- 1Planetary Science Institute, Tucson, AZ, United States
- 2NASA Goddard Space Flight Center, Greenbelt, MD, United States
- 3Jet Propulsion Laboratory, Pasadena, CA, United States
- 4Climate and Space Sciences and Engineering, University of Michigan, Ann Arbor, MI, United States
- 5U.S. Naval Research Laboratory, Washington, DC, United States
- 6Space Science Laboratory, University of Massachusetts Lowell, Lowell, MA, United States
- 7Space Sciences Laboratory, University of California-Berkeley, Berkeley, CA, United States
- 8National Solar Observatory, Boulder, CO, United States
- 9Center for Astrophysics & Space Sciences, University of California, San Diego, San Diego, CA, United States
- 10Department of Astrophysical and Planetary Sciences, University of Colorado, Boulder, CO, United States
There continue to be open questions regarding the solar wind and coronal mass ejections (CMEs). For example: how do magnetic fields within CMEs and corotating/stream interaction regions (CIRs/SIRs) evolve in the inner heliosphere? What is the radially distributed magnetic profile of shock-driving CMEs? What is the internal magnetic structure of CMEs that cause magnetic storms? It is clear that these questions involve the magnetic configurations of solar wind and transient interplanetary plasma structures, for which we have limited knowledge. In order to better understand the origin of the magnetic field variability in steady-state structures and transient events, it is necessary to probe the magnetic field in Earth-directed structures/disturbances. This is the goal of the Multiview Observatory for Solar Terrestrial Science (MOST) mission (Gopalswamy et al., 2022). For MOST to answer the aforementioned questions, we propose the instrument concept of the Faraday Effect Tracker of Coronal and Heliospheric structures (FETCH), a simultaneous quad-line-of-sight polarization radio remote-sensing instrument. With FETCH, spacecraft radio beams passing through the Sun–Earth line offer the possibility of obtaining information of plasma conditions via analysis of radio propagation effects such as Faraday rotation and wave dispersion, which provide information of the magnetic field and total electron content (TEC). This is the goal of the FETCH instrument, one of ten instruments proposed to be hosted on the MOST mission. The MOST mission will provide an unprecedented opportunity to achieve NASA’s heliophysics science goal to “explore and characterize the physical processes in the space environment from the Sun” (Gopalswamy et al., 2022).
1 Introduction
Within the interplanetary plasma, coronal mass ejections (CMEs) and stream interaction regions (SIRs) constitute very large and intensive transient structures. From their origins in the solar corona, CMEs and SIRs drive space weather on Earth, affecting society (Council, 2008; Eastwood et al., 2017; Oughton et al., 2017). CMEs are most impactful during solar maximum, while SIRs are notable in disrupting the Earth’s geomagnetic space during solar minimum (Zhang et al., 2007; Jian et al., 2015; Gopalswamy, 2016). Geomagnetic storms can bring numerous space weather hazards. Intense particle radiation can cause satellite malfunction and pose a health threat to astronauts. Ionospheric heating and expansion in turn increase drag on satellites, with debris degrading their orbits. Time-varying magnetic fields induce surges in electricity lines. Geomagnetically disturbed ionospheric scintillations can distort the amplitude and phase of traversing radio signals, causing a risk of navigation errors and signal loss. The Committee on the Societal and Economic Impacts of Severe Space Weather Events (2008) estimated a “severe geomagnetic storm scenario” that could incur 1 trillion in societal and economic damage (see references in Jensen et al., 2010 for specific examples).
Understanding and predicting the effects of CMEs and SIRs require sufficient observations of their plasma properties to inform predictive models, including various magnetohydrodynamic (MHD) models. To address these and related questions, the Solar Terrestrial Relations Observatory (STEREO) mission was launched in October 2006 (Kaiser et al., 2008). To observe solar wind plasma prior to the Earth’s L1 Lagrangian position (Kaiser et al., 2008; Panchenko et al., 2014), each STEREO spacecraft on either side of the Earth–Sun line possesses a pair of coronagraphs, Cor1 and Cor2, and a pair of heliospheric imagers (HIs), HI1 and HI2; combined together, they observe solar wind plasma in Thomson scattered light continuously from the low corona to beyond 1 au. Analysis of these datasets provides dramatic observations of plasma density structures associated with CMEs and SIRs; however, STEREO is unable to remotely observe one of the most important features in transient structures, namely, the magnetic field. In contrast, Faraday rotation (FR) observations have detected clear magnetic structures in several CME events (Kooi et al., 2022b, Kooi et al., 2021; Jensen and Russell, 2008). Numerical simulations of FR measure have demonstrated that for idealized models, the magnetic field orientation and helicity of a flux rope can be determined 2–3 days before it reaches 1 au, providing a valuable space weather forecast (Liu et al., 2007). FR observations can also resolve the portion of CME flux ropes that curves back to the Sun (Liu et al., 2007).
In addition to large-scale structures, studies have shown that FR observations can provide critical information on plasma waves, which are significant to the flow of turbulent energy in interplanetary space (Jensen et al., 2013; Efimov et al., 2019; Kooi et al., 2022c). For example, MHD waves facilitate the transfer of energy between spatial and temporal scale sizes as shown in turbulence studies (Viall and Borovsky, 2020). The waves have a significant role in discontinuities and shocks (Kilpua et al., 2022); they can drive particle heating and acceleration (Suzuki and Inutsuka, 2005), and their presence constrains the characteristics of the plasma that can support them (Hollweg et al., 1982; 2010).
In order to fully exploit FR to probe the magnetic field in Earth-directed structures (e.g., CMEs/SIRs) and other disturbances (e.g., MHD waves), we propose the Faraday Effect Tracker of Coronal and Heliospheric structures (FETCH) for measuring the inner heliospheric magnetic field (Gopalswamy et al., 2022). While photospheric and chromospheric magnetic fields can be readily measured using magnetographs, novel techniques are needed to measure the magnetic field with radio waves, especially at distances far from the Sun. FETCH is one of the proposed instruments on the Multiview Observatory for Solar Terrestrial Science (MOST) (Gopalswamy et al., 2022), for which the goal is to understand the magnetic coupling between the solar interior and the heliosphere and the origin and variability of the solar wind and transient structures. The MOST mission consists of a total of four spacecraft, two pairs of spacecraft distributed in the vicinity of each of the Sun–Earth Lagrange points L4 and L5 and close to the quadrature (see Figure 1). The FETCH-polarized radio transmitters and receivers will be on all four spacecraft to measure the integrated magnetic field along four lines of sight crossing the Sun–Earth line. The frequency of the radio signals is low enough to experience measurable changes in intensity, polarization angle, degree of linear polarization, and apparent Doppler frequency. Between four spacecraft, four propagation paths (aka lines-of-sight) are established for these crucial measurements (Figure 1).
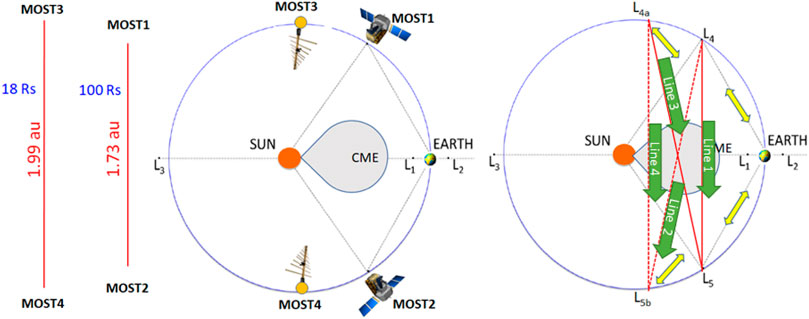
FIGURE 1. Overview of the MOST mission with the four constituent spacecraft at L4 (MOST1), L5 (MOST2), ahead of L4 (L4a, MOST3), and behind L5 (L5b, MOST4). MOST3 and 4 will carry only radio equipment for FETCH. MOST1 and 2 will have identical remote-sensing and in situ instrument suites. The approximate MOST1→MOST2 and MOST3→MOST4 distances are shown at the left, indicating the long signal paths for spacecraft radio signals. (Blue text shows the offset distance to the point-of-closest-approach between the LOS and the Sun). The red lines in the right indicate FETCH signal paths; the green arrows label these paths line 1–4 and give the forward directions. This enables a rudimentary radio tomographic image using FR (Fung et al., 2022). The yellow double arrows indicate communication links to Earth. Note that MOST3 and 4 slowly shift position between L4 and 5 and the locations shown throughout the MOST mission [adapted from Gopalswamy et al. (2022)].
2 FETCH goals and objectives: CMEs, SIRs, and MHD waves
FETCH is an active radio instrument (emitting radio waves for measuring rather than relying on astrophysical sources of radio waves such as pulsars), detecting the modifications of a transmitted signal caused by its traversal through a magnetized plasma. FETCH’s greatest technological challenge is achieving a sufficient signal-to-noise ratio, given the restrictions of power, antenna collecting areas, and distance. It will be the first instrument to pass very high frequency (VHF) radio signals between spacecraft, which are separated by 2 au at positions near L4 and L5. As we detail in the instrument concept later, the signal frequency, phase, polarization, amplitude, and spectral distribution will be combined to measure FR, total electron content (TEC), Faraday rotation fluctuations (FRF), and frequency fluctuations (FF) using established techniques (Efimov et al., 2000; Imamura et al., 2014; Wexler et al., 2017; Jensen et al., 2018; Wexler et al., 2021; Kooi et al., 2022a; Kooi et al., 2022b; Kooi et al., 2022c; Kooi et al., 2022d; Kooi et al., 2022e; Wood et al., 2022).
FR analysis of linearly polarized signals provides information on the LOS-integrated product of electron number density (ne) and LOS-aligned component of the magnetic field
with A = 2.36 × 104 in mks units rad m2 T−1 s−2 and the frequency of the signal fo in Hz. A convention that we commonly use to express FR is to normalize it by wavelength λ to calculate the rotation measure (RM).
The total electron content is the measure of the column density of plasma along the LOS. The TEC is critical to deconvolving the average LOS magnetic field strength from the RM, and with the use of tomography, TEC can provide the 3-D plasma density. The TEC can be obtained from both the radio signal’s group and phase velocities. The group velocity translates to an arrival time delay, famously measured with the differenced range versus integrated Doppler (DRVID) (Woo et al., 1976). In contrast, the phase velocity gives the change in TEC and is measured from an apparent Doppler shift in the FF of the narrowband signal after the Doppler shift has been removed (Jensen et al., 2016) [see Kooi et al. (2022a) for more information].
CMEs produce the largest scale and most intense disturbances in the heliosphere (Chen, 2011; Temmer, 2021). As they approach Earth, the magnetic ejecta may extend over 0.25 au, and the CME-driven shock may extend twice as far (Jian et al., 2006; Kilpua et al., 2017a; Manchester et al., 2017; Gopalswamy et al., 2018; Jian et al., 2018). At 1 au, CME velocities can exceed an impressive 2,000 km/s, CME magnetic fields can go over 100 nT, and the plasma densities have been recorded above 50 protons cm−3, that is ≈5–10 times larger than the ambient solar wind density values (Gopalswamy et al., 2009; Kilpua et al., 2017b). Previously observed flux ropes in FR had a simple structure within the lower corona (Jensen and Russell, 2008; Jensen et al., 2018). As CMEs expand into the solar wind, their structure becomes increasingly complex, and FETCH enables studying the structures of CMEs when this occurs. Measuring the plasma structure of CMEs and their evolution through space is crucial to predicting space weather as described using MHD models. As discussed earlier, space weather prediction is critical to protecting infrastructure, making this effort a significant safety issue. As we will discuss later, magnetic field measurements with FETCH are enhanced when a structure/disturbance is coherent and comparable in size to the line-of-sight; portions of it have magnetic fields oriented anti-/parallel to the line-of-sight, and it extends radially enough to characterize unique changes as it flows outward.
SIRs lasting more than one Carrington rotation are known as corotating interaction regions (CIRs). For the remainder of the paper, we refer more generally to SIRs. The geoeffectiveness of SIRs is variable; for example, they can cause longer geomagnetic storms than CME-driven events (Gonzalez et al., 1994; Chen et al., 2014), and their inclination could impact substorm intensity (Oliveira and Raeder, 2014). In situ SIR properties are sometimes observed to vary significantly between different spacecraft locations that are near the same heliocentric distance but at different helio-longitudes and helio-latitudes. For instance, while both STEREO A and B were located near 1 au from the Sun (with A and B spacecraft leading and following the Earth, respectively), multiple SIRs observed by the two spacecraft varied in profiles of velocity, magnetic field strength, density, and other parameters (Jian et al., 2019). The fast solar wind speed for the STEREO spacecraft are separated by tens of degrees in orbit that can differ from L1 spacecraft by up to 100 km/s, maximum magnetic field by 8 nT, and the peak proton density by 30 cm−3. Observation of such variability over large spatial scales would require either a large number of spacecraft for in situ measurements or the development of a remote-sensing technique as in FETCH.
MHD waves, such as Alfven and magnetosonic waves, have a significant role in the transfer of energy in the plasma of interplanetary space. Generated and modified by shocks and discontinuities, MHD waves provide important information on the plasma properties through which they propagate. Through both the magnetic field and density fluctuations, they are found in FR measurements where the FR plane of polarization angle fluctuates on a wide range of temporal and spatial scales. FR, the change in the plane of polarization along a signal’s propagation path, is due to the combined contribution of the electron density and magnetic field in the plasma through which the signal is passing. Distinguishing the cause of the FRF between the magnetic field and N-wave sources is benefited by comparison with fluctuations in the TEC. For example, frequently, the power in these FRFs is greater than the TEC fluctuations due to their MHD wave source, particularly if the waves are non-compressive (Hollweg et al., 1982 using Helios spacecraft).
The practice of detecting and analyzing MHD plasma waves with FRFs in interplanetary space is limited by the paucity of high time-resolution observations (e.g., MESSENGER spacecraft Jensen et al., 2013 and radio galaxies and quasars Sakurai and Spangler, 1994). With the available data, spectral analyses of FRFs have shown that the greatest amount of wave power is in the lowest frequencies with quasi-periodic fluctuations between 4 and 10 min: Efimov et al. (1993) and Chashei et al. (1999) both use Helios spacecraft and Wexler et al. (2017) uses the MESSENGER spacecraft. It is worth briefly summarizing here examples of the work that FETCH would be capable of with sufficient observation. With the Helios data (f = 2.3 GHz, λ = 13 cm), tantalizing details on mass and energy fluxes in the corona have emerged. Efimov et al. (2019) found little variation in turbulence with the solar cycle, but this contradicts the finding with MESSENGER (Jensen et al., 2013). Efimov et al. (2015) determined that the observed velocities were outward, the sum of the solar wind speed and Alfven velocity. Kooi et al. (2014) found possible evidence of Joule/wave heating of the coronal plasma. Various theoretical calculations suggest the benefits of these observations. For example, Hollweg et al. (2013) found reverse-flow Alfven waves to be weak, whereas oblique Alfven waves could drive MHD turbulence.
3 Science Traceability Matrix discussion
The pathway to achieving the objectives of the FETCH instrument can be described by a Science Traceability Matrix (STM). In this section, we explain the details of the technical requirements and project performance columns of the STM (Table 1). To understand the technical requirements, we need to establish the essential characteristics of two plasma observations collected with radio receivers, FR, and TEC. We then explain how interplanetary plasma structures of interest reveal themselves in these observables (STM-CME, STM-SIR, and STM-MHD).
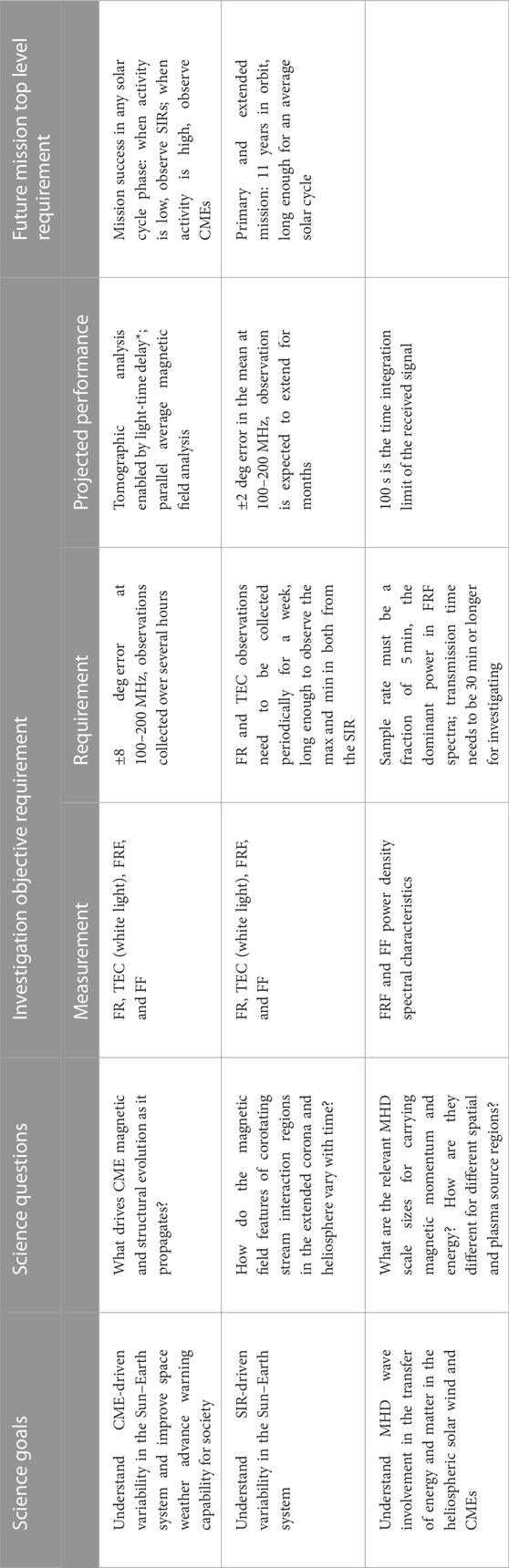
TABLE 1. Science Traceability Matrix for FETCH to support the MOST mission goal to understand the magnetic coupling of the Sun to the heliosphere. *Tomographic analysis approach comprises future work.
STM-CME: determining the minimum performance parameters for the FETCH instrument, ±8° in Table 1 for the plane of polarization resolution used three uniquely different sources. The first is an MHD model of the 2005 May 13 event (discussed imminently; Manchester et al., 2014). The second is the work by Howard (2011) looking at “typical” CME properties extrapolated from 1 au, which showed that a large (
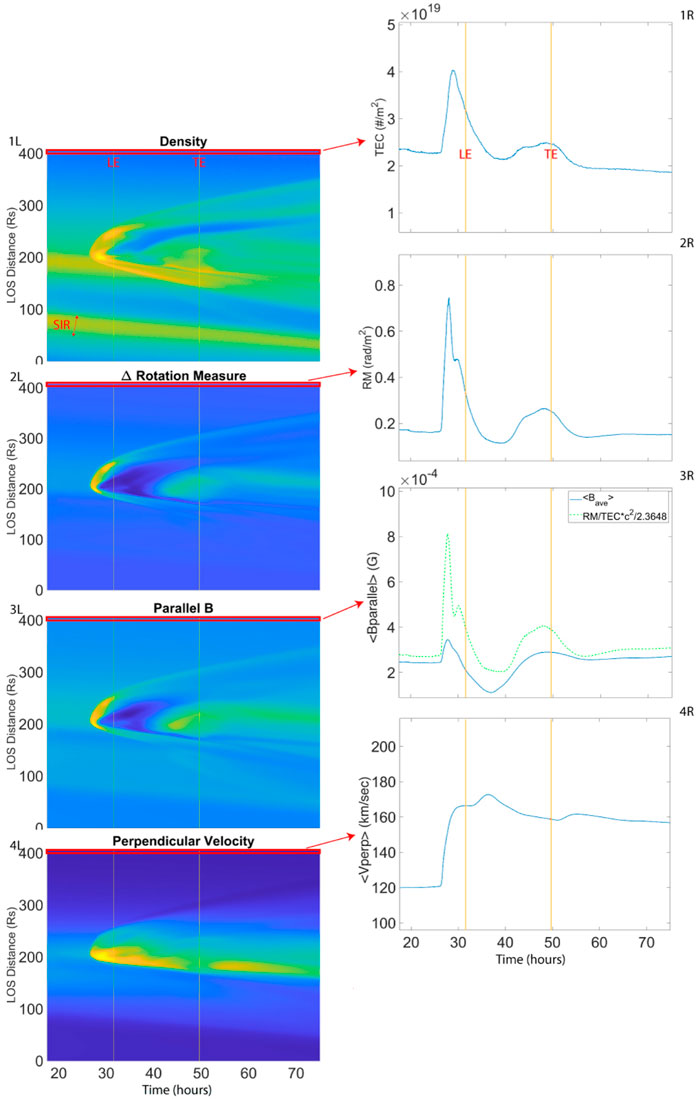
FIGURE 2. Simulated CME structure extracted along Line 3 (MOST 3→2) shown in a time series. The spatial distribution along the LOS of the density (panel 1L), the change in RM (panel 2L), the magnetic field parallel to the LOS (panel 3L), and the perpendicular velocity to the LOS (panel 4L) are shown to the left from the top to bottom, respectively, as functions of time. Here, the abscissa axis shows time with t = 0 since CME initiation in the AWSoM model, while the ordinate axis shows the distance along the LOS in solar radii. The plots to the right show LOS (integrated over the LOS distance) quantities. The red region is the location of the receiving spacecraft, and the arrow aids the reader to view the time profile collected at the location. From the top to bottom, respectively, we present the TEC (panel 1R), the RM observed at MOST2 (panel 2R), the average magnetic field (both modeled and calculated (panel 3R), and the average perpendicular velocity (panel 4R). LE and TE show the leading and trailing edges of the CME obtained from in situ analysis. The SIR is labeled.
STM-SIR: the expected FETCH results from probing stream interaction regions can be estimated by extracting the column density and LOS-FR time series from global heliospheric models. Figure 3 illustrates the L4–L5 LOS placement transverse to an SIR during the Carrington rotation 2109, using the AWSoM model as described by van der Holst et al. (2014). When the LOS is transverse to the SIR, the TEC is low, but TEC reaches a maximum as the SIR rotates across the LOS to approximately tangential orientation within the core of the SIR. From such a time series of TEC results, it is possible to estimate the density compression factor within the SIR. The FR curves show an asymmetrical temporal pattern with an initial steep change in FR accompanied by polarity reversal [see Borovsky (2020)], followed by a more gradual trail-off in the new polarity. These preliminary results suggest that FETCH will be valuable for evaluating inner heliosphere SIRs ahead of their arrival in the local geospace.
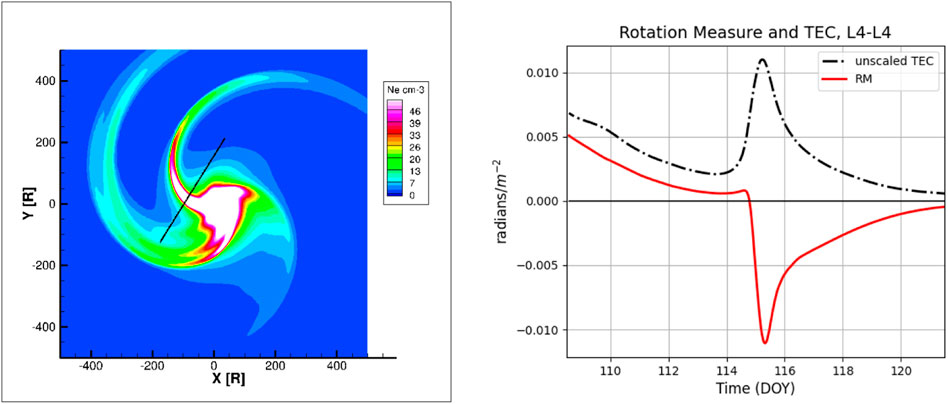
FIGURE 3. Left: AWSoM simulation of heliospheric density for CR2109. The spiral SIRs appear in enhanced density in the solar equatorial plane. The FETCH L4–L5 LOS (line 1 in Figure 1) is oriented approximately transverse to the SIR, where TEC reaches a minimum. Right: the FR and TEC (shown in solid red and black dot-dash, respectively) are observed as the rotation advancement brings the SIR across the FETCH L4–L5 LOS. An unscaled, concurrent TEC curve shows a similar placement of the peak but contrasts with the RM curve in its rate approaching zero.
STM-MHD: recall that the application of theory for analyzing the plasma waves detected is limited by the paucity of high time-resolution observations (e.g., MESSENGER spacecraft Jensen et al., 2013 and radio galaxies and quasars Sakurai and Spangler, 1994). However, spectral analyses of FRF have shown that the greatest amount of power spectral density is in periods between 4 and 10 min (Efimov et al., 1993; Chashei et al., 1999; both using Helios spacecraft). In analyzing Cassini FR data, Jensen and Russell (2007) found an integration limit of 100 s while minimizing error bars in the plane of polarization. Longer integrations lost coherence in the phase difference between the right- and left-handed polarizations; this is consistent with FRF changing the plane of polarization coherently (not randomly) with periods greater than 200 s. Investigating MHD waves with FR should be undertaken with 30 min of continuous observing to determine the maximum (4–10 min) wave power.
3.1 FETCH engineering design
The FETCH instrument has two transceiver channels for operation at two perpendicular linear polarizations and two frequencies. As shown in Figure 4 of the FETCH antenna design, the cross-dipole log-periodic antenna transmits and receives two polarizations, linearly in two perpendicular directions. The plane of polarization is measured using Stokes parameters, I, Q, U, and V, which fully characterize the signal and are measured from the real and imaginary signal amplitudes received by the two orthogonal antenna elements. We have tested the processing system by modeling low signal-to-noise ratio (SNR) conditions on the received signal in the two polarizations. Our initial Simulink simulation of the transceiver system was receiving the transmitted rotation in polarization. The total expected data needed for all four FETCH antennas combined are 22 MB per day for both measurements and ancillary/housekeeping data. FR resolution is a function of the background noise and the polarization of the signal relative to the orientations of the two receiving cross-dipole elements. The largest source of noise is the Milky Way galaxy. The notional antenna design was selected for its wide main beam, which enabled both spacecraft across the Earth–Sun line to be encompassed. This said, the receiving spacecraft, in two transmission beams, will distinguish between them due to their sufficiently different narrowband frequencies. The two frequencies enable separating Doppler motion from plasma effects, and they also enable distinguishing spacecraft polarization changes from those induced by the plasma. The plasma effects all vary with frequency, whereas the spacecraft’s position and orientation do not. The technology for FETCH signal processing is well established.
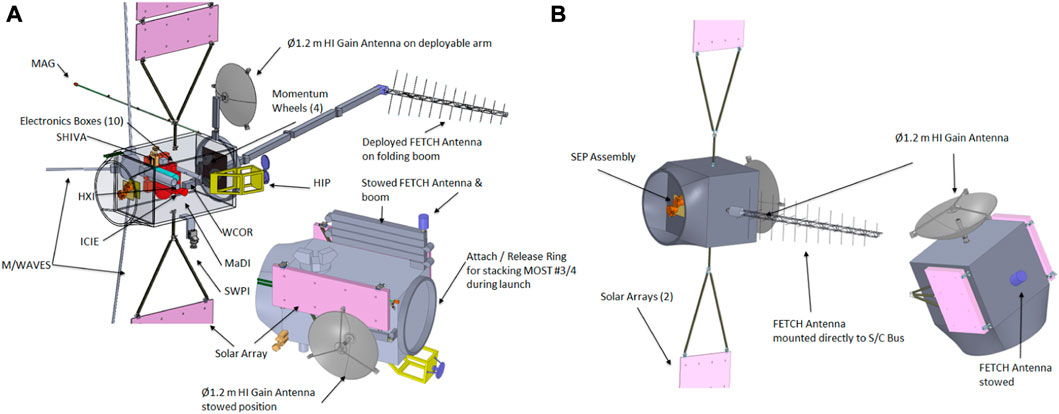
FIGURE 4. (A) MOST1 and 2 with the full instrument suite. (B) MOST3 and 4 with FETCH elements. See Gopalswamy et al., 2022 for details about the other instruments. The FETCH antenna is a log-periodic cross-dipole antenna extending approximately 3 m on its axis. The elements are illustrative only. The antenna boom on MOST1 and 2 is necessary to keep it out of the field of view of imagers.
FETCH is based on mature VHF technology, but the engineering design of the system is in the process of being optimized. There is no technology risk in which all of the individual components have been commercialized and fabrication facilities already exist; however, the solution space with respect to various engineering design parameters includes defining the exact frequencies, signal processing (e.g., pulse), and other antenna parameters. Using the NASA development descriptions in technology readiness level (TRL), FETCH components are in varying TRLs from its heritage technology roots in the Department of Energy Fast On-orbit Recording of Transient Event (FORTE) mission antenna (Huang and Roussel-Dupré, 2005) to its significant performance challenges that need to be met in 2 au of path length. Cost estimates were performed by qualitatively comparing the tasks required to reach a successfully demonstrated final product (TRL-8) against the typical funding available for those tasks. We estimate that this will take 4 years and cost 4.8 M. However, note that components with low permissible variation limits and tolerances may come with a significant cost. A 1M unexpected equipment cost (contingency) is possible due to these kinds of constraints occurring.
3.2 Summary and conclusion
Magnetic fields are of critical importance to heliospheric physics from wave heating to CMEs that drive space weather, yet observations to date have been limited to extremely sparse in situ observations and even more infrequent FR observations made with radio galaxies and other non-solar polarized sources (Kooi et al., 2022d). Only two spacecraft missions have had the conjunction of availability and resources for Faraday rotation fluctuation observation (Helios and MESSENGER, see Section 2). To vastly increase the availability of IMF observations, we propose the multi-spacecraft instrument FETCH, which is capable of nearly continuous FR-FRF observations along four separate lines of sight crossing the Sun–Earth line and passing from ≈0.14 to 0.5 au of the Sun. Viewing the solar wind plasma upstream of the Earth from an unobstructed perspective enables space weather studies, particularly of CMEs and SIRs. FETCH enables observing the radial profile of these disturbances, their internal magnetic structure, and their evolution. FETCH is an essential instrument for advancing space weather research. In future work, we will develop the rudimentary tomographic analysis enabled by the 2D + time FETCH configuration. The 3D reconstruction is a function of 1) radio signals sensitive to plasma structures larger than the Fresnel zone, 2) the light–time delay of signal propagation, 3) flow characteristics of the plasma, 4) coherence with distance both radially and azimuthally, and 5) a 3D CME or SIR plasma model using these boundary conditions to restrict the solution space. The FETCH configuration enables both coarse and fine tomographic reconstructions of the electron density and magnetic field strength, which enables us to decouple the plasma and magnetic field contributions to the FR signal in the ecliptic plane.
FETCH observations are essential for addressing NASA’s heliophysics science goal to “explore the physical processes at work in the space environment from the Sun to Earth and throughout the Solar System.” The MOST mission goal is “to understand the magnetic coupling of the Sun to the heliosphere.” FETCH is one of ten instruments comprising the MOST mission. Using the phenomenon of FR in conjunction with other radio propagation effects, FETCH enables the detection of the magnetic field within CMEs, SIRs, and MHD waves. CMEs and SIRs are events/structures important to Earth’s space weather. MHD waves not only transfer energy in the heliosphere but also convey important information through the medium.
Data availability statement
The raw data supporting the conclusion of this article will be made available by the authors, without undue reservation.
Author contributions
EJ was responsible for the organization of this article and contributed to all sections. LW provided feedback on design limitations and specifications of FETCH, in addition to knowledge of typical solar wind and CME parameters. TN-C provided feedback on the definition of the FETCH goals and contributed to all sections. DW Science Traceability Matrix discussion. NG contributed to all sections. DW contributed to all sections. JK contributed to all sections. MK contributed to Section 3. SF contributed and provided feedback to the Science Traceability Matrix, engineering design, science operational configuration, and determining the feasibility of the FETCH instrument. BW contributed to Section 3. SB contributed to Section 3. LJ contributed to Section 3. JL contributed to Section 3. AP contributed to all sections except Section 5 (FETCH engineering design). WM contributed to all sections except Section 5 (5 FETCH engineering design). MD contributed to Section 3.1. LL contributed to Section 3.1. LP contributed to Section 3.1. MS contributed to Section 3.1. BJ contributed to Section 2 and Section 3.
Funding
EJ was supported by ACS Engineering & Safety LLC for this project. Goddard Space Flight Center grant 80NSSC21K1991 supported the software purchases for this work. WM is supported by the NSF PRE-EVENTS grant no. 1663800 and the NSF SWQU grant no. PHY-2027555. High-performance computing support for this simulation was provided by the NSF/NCAR Yellowstone supercomputer. Basic research at the U.S. Naval Research Laboratory (NRL) is supported by 6.1 Base funding. LW was partially funded by Wind MO&DA funds (no grant numbers for civil servants). TN-C was partially funded by the Solar Orbiter program and Heliophysics Internal Funding 2022 (HIF 2022). This material is based on work supported by the National Science Foundation Graduate Research Fellowship under grant no. 1650115.
Acknowledgments
The authors would like to thank NASA/Goddard Space Flight Center and the Green Bank Telescope Observatory for supporting the science and technology behind this work. They would like to thank the Frontiers reviewer for their general audience technical writing suggestions.
Conflict of interest
The authors declare that the research was conducted in the absence of any commercial or financial relationships that could be construed as a potential conflict of interest.
Publisher’s note
All claims expressed in this article are solely those of the authors and do not necessarily represent those of their affiliated organizations, or those of the publisher, the editors, and the reviewers. Any product that may be evaluated in this article, or claim that may be made by its manufacturer, is not guaranteed or endorsed by the publisher.
References
Borovsky, J. E. (2020). What magnetospheric and ionospheric researchers should know about the solar wind. J. Atmos. Solar-Terrestrial Phys. 204, 105271. doi:10.1016/j.jastp.2020.105271
Chashei, I. V., Bird, M. K., Efimov, A. I., Andreev, V. E., and Samoznaev, L. N. (1999). Five-minute magnetic field fluctuations in the solar wind acceleration region. Sol. Phys. 189, 399–412. doi:10.1023/A:1005223531849
Chen, G.-m., Xu, J., Wang, W., and Burns, A. G. (2014). A comparison of the effects of CIR- and CME-induced geomagnetic activity on thermospheric densities and spacecraft orbits: Statistical studies. J. Geophys. Res. (Space Phys. 119, 7928–7939. doi:10.1002/2014JA019831
Chen, P. F. (2011). Coronal mass ejections: Models and their observational basis. Living Rev. Sol. Phys. 8, 1. doi:10.12942/lrsp-2011-1
Council, N. R. (2008). Severe space weather events: Understanding societal and economic impacts: A workshop report. Washington, DC: The National Academies Press. doi:10.17226/12507
Eastwood, J. P., Biffis, E., Hapgood, M. A., Green, L., Bisi, M. M., Bentley, R. D., et al. (2017). The economic impact of space weather: Where do we stand? Risk Anal. 37, 206–218. doi:10.1111/risa.12765
Efimov, A. I., Chashei, I. V., Shishov, V. I., and Bird, M. K. (1993). Faraday-rotation fluctuations during radio occultation of the circumsolar plasma. Astron. Lett. 19, 57.
Efimov, A. I., Lukanina, L. A., Chashei, I. V., Bird, M. K., and Pätzold, M. (2019). Solar wind magnetic field turbulence over the solar activity cycle inferred from coronal sounding experiments with helios linear-polarized signals. Astron. Rep. 63, 174–181. doi:10.1134/s106377291903003x
Efimov, A. I., Lukanina, L. A., Rogashkova, A. I., Samoznaev, L. N., Chashei, I. V., Bird, M. K., et al. (2015). Faraday-rotation fluctuations from radio-sounding measurements of the circumsolar plasma using polarized signals from the HELIOS-1 and HELIOS-2 space probes. Astron. Rep. 59, 313–326. doi:10.1134/S1063772915040022
Efimov, A. I., Samoznaev, L. N., Andreev, V. E., Chashei, I. V., and Bird, M. K. (2000). Quasi-harmonic faraday-rotation fluctuations of radio waves when sounding the outer solar corona. Astron. Lett. 26, 544–552. doi:10.1134/1.1306991
Fung, S. F., Benson, R. F., Galkin, I. A., Green, J. L., Reinisch, B. W., Song, P., et al. (2022). “Chapter 4 - radio-frequency imaging techniques for ionospheric, magnetospheric, and planetary studies,” in Understanding the space environment through global measurements. Editors Y. Colado-Vega, D. Gallagher, H. Frey, and S. Wing (Elsevier). 101–216. doi:10.1016/B978-0-12-820630-0.00006-4
Gonzalez, W. D., Joselyn, J. A., Kamide, Y., Kroehl, H. W., Rostoker, G., Tsurutani, B. T., et al. (1994). What is a geomagnetic storm? J. Geophys. Res. 99, 5771–5792. doi:10.1029/93ja02867
Gopalswamy, N., Akiyama, S., Yashiro, S., and Xie, H. (2018). Coronal flux ropes and their interplanetary counterparts. J. Atmos. Sol.-Terr. Phys. 180, 35–45. doi:10.1016/j.jastp.2017.06.004
Gopalswamy, N., Christe, S., Fung, S. F., Gong, Q., Jian, L., Kanekal, S. G., et al. (2022). “The Multiview observatory for solar terrestrial science (MOST),” in The decadal survey for solar and space physics (Heliophysics), 2024–2033. Submitted White Paper.
Gopalswamy, N. (2016). History and development of coronal mass ejections as a key player in solar terrestrial relationship. Geosci. Lett. 3, 8. doi:10.1186/s40562-016-0039-2
Gopalswamy, N., Yashiro, S., Michalek, G., Stenborg, G., Vourlidas, A., Freeland, S., et al. (2009). The SOHO/LASCO CME catalog. Earth Moon Planets 104, 295–313. doi:10.1007/s11038-008-9282-7
Hollweg, J. V., Bird, M. K., Volland, H., Edenhofer, P., Stelzried, C. T., and Seidel, B. L. (1982). Possible evidence for coronal Alfven waves. J. Geophys. Res. 87, 1. doi:10.1029/JA087iA01p00001
Hollweg, J. V., Cranmer, S. R., and Chandran, B. D. G. (2010). Coronal Faraday rotation fluctuations and a wave/turbulence-driven model of the solar wind. Astrophys. J. 722, 1495–1503. doi:10.1088/0004-637X/722/2/1495
Hollweg, J. V., Kaghashvili, E. K., and Chandran, B. D. G. (2013). Velocity-shear-induced mode coupling in the solar atmosphere and solar wind: Implications for plasma heating and MHD turbulence. Astrophys. J. 769, 142. doi:10.1088/0004-637x/769/2/142
Howard, T. (2011). Coronal mass ejections: An introduction, 376. Springer. doi:10.1007/978-1-4419-8789-1
Huang, Z., and Roussel-Dupré, R. (2005). Total electron content (TEC) variability at los alamos, new Mexico: A comparative study: FORTE-derived TEC analysis. Radio Sci. 40, RS6007. doi:10.1029/2004rs003202
Imamura, T., Tokumaru, M., Isobe, H., Shiota, D., Ando, H., Miyamoto, M., et al. (2014). Outflow structure of the quiet sun corona probed by spacecraft radio scintillations in strong scattering. Astrophys. J. 788, 117. doi:10.1088/0004-637X/788/2/117
Jensen, E. A., Frazin, R., Heiles, C., Lamy, P., Llebaria, A., Anderson, J. D., et al. (2016). The comparison of total electron content between radio and thompson scattering. Sol. Phys. 291, 465–485. doi:10.1007/s11207-015-0834-5
Jensen, E. A., Heiles, C., Wexler, D., Kepley, A. A., Kuiper, T., Bisi, M. M., et al. (2018). Plasma interactions with the space environment in the acceleration region: Indications of CME-trailing reconnection regions. Astrophys. J. 861, 118. doi:10.3847/1538-4357/aac5dd
Jensen, E. A., Hick, P. P., Bisi, M. M., Jackson, B. V., Clover, J., and Mulligan, T. (2010). Faraday rotation response to coronal mass ejection structure. Sol. Phys. 265, 31–48. doi:10.1007/s11207-010-9543-2
Jensen, E. A. (2007). High frequency Faraday rotation observations of the solar corona. Los Angeles, United States: Ph.D. thesis, University of California.
Jensen, E. A., Nolan, M., Bisi, M. M., Chashei, I., and Vilas, F. (2013). MESSENGER observations of magnetohydrodynamic waves in the solar corona from Faraday rotation. Sol. Phys. 285, 71–82. doi:10.1007/s11207-012-0162-y
Jensen, E. A., and Russell, C. T. (2008). Faraday rotation observations of CMEs. Geophys. Res. Lett. 35, L02103. doi:10.1029/2007GL031038
Jensen, E. A., and Russell, C. T. (2007). “Measuring the plane of polarization in a strongly circular signal,” in Solar physics and space weather instrumentation II. Editors S. Fineschi, and R. A. Viereck, 6689, 668910. of Society of Photo-Optical Instrumentation Engineers (SPIE) Conference Series. doi:10.1117/12.734860
Jian, L. K., Luhmann, J. G., Russell, C. T., and Galvin, A. B. (2019). Solar terrestrial Relations observatory (STEREO) observations of stream interaction regions in 2007 - 2016: Relationship with heliospheric current sheets, solar cycle variations, and dual observations. Sol. Phys. 294, 31. doi:10.1007/s11207-019-1416-8
Jian, L. K., MacNeice, P. J., Taktakishvili, A., Odstrcil, D., Jackson, B., Yu, H. S., et al. (2015). Validation for solar wind prediction at Earth: Comparison of coronal and heliospheric models installed at the CCMC. Space weather. 13, 316–338. doi:10.1002/2015SW001174
Jian, L. K., Russell, C. T., Luhmann, J. G., and Galvin, A. B. (2018). STEREO observations of interplanetary coronal mass ejections in 2007-2016. Astrophysical J. 855, 114. doi:10.3847/1538-4357/aab189
Jian, L., Russell, C. T., Luhmann, J. G., and Skoug, R. M. (2006). Properties of interplanetary coronal mass ejections at one AU during 1995 2004. Sol. Phys. 239, 393–436. doi:10.1007/s11207-006-0133-2
Kaiser, M. L., Kucera, T. A., Davila, J. M., Cyr, St.O. C., Guhathakurta, M., and Christian, E. (2008). The STEREO mission: An introduction. Space Sci. Rev. 136, 5–16. doi:10.1007/s11214-007-9277-0
Kilpua, E. K. J., Balogh, A., von Steiger, R., and Liu, Y. D. (2017b). Geoeffective properties of solar transients and stream interaction regions. Space Sci. Rev. 212, 1271–1314. doi:10.1007/s11214-017-0411-3
Kilpua, E. K. J., Good, S. W., Ala-Lahti, M., Osmane, A., Pal, S., Soljento, J. E., et al. (2022). Structure and fluctuations of a slow ICME sheath observed at 0.5 au by the Parker Solar Probe. Astronomy Astrophysics 663, A108. doi:10.1051/0004-6361/202142191
Kilpua, E., Koskinen, H. E. J., and Pulkkinen, T. I. (2017a). Coronal mass ejections and their sheath regions in interplanetary space. Living Rev. Sol. Phys. 14, 5. doi:10.1007/s41116-017-0009-6
Kooi, J. E., Ascione, M. L., Reyes-Rosa, L. V., Rier, S. K., and Ashas, M. (2021). VLA measurements of Faraday rotation through a coronal mass ejection using multiple lines of sight. Sol. Phys. 296, 11. doi:10.1007/s11207-020-01755-4
Kooi, J. E., Fischer, P. D., Buffo, J. J., and Spangler, S. R. (2014). Measurements of coronal Faraday rotation at 4.6 R ⊙. Astrophys. J. 784, 68. doi:10.1088/0004-637X/784/1/68
Kooi, J. E., Wexler, D. B., Jensen, E. A., Kenny, M. N., Nieves-Chinchilla, T., Wilson, I., et al. (2022a). Modern Faraday rotation studies to probe the solar wind. Front. Astron. Space Sci. 9, 841866. doi:10.3389/fspas.2022.841866
Kooi, J. E., Wexler, D. B., Jensen, E. A., Wood, B. E., Nieves-Chinchilla, T., Manchester, W. B., et al. (2022c). “Faraday rotation methods to detect coronal currents and MHD wave activity,” in The decadal survey for solar and space physics (Heliophysics) 2024-2033. Submitted White Paper.
Kooi, J. E., Wexler, D. B., Jensen, E. A., Wood, B. E., Nieves-Chinchilla, T., Manchester, W. B., et al. (2022e). “How to advance studies of coronal Faraday rotation,” in The decadal survey for solar and space physics (Heliophysics) 2024-2033. Submitted White Paper.
Kooi, J. E., Wexler, D. B., Jensen, E. A., Wood, B. E., Nieves-Chinchilla, T., Manchester, W. B., et al. (2022b). “Probing the magnetic field structure of coronal mass ejections with Faraday rotation,” in The decadal survey for solar and space physics (Heliophysics) 2024-2033. Submitted White Paper.
Kooi, J. E., Wexler, D. B., Jensen, E. A., Wood, B. E., Nieves-Chinchilla, T., Manchester, W. B., et al. (2022d). “Progressing towards Faraday rotation tomography of the solar wind,” in The decadal survey for solar and space physics (Heliophysics) 2024-2033. Submitted White Paper.
Liu, Y., Manchester, W. B., IV, Kasper, J. C., Richardson, J. D., and Belcher, J. W. (2007). Determining the magnetic field orientation of coronal mass ejections from Faraday rotation. Astrophys. J. 665, 1439–1447. doi:10.1086/520038
Manchester, W. B., van der Holst, B., and Lavraud, B. (2014). Flux rope evolution in interplanetary coronal mass ejections: The 13 may 2005 event. Plasma Phys. Control Fusion 56, 064006–064011. doi:10.1088/0741-3335/56/6/064006
Manchester, W., Kilpua, E. K. J., Liu, Y. D., Lugaz, N., Riley, P., Török, T., et al. (2017). The physical processes of CME/ICME evolution. Phys. Process. CME/ICME Evol. 212, 1159–1219. doi:10.1007/s11214-017-0394-0
Oliveira, D. M., and Raeder, J. (2014). Impact angle control of interplanetary shock geoeffectiveness. J. Geophys. Res. (Space Phys. 119, 8188–8201. doi:10.1002/2014JA020275
Oughton, E. J., Skelton, A., Horne, R. B., Thomson, A. W. P., and Gaunt, C. T. (2017). Quantifying the daily economic impact of extreme space weather due to failure in electricity transmission infrastructure. Space weather. 15, 65–83. doi:10.1002/2016sw001491
Panchenko, M., Macher, W., Rucker, H. O., Fischer, G., Oswald, T. H., Cecconi, B., et al. (2014). In-flight calibration of STEREO-B/WAVES antenna system. Radio Sci. 49, 146–156. doi:10.1002/2013rs005197
Sakurai, T., and Spangler, S. R. (1994). The study of coronal plasma structures and fluctuations with Faraday rotation measurements. Astrophys. J. 434, 773. doi:10.1086/174780
Suzuki, T. K., and Inutsuka, S.-i. (2005). Making the corona and the fast solar wind: A self-consistent simulation for the low-frequency alfvén waves from the photosphere to 0.3 au. Astrophysical J. Lett. 632, L49–L52. doi:10.1086/497536
Temmer, M. (2021). Space weather: The solar perspective. Living Rev. Sol. Phys. 18, 4. doi:10.1007/s41116-021-00030-3
van der Holst, B., Sokolov, I. V., Meng, X., Jin, M., Manchester, W. B., Tóth, G., et al. (2014). Alfvén wave solar model (AWSoM): Coronal heating. Astrophys. J. 782, 81. doi:10.1088/0004-637X/782/2/81
Viall, N. M., and Borovsky, J. E. (2020). Nine outstanding questions of solar wind physics. J. Geophys. Res. (Space Phys. 125, e2018JA026005. doi:10.1029/2018JA026005
Wexler, D. B., Jensen, E. A., Hollweg, J. V., Heiles, C., Efimov, A. I., Vierinen, J., et al. (2017). Faraday rotation fluctuations of MESSENGER radio signals through the equatorial lower corona near solar minimum. Space weather. 15, 310–324. doi:10.1002/2016SW001558
Wexler, D., Jensen, E., Gopalswamy, N., Wilson, L., Fung, S., Nieves-Chinchilla, T., et al. (2021). “FETCH concept: Investigating quiescent and transient magnetic structures in the inner heliosphere using Faraday rotation of spacecraft radio signals,” in AGU fall meeting abstracts, 2021. SH31A–05.
Woo, R., Yang, F. C., Yip, K. W., and Kendall, W. B. (1976). Measurements of large-scale density fluctuations in the solar wind using dual-frequency phase scintillations. Astrophys. J. 210, 568–574. doi:10.1086/154861
Wood, B. E., Kooi, J. E., Palmerio, E., Gibson, S. E., Lynch, B. J., Winslow, R. M., et al. (2022). “Sensing CME magnetic fields en route to 1 AU,” in The decadal survey for solar and space physics (Heliophysics) 2024-2033. Submitted White Paper.
Keywords: solar corona, coronal magnetic fields, coronal plasma density, coronal mass ejection, polarimetry, Faraday rotation (FR), MHD waves, space weather
Citation: Jensen EA, Gopalswamy N, Wilson LB III, Jian LK, Fung SF, Nieves-Chinchilla T, Shelton M, Li L, Deshpande M, Purves L, Lazio J, Manchester WB IV, Wood BE, Kooi JE, Wexler DB, Bale S, Pevtsov A, Jackson BV and Kenny MN (2023) The Faraday Effect Tracker of Coronal and Heliospheric Structures (FETCH) instrument. Front. Astron. Space Sci. 10:1064069. doi: 10.3389/fspas.2023.1064069
Received: 07 October 2022; Accepted: 30 March 2023;
Published: 17 April 2023.
Edited by:
Benoit Lavraud, UMR5804 Laboratoire d’astrophysique de Bordeaux (LAB), FranceReviewed by:
Evangelia Samara, KU Leuven, BelgiumCopyright © 2023 Jensen, Gopalswamy, Wilson, Jian, Fung, Nieves-Chinchilla, Shelton, Li, Deshpande, Purves, Lazio, Manchester, Wood, Kooi, Wexler, Bale, Pevtsov, Jackson and Kenny. This is an open-access article distributed under the terms of the Creative Commons Attribution License (CC BY). The use, distribution or reproduction in other forums is permitted, provided the original author(s) and the copyright owner(s) are credited and that the original publication in this journal is cited, in accordance with accepted academic practice. No use, distribution or reproduction is permitted which does not comply with these terms.
*Correspondence: Elizabeth A. Jensen, ZWplbnNlbkBwc2kuZWR1