for detoxification of chlorpyrifos poison: in vitro and in vivo studies. Hum Exp Toxicol. (2021) 40:1286–95. doi: 10.1177/0960327121993207
38. Ma J, Jiang L, Liu G. Cell membrane-coated nanoparticles for the treatment of bacterial infection. Wiley Interdiscip Rev Nanomedicine Nanobiotechnology. (2022) 14:e1825. doi: 10.1002/wnan.1825
39. Shi M, Shen K, Yang B, Zhang P, Lv K, Qi H, et al. An electroporation strategy to synthesize the membrane-coated nanoparticles for enhanced anti-inflammation therapy in bone infection. Theranostics. (2021) 11:2349–63. doi: 10.7150/thno.48407
40. Chen B, Li F, Zhu XK, Xie W, Hu X, Zan MH, et al. Highly biocompatible and recyclable biomimetic nanoparticles for antibiotic-resistant bacteria infection. Biomater Sci. (2021) 9:826–34. doi: 10.1039/D0BM01397H
41. Xu L, Zhu Z, Sun DW. Bioinspired Nanomodification Strategies: Moving from Chemical-Based Agrosystems to Sustainable Agriculture. ACS Nano. (2021) 15:12655–86. doi: 10.1021/acsnano.1c03948
42. Li P, Kim S, Tian B. Nanoenabled trainable systems: from biointerfaces to biomimetics. ACS Nano. (2022) 16:19651–64. doi: 10.1021/acsnano.2c08042
43. Shih CP, Tang X, Kuo CW, Chueh DY, Chen P. Design principles of bioinspired interfaces for biomedical applications in therapeutics and imaging. Front Chem. (2022) 10:1215–42. doi: 10.3389/fchem.2022.990171
44. Bu Y, Zhang X, Zhu A, Li L, Xie X, Wang S. Inside-out-oriented cell membrane biomimetic magnetic nanoparticles for high-performance drug lead discovery. Anal Chem. (2021) 93:7898–907. doi: 10.1021/acs.analchem.1c00567
45. Yang G, Chen S, Zhang J. Bioinspired biomimetic nanotherapies for the treatment of infectious diseases. Front Pharmacol. (2019) 10:751–70. doi: 10.3389/fphar.2019.00751
46. Jan N, Madni A, Khan S, Shah H, Akram F, Khan A, et al. Biomimetic cell membrane-coated poly(lactic-co-glycolic acid) nanoparticles for biomedical applications. Bioeng Transl Med. (2022) 10441:1–36. doi: 10.1002/btm2.10441
47. Guido C, Maiorano G, Cortese B, D'amone S, Palamà IE. Biomimetic nanocarriers for cancer target therapy. Bioeng. (2020) 7:111–21. doi: 10.3390/bioengineering7030111
48. Thamphiwatana S, Angsantikul P, Escajadillo T, Zhang Q, Olson J, Luk BT, et al. Macrophage-like nanoparticles concurrently absorbing endotoxins and proinflammatory cytokines for sepsis management. Proc Natl Acad Sci U S A. (2017) 114:11488–93. doi: 10.1073/pnas.1714267114
49. Acevedo-Villanueva KY, Lester B, Renu S, Han Y, Shanmugasundaram R, Gourapura R, et al. Efficacy of chitosan-based nanoparticle vaccine administered to broiler birds challenged with Salmonella. PLoS ONE. (2020) 15:187–98. doi: 10.1371/journal.pone.0231998
50. Kim JK, Uchiyama S, Gong H, Stream A, Zhang L, Nizet V. Engineered biomimetic platelet membrane-coated nanoparticles block staphylococcus aureus cytotoxicity and protect against lethal systemic infection. Engineering. (2021) 7:1149–56. doi: 10.1016/j.eng.2020.09.013
51. Liu J, Ding H, Zhao M, Tu F, He T, Zhang L, et al. Functionalized erythrocyte membrane-coated nanoparticles for the treatment of klebsiella pneumoniae-induced sepsis. Front Microbiol. (2022) 13:2289–306. doi: 10.3389/fmicb.2022.901979
52. Liu J, Chen X, Xu L, Tu F, Rui X, Zhang L, et al. Neutrophil membrane-coated nanoparticles exhibit increased antimicrobial activities in an anti-microbial resistant K. pneumonia infection model. Nanomed J. (2023) 48:102640. doi: 10.1016/j.nano.2022.102640
53. Fang RH, Kroll AV, Gao W, Zhang L. Cell membrane coating nanotechnology. Adv Mater. (2018) 30:1–29. doi: 10.1002/adma.201706759
54. Hu CMJ, Fang RH, Wang KC, Luk BT, Thamphiwatana S, Dehaini D, et al. Nanoparticle biointerfacing by platelet membrane cloaking. Nature. (2015) 526:118–21. doi: 10.1038/nature15373
55. Zan G, Wu Q. Biomimetic bioinspired synthesis of nanomaterials /nanostructures. Adv Mater. (2016) 28:2099–147. doi: 10.1002/adma.201503215
56. Sushnitha M, Evangelopoulos M, Tasciotti E, Taraballi F. Cell membrane-based biomimetic nanoparticles and the immune system: immunomodulatory interactions to therapeutic applications. Front Bioeng Biotechnol. (2020) 8:627. doi: 10.3389/fbioe.2020.00627
57. Jiménez-Jiménez C, Manzano M, Vallet-Reg, í M. Nanoparticles coated with cell membranes for biomedical applications. Biology (Basel). (2020) 9:1–17. doi: 10.3390/biology9110406
58. Gareev KG, Grouzdev DS, Koziaeva VV, Sitkov NO, Gao H, Zimina TM, et al. Biomimetic nanomaterials: diversity, technology, biomedical applications. Nanomater. (2022) 12:2485–504. doi: 10.3390/nano12142485
59. Hu CMJ, Zhang L, Aryal S, Cheung C, Fang RH, Zhang L. Erythrocyte membrane-camouflaged polymeric nanoparticles as a biomimetic delivery platform. Proc Natl Acad Sci U S A. (2011) 108:10980–5. doi: 10.1073/pnas.1106634108
60. Li R, He Y, Zhang S, Qin J, Wang J. Cell membrane-based nanoparticles: a new biomimetic platform for tumor diagnosis and treatment. Acta Pharm Sin B. (2018) 8:14–34. doi: 10.1016/j.apsb.2017.11.009
61. Bidkar AP, Sanpui P, Ghosh SS. Red blood cell-membrane-coated poly(lactic-co-glycolic acid) nanoparticles for enhanced chemo- a nd hypoxia-activated therapy. ACS Appl Bio Mater. (2019) 2:4077–86. doi: 10.1021/acsabm.9b00584
62. Xuan M, Shao J, Li J. Cell membrane-covered nanoparticles as biomaterials. Natl Sci Rev. (2019) 6:551–61. doi: 10.1093/nsr/nwz037
63. Liu Y, Luo J, Chen X, Liu W, Chen T. Cell membrane coating technology: a promising strategy for biomedical applications. Nanomicro Lett. (2019) 11:100–31. doi: 10.1007/s40820-019-0330-9
64. Liu T, Shi C, Duan L, Zhang Z, Luo L, Goel S, et al. A highly hemocompatible erythrocyte membrane-coated ultrasmall selenium nanosystem for simultaneous cancer radiosensitization and precise antiangiogenesis. J Mater Chem B. (2018) 6:4756–64. doi: 10.1039/C8TB01398E
65. Guo P, Huang J, Zhao Y, Martin CR, Zare RN, Moses M . A., et al. Nanomaterial preparation by extrusion through nanoporous membranes. Wiley Online Libr. (2018) 14:1–23. doi: 10.1002/smll.201703493
66. Piao JG, Wang L, Gao F, You YZ, Xiong Y, Yang L. Erythrocyte membrane is an alternative coating to polyethylene glycol for prolonging the circulation lifetime of gold nanocages for photothermal therapy. ACS Nano. (2014) 8:10414–25. doi: 10.1021/nn503779d
67. Gao C, Lin Z, Jurado-Sánchez B, Lin X, Wu Z, He Q. Stem cell membrane-coated nanogels for highly efficient in vivo tumor targeted drug delivery. Small. (2016) 12:4056–62. doi: 10.1002/smll.201600624
68. Rao L, He Z, Meng QF, Zhou Z, Bu LL, Guo SS, et al. Effective cancer targeting and imaging using macrophage membrane-camouflaged upconversion nanoparticles. J Biomed Mater Res A. (2017) 105:521–30. doi: 10.1002/jbm.a.35927
69. Copp JA, Fang RH, Luk BT, Hu CMJ, Gao W, Zhang K, et al. Clearance of pathological antibodies using biomimetic nanoparticles. Proc Natl Acad Sci. (2014) 111:13481–97. doi: 10.1073/pnas.1412420111
70. Bose RJ, Kim B. J., Arai Y, bo Han I, Moon JJ, Paulmurugan R, et al. Bioengineered stem cell membrane functionalized nanocarriers for therapeutic targeting of severe hindlimb ischemia. Biomaterials. (2018) 185:360–70. doi: 10.1016/j.biomaterials.2018.08.018
71. Kang T, Zhu Q, Wei D, Feng J, Yao J, Jiang T, et al. Nanoparticles coated with neutrophil membranes can effectively treat cancer metastasis. ACS Nano. (2017) 11:1397–411. doi: 10.1021/acsnano.6b06477
72. Tang J, Shen D, Caranasos TG, Wang Z, Vandergriff AC, Allen TA, et al. Therapeutic microparticles functionalized with biomimetic cardiac stem cell membranes and secretome. Nat Commun. (2017) 8:13724–50. doi: 10.1038/ncomms13724
73. De Ávila BEF, Angsantikul P, Ramírez-Herrera DE, Soto F, Teymourian H, Dehaini D, et al. Hybrid biomembrane-functionalized nanorobots for concurrent removal of pathogenic bacteria and toxins. Sci Robot. (2018) 3:485–98. doi: 10.1126/scirobotics.aat0485
74. Kim K, Lee WG. Electroporation for nanomedicine: a review. J Mater Chem B. (2017) 5:2726–38. doi: 10.1039/C7TB00038C
75. Rao L, Cai B, Bu LL, Liao QQ, Guo SS, Zhao XZ, et al. Microfluidic electroporation-facilitated synthesis of erythrocyte membrane-coated magnetic nanoparticles for enhanced imaging-guided cancer therapy. ACS Nano. (2017) 11:3496–505. doi: 10.1021/acsnano.7b00133
76. Andriola Silva AK, Di Corato R, Pellegrino T, Chat S, Pugliese G, Luciani N, et al. Cell-derived vesicles as a bioplatform for the encapsulation of theranostic nanomaterials. Nanoscale. (2013) 5:11374–84. doi: 10.1039/c3nr01541f
77. Andriola Silva AK, Di Corato R, Pellegrino T, Chat S, Pugliese G, Luciani N, et al. Cell-derived vesicles as a bioplatform for the encapsulation of theranostic nanomaterials. Nanoscale. (2013) 5:11374–84. doi: 10.1039/C3NR01541F
78. He Y, Li R, Liang J, Zhu Y, Zhang S, Zheng Z, et al. Drug targeting through platelet membrane-coated nanoparticles for the treatment of rheumatoid arthritis. Nano Res. (2018) 11:6086–101. doi: 10.1007/s12274-018-2126-5
79. Cao X, Hu Y, Luo S, Wang Y, Gong T, Sun X, et al. Neutrophil-mimicking therapeutic nanoparticles for targeted chemotherapy of pancreatic carcinoma. Acta Pharm Sin B. (2019) 9:575–84. doi: 10.1016/j.apsb.2018.12.009
80. Sun K, Yu W, Ji B, Chen C, Yang H, Du Y, et al. Saikosaponin D loaded macrophage membrane-biomimetic nanoparticles target angiogenic signaling for breast cancer therapy. Appl Mater Today. (2020) 18:100505. doi: 10.1016/j.apmt.2019.100505
81. Ben-Akiva E, Meyer RA, Yu H, Smith JT, Pardoll DM, Green JJ. Biomimetic anisotropic polymeric nanoparticles coated with red blood cell membranes for enhanced circulation and toxin removal. Sci Adv. (2020) 6:1932–43. doi: 10.1126/sciadv.aay9035
82. Dou H, Destache CJ, Morehead JR, Mosley RL, Boska MD, Kingsley J, et al. Development of a macrophage-based nanoparticle platform for antiretroviral drug delivery. Blood. (2006) 108:2827–35. doi: 10.1182/blood-2006-03-012534
83. Mitchell MJ, Billingsley MM, Haley RM, Wechsler ME, Peppas NA, Langer R. Engineering precision nanoparticles for drug delivery. Nat Rev Drug Discov. (2020) 20:101–24. doi: 10.1038/s41573-020-0090-8
84. Mubeen B, Nayab Ansar A, Rasool R, Ullah I, Sarim Imam S, Ghoneim MM, et al. Nanotechnology as a novel approach in combating microbes providing an alternative to antibiotics. Antibiotics (Basel). (2021) 10:1473–97. doi: 10.3390/antibiotics10121473
85. Chugh V, Vijaya Krishna K, Pandit A. Cell membrane-coated mimics: a methodological approach for fabrication, characterization for therapeutic applications, and challenges for clinical translation. ACS Nano. (2021) 15:17080–123. doi: 10.1021/acsnano.1c03800
86. Chen Y, Zhang Y, Zhuang J, Lee JH, Wang L, Fang RH, et al. Cell-Membrane-Cloaked oil nanosponges enable dual-modal detoxification article. ACS Nano. (2019) 13:7209–15 doi: 10.1021/acsnano.9b02773
87. Zhang X, Angsantikul P, Ying M, Zhuang J, Zhang Q, Wei X, et al. Remote loading of small molecule therapeutics into cholesterol-enriched cell membrane-derived vesicles. Angew Chem Int Ed Eng. (2017) 56:14075–9. doi: 10.1002/anie.201707598
88. Annamalai T, Pina-Mimbela R, Kumar A, Binjawadagi B, Liu Z, Renukaradhya GJ, et al. Evaluation of nanoparticle-encapsulated outer membrane proteins for the control of Campylobacter jejuni colonization in chickens. Poult Sci. (2013) 92:2201–11. doi: 10.3382/ps.2012-03004
89. Escajadillo T, Olson J, Luk BT, Zhang L, Nizet V. A red blood cell membrane-camouflaged nanoparticle counteracts streptolysin O-mediated virulence phenotypes of invasive group A Streptococcus. Front Pharmacol. (2017) 8:477–86. doi: 10.3389/fphar.2017.00477
90. Koo J, Escajadillo T, Zhang L, Nizet V, Lawrence SM. Erythrocyte-Coated Nanoparticles Block Cytotoxic Effects of Group B Streptococcus β-Hemolysin/Cytolysin. Front. Pediatr. (2019) 7:410–23. doi: 10.3389/fped.2019.00410
91. Cobo-Angel CG, Jaramillo-Jaramillo AS, Palacio-Aguilera M, Jurado-Vargas L, Calvo-Villegas EA, Ospina-Loaiza DA, et al. Potential group B Streptococcus interspecies transmission between cattle and people in Colombian dairy farms. Sci Rep. (2019) 9:1–9. doi: 10.1038/s41598-019-50225-w
92. Angsantikul P, Thamphiwatana S, Zhang Q, Spiekermann K, Zhuang J, Fang RH, et al. Coating nanoparticles with gastric epithelial cell membrane for targeted antibiotic delivery against Helicobacter pylori infection. Adv Ther. (2018) 1:1–20. doi: 10.1002/adtp.201800016
93. González-Martín M, Silva V, Poeta P, Corbera JA, Tejedor-Junco MT. Microbiological aspects of osteomyelitis in veterinary medicine: drawing parallels to the infection in human medicine. Vet Q. (2022) 42:1–25. doi: 10.1080/01652176.2021.2022244
94. Park JH, Jiang Y, Zhou J, Gong H, Mohapatra A, Heo J, et al. Genetically engineered cell membrane-coated nanoparticles for targeted delivery of dexamethasone to inflamed lungs. Sci Adv. (2021) 7:7820–36. doi: 10.1126/sciadv.abf7820
95. Shen S, Han F, Yuan A, Wu L, Cao J, Qian J, et al. Engineered nanoparticles disguised as macrophages for trapping lipopolysaccharide and preventing endotoxemia. Biomaterials. (2019) 189:60–8. doi: 10.1016/j.biomaterials.2018.10.029
96. Wang C, Wang Y, Zhang L, Miron RJ, Liang J, Shi M, et al. Pretreated macrophage-membrane-coated gold nanocages for precise drug delivery for treatment of bacterial infections. Adv Mater. (2018) 30. doi: 10.1002/adma.201804023
97. Gao F, Xu L, Yang B, Fan F, Yang L. Kill the real with the fake: eliminate intracellular staphylococcus aureus using nanoparticle coated with its extracellular vesicle membrane as active-targeting drug carrier. ACS Infect Dis. (2019) 5:218–27. doi: 10.1021/acsinfecdis.8b00212
98. Hou X, Zeng H, Chi X, Hu X. Pathogen receptor membrane-coating facet structures boost nanomaterial immune escape and antibacterial performance. Nano Lett. (2021) 21:9966–75. doi: 10.1021/acs.nanolett.1c03427
99. Ahsan H, Nawaz Z, Aslam B, Gul M, Shahid M, Siddique AB. Research Article Isolation, molecular characterization and antimicrobial susceptibility testing of Pseudomonas aeruginosa from skin infection of dogs. Cont Vet J. (2022) 2:76–80.
100. Zhou J, Krishnan N, Guo Z, Ventura CJ, Holay M, Zhang Q, et al. Nanotoxoid vaccination protects against opportunistic bacterial infections arising from immunodeficiency. Sci Adv. (2022) 8:5492–511. doi: 10.1126/sciadv.abq5492
101. Renu S, Markazi AD, Dhakal S, Lakshmanappa YS, Shanmugasundaram R, Selvaraj RK, et al. Oral deliverable mucoadhesive chitosan-salmonella subunit nanovaccine for layer chickens. Int J Nanomedicine. (2020) 15:761–73. doi: 10.2147/IJN.S238445
102. Paczosa MK, Mecsas J. Klebsiella pneumoniae: going on the offense with a strong defense. Microbiol Mol Biol Rev. (2016) 80:629–61. doi: 10.1128/MMBR.00078-15
103. Davis GS, Price LB. Recent research examining links among klebsiella pneumoniae from food, food animals, and human extraintestinal infections. Curr Environ Heal reports. (2016) 3:128–35. doi: 10.1007/s40572-016-0089-9
104. Liu Y, Liu C, Zheng W, Zhang X, Yu J, Gao Q, et al. PCR detection of Klebsiella pneumoniae in infant formula based on 16S–23S internal transcribed spacer. Int J Food Microbiol. (2008) 125:230–5. doi: 10.1016/j.ijfoodmicro.2008.03.005
105. Nawaz M, Khan SA, Tran Q, Sung K, Khan AA, Adamu I, et al. Isolation and characterization of multidrug-resistant Klebsiella spp. isolated from shrimp imported from Thailand. Int J Food Microbiol. (2012) 155:179–84. doi: 10.1016/j.ijfoodmicro.2012.02.002
106. Wu H, Liu B, Liu J, Pan Y, Yuan L, Hu Phenotypic G molecular characterization of CTX-M-14 extended-spectrum β-lactamase and plasmid-mediated ACT-like AmpC β-lactamase produced by Klebsiella pneumoniae isolates from chickens in Henan Province China. Genet Mol Res. (2012) 11:3357–64. doi: 10.4238/2012.September.24.1
107. Falomir MP, Rico H, Gozalbo D. Enterobacter and klebsiella species isolated from fresh vegetables marketed in Valencia (Spain) and their clinically relevant resistances to chemotherapeutic agents. Food borne Path n Dis. (2013) 10:1002–1007. doi: 10.1089/fpd.2013.1552
108. Theocharidi NA, Balta I, Houhoula D, Tsantes AG, Lalliotis GP, Polydera AC, et al. High prevalence of klebsiella pneumoniae in greek meat products: detection of virulence and antimicrobial resistance genes by molecular techniques. Foods. (2022) 11:708–29. doi: 10.3390/foods11050708
109. Dcosta VM, King CE, Kalan L, Morar M, Sung WWL, Schwarz C, et al. Antibiotic resistance is ancient. Nat. (2011) 477:457–61. doi: 10.1038/nature10388
111. Retamal P, Gaspar J, Benavides MB, Saenz L, Galarce N, Aravena T, et al. Virulence and antimicrobial resistance factors in Salmonella enterica serotypes isolated from pigs and chickens in central Chile. Front Vet Sci. (2022) 9:1451–76. doi: 10.3389/fvets.2022.971246
112. Elez R, Elsohaby I, El-Gazzar N, Tolba HMN, Abdelfatah EN, Abdellatif SS, et al. Antimicrobial Resistance of Salmonella enteritidis and Salmonella typhimurium Isolated from Laying Hens, Table Eggs, and humans with respect to antimicrobial activity of biosynthesized silver nanoparticles. Anim. (2021) 11: 3554 11:3554. doi: 10.3390/ani11123554
113. Aung KT, Khor WC, Ong KH, Tan WL, Wong ZN, Oh JQ, et al. Characterisation of Salmonella enteritidis ST11 and ST1925 associated with human intestinal and extra-intestinal infections in Singapore. Int J Environ Res Public Health. (2022) 19:5671–92. doi: 10.3390/ijerph19095671
114. Khatun MF, Khan MAS, Ahmed MF, Rahman MM, Rahman SR. Assessment of foodborne transmission of Salmonella enteritidis in hens and eggs in Bangladesh. Vet Med Sci. (2022) 8:2032–9. doi: 10.1002/vms3.874
115. Hassan M, Ali A, Wajid M, Ahmad A, Saleemi MK, Sarwar Y, et al. Purification and antigenic detection of lipopolysaccharides of Salmonella enterica serovar Typhimurium isolate from Faisalabad, Pakistan. Pak Vet J. (2021) 41:434–8. doi: 10.29261/pakvetj/2021.046
116. Gantois I, Ducatelle R, Pasmans F, Haesebrouck F, Gast R, Humphrey TJ, et al. Mechanisms of egg contamination by Salmonella Enteritidis. FEMS Microbiol Rev. (2009) 33:718–38. doi: 10.1111/j.1574-6976.2008.00161.x
117. Hoelzer K, Switt AIM, Wiedmann M. Animal contact as a source of human non-typhoidal salmonellosis. Vet Res. (2011) 42:34–46. doi: 10.1186/1297-9716-42-34
118. Ezzat M, Abdelwahab A, Hassanin I, Mahmoud AE, Ismail SM, El-Tarabili RM. Risk factors, antibiotic profile, and molecular detection of virulence and antibiotic resistance genes of enteric bacteria in diarrheic calves in Egypt. Int J Vet Sci. (2023) 12:161–168. doi: 10.47278/journal.ijvs/2022.186
119. Eng SK, Pusparajah PN, Mutalib SA, Ser HL, Chan KG, Lee LH. Salmonella: A review on pathogenesis, epidemiology and antibiotic resistance. Fron life Sci. (2015) 8:284–93. doi: 10.1080/21553769.2015.1051243
120. da Silva KE, Tanmoy AM, Pragasam AK, Iqbal J, Sajib MSI, Mutreja A, et al. The international and intercontinental spread and expansion of antimicrobial-resistant Salmonella Typhi: a genomic epidemiology study. Lancet Microbe. (2022) 3:567–77. doi: 10.1016/S2666-5247(22)00093-3
121. Awad A, Gwida M, Khalifa E, Sadat A. Phenotypes, antibacterial-resistant profile, and virulence-associated genes of Salmonella serovars isolated from retail chicken meat in Egypt. Vet World. (2020) 13:440–63. doi: 10.14202/vetworld.2020.440-445
122. Adel WA, Ahmed AM, Hegazy Y, Torky HA, Shimamoto T. High prevalence of ESBL and plasmid-mediated quinolone resistance genes in salmonella enterica isolated from retail meats and slaughterhouses in Egypt. Antibiot. (2021) 10:881–90. doi: 10.3390/antibiotics10070881
123. Torky HA, Khaliel SAE, Sedeek EK, Tawfik RG, Bkheet AAE, Ebied SK, et al. Silver nanoparticle effect on Salmonella enterica isolated from Northern West Egypt food, poultry, and calves. Appl Microbiol Biotechnol. (2022) 106:5701–37. doi: 10.1007/s00253-022-12102-x
124. Ruvalcaba-Gómez JM, Villagrán Z, Valdez-Alarcón JJ, Martínez-Núñez M, Gomez-Godínez LJ, Ruesga-Gutiérrez E, et al. Non-antibiotics strategies to control salmonella infection in poultry. Anim an Open Access J from MDPI. (2022) 12. doi: 10.3390/ani12010102
125. El-Saadony MT, Salem HM, El-Tahan AM, Abd El-Mageed TA, Soliman SM, Khafaga AF, et al. The control of poultry salmonellosis using organic agents: an updated overview. Poult Sci. (2022) 101. doi: 10.1016/j.psj.2022.101716
126. Javed MU, Ijaz M, Fatima Z, Anjum AA, Aqib AI, Ali MM, et al. Frequency and antimicrobial susceptibility of methicillin and vancomycin-resistant staphylococcus aureus from bovine milk. Pak Vet J. (2021) 41:463–8. doi: 10.29261/pakvetj/2021.060
127. Turner NA, Sharma-Kuinkel BK, Maskarinec SA, Eichenberger EM, Shah PP, Carugati M, et al. Methicillin-resistant Staphylococcus aureus: an overview of basic and clinical research. Nat. Rev. Microbiol. (2019) 17:203–18. doi: 10.1038/s41579-018-0147-4
128. Algammal AM, Hetta HF, Elkelish A, Alkhalifah DHH, Hozzein WN, Batiha GES, et al. Methicillin-Resistant Staphylococcus aureus (MRSA): one health perspective approach to the bacterium epidemiology, virulence factors, antibiotic-resistance, zoonotic impact. Infect Drug Resist. (2020) 13:3255–64. doi: 10.2147/IDR.S272733
129. Devriese LA, Van Damme LR, Fameree L. Methicillin (cloxacillin)-resistant Staphylococcus aureus strains isolated from bovine mastitis cases. Zentralbl Veterinarmed B. (1972) 19:598–605. doi: 10.1111/j.1439-0450.1972.tb00439.x
130. Aires-de-Sousa M. Methicillin-resistant Staphylococcus aureus among animals: current overview. Clin Microbiol Infect. (2017) 23:373–380. doi: 10.1016/j.cmi.2016.11.002
131. Hizlisoy H, Ertas Onmaz N, Karadal F, Al S, Yildirim Y, Gonulalan Z, et al. Antibiotic Resistance Gene Profiles of Staphylococcus aureus Isolated From Foods of Animal Origin. Kafkas Univ Vet Fak Derg. (2018) 24:243–9.
132. Anjum MF, Marco-Jimenez F, Duncan D, Marín C, Smith RP, Evans SJ. Livestock-Associated Methicillin-Resistant Staphylococcus aureus From Animals and Animal Products in the UK. Front Microbiol. (2019) 10:1–21. doi: 10.3389/fmicb.2019.02136
133. Javed S, McClure J, Syed MA, Obasuyi O, Ali S, Tabassum S, et al. Epidemiology and molecular characterization of Staphylococcus aureus causing bovine mastitis in water buffaloes from the Hazara division of Khyber Pakhtunkhwa, Pakistan. PLoS ONE. (2022) 17:1–18. doi: 10.1371/journal.pone.0268152
134. Chon J, Sung K, Khan S, Chon J, Sung K, Khan S. Methicillin-resistant Staphylococcus aureus (MRSA) in food- producing and companion animals and food products. Front Staphylococcus aureus. (2017) 47–102. doi: 10.5772/66645
135. Asyraf M, Masimen A, Maulidiani M. Overcoming methicillin-resistance Staphylococcus aureus (MRSA) using antimicrobial peptides-silver nanoparticle. J Antibiot. (2022) 11:951–67. doi: 10.3390/antibiotics11070951
136. Oliveira D, Borges A, Simões M. Staphylococcus aureus Toxins and Their Molecular Activity in Infectious Diseases. Toxins (Basel). (2018) 10:1–31. doi: 10.3390/toxins10060252
137. Ijaz M, Manzoor A, Tahir Mohy-Ud-Din M, Hassan F, Mohy-Ud-Din Z, Ans M, et al. Pakistan Veterinary Journal An Economical Non-Antibiotic Alternative to Antibiotic Therapy for Subclinical Mastitis in Cows. Front Vet Sci. (2021) 9:966533. doi: 10.3389/fvets.2022.966533
138. Murrad S, Ali MA, Rabbani M, Anjum AA, Avais M, Yaqub T, et al. Antibiotic resistance pattern of mastitis causing escherichia coli toxinotypes. Pak Vet J. (2020) 40:264–6. doi: 10.29261/pakvetj/2019.004
139. Huang R, Cai GQ, Li J, Li XS, Liu HT, Shang XL, et al. Platelet membrane-camouflaged silver metal-organic framework drug system against infections caused by methicillin-resistant Staphylococcus aureus. J. Nanobiotechnol. (2021) 19:1–19. doi: 10.1186/s12951-021-00978-2
140. Ramos S, Silva V, de Lurdes Enes Dapkevicius M, Caniça M, Tejedor-Junco MT, Igrejas G, et al. Escherichia coli as commensal and pathogenic bacteria among food-producing animals: health implications of extended spectrum β-lactamase (ESBL) production. Anim. (2020) 10:1–15. doi: 10.3390/ani10122239
141. Hunt JM. Shiga toxin-producing Escherichia coli (STEC). Clin Lab Med. (2010) 30:21–45. doi: 10.1016/j.cll.2009.11.001
142. Zhang H, Ur Rehman M, Li K, Luo H, Lan Y, Nabi F, et al. Pakistan veterinary journal antimicrobial resistance of Escherichia coli isolated from Tibetan piglets suffering from white score diarrhea. Pak Vet J. (2017) 37:43–46.
143. Irshad H, Binyamin I, Ahsan A, Riaz A, Shahzad MA, Qayyum M, et al. Occurrence and molecular characterization of Shiga toxin-producing Escherichia coli isolates recovered from cattle and goat meat obtained from retail meat shops in Rawalpindi and Islamabad. Pak vet J. (2020) 40:295–300. doi: 10.29261/pakvetj/2020.045
144. Khan AY, Ahmad SS, Avais M, Ashraf K. Molecular prevalence with associated risk factors and haemato-serum electrolyte analysis of E. coli O157:H7 in Canine pups with diarrhoea. Pak Vet J. (2022) 42:161–6. doi: 10.29261/pakvetj/2022.009
145. Mehmood K, Bilal RM, Zhang H. Study on the genotypic and phenotypic resistance of tetracycline antibiotic in escherichia coli strains isolated from free ranging chickens of anhui province, china. Agrobiol Rec. (2020) 2:63–8. doi: 10.47278/journal.abr/2020.014
146. Stein RA, Katz DE. Escherichia coli, cattle and the propagation of disease. FEMS Microbiol. Lett. (2017) 364:50–57 doi: 10.1093/femsle/fnx050
147. El-Demerdash AS, Bakry NR, Aggour MG, Elmasry SS, Mowafy RE, Erfan A, et al. Bovine Mastitis in Egypt: bacterial etiology and evaluation of diagnostic biomarkers. Int J Vet Sci. (2022) 12:60–9. doi: 10.47278/journal.ijvs/2022.161
148. Kim SM, Eo KY, Park TM, Cho GJ. Evaluation of usefulness of infrared thermography for the detection of mastitis based on teat skin surface temperatures in dairy cows. Inter J Vet Sci. (2022) 12:1–6. doi: 10.47278/journal.ijvs/2022.151
149. Suojala L, Kaartinen L, Pyörälä S. Treatment for bovine Escherichia coli mastitis – an evidence-based approach. J Vet Pharmacol Ther. (2013) 36:521–31. doi: 10.1111/jvp.12057
150. Van TTH, Yidana Z, Smooker PM, Coloe PJ. Antibiotic use in food animals worldwide, with a focus on Africa: Pluses and minuses. J Glob Antimicrob Resist. (2020) 20:170–7. doi: 10.1016/j.jgar.2019.07.031
151. Kusters JG, Van Vliet A, Kuipers EJ. Pathogenesis of Helicobacter pylori infection. Clin Microbiol Rev. (2006) 19:449–63. doi: 10.1128/CMR.00054-05
152. Yang H, Hu B. (2022). Immunological perspective: Helicobacter pylori infection and gastritis. Mediators Inflamm. (2022) 2022:156–189. doi: 10.1155/2022/2944156
153. Kolodzieyski L, Kim B, Park H, Yoon HS, Lim CW. Prevalence of gastrospirillum-like organisms in pigs, cattle, and dogs: a comparison of diagnostic methods between species. Vet Med (Praha). (2008) 53:193–202. doi: 10.17221/1919-VETMED
154. Quaglia NC, Dambrosio A, Normanno G, Parisi A, Patrono R, Ranieri G, et al. High occurrence of Helicobacter pylori in raw goat, sheep and cow milk inferred by glmM gene: a risk of food-borne infection? Int. J Food Microbiol. (2008) 124:43–7. doi: 10.1016/j.ijfoodmicro.2008.02.011
155. Momtaz H, Dabiri H, Souod N, Gholami M. Study of Helicobacter pylori genotype status in cows, sheep, goats and human beings. BMC Gastroenterol. (2014) 14:61–96. doi: 10.1186/1471-230X-14-61
156. Zhou Y, Zhong Z, Hu S, Wang J, Deng Y, Li X, et al. A Survey of Helicobacter pylori Antibiotic-Resistant Genotypes and Strain Lineages by Whole-Genome Sequencing in China. Antimicrob. Agents Chemother. (2022) 66:2188–221. doi: 10.1128/aac.02188-21
157. Zhang Y, Chen Y, Lo C, Zhuang J, Angsantikul P, Zhang Q, et al. Inhibition of pathogen adhesion by bacterial outer membrane-coated nanoparticles. Angew Chem Int Ed Engl. (2019) 58:11404–8. doi: 10.1002/anie.201906280
158. Hankel J, Jung K, Kuder H, Keller B, Keller C, Galvez E, et al. Caecal microbiota of experimentally campylobacter jejuni-infected chickens at different ages. Front Microbiol. (2019) 10:2303–21. doi: 10.3389/fmicb.2019.02303
159. Ghazi W, Hakeem A, Fathima S, Shanmugasundaram R, Selvaraj RK. Campylobacter jejuni in poultry : pathogenesis and control strategies. Microorganisms. (2022) 10:1–18. doi: 10.3390/microorganisms10112134
160. Rouger A, Tresse O, Zagorec M. Bacterial Contaminants of Poultry Meat: Sources, Species, and Dynamics. Microorganisms. (2017) 5:1–26. doi: 10.3390/microorganisms5030050
161. Hermans D, Pasmans F, Messens W, Martel A, Van Immerseel F, Rasschaert G, et al. Poultry as a host for the zoonotic pathogen Campylobacter jejuni. Vector Borne Zoonotic Dis. (2012) 12:89–98. doi: 10.1089/vbz.2011.0676
162. Neal-McKinney JM, Samuelson DR, Eucker TP, Nissen MS, Crespo R, et al. Reducing Campylobacter jejuni colonization of poultry via vaccination. PLoS One. (2014) 9:1–9. doi: 10.1371/journal.pone.0114254
163. Johnson TJ, Shank JM, Johnson JG. Current potential treatments for reducing Campylobacter colonization in animal hosts and disease in humans. Front Microbiol. (2017) 8:487–521. doi: 10.3389/fmicb.2017.00487
164. Ushanov L. Reduction of C. jejuni may require complex approach. Ann Agrar Sci. (2018) 16:422–6. doi: 10.1016/j.aasci.2018.07.001
165. Singh A, Khan A, Ghosh T, Mondal S, Mallick AI. Naturally secreted bacterial outer membrane vesicles: potential platform for a vaccine against Campylobacter jejuni. bioRxiv. (2020) 10:1–23. doi: 10.1101/2020.10.16.342261
166. Singh A, Khan A, Ghosh T, Mondal S, Mallick AI. Gut microbe-derived outer membrane vesicles: a potential platform to control cecal load of Campylobacter jejuni. ACS Infect Dis. (2021) 7:1186–99. doi: 10.1021/acsinfecdis.0c00744
167. Lyhs U, Kulkas L, Katholm J, Waller KP, Saha K, Tomusk RJ, et al. Streptococcus agalactiae serotype IV in humans and cattle, Northern Europe. Emerg Infect Dis. (2016) 22:2097. doi: 10.3201/eid2212.151447
168. Sørensen UBS, Klaas IC, Boes J, Farre M. The distribution of clones of Streptococcus agalactiae (group B streptococci) among herdspersons and dairy cows demonstrates lack of host specificity for some lineages. Vet Microbiol. (2019) 235:71–9. doi: 10.1016/j.vetmic.2019.06.008
169. Delannoy CMJ, Crumlish M, Fontaine MC, Pollock J, Foster G, Dagleish MP, et al. Human Streptococcus agalactiae strains in aquatic mammals and fish. BMC Microbiol. (2013) 13:1–9. doi: 10.1186/1471-2180-13-41
170. Numberger D, Siebert U, Fulde M, Valentin-Weigand P. Streptococcal infections in marine mammals. Microorganisms. (2021) 9:1–25. doi: 10.3390/microorganisms9020350
171. Goyette-Desjardins G, Auger JP, Xu J, Segura M, Gottschalk M. Streptococcus suis, an important pig pathogen and emerging zoonoticagent—an update on the worldwide distribution based on serotyping and sequencetyping. Emerg Microbes Infect. (2014) 3:45–62. doi: 10.1038/emi.2014.45
172. Thongkamkoon P, Kiatyingangsulee T, Gottschalk M. Serotypes of Streptococcus suis isolated from healthy pigs in Phayao Province, Thailand. BMC Res Notes. (2017) 10:1–7. doi: 10.1186/s13104-016-2354-2
173. Segura M, Aragon V, Brockmeier SL, Gebhart C, de Greeff A, Kerdsin A, et al. Update on Streptococcus suis research and prevention in the era of antimicrobial restriction: 4th international workshop on S. suis. Pathog. (2020) 9:374–97. doi: 10.3390/pathogens9050374
174. Kerdsin A, Segura M, Fittipaldi N, Gottschalk M. Sociocultural Factors Influencing Human Streptococcus suis Disease in Southeast Asia. Foods. (2022) 11:1190–225. doi: 10.3390/foods11091190
175. Kabelitz T, Aubry E, van Vorst K, Amon T, Fulde M. The role of Streptococcus spp. in Bovine Mastitis. Microorganisms. (2021) 9:1497–521. doi: 10.3390/microorganisms9071497
176. Neila-Ibáñez C, Brogaard L, Pailler-García L, Martínez J, Segalés J, Segura M, et al. Piglet innate immune response to Streptococcus suis colonization is modulated by the virulence of the strain. Vet Res. (2021) 52:145–69. doi: 10.1186/s13567-021-01013-w
177. van Driel ML, de Sutter AI, Habraken H, Thorning S, Christiaens T. Different antibiotic treatments for group A streptococcal pharyngitis cochrane database. Syst Rev. (2016) 9:CD004406. doi: 10.1002/14651858.CD004406.pub4
178. Haenni M, Lupo A, Madec YJ. Antimicrobial resistance in Streptococcus spp. Microbiol Spectr. (2018) 6:1–32. doi: 10.1128/microbiolspec.ARBA-0008-2017
179. Alves-Barroco C, Rivas-García L, Fernandes AR, Baptista PV. Tackling multidrug resistance in streptococci – from novel biotherapeutic strategies to nanomedicines. Front Microbiol. (2020) 11:2487–508. doi: 10.3389/fmicb.2020.579916
Keywords: bacteria, nanoparticles, cell, infection, animals, antibiotic, food
Citation: Altaf S and Alkheraije KA (2023) Cell membrane-coated nanoparticles: An emerging antibacterial platform for pathogens of food animals. Front. Vet. Sci. 10:1148964. doi: 10.3389/fvets.2023.1148964
Received: 20 January 2023; Accepted: 14 February 2023;
Published: 06 March 2023.
Edited by:
Khalid Mehmood, Islamia University of Bahawalpur, PakistanReviewed by:
Seyed Mostafa Hosseini, Hamadan University of Medical Sciences, IranSamah Attia Algharib, Faculty of Veterinary Medicine, Egypt
Riaz Hussain, Islamia University of Bahawalpur, Pakistan
Copyright © 2023 Altaf and Alkheraije. This is an open-access article distributed under the terms of the Creative Commons Attribution License (CC BY). The use, distribution or reproduction in other forums is permitted, provided the original author(s) and the copyright owner(s) are credited and that the original publication in this journal is cited, in accordance with accepted academic practice. No use, distribution or reproduction is permitted which does not comply with these terms.
*Correspondence: Khalid Ali Alkheraije, ay5hbGtoZXJhaWplQHF1LmVkdS5zYQ==